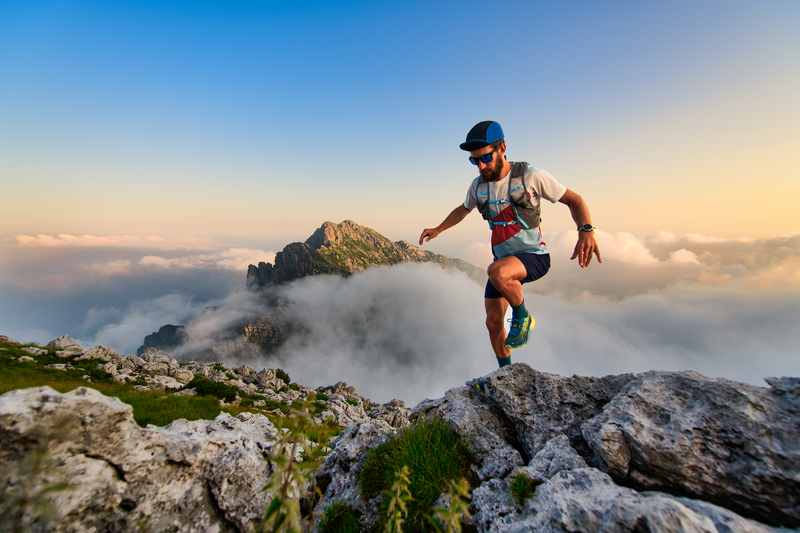
95% of researchers rate our articles as excellent or good
Learn more about the work of our research integrity team to safeguard the quality of each article we publish.
Find out more
ORIGINAL RESEARCH article
Front. Toxicol. , 05 September 2022
Sec. Nanotoxicology
Volume 4 - 2022 | https://doi.org/10.3389/ftox.2022.974429
This article is part of the Research Topic Methods and Protocols in Nanotoxicology View all 10 articles
Engineered nanomaterials have been found to induce oxidative stress. Cellular oxidative stress, in turn, can result in the induction of antioxidant and detoxification enzymes which are controlled by the nuclear erythroid 2-related factor 2 (NRF2) transcription factor. Here, we present the results of a pre-validation study which was conducted within the frame of BIORIMA (“biomaterial risk management”) an EU-funded research and innovation project. For this we used an NRF2 specific chemically activated luciferase expression reporter gene assay derived from the human U2OS osteosarcoma cell line to screen for the induction of the NRF2 mediated gene expression following exposure to biomedically relevant nanobiomaterials. Specifically, we investigated Fe3O4-PEG-PLGA nanomaterials while Ag and TiO2 “benchmark” nanomaterials from the Joint Research Center were used as reference materials. The viability of the cells was determined by using the Alamar blue assay. We performed an interlaboratory study involving seven different laboratories to assess the applicability of the NRF2 reporter gene assay for the screening of nanobiomaterials. The latter work was preceded by online tutorials to ensure that the procedures were harmonized across the different participating laboratories. Fe3O4-PEG-PLGA nanomaterials were found to induce very limited NRF2 mediated gene expression, whereas exposure to Ag nanomaterials induced NRF2 mediated gene expression. TiO2 nanomaterials did not induce NRF2 mediated gene expression. The variability in the results obtained by the participating laboratories was small with mean intra-laboratory standard deviation of 0.16 and mean inter laboratory standard deviation of 0.28 across all NRF2 reporter gene assay results. We conclude that the NRF2 reporter gene assay is a suitable assay for the screening of nanobiomaterial-induced oxidative stress responses.
It is a well-established paradigm that ambient particulate matter as well as engineered nanomaterials can trigger oxidative stress (Li et al., 2008; Stone et al., 2017). Under normal physiological conditions, reactive oxygens species (ROS) are continuously formed and immediately neutralized by antioxidant defences such as glutathione (GSH) and an array of antioxidant enzymes. However, under conditions of excessive ROS production, which may occur in cells exposed to engineered nanomaterials or other toxicants, the natural antioxidant defences of the cell may be overwhelmed (Sies and Jones, 2020). Oxidative stress is characterized by a cellular depletion of GSH while oxidized glutathione (GSSG) accumulates. Cells respond to this drop in the GSH/GSSG ratio by several protective or damage related signalling responses (Aguilano et al., 2014).
The NRF2–KEAP1 system plays a key role in maintaining redox homeostasis in eukaryotes (Sies and Jones, 2020). KEAP1 (Kelch-like ECH-associated protein 1) acts as a cysteine thiol-rich sensor of redox insults, whereas NRF2 (nuclear erythroid 2-related factor 2) is a transcription factor that regulates electrophile responsive element (EpRE)-mediated gene expression to switch on a battery of cytoprotective genes. Upon associating with other transcription factors, NRF2 binds to the EpRE and activates EpRE-mediated gene expression, including the genes encoding for detoxifying enzymes and proteins, such as glutathione peroxidase (GPx), NAD(P)H-quinone oxidoreductase (NQO1), superoxide dismutase (SOD), catalase (CAT), peroxiredoxin (PRx), glutathione S-transferase (GST), γ-glutamylcysteine synthetase (γ-GCS) and glutamate-cysteine ligase (GCL) genes. At higher levels of oxidative stress, this protective response is overtaken by cytotoxicity (Li et al., 2008).
Several recent in vitro studies reported the activation of the NRF2 pathway following exposure to a variety of engineered nanomaterials, including CeO2 nanomaterials (Choi et al., 2021), SiO2 nanomaterials (Cui et al., 2021), and ZnO nanomaterials (Zhang et al., 2021). Moreover, Kim et al. (2021) investigated seven different metal oxides (CuO, Co3O4, NiO, TiO2, CeO2, Fe2O3, and ZnO) using the ARE-NRF2 Luciferase KeratinoSens™ assay that is based on stably transfected immortalised human keratinocytes (HaCaT). CuO nanomaterials but not Co3O4, NiO, TiO2, CeO2, Fe2O3, or ZnO nanomaterials induced a positive response. The latter assay is recognized as a Test Guideline by the OECD since 2018 (Test No. 442D: In vitro Skin Sensitisation). Using a NRF2/ARE Responsive Luciferase Reporter HEK293 Cell Line, it has been shown that CuO, Mn2O3 and ZnO nanomaterials strongly induce the NRF2 mediated gene expression, while a recent study showed that Fe2O3 materials of different sizes induced limited gene expression in these reporter cells (Seleci et al., 2022). Using HEK293 cells, Ag nanomaterials have also been shown in several studies to trigger an NRF2 response in a range of different cell types (Miranda et al., 2022, and see other references therein).
Nanotoxicological studies have been conducted using a plethora of cell-based assays but there is a need for robust (validated) assays that are suitable for high-throughput screening of nanomaterials to improve safety assessment practices (Nymark et al., 2020). In the current study, we applied a reporter gene assay for the screening of oxidative stress induction by nanobiomaterials. This pre-validation study was performed within the EU-funded research project BIORIMA (“biomaterial risk management”). The overarching goal of the BIORIMA project has been to develop a risk management framework for nanobiomaterials used in medical devices and advanced therapy medicinal products (Giubilato et al., 2020). Hazard assessment of nanobiomaterials is one of the important elements of this framework and the approaches to assess the hazard potential of nanobiomaterials can either be based on methods adopted from classical toxicology (of chemicals and other particles) or on alternative methods, including in vitro and in vivo methods and in silico modelling (Giubilato et al., 2020).
In the present study, we pre-validated a reporter gene assay which is based on human osteoblastic osteosarcoma U2OS cells that express luciferase through transfection with a vector carrying antioxidant response elements (ARE) upstream of a luciferase reporter gene (van der Linden et al., 2014). Participating laboratories were recruited from the BIORIMA consortium, of which 7 laboratories fully completed all experiments. Cells were exposed to Fe3O4-PEG-PLGA (Fe3O4 -PolyEthylene Glycol - PolyLactide-co-Glycolide Acid) nanomaterials and to Ag and TiO2 “benchmark” nanomaterials. The Fe3O4-PEG-PLGA nanomaterials are envisioned for a variety of applications in medicine, including as a magnetic hyperthermia agent, an in vivo imaging/contrast agent, and an active targeting and drug delivery agent (Cazzagon et al., 2022), and for this reason, these materials were selected as one example of a relevant nanobiomaterial.
Curcumin (Sigma cat no. C1386), dichlorvos (Sigma cat no. 45441), and mannitol (Sigma cat no. M9647) were purchased from Sigma Aldrich (Amsterdam, Netherlands), and dimethyl sulfoxide (DMSO) (Arcos cat no. 167852500) was purchased from Acros Organics (Geel, Belgium). Dulbecco’s Modified Eagle Medium with Ham’s Nutrient Mixture F-12 (1:1) (DMEM/F12) without phenol red (Gibco cat no. 31331-028), Trypsin 0.5% EDTA (10x) (Gibco cat no. 15400-054), nonessential amino acids (NEAA) (Gibco cat no. 11140-035), and phosphate-buffered saline (Gibco cat no. 20012019) were from Gibco (Carlsbad, CA), geneticin (G418) (Duchefa cat no. G0175001) from Duchefa (Haarlem, Netherlands), and penicillin/streptomycin, pH 7.4 (P/S) (Invitrogen cat no. 15070063) from Invitrogen (Breda, Netherlands). Fetal bovine serum (FBS) (Gibco cat no. 10270-106) and dextran-coated charcoal-stripped fetal calf serum (DCC-FCS) (Gibco cat no. 12676029) were both purchased from Gibco.
Fe3O4-PEG-PLGA nanomaterials were provided by Colorobbia Holding S.p.A (Firenze, Italy) in the framework of the BIORIMA research project and synthesized as described (D'Elios et al., 2018). Both Ag and TiO2 nanomaterials (designated NM300K and NM101, respectively) were from the nanomaterial repository of the Joint Research Center of the European Commission (Ispra, Italy). Ag nanomaterials were provided as a suspension. The NANOGENOTOX protocol was used for dispersion of TiO2 (Farcal et al., 2015).
The dissolution and aggregation of the Ag nanomaterials was monitored by small angle X-ray scattering (SAXS) following incubation for 18 days at 37°C in MEM media (Invitrogen cat no. 51200) supplemented with 4% FBS (Sigma cat no. F7524), 1% Glutamax (Invitrogen cat. no. 35050-038), 1% non-essential amino acids (Invitrogen cat no. 11140), 1% sodium pyruvate (Sigma cat no. S8636), 1% penicillin-streptomycin (Invitrogen cat no. 15140-122) and 1% HEPES (Invitrogen cat no. 15630). SAXS measurements were carried out on Xeuss 2.0 (Xenocs) and ChemSaxs (lab design, CEA) high-resolution X-ray spectrometers in Kapton capillaries at a concentration of 0.5 mg/ml. The signal of the baselines was subtracted and data were fitted with PySAXS software (https://pypi.org/project/pySAXS/). SAXS experiments were performed by one of the participating laboratories.
TEM was performed by using a FEI TECNAI F20 microscope operating at 200 keV. The suspension was drop-casted on a holey carbon film supported by a gold grid. The specimen was then dried at 60°C. To gather information about particles morphology the images were taken in phase contrast mode and high-angle annular dark-field scanning transmission mode (HAADF-STEM). High resolution (HREM) and Selected Area Electron Diffraction (SAED) analyses were performed to investigate the crystalline phase structure and composition. To calculate the mean particle diameter more than 100 particles were measured. TEM experiments were performed by one of the participating laboratories.
Hydrodynamic sizes and zeta potential of Fe3O4-PEG-PLGA nanomaterials were determined as previously described in the NanoREG project (Bhattacharya et al., 2017). In short, concentrations of the test samples were adjusted from the 1 mg/ml respective stock suspensions using either endotoxin free water or the medium with or without FBS to a concentration of 25 μg/ml for the measurements. Particle size distribution and zeta potential of the test samples were measured by dynamic light scattering (DLS) technique using Malvern Zetasizer Nano ZS. Three measurements with no pause were taken for particle size distribution and for the zeta potential values of each test material at 0, and 24 h at a temperature of 25°C. DLS experiments were performed by one of the participating laboratories.
The Limulus Amoebocyte Lysate (LAL) assay was applied to detect bacterial endotoxin contamination as described earlier (Kroll et al., 2013; Eder et al., 2022). The Limulus Amoebocyte Lysate PYROTELL®–T assay was purchased from Associates of Cape Cod, Inc. (East Falmouth, MA) and used according to the manufacturer’s instructions. Data analysis was performed using PYROS® Software (Associates of Cape Cod, Inc.).
U2OS-NRF2 cells were kindly provided by Bio Detection Systems (Amsterdam, Netherlands). The human osteoblastic osteosarcoma U2OS-NRF2 cells (van der Linden et al., 2014) express two oligos containing four different EPRE sequences: 1 × consensus EPRE (TCACAGTGACTAAGCAAAAT), 1 × hNQO1 EPRE (TCACAGTGAC TCAGCA-GAAT), 1 × hGCLM EPRE (AGACAATGACTAAGCAGAAA) and 1 × hGCLC EPRE(TCACAGTCAGTAAGTGATGG). The two oligos were ligated into a promoter-less luciferase reporter-construct pLuc. Because the U2OS cells express the NRF2 pathway endogenously, a selection construct (pSG5-neo) was used. The cells were cultured in DMEM/F12 supplemented with 10% FCS and penicillin/streptomycin (final concentrations 10 U/ml and 10 μg/ml, respectively) (designated as growth medium). Once per week, 200 μg/ml G418 was added to the culture medium to maintain selection pressure. Cells were maintained at 37°C in a humidified atmosphere with 5% CO2.
Cytotoxicity of nanobiomaterials was evaluated by the Alamar blue (resazurin) assay as described (Keshavan et al., 2021). The cell viability experiments were performed by one of the participating laboratories, prior the “round robin” pre-validation experiments. The cells were trypsinized, counted, and resuspended in cell culture medium without phenol red and supplemented with 5% dextran-coated charcoal-stripped FCS (DCC–FCS), to a final concentration of 104cells/well (100 µl). Cells were seeded in 96-well plates and exposed to test materials or were maintained in DCC–FCS alone (negative control). The assay reagent (Thermo Scientific, Sweden) (10% [v/v] solution of AlamarBlue® reagent) was added to each well to monitor the cellular metabolic function. The samples were analyzed using a spectrophotometer (Tecan Infinite® F200).
The potential induction of NRF2 mediated gene expression by nanobiomaterials was tested by measuring the induction of luciferase activity in the NRF2-U2OS cells. Protocols are available upon request. In brief, the cells were trypsinized, counted, and resuspended in cell culture medium without phenol red and supplemented with 5% dextran-coated charcoal-stripped FCS (DCC–FCS) at a final concentration of 104cells/well (100 µl) in a 96-well plate without using the most outer wells. The plates were incubated for 24 h in a humidified atmosphere at 37°C under 5% CO2. Following this pre-incubation one reference plate was exposed containing 9 serial dilutions in the range of 1 × 10−4 M to 1 × 10−8 M (log10 dilution steps) of the reference compound curcumin, as well as a positive control dichlorvos (1 × 10−5–7 × 10−7 M) and a negative control mannitol (1 × 10−3–1 × 10−5 M). Dichlorvos was included as a positive control as it is known to induce a response in this assay, while the negative control (i.e., mannitol) should not. Curcumin was chosen as reference compound, as it usually results in a dose-effect response in the current assay. It is good practice to select different chemicals as reference chemical and positive control. The cells were exposed to reference compounds by adding the compounds from a 200 x concentrated stock solution in DMSO to exposure medium (5% DCC-FCS in DMEM/F12 without phenol red). Following exposure to the test materials, cells were further processed for the luciferase induction assay. Cells were rinsed using PBS followed by lysis through 30 µl low salt buffer (Tris, 25 mM, DTT 2.0 mM, CDTA 2.0 mM), and a subsequent freezing step at −80°C ensured complete cell lysis. Luciferase was measured using a flash mix protocol (BDS, Amsterdam, Netherlands). The flash mix or illuminate mix contained 20 mM tricine, 1.07 mM (MgCO3)4 Mg(OH)2.5 H2O, 2.67 mM MgSO4 x 7 H2O, EDTA 0.1 mM, DTT 1.5 mM, D-Luciferine 539 mM, ATP 5.49 mM. The measurements were performed in the different laboratories using a luminometer with two injectors, one to initiate the reaction (through the addition of the Luciferin present in the illuminate mix) and one for stopping the enzymatic reaction with NaOH. The reaction was thus stopped by adding 100 µL of 0.2 M NaOH. A threshold of induction factor of 1.5 was set for the NRF2 mediated gene expression, as described before (van der Linden et al., 2014).
Fe3O4-PEG-PLGA nanomaterials were selected as a representative and novel nanobiomaterial for the present study. These nanomaterials are envisioned both for therapeutic and diagnostic applications. The “benchmark” TiO2 nanomaterials were included as an inert (non-cytotoxic) nanomaterial and Ag nanomaterials were included as a nanomaterial that most likely would elicit NRF2 mediated gene expression (based on the available literature, see above), though cytotoxicity at higher concentrations of the latter nanomaterials could not be excluded. Additional positive and negative chemical controls (dichlorvos and mannitol) were included for the assay based on the manufacturer’s recommendations.
The participating laboratories were trained (online) on the execution of the NRF2 reporter gene assay, quality control measures, and data analysis (for a schematic of the workflow, refer to Figure 1). The following laboratories/institutions participated in the pre-validation study: Karolinska Institutet, Wageningen University, University of Torino, Université Grenoble-Alpes, Edinburgh Napier University, University of Rome Tor Vergata, Université Paris Cité, and Tokyo University of Science. However, one of these laboratories only tested Fe3O4-PEG-PLGA and not the other “benchmark” nanomaterials and the results are therefore shown separately. Protocols were extensively discussed and agreed upon during online meetings and tutorials. Chemicals and cell culture reagents were procured from the same source, and the NRF2-U2OS cell line was distributed to all the laboratories. The plate layout for the reporter gene assay was decided. Each experiment thus included one reference plate and three experimental plates. The three upper rows (B-C-D) of the reference plate as well as each experimental plate contained a full concentration range of the reference compound curcumin dissolved in DMSO. The lower part (rows E-F-G) contained the positive and negative control (reference plate) or one of the three nanobiomaterials under investigation (Fe3O4-PEG-PLGA, TiO2, Ag). The participating laboratories also harmonized the exposure conditions. Hence, Fe3O4-PEG-PLGA and Ag nanomaterials were diluted from a stock of 3000 μg/ml in dispersant provided with the particles at a concentration range of 0.21 µg/ml–3000 μg/ml followed by a second 30 x dilution step in exposure medium to an exposure range of 0.001–100 μg/ml. For TiO2, freshly prepared suspensions were made using the NANOGENOTOX dispersion protocol (Farcal et al., 2015). The reporter cells were exposed for 24 h in a humidified atmosphere at 37°C under 5% CO2.
FIGURE 1. Workflow of the pre-validation experiments. The number of laboratories involved is indicated.
Data were exported to Excel (Microsoft) for further processing. Cytotoxicity was expressed as % viability towards the unexposed cells. For the NRF2 reporter gene experiments, the results were presented as the Induction Factor (IF), which is the measured relative light unit (RLU) value divided by the mean RLU value of the solvent control. When the induction factor of curcumin was over 8, the NRF2-U2OS reporter gene assay was regarded to be effective. Samples presenting 1.5 fold or higher induction were considered as inducers of NRF2 mediated-gene expression (van der Linden et al., 2014). Graphs were prepared in Prism 9.0 (GraphPad Software, Inc.) by analysing data using non-linear curve fitting (agonist versus response). To evaluate the variability of results and reproducibility of the assay, both the intra-laboratory and inter laboratory standard deviations were calculated across all NRF2 reporter gene assay results and plotted in a heatmap. Statistical analysis was performed using GraphPad Prism version 8.3.0.
Interlaboratory standard deviation of the assay results of all participating laboratories was calculated in accordance with ISO standards 5725-1 and 5725-2 for accuracy (trueness and precision) of measurement methods and results.
The Fe3O4-PEG-PLGA nanomaterials obtained from Colorobbia and the corresponding dispersant were evaluated for sterility (endotoxin content). Both were found to contain endotoxin levels below the US FDA-mandated level for medical devices (data not shown). The Fe3O4-PEG-PLGA nanomaterials were visualized by TEM. TEM phase contrast images (Figure 2A) and HAADF-STEM images (Figure 2B) indicated regular morphology with a mean particle diameter of 12 ± 4 nm. The higher magnification HREM phase contrast images (Figure 2C) disclosed a cubic crystal structure consistent with the magnetite lattice, and polycrystalline pattern rings collected by SAED (Figure 2D) were indexed as crystalline magnetite, identified as the unique phase composition. The benchmark materials were fully characterised, see Comero et al. (2011) for Ag, and Rasmussen et al. (2014) for the TiO2 nanomaterials.
FIGURE 2. Transmission electron microscopy images of as-synthesized Fe3O4-PEG-PLGA nanomaterials. (A) TEM phase contrast image; (B) HAADF-STEM image; (C) HREM phase contrast image; (D) SAED polycrystalline pattern rings. Scale bars: (A,B) 50 nm; (C) 10 nm.
SAXS analysis showed that there was little or no dissolution of the Ag nanomaterials following incubation at 37°C for 18 days in culture media. The average size of these nanomaterials did not change during incubation (15 ± 0.2 nm and 15 ± 0.2 nm at t = 0 and t = 18 days, respectively). The nanobiomaterials were also analysed with respect to hydrodynamic diameter and zeta potential in the relevant cell culture medium. Previously the dissolution of the Fe3O4-PEG-PLGA nanomaterials in cell culture media was shown to be less than 0.5% within 24 h (data not shown). Together the data indicated that all the test materials were stable following incubation in cell culture medium for 24 h at the exposure conditions for the NRF2 reporter gene assay (Supplementary Figures S1A,B).
For a correct interpretation of the results from the reporter gene assay, the potential of the test materials to reduce cell viability should be assessed. To this end, the Alamar blue assay was used. Fe3O4-PEG-PLGA nanomaterials were non-cytotoxic towards U2OS cells and only a slight decrease in cell viability (metabolic capacity) was evidenced at the highest tested concentration of 100 μg/ml (Figure 3A). Similarly, TiO2 nanomaterials were non-cytotoxic at low concentrations but a markedly decreased viability at the highest concentration of 100 μg/ml (Figure 3B) was noted. In contrast, for Ag nanomaterials, a dose-dependent loss of cell viability was observed (Figure 3C). The potential cytotoxic effects of the reference compounds curcumin, dichlorvos (positive control) and mannitol (negative control) were also evaluated (Figure 4). Neither dichlorvos or mannitol affected cell viability of the U2OS cells, while curcumin at a concentration of 500 nM and higher reduced U2OS cell viability in a dose-dependent manner (Figure 4).
FIGURE 3. Cytotoxicity assessment. U2OS cells were exposed for 24 h to (A) Fe3O4-PEG-PLGA, (B) TiO2 nanomaterials, and (C) Ag nanomaterials or dispersants and cell viability (metabolic capacity) was evaluated using the Alamar blue assay. Data are mean values ± S.D. of three independent experiments.
FIGURE 4. Cytotoxicity of control chemicals used in the NRF2 reporter gene assay. U2OS cells were exposed to curcumin, dichlorvos, and mannitol for 24 h and evaluated using the Alamar blue assay.
Next, the induction of the NRF2 pathway was assessed. NRF2-U2OS cells were exposed to increasing concentrations of the reference compound (curcumin), and to the positive and negative controls (Figure 5). Both the reference compound and the positive control (dichlorvos) induced NRF2 mediated gene expression while exposure to mannitol did not (Figure 5).
FIGURE 5. Induction of NRF2 mediated gene expression by the reference compound (curcumin) and negative (mannitol) and positive controls (dichlorvos). The results are presented as induction factor, the fold induction over the solvent control. The data are presented as mean values ± S.D. of three independent experiments.
Eight laboratories participated in the “round robin” pre-validation study, of which seven used Fe3O4-PEG-PLGA, Ag, and TiO2 nanomaterials (Figure 6), whereas one partner only used Fe3O4-PEG-PLGA nanomaterials (Supplementary Figure S2). The results consistently showed that TiO2 did not induce NRF2-mediated gene expression. However, exposure to Ag nanomaterials induced NRF2-mediated gene expression in a dose-dependent manner in all experiments (Figures 6A–G). Some differences could be observed in the magnitude of responses (i.e., induction factor) of similar concentration in the different laboratories. It was consistently found that the NRF2 mediated gene expression declined at the highest concentrations which likely is due to the cytotoxicity following exposure to the Ag nanomaterials at the higher concentrations. Finally, following exposure to Fe3O4-PEG-PLGA minimal induction of NRF2-mediated gene expression was observed (Figures 6A–G). Hence, while three of the participating laboratories reported no induction, the results from 5 other laboratories showed a minor induction at 30 or 100 μg/ml, while some reported a lower induction factor for the 100 μg/ml samples compared to 30 μg/ml. Finally the inter and intra-laboratory standard deviations of the assay results were calculated.
FIGURE 6. Interlaboratory study. Induction of NRF2 mediated gene expression following exposure of Fe3O4-PEG-PLGA, Ag nanomaterials and TiO2 nanomaterials. Each graph (A–G) represents the results of an individual laboratory. Each experiment was performed according to the same harmonized protocol. The results are presented as induction factor, the fold induction over the solvent control. The data are presented as mean values ± S.D. of three independent experiment. The numbers represent the individual participating laboratories. Black bars: Fe3O4-PEG-PLGA, light grey bars: Ag; dark grey bars: TiO2.
The inter-laboratory standard deviation ranged from 0.044 to 1.221 with a mean of 0.28 (Figure 7). The mean intra-laboratory standard deviation was 0.16 (Supplementary Figure S3).
FIGURE 7. Heatmap of the interlaboratory standard deviation of the NRF2 induction results from all participating laboratories. The combinations of concentration and nanomaterials that resulted in higher variability of assay results across the partner laboratories are indicated by darker boxes in the heatmap.
To verify the lack of interference of the test materials with the measurement of luciferase activity, the U2OS-NRF2 cells were fixed at the end of exposure by adding 50 µl of paraformaldehyde at 4% in PBS for 30 min at room temperature just before cell lysis to perform the luciferase induction assay as described above. No interference was observed (data not shown).
In this study, we aimed to perform a pre-validation study of a NRF2 reporter gene assay to screen for activation of NRF2 mediated gene expression following exposure to nanobiomaterials, as a proxy for oxidative stress. The pre-validation was conducted through the participation of eight laboratories. Fe3O4-PEG-PLGA nanomaterials were selected as a representative nanobiomaterial and results were compared to “benchmark” nanomaterials from the JRC namely TiO2 (NM101) and Ag (NM300K) along with positive and negative chemical controls. For the TiO2 nanomaterials, none of the participating laboratories observed an induction above the threshold. For the Fe3O4-PEG-PLGA nanomaterials, some laboratories measured an induction just above the threshold while for others the induction levels did not reach the threshold. All laboratories could detect a dose dependent induction following exposure to the Ag nanomaterials (though with different induction factors) indicating that the NRF2 reporter gene assay can be easily applied by different laboratories. Overall, interlaboratory standard deviation was acceptable and the NRF2 reporter gene assay for quantifying oxidative stress caused by nanomaterials is suitable for application in different laboratories. Based on these preliminary findings, we suggest that the assay may be considered for formal validation as an assay for rapid screening of nanobiomaterials.
Engineered nanomaterials can induce ROS production via several mechanisms such as the Fenton reaction, redox cycling, and radical generation (Bi and Westerhoff, 2019), which in turn can activate the NRF2 mediated gene expression. Several previous studies have shown that metal oxides including CuO and ZnO nanomaterials can elicit NRF2 activation (Kim et al., 2021; Zhang et al., 2021). Moreover, Ag nanomaterials exposure was found to trigger NRF2 activation which is thus in line with the present findings. The results from the present study showed that Fe3O4-PEG-PLGA nanomaterials elicited a very modest activation in U2OS-NRF2 cells. However, with respect to Fe3O4-PEG-PLGA, no literature was found on their potential for the induction of NRF2 mediated gene expression. In a recent study different acellular assays along with a HEK293 cell-based NRF2 reporter assay were compared to study the generation of ROS and antioxidant responses of engineered nanomaterials. It is notable that the HEK293 cell-based NRF2 reporter assay did not show any concentration-dependent reactivity for the Fe-based nanomaterials (Seleci et al., 2022), while Fe3O4 nanomaterials have been shown to be able to induce oxidative stress in rodents (Wu et al., 2022). The U2OS-NRF2 cells have a low number of receptors expressed, so that the potential for crosstalk between signal transduction pathways is very low. Furthermore, the U2OS cell line has a low overall metabolic capacity so that reactive compounds, or metabolites have a better chance and a higher sensitivity to activate reporter systems. In comparison to the KeratinoSens™ method validated by OECD (Test No. 442D) for in vitro skin sensitization which has one ARE-response element upfront of the ARE-reporter construct, the U2OS-NRF2 cells as used in the current study has a tandem of four AREs upstream of the luciferase reporter and may thus be more sensitive to inducers acting via the NRF2-pathway. However, care needs to be taken when comparing reporter assays in different cell systems and in vivo data as different abundancies of thiol-containing ligands (i.e., GSH and metallothioneins) can influence the presence of intracellular ROS levels (Bobyk et al., 2019) and may thus influence the sensitivity of cell based NRF2 reporter gene assays.
In addition to the aforementioned in vitro studies, a number of in vivo studies performed in rodents have also shown that nanomaterials can trigger NRF2 activation. For instance, Sun et al. (2012) showed that long-term exposure to TiO2 nanomaterials induced the expression of NRF2, heme oxygenase 1 (HO-1), and glutamate-cysteine ligase catalytic subunit (GCLC). Other investigators have reported that intratracheal administration of ZnO nanomaterials induced elevation of NRF2 and HO-1 expression in the aorta of mice (Zhang et al., 2021). Furthermore, members of the BIORIMA consortium previously investigated the role of NRF2 in pulmonary inflammation following exposure to ZnO nanomaterials using Nrf2-null mice (Sehsah et al., 2019). Mice were administered 20 nm ZnO nanomaterials via pharyngeal aspiration and the study demonstrated infiltration of inflammatory cells in the lung of mice, but minimally induced NRF2-dependent antioxidant enzymes. The authors concluded that NRF2 plays a role in negative regulation on ZnO nanoparticle-induced neutrophil migration (Sehsah et al., 2019).
Several studies have been undertaken in recent years to improve the quality of nanotoxicological investigations including a number of interlaboratory comparisons (aka round robins). For instance, a US consortium funded by the NIEHS conducted cell-based assays on a panel of nanomaterials including several forms of TiO2 and ZnO nanomaterials as well as multi-walled carbon nanotubes focusing on cell viability and cytokine (IL-1β) production (Xia et al., 2013). The importance of using well-characterized nanomaterials and positive and negative controls was emphasized. Several pan-European projects have also addressed the harmonization of in vitro test protocols for the assessment of nanomaterials (e.g., Dusinska et al., 2015; Farcal et al., 2015; Kermanizadeh et al., 2016; Piret et al., 2017). These efforts have put a spotlight on the crucial importance of harmonized test protocols while acknowledging that the path to regulatory-relevant results can be both arduous and long (Teunenbroek et al., 2017).
The OECD Working Party on Manufactured Nanomaterials (WPMN) has reviewed the need for adaptation of the existing OECD Test Guidelines (TGs) and Guidance Documents (GDs) as well as developing new TGs and GDs to address nanomaterials (Rasmussen et al., 2019). Indeed, in the frame of the so-called Malta Initiative, 18 European countries, several Directorates-General of the European Commission, the European Chemicals Agency (ECHA), and other organizations collaborate with the aim of making legislation enforceable, in particular for chemicals (Mech et al., 2022). This European action is currently focused on amending the OECD TGs with respect to nanomaterials to ensure that they are fit-for-purpose. The present reporter gene assay which reflects an important biological endpoint namely oxidative stress is well aligned with these efforts, although further validation is certainly required.
In conclusion, we have successfully performed a pre-validation “round robin” using the NRF2 reporter gene assay using Fe3O4-PEG-PLGA vs. TiO2 (NM101) and Ag (NM300K) nanomaterials. The assay was readily adopted by different laboratories. It is worth noting that other reporter gene assays have previously been subjected to validation and that the estrogen receptor (ER) reporter gene assay and androgen receptor (AR)-reporter gene have recently been included in OECD TG 455 and TG 458, respectively. We suggest that the results of the present interlaboratory study may serve as a starting point for a larger validation study to develop the NRF2 gene reporter assay for the screening of the induction of oxidative stress responses triggered by nanobiomaterials. Indeed, high-throughput screening using in vitro assays could speed up the hazard assessment of nano (bio) materials (Fadeel et al., 2018).
The raw data supporting the conclusions of this article will be made available by the authors, without undue reservation.
NFR2 pre-validation experiments at the participating laboratories were done by SM, LdH, IM, GA, EB, CR, DB, MC, GH, EM, LY, LC, FC, AP, AB, SB, and GI. Characterization of materials including endotoxin assessment: JS, MB, AC, OT, and SD. LdH and HB analyzed the NRF2 data, and KE and AM performed the statistical analysis. BF and HB conceptualized and designed the study and wrote the manuscript. All authors reviewed the final version of the manuscript and approved its submission to the journal.
The work was supported by the European Union’s Horizon 2020 Research and Innovation Programme under grant agreement no. 760928 (BIORIMA). The U2OS-NRF2 cells were kindly provided by Bio Detection Systems (BDS), Amsterdam, Netherlands.
We thank Harrie Besselink (BDS) for arranging the online tutorials on the use of the NRF2-chemically activated luciferase expression (CALUX®) assay. We also acknowledge the SWAXSLab platform (CEA, Université Paris Saclay, France).
The authors declare that the research was conducted in the absence of any commercial or financial relationships that could be construed as a potential conflict of interest.
All claims expressed in this article are solely those of the authors and do not necessarily represent those of their affiliated organizations, or those of the publisher, the editors and the reviewers. Any product that may be evaluated in this article, or claim that may be made by its manufacturer, is not guaranteed or endorsed by the publisher.
The Supplementary Material for this article can be found online at: https://www.frontiersin.org/articles/10.3389/ftox.2022.974429/full#supplementary-material
Aquilano, K., Baldelli, S., and Ciriolo, M. R. (2014). Glutathione: New roles in redox signaling for an old antioxidant. Front. Pharmacol. 5, 196. doi:10.3389/fphar.2014.00196
Bhattacharya, K., Kilic, G., Costa, P. M., and Fadeel, B. (2017). Cytotoxicity screening and cytokine profiling of nineteen nanomaterials enables hazard ranking and grouping based on inflammogenic potential. Nanotoxicology 11 (6), 809–826. doi:10.1080/17435390.2017.1363309
Bi, X., and Westerhoff, P. (2019). Ferric reducing reactivity assay with theoretical kinetic modeling uncovers electron transfer schemes of metallic-nanoparticle-mediated redox in water solutions. Environ. Sci. Nano 6 (6), 1791–1798. doi:10.1039/c9en00258h
Bobyk, L., Tarantini, A., Beal, D., Veronesi, G., Kieffer, I., Motellier, S., et al. (2019). Toxicity and chemical transformation of silver nanoparticles in A549 lung cells: Dose-rate-dependent genotoxic impact. Environ. Sci. Nano 8 (3), 806–821. doi:10.1039/d0en00533a
Cazzagon, V., Giubilato, E., Pizzol, L., Ravagli, C., Doumett, S., Baldi, G., et al. (2022). Occupational risk of nano-biomaterials: Assessment of nano-enabled magnetite contrast agent using the BIORIMA decision support system. NanoImpact 25, 100373. doi:10.1016/j.impact.2021.100373
Choi, J. H., Lee, H., Lee, H., and Lee, H. (2021). Dopant-dependent toxicity of CeO2 nanoparticles is associated with dynamic changes in H3K4me3 and H3K27me3 and transcriptional activation of NRF2 gene in HaCaT human keratinocytes. Int. J. Mol. Sci. 22 (6), 3087. doi:10.3390/ijms22063087
Comero, S., Klein, C., Stahlmecke, B., Romazanov, J., Kuhlbusch, T., Van Doren, E., et al. (2011). NM-300 silver characterisation, stability, homogeneity. EUR 24693 EN. Luxembourg (Luxembourg): Publications Office of the European Union, JRC60709.
Cui, G., Li, Z., Cao, F., Li, P., Jin, M., Hou, S., et al. (2021). Activation of Nrf2/HO-1 signaling pathway attenuates ROS-mediated autophagy induced by silica nanoparticles in H9c2 cells. Environ. Toxicol. 36 (7), 1389–1401. doi:10.1002/tox.23134
D'Elios, M. M., Aldinucci, A., Amoriello, R., Benagiano, M., Bonechi, E., Maggi, P., et al. (2018). Myelin-specific T cells carry and release magnetite PGLA-PEG COOH nanoparticles in the mouse central nervous system. RSC Adv. 8 (2), 904–913. doi:10.1039/c7ra11290d
Dusinska, M., Boland, S., Saunders, M., Juillerat-Jeanneret, L., Tran, L., Pojana, G., et al. (2015). Towards an alternative testing strategy for nanomaterials used in nanomedicine: Lessons from NanoTEST. Nanotoxicology 9 (1), 118–132. doi:10.3109/17435390.2014.991431
Eder, K. M., Marzi, A., Barroso, Á., Ketelhut, S., Kemper, B., and Schnekenburger, J. (2022). Label-free digital holographic microscopy for in vitro cytotoxic effect quantification of organic nanoparticles. Cells 11 (4), 644. doi:10.3390/cells11040644
Fadeel, B., Farcal, L., Hardy, B., Vázquez-Campos, S., Hristozov, D., Marcomini, A., et al. (2018). Advanced tools for the safety assessment of nanomaterials. Nat. Nanotechnol. 13 (7), 537–543. doi:10.1038/s41565-018-0185-0
Farcal, L., Torres Andón, F., Di Cristo, L., Rotoli, B. M., Bussolati, O., Bergamaschi, E., et al. (2015). Comprehensive in vitro toxicity testing of a panel of representative oxide nanomaterials: First steps towards an intelligent testing strategy. PLoS One 10 (5), e0127174. doi:10.1371/journal.pone.0127174
Giubilato, E., Cazzagon, V., Amorim, M. J. B., Blosi, M., Bouillard, J., Bouwmeester, H., et al. (2020). Risk management framework for nano-biomaterials used in medical devices and advanced therapy medicinal products. Materials 13 (20), 4532. doi:10.3390/ma13204532
Kermanizadeh, A., Gosens, I., MacCalman, L., Johnston, H., Danielsen, P. H., Jacobsen, N. R., et al. (2016). A multilaboratory toxicological assessment of a panel of 10 engineered nanomaterials to human health--ENPRA Project--the highlights, limitations, and current and future challenges. J. Toxicol. Environ. Health. B Crit. Rev. 19 (1), 1–28. doi:10.1080/10937404.2015.1126210
Keshavan, S., Gupta, G., Martin, S., and Fadeel, B. (2021). Multi-walled carbon nanotubes trigger lysosome-dependent cell death (pyroptosis) in macrophages but not in neutrophils. Nanotoxicology 15 (9), 1125–1150. doi:10.1080/17435390.2021.1988171
Kim, S. H., Lee, D., Lee, J., Yang, J. Y., Seok, J., Jung, K., et al. (2021). Evaluation of the skin sensitization potential of metal oxide nanoparticles using the ARE-Nrf2 Luciferase KeratinoSensTM assay. Toxicol. Res. 37 (2), 277–284. doi:10.1007/s43188-020-00071-0
Kroll, A., Gietl, J. K., Wiesmüller, G. A., Günsel, A., Wohlleben, W., Schnekenburger, J., et al. (2013). In vitro toxicology of ambient particulate matter: Correlation of cellular effects with particle size and components. Environ. Toxicol. 28 (2), 76–86. doi:10.1002/tox.20699
Li, N., Xia, T., and Nel, A. E. (2008). The role of oxidative stress in ambient particulate matter-induced lung diseases and its implications in the toxicity of engineered nanoparticles. Free Radic. Biol. Med. 44 (9), 1689–1699. doi:10.1016/j.freeradbiomed.2008.01.028
Mech, A., Gottardo, S., Amenta, V., Amodio, A., Belz, S., Bøwadt, S., et al. (2022). Safe- and sustainable-by-design: The case of smart nanomaterials. A perspective based on a European workshop. Regul. Toxicol. Pharmacol. 128, 105093. doi:10.1016/j.yrtph.2021.105093
Miranda, R. R., Oliveira, A. C. S., Skytte, L., Rasmussen, K. L., and Kjeldsen, F. (2022). Proteome-wide analysis reveals molecular pathways affected by AgNP in a ROS-dependent manner. Nanotoxicology 16 (1), 73–87. doi:10.1080/17435390.2022.2036844
Nymark, P., Bakker, M., Dekkers, S., Franken, R., Fransman, W., García-Bilbao, A., et al. (2020). Toward rigorous materials production: New approach methodologies have extensive potential to improve current safety assessment practices. Small 16 (6), e1904749. doi:10.1002/smll.201904749
Piret, J. P., Bondarenko, O. M., Boyles, M. S. P., Himly, M., Ribeiro, A. R., Benetti, F., et al. (2017). Pan-European inter-laboratory studies on a panel of in vitro cytotoxicity and pro-inflammation assays for nanoparticles. Arch. Toxicol. 91 (6), 2315–2330. doi:10.1007/s00204-016-1897-2
Rasmussen, K., Mast, J., De Temmerman, P., Verleysen, E., Waegeneers, N., Van Steen, F., et al. (2014). Titanium dioxide, NM-100, NM-101, NM-102, NM-103, NM-104, NM-105: Characterisation and physico-chemical properties. Luxembourg (Luxembourg): Publications Office of the European Union, JRC86291. EUR 26637.
Rasmussen, K., Rauscher, H., Kearns, P., González, M., and Riego Sintes, J. (2019). Developing OECD test guidelines for regulatory testing of nanomaterials to ensure mutual acceptance of test data. Regul. Toxicol. Pharmacol. 104, 74–83. doi:10.1016/j.yrtph.2019.02.008
Sehsah, R., Wu, W., Ichihara, S., Hashimoto, N., Hasegawa, Y., Zong, C., et al. (2019). Role of Nrf2 in inflammatory response in lung of mice exposed to zinc oxide nanoparticles. Part. Fibre Toxicol. 16 (1), 47. doi:10.1186/s12989-019-0328-y
Seleci, A. G., Tsiliki, G., Werle, K., Elam, D. A., Okpowe, O., Seidel, K., et al. (2022). Determining nanoform similarity via assessment of surface reactivity by abiotic and in vitro assays. NanoImpact 26, 100390. doi:10.1016/j.impact.2022.100390
Sies, H., and Jones, D. P. (2020). Reactive oxygen species (ROS) as pleiotropic physiological signalling agents. Nat. Rev. Mol. Cell Biol. 21 (7), 363–383. doi:10.1038/s41580-020-0230-3
Stone, V., Miller, M. R., Clift, M. J. D., Elder, A., Mills, N. L., Møller, P., et al. (2017). Nanomaterials versus ambient ultrafine particles: An opportunity to exchange toxicology knowledge. Environ. Health Perspect. 125 (10), 106002. doi:10.1289/EHP424
Sun, Q., Tan, D., Zhou, Q., Liu, X., Cheng, Z., Liu, G., et al. (2012). Oxidative damage of lung and its protective mechanism in mice caused by long-term exposure to titanium dioxide nanoparticles. J. Biomed. Mat. Res. A 100 (10), 2554–2562. doi:10.1002/jbm.a.34190
Teunenbroek, T. V., Baker, J., and Dijkzeul, A. (2017). Towards a more effective and efficient governance and regulation of nanomaterials. Part. Fibre Toxicol. 14 (1), 54. doi:10.1186/s12989-017-0235-z
van der Linden, S. C., von Bergh, A. R., van Vught-Lussenburg, B. M., Jonker, L. R., Teunis, M., Krul, C. A., et al. (2014). Development of a panel of high-throughput reporter-gene assays to detect genotoxicity and oxidative stress. Mutat. Res. Genet. Toxicol. Environ. Mutagen. 760, 23–32. doi:10.1016/j.mrgentox.2013.09.009
Wu, L., Wen, W., Wang, X., Huang, D., Cao, J., Qi, X., et al. (2022). Ultrasmall iron oxide nanoparticles cause significant toxicity by specifically inducing acute oxidative stress to multiple organs. Part. Fibre Toxicol. 19 (1), 24. doi:10.1186/s12989-022-00465-y
Xia, T., Hamilton, R. F., Bonner, J. C., Crandall, E. D., Elder, A., Fazlollahi, F., et al. (2013). Interlaboratory evaluation of in vitro cytotoxicity and inflammatory responses to engineered nanomaterials: The NIEHS nano GO consortium. Environ. Health Perspect. 121 (6), 683–690. doi:10.1289/ehp.1306561
Keywords: Nrf2, nanomaterial, interlaboratory validation, oxidative stress, nanotoxicology
Citation: Martin S, de Haan L, Miro Estruch I, Eder KM, Marzi A, Schnekenburger J, Blosi M, Costa A, Antonello G, Bergamaschi E, Riganti C, Beal D, Carrière M, Taché O, Hutchison G, Malone E, Young L, Campagnolo L, La Civita F, Pietroiusti A, Devineau S, Baeza A, Boland S, Zong C, Ichihara G, Fadeel B and Bouwmeester H (2022) Pre-validation of a reporter gene assay for oxidative stress for the rapid screening of nanobiomaterials. Front.Toxicol. 4:974429. doi: 10.3389/ftox.2022.974429
Received: 21 June 2022; Accepted: 09 August 2022;
Published: 05 September 2022.
Edited by:
Vijayakumar Sekar, Shandong University, ChinaReviewed by:
Monika Mortimer, China Jiliang University, ChinaCopyright © 2022 Martin, de Haan, Miro Estruch, Eder, Marzi, Schnekenburger, Blosi, Costa, Antonello, Bergamaschi, Riganti, Beal, Carrière, Taché, Hutchison, Malone, Young, Campagnolo, La Civita, Pietroiusti, Devineau, Baeza, Boland, Zong, Ichihara, Fadeel and Bouwmeester. This is an open-access article distributed under the terms of the Creative Commons Attribution License (CC BY). The use, distribution or reproduction in other forums is permitted, provided the original author(s) and the copyright owner(s) are credited and that the original publication in this journal is cited, in accordance with accepted academic practice. No use, distribution or reproduction is permitted which does not comply with these terms.
*Correspondence: Hans Bouwmeester, aGFucy5ib3V3bWVlc3RlckB3dXIubmw=
†Present address: Antonio Pietroiusti, Saint Camillus International University of Health Sciences, Rome, Italy
Disclaimer: All claims expressed in this article are solely those of the authors and do not necessarily represent those of their affiliated organizations, or those of the publisher, the editors and the reviewers. Any product that may be evaluated in this article or claim that may be made by its manufacturer is not guaranteed or endorsed by the publisher.
Research integrity at Frontiers
Learn more about the work of our research integrity team to safeguard the quality of each article we publish.