- 1Departamento de Farmacología Otto Orsingher, Facultad de Ciencias Químicas, Universidad Nacional de Córdoba, Córdoba, Argentina
- 2Instituto de Farmacología Experimental de Córdoba-Consejo Nacional de Investigaciones Científicas y Técnicas (IFEC-CONICET), Facultad de Ciencias Químicas, Universidad Nacional de Córdoba, Córdoba, Argentina
Caenorhabditis elegans (C. elegans) is a model organism widely used to evaluate the mechanistic aspects of toxicants with the potential to predict responses comparable to those of mammals. We report here the consequences of developmental lead (Pb) exposure on behavioral responses to ethanol (EtOH) in C. elegans. In addition, we present data on morphological alterations in the dopamine (DA) synapse and DA-dependent behaviors aimed to dissect the neurobiological mechanisms that underlie the relationship between these neurotoxicants. Finally, the escalation to superior animals that parallels the observed effects in both experimental models with references to EtOH metabolism and oxidative stress is also discussed. Overall, the literature revised here underpins the usefulness of C. elegans to evidence behavioral responses to a combination of neurotoxicants in mechanistic-orientated studies.
Introduction
As a model organism first described by Brenner in 1973 (Brenner, 1973), Caenorhabditis elegans (C. elegans) is a suitable model to study the neurobiological basis of toxicity. This small living invertebrate has provided invaluable evidence for the neurotoxic mechanisms of several elements, including lead (Pb) (Chen et al., 2013; Jiang et al., 2016; Soares et al., 2017). Additionally, many responses to ethanol (EtOH), not only in movement-related behaviors, but also in other parameters, have revealed its potential to study the neurobiological bases of drugs of abuse (Grotewiel and Bettinger, 2015; Engleman et al., 2016; Katner et al., 2019).
Thus, in the present review, we intended to provide evidence to support the role of C. elegans as a prominent organism for the study of the mechanisms underpinning the adverse effects of Pb and the specific factors involved in the vulnerability to EtOH addiction (Bettinger and Davies, 2014; Engleman et al., 2016; Khanh, 2018). Furthermore, its potential translational value from studies in mammals was also considered.
C. elegans as a model in toxicological studies
This transparent, free-living nematode has the potential to predict responses comparable to mammals (Anderson et al., 2004; Hunt et al., 2018; Meneely et al., 2019), without the ethical issues involved in higher animal experimentation (Casey et al., 2015). The simplicity of the cell lineage and the existence of a variety of transgenic animals determine that C. elegans has become a model widely used in toxicity tests to evaluate the mechanistic aspects of a myriad of substances (Hunt et al., 2020). Its well-described nervous system allows for studying the cellular mechanisms that underlie neurodegeneration (Caldwell et al., 2020), including Parkinson’s disease (Maulik et al., 2017; Cooper and van Raamsdonk, 2018; Gaeta et al., 2019) and Alzheimer’s diseases (Paul et al., 2020; Y. Wu and Luo, 2005), along with the pesticides and metals possibly involved in their etiology or progression (Gonzalez-Hunt et al., 2014; Soares et al., 2017; Sedensky and Morgan, 2018; Martins et al., 2022). To this end, manganese, mercury, and Pb in particular are the metals considered the most potentially hazardous to human health (Avila et al., 2016; Caito and Aschner, 2016; Lu et al., 2018; Akinyemi et al., 2019).
Lead
This toxic, persistent, and non-essential metal that accumulates in the environment and living organisms induces damage to all systems, including the central nervous system (Virgolini and Aschner, 2021). Early-life Pb exposure may cause an imprint that can be evident later in life or in response to a variety of challenges (Silbergeld, 1992; Mitra et al., 2017; Vorvolakos et al., 2016; Virgolini et al., 2019). At the functional level, many neurotransmitters have been studied after Pb exposure (Xing et al., 2009a; Xing et al., 2009b; Sudama et al., 2013), with the deleterious effects on DAergic neurons among the best described in different experimental models (Zuch et al., 1998; NourEddine et al., 2005; Szczerbak et al., 2007).
Several studies show that short periods of Pb exposure during adulthood (less than 12 h) resulted in decreased locomotion (Boyd et al., 2003; Wang and Xing, 2009), changes in movement patterns (Wang and Xing, 2008), or reduced feeding behavior (Anderson et al., 2001, 2004). Other reports indicate that acute exposure to this metal decreased memory (Ye et al., 2008) or associative learning in a thermotaxis assay (Zhang et al., 2010), effects that are reversed by the pretreatment with antioxidant agents such as dimethyl sulfoxide (DMSO) or N-acetylcysteine (NAC) (Wu et al., 2012). These results suggest that oxidative stress could be involved in the mechanism of neurotoxicity exerted by this metal (Virgolini and Aschner, 2021). Importantly, Guo et al. observed defects in the reproductive capacity of the worm (decreased egg-laying numbers and lengthened generation of progeny), alterations present across all stages, with developing larvae being more vulnerable to Pb than young adults (Guo et al., 2009).
Regarding long exposures, Tiwari et al. inform alterations not only in locomotor activity, but also in the growth pattern in nematodes exposed to sublethal Pb concentrations (3 μm, 15 μm, or 30 μm Pb) for 24 h. In these studies, they reported dose-dependent alterations in reverse movements, a decrease in body length, and an increase in the peristaltic velocity (Tiwari et al., 2020). In this line, other researchers reported reduced body bends, head movements, and reverse movements after Pb exposure, mitigated by pretreatment with selenium (Li et al., 2013).
In terms of chronic treatments, Wang and Yang (2007) showed that sustained exposure to Pb for 3 days induces multiple dose-dependent biological effects in the nematode, including shortened half-life, decreased body size, reproductive abnormalities, and defects in the function of the nervous and muscular systems, with many effects transferable to the progeny (Wang and Yang, 2007). In the same line, Yu et al. reported growth inhibition and changes in movement patterns, which turned out to be more evident in the second generation, data that reinforces the importance of the developmental stage at which Pb exposure occurs (Yu et al., 2013). Interestingly, Pb-induced changes in growth, feeding, and reproduction persisted for up to four generations and may even be more noticeable in the last one (Yu et al., 2016). Moreover, transgenerational alterations in parameters such as growth rate, motility, feeding, and/or reproduction have been reported when Pb exposure occurred during stages spanning gonad and egg development (Wang and Yang, 2007; Yu et al., 2013, 2016).
Regarding behavioral alterations, Sun et al. (2016) reported a decrease in locomotor activity as well as a shorter lifespan in worms exposed for 36 h to 8.5 μM Pb(NO3)2, from the L1 stage to the adult L4 (Sun et al., 2016). Interestingly, Monteiro et al. (2014) observed the opposite phenomenon: exposure to moderate to high Pb doses for 4 days reduced larval movement and reproduction (Monteiro et al., 2014). However, after low concentrations (less than 0.5 µm), a stimulatory effect on reproduction and growth was observed, possibly due to survival or a dispersal strategy manifested in a stressful environment (Roh et al., 2006; Monteiro et al., 2014). This behavior may be indicative of hormesis, a phenomenon that occurs when low concentrations of a toxicant elicit an adaptive response, which is stimulant in this case, protecting the organism against subsequent exposures to higher doses of the same pollutant (Wang and Xing, 2009; Zhao and Wang, 2012).
Overall, although still scarce, the reported evidence demonstrates that C. elegans is a suitable model to study the adverse effects of Pb exposure in immature and adult organisms in terms of developmental neurotoxicity (Ruszkiewicz et al., 2018) and transgenerational studies (Zhao et al., 2022).
Ethanol
Ethanol (EtOH) is an easily accessible drug of abuse that induces biphasic responses in living organisms depending on its metabolism and effects on the CNS (Pohorecky, 1977; Hendler et al., 2013; Virgolini and Pautassi, 2022). In humans, acute exposure to low EtOH doses induces hyperactivity and euphoria, mild doses are anxiolytic, while high exposures cause impaired coordination and balance, sedation, and even death (Zhu et al., 2014). Although these behaviors can be a sensitive indicator of toxicity, they are a complex phenomenon hard to quantify in higher organisms. Based on these considerations, C. elegans allows the assessment of simple behaviors that are shown as alterations in locomotion and measurable as changes in speed or direction closely related to behaviors observed in humans. It has been demonstrated that worms exposed to EtOH evidenced initial hyperactivity followed by immobility, which is reversed when EtOH exposure ceased (Wu et al., 2019). Similarly, low EtOH concentrations (17–52 mM) produce hyperactivity, whereas amounts between 100 and 400 mM decrease motility (Dhawan et al., 1999; Morgan and Sedensky, 1995; reviewed in Scholz and Mustard, 2013). In addition, acute exposure to this drug induces a dose-dependent depression in locomotion and egg-laying behavior at comparable internal EtOH concentrations known to induce intoxication in humans and other mammals (Alaimo et al., 2012).
Furthermore, this nematode shows two well-described behaviors in vertebrates known as tolerance and sensitization that are distinctive in humans in response to excessive consumption of psychoactive substances (Lee et al., 2009; Bettinger and Davies, 2014; Grotewiel and Bettinger, 2015). Interestingly, continuous exposure to EtOH generates the development of a behavioral phenomenon representative of neuronal plasticity called “acute functional tolerance” (AFT) (Davies and McIntire, 2004), first described in rodents (LeBlanc et al., 1975). This behavior is evident when worms recover part of their mobility after the decrease in the speed of movement or locomotion on a solid agar surface as a consequence of the exposure to high EtOH concentrations (Raabe et al., 2014; Davies et al., 2015). Notably, both neuronal plasticity and the mechanisms underlying AFT are considered a compensatory response to the environmental insult elicited by the actions of EtOH (Raabe et al., 2014).
In addition to the above-described behavior, C. elegans exhibits the fundamental features of EtOH withdrawal symptoms reported in higher animals, including humans (Scott et al., 2017). Several behaviors modified by EtOH withdrawal can be partially or fully reversed by re-exposure to a low EtOH dose (Scott et al., 2017). In this regard, McIntire (2010) demonstrated that during EtOH abstinence the worms showed altered posture and impaired ability to direct themselves towards food (McIntire, 2010). These and others researchers (Crowder, 2004) also reported the involvement of slo-1, a highly conserved gene encoding for the calcium- and voltage-gated long-conductance K channel (also called BK potassium channel or SLO-1, homologous to the same proteins in humans). Interestingly many other responses associated with EtOH also appear to be modulated by the expression of this gene (Davies et al., 2003; Scott et al., 2017).
Finally, in a recent work, Sterken et al. (2021) studied the time-course transcriptional modifications of EtOH exposure. They reported that 400 mM EtOH induced transcriptional profiles in many genes at long exposure periods. Oppositely, short exposures to EtOH (up to 2 h) induced the expression of enzymes involved in its metabolism, particularly ADH, the enzyme involved in EtOH oxidation to acetaldehyde. On the other side, longer exposures (8 h or more) had much more profound effects on the transcriptome and genes involved in neuronal function, lipid microenvironment, and physiological responses to EtOH, including direct targets of this drug (Sterken et al., 2021).
Overall, this evidence demonstrates that despite some limitations, C. elegans is a powerful tool for identifying critical developmental periods in which EtOH could cause subsequent delays (Lin et al., 2013). In addition, this model organism permits the assessment of simple behaviors and the identification of epigenetic factors, genes, and/or proteins that regulate EtOH-related effects that may be potential therapeutic targets for the treatment of alcohol use disorders (AUD) (Khanh, 2018).
Dopamine and the lead/ethanol interaction in C. elegans
Dopamine neurotransmission is related to processes of memory, motivation, reward, locomotion, and addiction, among others (Beaulieu and Gainetdinov, 2011; Koob and Volkow, 2016). Interestingly, C. elegans show comparable responses to mammals and other higher organisms regarding substances that affect the DAergic neurotransmission, including the conditioned preference to cues previously associated with drugs of abuse (Lee et al., 2009; Musselman et al., 2012; Katner et al., 2016; Engleman et al., 2018). Thus, alterations in the integrity of this synapse could determine differential responses to neurotoxicants including EtOH, a drug that shares neurobiological mechanisms with other substances promoting DA release in nerve terminals (Söderpalm and Ericson, 2011).
In this line, several reports in higher organisms have provided evidence pointing to the DAergic system as a target of metals, including Pb-induced neurotoxicity (Cory-Slechta and Widzowski, 1991; Pokora et al., 2002; NourEddine et al., 2005). Moreover, multiple DAergic targets sensitive to the toxic action of Pb are simultaneously affected, increasing the vulnerability of this neurotransmitter to Pb toxicity in mammals (White et al., 2007). In C. elegans Lu et al. (2018) reported that 60 µM PbCl2 administered to adult worms damages the DAergic neurons in 40% of the population, presenting an abnormal phenotype that included alterations in neuronal processes evidenced as a reduction of cell bodies. Moreover, acute treatment with 5 mm Pb acetate to L1 worms induced signs of alterations in almost 80% of the DAergic neurons, accompanied by a reduction in DA levels (Akinyemi et al., 2019). This evidence suggests that alterations in the DAergic neurotransmission are present after both, early-life and adult Pb exposure.
In this regard, results from our laboratory demonstrated that developmental Pb exposure induces morphological alterations in DAergic neurons in a concentration-dependent fashion [0–240 μM Pb (NO3)2]. In this line, the lowest concentration assessed [24 μm Pb (NO3)2], although showing minimal alterations in the DAergic synapse, was sufficient to alter the Basal Slowing Response (BSR), a behavior dependent on the integrity of the DAergic system. Interestingly, this response was improved after EtOH (200 mm) only in the Pb-exposed animals that overexpress tyrosine hydroxylase (TH) or are null-mutant of the vesicular transporter (VMAT), whereas the strains lacking the DOP-4 receptor or TH-deficient showed a non-significant-reversal by the drug. These results suggest that EtOH may exert a compensatory effect in the DAergic synapse functional alterations reported in the Pb-exposed animals (Albrecht P. A. et al., 2022).
Furthermore, we have recently demonstrated that control animals treated with 200 mm EtOH reproduced the behavioral phenomenon known as AFT (Davies et al., 2003). Oppositely, perinatally-Pb exposed worms evidenced hyperactivity, which along with a high rate of recovery, was related to impaired EtOH metabolism. To this end, we demonstrated reduced ADH activity as result of early-life Pb exposure. Notably, this effect was not observed in response to 100 mm or 400 mm EtOH, suggesting the requirement of optimal EtOH concentrations for its manifestation. Finally, when another behavior was evaluated, Pb-exposed worms evidenced positive chemotaxis to a site where EtOH was present, revealing the preference of these animals for the drug (Albrecht et al., 2022b, in revision).
From worms to rats: The Pb and EtOH interaction
The above-described stimulant and motivational effects elicited by EtOH in nematodes exposed to Pb during development represent a behavioral phenomenon already described by us in a rodent model. In this regard, Mattalloni et al. reported that 35-day-old Wistar rats perinatally exposed to 220 ppm Pb self-administrated EtOH with a higher break-point than controls. They also consumed more EtOH than their respective controls and presented enhanced locomotor activity after the last voluntary consumption session (Mattalloni et al., 2013; Mattalloni et al., 2017). As with worms, we ascribed these effects to differences in the activity of the enzymes involved in EtOH oxidation (results not shown, reviewed in Virgolini et al., 2017) and their interrelation with oxidative stress (Virgolini et al., 2019), although the participation of the DAergic system was not assessed and thus cannot be discarded. Thus, despite the differences in the experimental design and animal model used in these approaches, we observed in both cases enhanced stimulant and motivational responses to EtOH as a consequence of early-life Pb exposure. These findings raise the possibility of a translational phenomenon from one model to other in the behavioral responses to EtOH. Thus, despite the few limitations of the C. elegans model such as the absence of some neurotransmitter systems (noradrenaline) or the scarce evidence regarding others (such as opioids), the results reported here allow us to propose mechanisms of toxicity that may be common for both animal species (Figure 1).
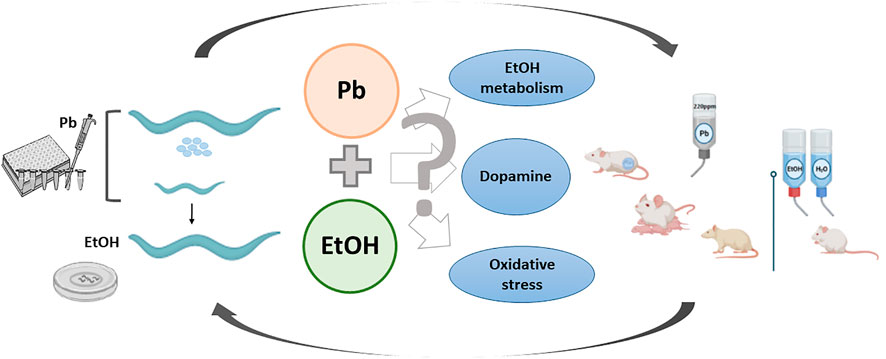
FIGURE 1. Lead and ethanol exposure protocols in two animal models and possible underlying toxic mechanisms resultant of their interaction.
Conclusions and futures perspectives
The evidence mentioned in this review underpins the usefulness of C. elegans in mechanistic studies of environmentally-relevant toxicants such as Pb, even at low exposure concentrations, which may have potential adverse effects later in life. In addition, the revised literature points to this organism as an appropriate tool for a comprehensive phenotypic approach to drugs of abuse, particularly EtOH and associated AUD. This, along with C. elegans genetics can be used to evidence the interconnections between different components of behavior and the involvement of environmental toxicants in the modification of drug-induced behaviors from the epigenetic perspective (Scholz and Mustard, 2013; Scholz, 2019). Furthermore, C. elegans can be a reliable research platform to test the efficacy of pharmacological compounds used to treat AUDs and the mechanisms of toxicity of environmental contaminants mixtures. Overall, the data provided here and the ample literature on C. elegans position this organism in the spotlight as a first-line in vivo model to perform exploratory toxicity assessment with potential and accurate escalation to superior animals.
Author contributions
All authors have contributed to the performance of the studies performed by us mentioned in the manuscript. PA and MV have written the revision and discussed the articles cited as references.
Funding
PA was granted a CONICET fellowship to pursue her Ph. D and a Fulbright fellowship for a short stay in the U.S. Founded by Agencia Nacional de Promoción Científica y Tecnológica (PICT 2017-0874) and Secretaría de Ciencia y Tecnología (Se.C. y T.) U.N.C. (MV).
Acknowledgments
We wish to acknowledge Michael Aschner from Albert Einstein College of Medicine, NY, U.S. for his generosity to open his laboratory to perform the experiments with the DA-modified strains.
Conflict of interest
The authors declare that the research was conducted in the absence of any commercial or financial relationships that could be construed as a potential conflict of interest.
Publisher’s note
All claims expressed in this article are solely those of the authors and do not necessarily represent those of their affiliated organizations, or those of the publisher, the editors and the reviewers. Any product that may be evaluated in this article, or claim that may be made by its manufacturer, is not guaranteed or endorsed by the publisher.
References
Akinyemi, A. J., Miah, M. R., Ijomone, O. M., Tsatsakis, A., Soares, F. A. A., Tinkov, A. A., et al. (2019). Lead (Pb) exposure induces dopaminergic neurotoxicity in Caenorhabditis elegans: Involvement of the dopamine transporter. Toxicol. Rep. 6, 833–840. doi:10.1016/j.toxrep.2019.08.001
Alaimo, J. T., Davis, S. J., Song, S. S., Burnette, C. R., Grotewiel, M., Shelton, K. L., et al. (2012). Ethanol metabolism and osmolarity modify behavioral responses to ethanol in C. elegans. Alcohol. Clin. Exp. Res. 36 (11), 1840–1850. doi:10.1111/j.1530-0277.2012.01799.x
Albrecht, P. A., Fernandez-Hubeid, L. E., Deza-Ponzio, R., Martins, A. C., Aschner, M., and Virgolini, M. B. (2022a). Developmental lead exposure affects dopaminergic neuron morphology and modifies basal slowing response in Caenorhabditis elegans: effects of ethanol. NeuroToxicology 91, 349–359. doi:10.1016/j.neuro.2022.06.005
Albrecht, P. A., Fernandez-Hubeid, L. E., Deza-Ponzio, R., Romero, V. L., Gonzales-Moreno, C., Carranza, A., et al. (2022b). Reduced acute functional tolerance and enhanced motivational response to ethanol in Caenorhabditis elegans exposed to lead during development. Neurotoxicology Teratol.
Anderson, G. L., Boyd, W. A., and Williams, P. L. (2001). Assessment of sublethal endpoints for toxicity testing with the nematode Caenorhabditis elegans. Environ. Toxicol. Chem. 20 (4), 833–838. doi:10.1002/etc.5620200419
Anderson, G. L., Cole, R. D., and Williams, P. L. (2004). Assessing behavioral toxicity with Caenorhabditis elegans. Environ. Toxicol. Chem. 23 (5), 1235–1240. doi:10.1897/03-264
Avila, D. S., Benedetto, A., Au, C., Bornhorst, J., and Aschner, M. (2016). Involvement of heat shock proteins on Mn-induced toxicity in Caenorhabditis elegans. BMC. Pharmacol. Toxicol. 17 (1), 1–9. doi:10.3389/fphys.2018.01200
Beaulieu, J.-M., and Gainetdinov, R. R. (2011). The physiology, signaling, and pharmacology of dopamine receptors. Pharmacol. Rev. 63 (1), 182–217. doi:10.1124/pr.110.002642
Bettinger, J. C., and Davies, A. G. (2014). The role of the BK channel in ethanol response behaviors: Evidence from model organism and human studies. Front. Physiol. 5, 346–349. doi:10.3389/fphys.2014.00346
Boyd, W. A., Cole, R. D., Anderson, G. L., and Williams, P. L. (2003). The effects of metals and food availability on the behavior of Caenorhabditis elegans. Environ. Toxicol. Chem. 22 (12), 3049–3055. doi:10.1897/02-565
Brenner, S. (1973). The genetics of behaviour. Br. Med. Bull. 29 (3), 269–271. doi:10.1093/oxfordjournals.bmb.a071019
Caito, S. W., and Aschner, M. (2016). NAD+ supplementation attenuates methylmercury dopaminergic and mitochondrial toxicity in Caenorhabditis elegans. Toxicol. Sci. 151 (1), 139–149. doi:10.1093/toxsci/kfw030
Caldwell, K. A., Willicott, C. W., and Caldwell, G. A. (2020). Modeling neurodegeneration in Caenorhabditis elegans. Dis. Model. Mech. 13 (10), dmm046110. doi:10.1242/dmm.046110
Casey, W., Jacobs, A., Maull, E., Matheson, J., Clarke, C., and Lowit, A. (2015). A new path forward: The interagency coordinating committee on the validation of alternative methods (ICCVAM) and national toxicology program’s interagency center for the evaluation of alternative toxicological methods (NICEATM). J. Am. Assoc. Lab. Anim. Sci. 54 (2), 170–173.
Chen, P., Martinez-Finley, E. J., Bornhorst, J., Chakraborty, S., and Aschner, M. (2013). Metal-induced neurodegeneration in C. elegans. Front. Aging Neurosci. 5, 18–11. doi:10.3389/fnagi.2013.00018
Cooper, J. F., and van Raamsdonk, J. M. (2018). Modeling Parkinson’s disease in C. elegans. J. Park. Dis. 8 (1), 17–32. doi:10.3233/JPD-171258
Cory-Slechta, D. A., and Widzowski, D. v. (1991). Low level lead exposure increases sensitivity to the stimulus properties of dopamine D1 and D2 agonists. Brain Res. 553 (1), 65–74. doi:10.1016/0006-8993(91)90231-J
Crowder, C. M. (2004). Ethanol targets: A BK channel cocktail in C. elegans. Trends Neurosci. 27 (10), 579–582. doi:10.1016/j.tins.2004.07.006
Davies, A. G., Blackwell, G. G., Raabe, R. C., and Bettinger, J. C. (2015). An assay for measuring the effects of ethanol on the locomotion speed of Caenorhabditis elegans. J. Vis. Exp. 2015 (98), 1–7. doi:10.3791/52681
Davies, A. G., and McIntire, S. L. (2004). Using C. elegans to screen for targets of ethanol and behavior-altering drugs. Biol. Proced. 6 (1), 113–119. doi:10.1251/bpo79
Davies, A. G., Pierce-Shimomura, J. T., Kim, H., VanHoven, M. K., Thiele, T. R., Bonci, A., et al. (2003). A central role of the BK Potassium Channel in behavioral responses to ethanol in C. elegans. Cell 115 (6), 655–666. doi:10.1016/S0092-8674(03)00979-6
Dhawan, R., Dusenbery, D. B., and Williams, P. L. (1999). Comparison of lethality, reproduction, and behavior as toxicological endpoints in the nematode Caenorhabditis elegans. J. Toxicol. Environ. Health. A 58 (7), 451–462. doi:10.1080/009841099157179
Engleman, E. A., Katner, S. N., and Neal-Beliveau, B. S. (2016). “Caenorhabditis elegans as a model to study the molecular and genetic mechanisms of drug addiction,” in Progress in molecular biology and translational science (Netherlands: Elsevier), 137. doi:10.1016/bs.pmbts.2015.10.019
Engleman, E. A., Steagall, K. B., Bredhold, K. E., Breach, M., Kline, H. L., Bell, R. L., et al. (2018). Caenorhabditis elegans show preference for stimulants and potential as a model organism for medications screening. Front. Physiol. 9, 1200. doi:10.3389/fphys.2018.01200
Gaeta, A. L., Caldwell, K. A., and Caldwell, G. A. (2019). Found in translation: the utility of C. elegans alpha-synuclein models of Parkinson’s disease. Brain Sci. 9 (4), 73. doi:10.3390/brainsci9040073
Gonzalez-Hunt, C. P., Leung, M. C., Bodhicharla, R. K., McKeever, M. G., Arrant, A. E., Margillo, K. M., et al. (2014). Exposure to mitochondrial genotoxins and dopaminergic neurodegeneration in Caenorhabditis elegans. PloS one 9 (12), e114459. doi:10.1371/journal.pone.0114459
Grotewiel, M., and Bettinger, J. C. (2015). Drosophila and Caenorhabditis elegans as discovery platforms for genes involved in human alcohol use disorder. Alcohol. Clin. Exp. Res. 39 (8), 1292–1311. doi:10.1111/acer.12785
Guo, Y., Yang, Y., and Wang, D. (2009). Induction of reproductive deficits in nematode Caenorhabditis elegans exposed to metals at different developmental stages. Reprod. Toxicol. 28 (1), 90–95. doi:10.1016/j.reprotox.2009.03.007
Hendler, R. A., Ramchandani, V. A., Gilman, J., and Hommer, D. W. (2013). Stimulant and sedative effects of alcohol. Curr. Top. Behav. Neurosci. 13, 489–509. doi:10.1007/7854_2011_135
Hunt, P. R., Camacho, J. A., and Sprando, R. L. (2020)., 23–24. Netherlands: Elsevier B.V, 23–28. doi:10.1016/j.cotox.2020.02.004Caenorhabditis elegans for predictive toxicologyCurr. Opin. Toxicol.
Hunt, P. R., Olejnik, N., Bailey, K. D., Vaught, C. A., and Sprando, R. L. (2018). C. elegans Development and Activity Test detects mammalian developmental neurotoxins. Food Chem. Toxicol. 121, 583–592. doi:10.1016/j.fct.2018.09.061
Jiang, Y., Chen, J., Wu, Y., Wang, Q., and Li, H. (2016). Sublethal toxicity endpoints of heavy metals to the nematode Caenorhabditis elegans. PLoS ONE 11 (1), e0148014. doi:10.1371/journal.pone.0148014
Katner, S. N., Bredhold, K. E., Steagall, K. B., Bell, R. L., Neal-Beliveau, B. S., Cheong, M. C., et al. (2019). Caenorhabditis elegans as a model system to identify therapeutics for alcohol use disorders. Behav. Brain Res. 365, 7–16. doi:10.1016/j.bbr.2019.02.015
Katner, S. N., Neal-Beliveau, B. S., and Engleman, E. A. (2016). Embryonic methamphetamine exposure inhibits methamphetamine cue conditioning and reduces dopamine concentrations in adult N2 Caenorhabditis elegans. Dev. Neurosci. 38 (2), 139–149. doi:10.1159/000445761
Khanh, N. (2018). A systematic review Caenorhabditis elegans (C. elegans) -A host model organism to study drug-induced responses to the effects of stimulant and depressant drugs. J. Biomed. Res. Stud. 1 (1), 1–24.
Koob, G. F., and Volkow, N. D. (2016). Neurobiology of addiction: a neurocircuitry analysis. Lancet. Psychiatry 3 (8), 760–773. doi:10.1016/S2215-0366(16)00104-8
LeBlanc, A. E., Kalant, H., and Gibbins, R. J. (1975). Acute tolerance to ethanol in the rat. Psychopharmacologia 41 (1), 43–46. doi:10.1007/BF00421304
Lee, J., Jee, C., and McIntire, S. L. (2009). Ethanol preference in C. elegans. Genes Brain Behav. 8 (6), 578–585. doi:10.1111/j.1601-183X.2009.00513.x
Li, W. H., Shi, Y. C., Tseng, I. L., and Liao, V. H. C. (2013). Protective efficacy of selenite against lead-induced neurotoxicity in Caenorhabditis elegans. PLoS ONE 8 (4), e62387. doi:10.1371/journal.pone.0062387
Lin, C. H., Sa, S., Chand, J., and Rankin, C. H. (2013). Dynamic and persistent effects of ethanol exposure on development: An in vivo analysis during and after embryonic ethanol exposure in Caenorhabditis elegans. Alcohol. Clin. Exp. Res. 37, 190–198. doi:10.1111/j.1530-0277.2012.01856.x
Lu, C., Svoboda, K. R., Lenz, K. A., Pattison, C., and Ma, H. (2018). Toxicity interactions between manganese (Mn) and lead (Pb) or cadmium (Cd) in a model organism the nematode C. elegans. Environ. Sci. Pollut. Res. Int. 25 (16), 15378–15389. doi:10.1007/s11356-018-1752-5
Martins, A. C., Virgolini, M. B., Tinkov, A. A., Skalny, A. V., Tirumala, R. P., Farina, M., et al. (2022). Iron overload and neurodegenerative diseases: What can we learn from Caenorhabditis elegans? Toxicol. Res. Appl. 6, 239784732210918. doi:10.1177/23978473221091852
Mattalloni, M. S., de Giovanni, L. N., Molina, J. C., Cancela, L. M., and Virgolini, M. B. (2013). Participation of catalase in voluntary ethanol consumption in perinatally low-level lead-exposed rats. Alcohol. Clin. Exp. Res. 37 (10), 1632–1642. doi:10.1111/acer.12150
Mattalloni, M. S., Deza-Ponzio, R., Albrecht, P. A., Cancela, L. M., and Virgolini, M. B. (2017). Developmental lead exposure induces opposite effects on ethanol intake and locomotion in response to central vs. systemic cyanamide administration. Alcohol 58, 1–11. doi:10.1016/j.alcohol.2016.11.002
Maulik, M., Mitra, S., Bult-Ito, A., Taylor, B. E., and Vayndorf, E. M. (2017). Behavioral phenotyping and pathological indicators of Parkinson's disease in C. elegans models. Front. Genet. 8, 77. doi:10.3389/fgene.2017.00077
Meneely, P. M., Dahlberg, C. L., and Rose, J. K. (2019). Working with worms: Caenorhabditis elegans as a model organism. Curr. Protoc. Essent. Lab. Tech. 19 (1). doi:10.1002/cpet.35
Mitra, P., Sharma, S., Purohit, P., and Sharma, P. (2017). Clinical and molecular aspects of lead toxicity: An update. Crit. Rev. Clin. Lab. Sci. 54 (7–8), 506–528.
Monteiro, L., Brinke, M., dos Santos, G., Traunspurger, W., and Moens, T. (2014). Effects of heavy metals on free-living nematodes: A multifaceted approach using growth, reproduction and behavioural assays. Eur. J. Soil Biol. 62, 1–7. doi:10.1016/j.ejsobi.2014.02.005
Morgan, P. G., and Sedensky, M. M. (1995). Mutations affecting sensitivity to ethanol in the nematode, Caenorhabditis elegans. Alcohol. Clin. Exp. Res. 19 (6), 1423–1429. doi:10.1111/j.1530-0277.1995.tb01002.x
Musselman, H. N., Neal-Beliveau, B., Nass, R., and Engleman, E. A. (2012). Chemosensory cue conditioning with stimulants in a Caenorhabditis elegans animal model of addiction. Behav. Neurosci. 126 (3), 445–456. doi:10.1037/a0028303
NourEddine, D., Miloud, S., and Abdelkader, A. (2005). Effect of lead exposure on dopaminergic transmission in the rat brain. Toxicology 207 (3), 363–368. doi:10.1016/j.tox.2004.10.016
Paul, D., Chipurupalli, S., Justin, A., Raja, K., and Mohankumar, S. K. (2020). Caenorhabditis elegans as a possible model to screen anti-Alzheimer’s therapeutics. J. Pharmacol. Toxicol. Methods 106, 106932. doi:10.1016/J.VASCN.2020.106932
Pohorecky, L. A. A. (1977). Biphasic action of ethanol. Biobehav. Rev. 1 (4), 231–240. doi:10.1016/0147-7552(77)90025-0
Pokora, M. J., Richfield, E. K., and Cory-Slechta, D. A. (2002). Preferential vulnerability of nucleus accumbens dopamine binding sites to low-level lead exposure: Time course of effects and interactions with chronic dopamine agonist treatments. J. Neurochem. 67 (4), 1540–1550. doi:10.1046/j.1471-4159.1996.67041540.x
Raabe, R. C., Mathies, L. D., Davies, A. G., and Bettinger, J. C. (2014). The omega-3 fatty acid eicosapentaenoic acid is required for normal alcohol response behaviors in C. elegans. PLoS One 9 (8), e105999. doi:10.1371/journal.pone.0105999
Roh, J. Y., Lee, J., and Choi, J. (2006). Assessment of stress-related gene expression in the heavy metal-exposed nematode Caenorhabditis elegans: A potential biomarker for metal-induced toxicity monitoring and environmental risk assessment. Environ. Toxicol. Chem. 25 (11), 2946–2956. doi:10.1897/05-676R.1
Ruszkiewicz, J. A., Pinkas, A., Miah, M. R., Weitz, R. L., Lawes, M. J. A., Akinyemi, A. J., et al. (2018). C. elegans as a model in developmental neurotoxicology. Toxicol. Appl. Pharmacol. 354, 126–135. doi:10.1016/j.taap.2018.03.016
Scholz, H., and Mustard, J. A. (2013). Invertebrate models of alcoholism. Curr. Top. Behav. Neurosci. 23, 433–457. doi:10.1007/7854_2011_128
Scholz, H. (2019). Unraveling the mechanisms of behaviors associated with AUDs using flies and worms. Alcohol. Clin. Exp. Res. 43 (11), 2274–2284. doi:10.1111/acer.14199
Scott, L. L., Davis, S. J., Yen, R. C., Ordemann, G. J., Nordquist, S. K., Bannai, D., et al. (2017). Behavioral deficits following withdrawal from chronic ethanol are influenced by SLO channel function in Caenorhabditis elegans. Genetics 206 (3), 1445–1458. doi:10.1534/genetics.116.193102
Sedensky, M. M., and Morgan, P. G. (2018). Using Caenorhabditis elegans to study neurotoxicity. Handb. Dev. Neurotoxicology, 153–160. doi:10.1016/B978-0-12-809405-1.00013-4
Silbergeld, E. K. (1992). Mechanisms of lead neurotoxicity, or looking beyond the lamppost. FASEB J. 6 (13), 3201–3206. doi:10.1096/fasebj.6.13.1397842
Soares, F. A., Fagundez, D. A., and Avila, D. S. (2017). “Neurodegeneration induced by metals in Caenorhabditis elegans,” in Advances in neurobiology (New York: Springer), 18, 355–383. doi:10.1007/978-3-319-60189-2_18
Söderpalm, B., and Ericson, M. (2011). Neurocircuitry involved in the development of alcohol addiction: The dopamine system and its access points. Curr. Top. Behav. Neurosci. 13, 127–161. doi:10.1007/7854_2011_170
Sterken, M. G., van Wijk, M. H., Quamme, E. C., Riksen, J. A. G., Carnell, L., Mathies, L. D., et al. (2021). Transcriptional analysis of the response of C. elegans to ethanol exposure. Sci. Rep. 11 (1), 10993. doi:10.1038/s41598-021-90282-8
Sudama, G., Zhang, J., Isbister, J., and Willett, J. D. (2013). Metabolic profiling in Caenorhabditis elegans provides an unbiased approach to investigations of dosage dependent lead toxicity. Metabolomics 9 (1), 189–201. doi:10.1007/s11306-012-0438-0
Sun, L., Wu, Q., Liao, K., Yu, P., Cui, Q., Rui, Q., et al. (2016). Contribution of heavy metals to toxicity of coal combustion related fine particulate matter (PM2.5) in Caenorhabditis elegans with wild-type or susceptible genetic background. Chemosphere 144, 2392–2400. doi:10.1016/j.chemosphere.2015.11.028
Szczerbak, G., Nowak, P., Kostrzewa, R. M., and Brus, R. (2007). Maternal lead exposure produces long-term enhancement of dopaminergic reactivity in rat offspring. Neurochem. Res. 32 (10), 1791–1798.
Tiwari, S. S., Tambo, F., and Agarwal, R. (2020). Assessment of lead toxicity on locomotion and growth in a nematode Caenorhabditis elegans. J. Appl. Nat. Sci. 12 (1), 36–41. doi:10.31018/jans.v12i1.2227
Virgolini, M. B., and Aschner, M. (2021). “Molecular mechanisms of lead neurotoxicity,” in Neurotoxicity of metals: Old issues and new developments. 1st ed. (Netherlands: Elsevier), 5. doi:10.1016/bs.ant.2020.11.002
Virgolini, M. B., Mattalloni, M. S., Albrecht, P. A., Deza-Ponzio, R., and Cancela, L. M. (2017). Modulation of ethanol-metabolizing enzymes by developmental lead exposure: Effects in voluntary ethanol consumption. Front. Behav. Neurosci. 11, 95–96. doi:10.3389/fnbeh.2017.00095
Virgolini, M. B., Mattalloni, M. S., Deza-Ponzio, R., Albrecht, P. A., and Cancela, L. M. (2019). “Lead exposure and ethanol intake: Oxidative stress as a converging mechanism of action,” in Neuroscience of alcohol (Cambridge: Academic Press), 515–525.
Virgolini, M. B., and Pautassi, R. M. (2022). “Converging mechanisms in ethanol neurotoxicity,” in Advances in Neurotoxicology (Netherlands: Elsevier). doi:10.1016/bs.ant.2022.06.002
Wang, D., and Xing, X. (2008). Assessment of locomotion behavioral defects induced by acute toxicity from heavy metal exposure in nematode Caenorhabditis elegans. J. Environ. Sci. 20 (9), 1132–1137. doi:10.1016/S1001-0742(08)62160-9
Wang, D., and Xing, X. (2009). Pre-treatment with mild metal exposure suppresses the neurotoxicity on locomotion behavior induced by the subsequent severe metal exposure in Caenorhabditis elegans. Environ. Toxicol. Pharmacol. 28 (3), 459–464. doi:10.1016/j.etap.2009.07.008
Wang, D., and Yang, P. (2007). Multi-biological defects caused by lead exposure exhibit transferable properties from exposed parents to their progeny in Caenorhabditis elegans. J. Environ. Sci. 19 (11), 1367–1372. doi:10.1016/S1001-0742(07)60223-X
White, L. D., Cory-Slechta, D. A., Gilbert, M. E., Tiffany-Castiglioni, E., Zawia, N. H., Virgolini, M., et al. (2007). New and evolving concepts in the neurotoxicology of lead. Toxicol. Appl. Pharmacol. 225 (1), 1–27. doi:10.1016/j.taap.2007.08.001
Wu, Q., Liu, P., Li, Y., Du, M., Xing, X., and Wang, D. (2012). Inhibition of ROS elevation and damage to mitochondrial function prevents lead-induced neurotoxic effects on structures and functions of AFD neurons in Caenorhabditis elegans. J. Environ. Sci. 24 (4), 733–742. doi:10.1016/S1001-0742(11)60835-8
Wu, Y., and Luo, Y. (2005). Transgenic C. elegans as a model in alzheimers research. Curr. Alzheimer Res. 2 (1), 37–45. doi:10.2174/1567205052772768
Wu, Z.-Q., Li, K., Ma, J.-K., and Li, Z.-J. (2019). Effects of ethanol intake on anti-oxidant responses and the lifespan of Caenorhabditis elegans. CyTA - J. Food 17 (1), 288–296. doi:10.1080/19476337.2018.1564794
Xing, X., Rui, Q., Du, M., and Wang, D. Y. (2009a). Exposure to lead and mercury in young larvae induces more severe deficits in neuronal survival and synaptic function than in adult nematodes. Arch. Environ. Contam. Toxicol. 56 (4), 732–741. doi:10.1007/s00244-009-9307-x
Xing, X., Rui, Q., and Wang, D. (2009b). Lethality toxicities induced by metal exposure during development in nematode Caenorhabditis elegans. Bull. Environ. Contam. Toxicol. 83 (4), 530–536. doi:10.1007/s00128-009-9816-3
Ye, H., Ye, B., and Wang, D. (2008). Trace administration of vitamin E can retrieve and prevent UV-irradiation- and metal exposure-induced memory deficits in nematode Caenorhabditis elegans. Neurobiol. Learn. Mem. 90 (1), 10–18. doi:10.1016/j.nlm.2007.12.001
Yu, Z. Y., Chen, X. X., Zhang, J., Wang, R., and Yin, D. Q. (2013). Transgenerational effects of heavy metals on L3 larva of Caenorhabditis elegans with greater behavior and growth inhibitions in the progeny. Ecotoxicol. Environ. Saf. 88, 178–184. doi:10.1016/j.ecoenv.2012.11.012
Yu, Z. Y., Zhang, J., and Yin, D. Q. (2016). Multigenerational effects of heavy metals on feeding, growth, initial reproduction and antioxidants in Caenorhabditis elegans. PLoS ONE 11 (4), e0154529–13. doi:10.1371/journal.pone.0154529
Zhang, Y., Ye, B., and Wang, D. (2010). Effects of metal exposure on associative learning behavior in nematode Caenorhabditis elegans. Arch. Environ. Contam. Toxicol. 59 (1), 129–136. doi:10.1007/s00244-009-9456-y
Zhao, Y., Chen, J., Wang, R., Pu, X., and Wang, D. (2022). A review of transgenerational and multigenerational toxicology in the in vivo model animal Caenorhabditis elegans. J. Appl. Toxicol. Online ahead of print. doi:10.1002/jat.4360
Zhao, Y. L., and Wang, D. Y. (2012). Formation and regulation of adaptive response in nematode Caenorhabditis elegans. Oxid. Med. Cell. Longev. 2012, 564093. doi:10.1155/2012/564093
Zhu, G., Zhang, F., and Li, W. (2014). Nematodes feel a craving - using Caenorhabditis elegans as a model to study alcohol addiction. Neurosci. Bull. 30 (4), 595–600. doi:10.1007/s12264-014-1451-7
Keywords: lead-exposure, ethanol, dopamine, Caenorabditis elegans, rats
Citation: Albrecht PA, Fernandez-Hubeid LE, Deza-Ponzio R and Virgolini MB (2022) The intertwining between lead and ethanol in the model organism Caenorhabditis elegans. Front.Toxicol 4:991787. doi: 10.3389/ftox.2022.991787
Received: 12 July 2022; Accepted: 18 August 2022;
Published: 20 September 2022.
Edited by:
Alice Villalobos, Texas Tech University Health Sciences Center, United StatesReviewed by:
Vivek Lawana, North American Science Associates, Inc., United StatesCopyright © 2022 Albrecht, Fernandez-Hubeid, Deza-Ponzio and Virgolini. This is an open-access article distributed under the terms of the Creative Commons Attribution License (CC BY). The use, distribution or reproduction in other forums is permitted, provided the original author(s) and the copyright owner(s) are credited and that the original publication in this journal is cited, in accordance with accepted academic practice. No use, distribution or reproduction is permitted which does not comply with these terms.
*Correspondence: M. B. Virgolini, bWlyaWFtLnZpcmdvbGluaUB1bmMuZWR1LmFy