- 1Departamento de Neurobiología Conductual y Cognitiva, Instituto de Neurobiología, Universidad Nacional Autónoma de México, Querétaro, México
- 2Departamento de Neurobiología Celular y Molecular, Instituto de Neurobiología, Universidad Nacional Autónoma de México, Querétaro, México
The herbicide atrazine (ATR; 2-chloro-4-ethylamino-6-isopropylamino-s-triazine) is widely used to destroy grasses and broadleaf weeds in crops and some fruits. Studies in rodents have shown that acute, repeated or chronic exposure to ATR is associated with alterations in the nigrostriatal dopaminergic pathway, whereas its effects on GABAergic and glutamatergic pathways have only recently been reported. Sprague-Dawley male rats were exposed daily to 1 or 10 mg ATR/kg of BW for 13 months to evaluate the ATR effects on GABAergic and glutamatergic systems. At the end of the ATR treatment, the levels of mRNA of several genes involved in the production, vesiculation, reuptake, and receptors of GABA and Glu in the striatum (STR), nucleus accumbens (NAcc), prefrontal cortex (PFC), ventral midbrain (vMID) and hippocampus (HIPP) were evaluated by absolute qPCR. For the GABAergic genes, increased expression of GAD67 and Slc32a1 in STR and/or vMID in rats exposed to 1 and/or 10 mg ATR were detected. With regard to the expression of genes involved in the glutamatergic system, Slc17a6 and Grin1 in HIPP of rats exposed to 1 and/or 10 mg ATR, increased as was Gria1 in STR and PFC in the group exposed to 1 mg ATR. In the same fashion, Slc1a3 expression and MGLUR1 increased in STR of rats exposed to 1 and 10 mg ATR groups. The expression of the glutaminases gls (variants 1 and 2) was greater in STR, NAcc, HIPP, and PFC of rats exposed to 1 and/or 10 mg ATR. These findings show that the GABAergic and, especially glutamatergic systems are targets of ATR exposure.
Introduction
Atrazine (ATR; 2-chloro-4-ethylamino-6-isopropylamino-s-triazine) is a selective and systemic organochlorinated herbicide that belongs to the chlorotriazines family. This herbicide is a pre-and post-emergent agent used to kill weeds in corn, wheat, sugar cane cultures, among others (Rowe et al., 2006). The widespread use of ATR in several countries worldwide is associated with high human exposure to tainted waters, soils, and food (Jablonowski et al., 2011; Sun et al., 2017).
Studies in rodents have shown that exposure to ATR is associated with alterations in the basal ganglia nuclei’s integrity. The basal ganglia include the striatum, globus pallidus, substantia nigra, and the subthalamic nucleus. These nuclei’s functionality is regulated by the interaction between the cortex, thalamus, and other brain structures by GABAergic, glutamatergic and dopaminergic systems. The basal ganglia nuclei that have GABAergic projection neurons are striatum, entopeduncular nucleus (EP) or internal segment of the globus pallidus in primates (Gpi), substantia nigra pars reticulata (SNpr) (located in the ventral midbrain), and the external segment of the globus pallidus in primates (GPe) (Bolam et al., 2000). It is important to note that the cortex innervates the striatum by a glutamatergic projection; another structure leading to a glutamatergic projection is the subthalamic nucleus that sends its projections to the output nuclei (GPi/SNpr) (Bolam et al., 2000). Furthermore, the substantia nigra pars compacta and ventral tegmental area send their dopaminergic projections to the striatum and nucleus accumbens, respectively (Cooper et al., 2003). It is important to note that these nuclei regulate movement, reward, cognition, memory, and other brain functions.
Several studies have described the effects of ATR exposure on the basal ganglia, the nigrostriatal dopaminergic system being the most studied under several paradigms of treatment such as acute, repeated, or chronic administration. Regarding chronic ATR exposure, daily exposure to 10 mg ATR/kg for 1 year produces hyperactivity, decreases striatal dopamine levels, and diminishes the number of tyrosine hydroxylase positive cells in the substantia nigra pars compacta (Bardullas et al., 2011; Bardullas et al., 2013). The repeated exposure to 100 mg ATR/kg (six IP injections over 2 weeks) causes hypoactivity, decreases in the in striatal specific binding to D1-DA receptors, decreases the striatal levels of dopamine, and produces alterations in the mRNA expression of vesicular monoamine transporter-2 (VMAT-2), dopamine transporter (DAT) and tyrosine hydroxylase (TH) (Rodriguez et al., 2013; Marquez-Ramos et al., 2017) of the male albino rat. While Coban and Filipov (2007) reported decreases in striatal dopamine levels accompanied by a reduced number of tyrosine hydroxylase positive cells in the substantia nigra pars compacta after daily injection of 5—250 mg ATR/kg during 14 days in the C57BL6J male mice.
However, few studies have evaluated the toxic effects of ATR exposure in the GABAergic or glutamatergic neurotransmitter systems in rodents. In this respect, Shafer et al. (1999) found that ATR can interfere with the binding of Ro15-4513 (an agonist of the benzodiazepine site) GABA-A receptors in a non-competitive manner in brain cortical tissue of rats. While Rodriguez et al. (2017) found that the acute exposure to 100 mg/kg ATR caused hypoactivity, which was relatively annulled by saclofen (GABA-B antagonist), and increased the number of c-Fos-positive cells in the central amygdala, substantia nigra, subthalamic nucleus, superior colliculus, and thalamus 90 min after the IP injection. This protocol of ATR exposure did not affect GABA or glutamate tissue levels on the striatum, nucleus accumbens, prefrontal cortex, ventral midbrain, dorsal midbrain, amygdala, or hippocampus. A recent study by Chavez-Pichardo et al. (2020) found that rats chronically treated with 1 or 10 mg ATR/kg for 13 months showed hyperactivity, anxiety, and alterations in tissue levels of GABA, glutamate, and glutamine in striatum, nucleus accumbens, ventral midbrain, the amygdala, and the prefrontal cortex. In addition, they also found increased striatal extracellular levels and increased release of glutamate in rats exposed to 10 mg ATR/kg.
This study is a follow-up study of our previous reports with the specific aim to explore the possible toxic effects of the chronic exposure to 1 or 10 mg ATR/kg on the mRNA expression of genes associated with neurotransmitter production upon GABAergic and glutamatergic signaling pathways, as indicators of possible disarrays in the regulation of various genes that could impact cellular functions or neurological pathways in the striatum, nucleus accumbens, prefrontal cortex, ventral midbrain and hippocampus, regions already reported to be affected by ATR exposure in a similar protocol of treatment (Chavez-Pichardo et al., 2020).
Materials and methods
Chemicals
Atrazine was obtained from ChemService (West Chester, PA, United States). Unless otherwise stated, reagents for qPCR were obtained from Sigma-Aldrich (St. Louis, MO, United States).
Animals
Thirty twenty-one-day-old male Sprague-Dawley rats were obtained from Envigo, Mexico (Mexico City, Mexico). Rats were kept under a 12-h inverted light/dark cycle (lights on at 18:00 h) with access to food and water ad libitum.
Ethics
Experiments were approved by the local Committee of Bioethics and carried outfitting to the Official Mexican Standard NOM- 062-ZOO-1999 titled “Especificaciones técnicas para la producción, cuidado y uso de los animales de laboratorio,” which agrees with the guidelines of the Institutional Animal Care and Use Committee Guidebook (NIH Publication 80–23, Bethesda, MD, United States, 1996).
Experimental design
Male rats were randomly divided into three groups at arrival to the laboratory facilities. Two groups (n = 10 rats per group) were daily exposed to 1 or 10 mg ATR/kg of B.W. mixed with regular Lab Diet in hand-made pellets, while the control group received pellets without ATR for 13 months. Once rats reached 300 g, they were kept at this bodyweight by food restriction, as already reported (Chavez-Pichardo et al., 2020). Locomotor activity (24 h) was evaluated monthly for 1 year. After ending ATR treatment, rats were sacrificed by decapitation, the brain was extracted, and the striatum, nucleus accumbens, prefrontal cortex, ventral midbrain, and hippocampus were frozen −80°C until analysis. Tissue was collected to evaluate mRNA expression of genes related to production, vesiculation, reuptake, and receptors of the GABAergic and the glutamatergic systems (Figure 1).
Spontaneous locomotor activity
Rats were individually placed in a locomotor activity chamber where spontaneous locomotor activity was measured, and data was collected for 24-h as previously described by our group (Chavez-Pichardo et al., 2020). The parameters recorded to assess the effects of ATR exposure were horizontal activity described as the total number of beam interruptions on the horizontal axis; vertical episode activity, identified as the total number of rearings recorded on the vertical sensor; and the location parameters margin and center distance, defined as the distance traveled in the margin or the center of the activity chamber during a given period.
Quantitative PCR
Total RNA was extracted from the prefrontal cortex, striatum, nucleus accumbens, hippocampus, and ventral midbrain with Trizol Reagent (Life Technologies) and cDNA was reverse transcribed with Thermo Scientific RevertAid First Strand cDNA Synthesis (Thermo Scientific) from 2 μg of total RNA using 0.5 μg oligo (dT) primer (Thermo Scientific, Wilmington, DE, United States). Specific oligonucleotides were verified with the Real-time qPCR tool IDT. PCR products were obtained using a proofreading DNA polymerase (Thermo Scientific), and they were cloned into pJET1.2/blunt vector (Thermo Scientific). Constructs were verified by sequencing, and standard curves were prepared in the range of 105 to 109 molecules/μL. In all cases, qPCR reactions contained 1 μL of reverse-transcribed product, Maxima SYBR Green/ROX qPCR Master Mix (Thermo Scientific), and 200–500 nm forward and reversed oligonucleotides in a final volume of 10 μL. According to the manufacturer’s instructions, a Step One (Applied Biosystems) instrument was used for detection and data analysis. The absolute mRNA concentration was expressed as molecules per microgram of total mRNA used in the RT reaction, and Ct values were interpolation in the standard curve and normalized using reference gene cyclophilin A in each experimental sample. The list of primers utilized for qPCR are shown in Table 1, and experimental conditions are shown in Table 2.
Statistical analysis
Spontaneous locomotor activity was analyzed using a two-way analysis of variance with repeated measures (RMANOVA; treatment x time) followed by post hoc tests (Fisher’s LSD). The mRNA statistical analysis was evaluated with nonparametric tests (Kruskal-Wallis and Mann-Whitney’s U tests for pairwise comparisons) because the data did not meet the requirements for parametric tests. Statistical significance level p < 0.05 was defined in all cases.
Results
Chronic ATR exposure causes alterations in spontaneous locomotor activity after one year of sustained treatment
Only after 1 year of daily ATR treatment there were found significant treatment effects [F = (2, 27) = 8.392, p = 0.0015] and 24-h sampling effects [F = (7, 189) = 18.768, p < 0.0001] and interaction effects (ATR treatment X sample) [F = (7, 189) = 5.294, p < 0.0001] for vertical episode activity. The one-way ANOVAs unveiled differences among groups along the 24-h-long recording [F´s = (2, 27) = 6.066–8.314, p < 0.05]. The post hoc tests showed hyperactivity during the 24-h recording in the group treated with 10 mg ATR/kg in comparison to 1 mg ATR/kg and control groups (Figure 2). No interaction (ATR treatment x sample) effects were found, and only sample effects were significant for horizontal activity, margin and center distance [F‘s (7, 189) = 30.903–61.165, p < 0.05].
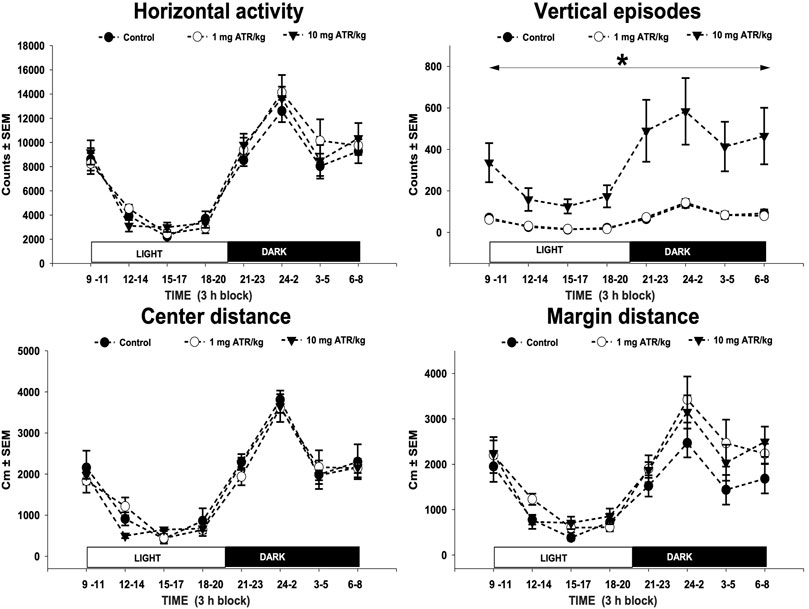
FIGURE 2. Horizontal activity, vertical episode activity, center distance, and margin distance of recorded throughout a 24-h dark/light cycle. Spontaneous locomotor activity was evaluated at 12 months of exposure to 1 or 10 mg ATR/kg. Chronic exposure to 10 mg ATR/kg caused a significant increase in vertical activity in comparison to 1 mg ATR/kg and control groups. Data were analyzed using a two-way analysis of variance with repeated measures (treatment x time) followed by Fisher’s LSD tests for pairwise comparisons.* Denotes differences of 10 mg ATR/kg group in comparison to the 1 mg ATR/kg and control groups, p < 0.05 (n = 10).
Effects of the chronic exposure in the mRNA expression of genes related to the metabolism of GABAergic and glutamatergic systems
To evaluate the effects of chronic ATR exposure on the GABAergic and glutamatergic systems, the mRNAs’ expression for several genes was assessed in the striatum, nucleus accumbens, ventral midbrain, prefrontal cortex, and hippocampus (Table 3).
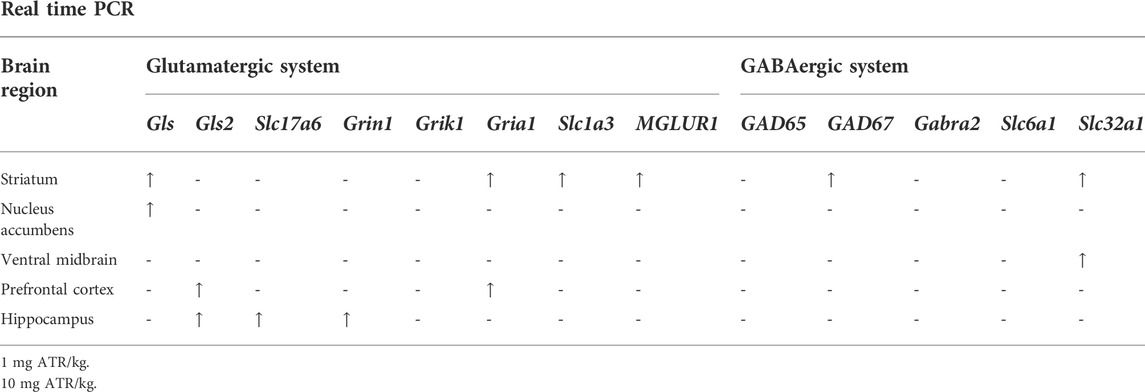
TABLE 3. Summary of the effects of chronic ATR exposure on the expression genes associated with GABAergic and glutamatergic systems.
ATR treatment effects were found for Gls variant 1, Gria1, Slc1a3, MGLUR1, GAD 67, Slc32a1 mRNA expression [H´s = (2, N = 30) = 7.185–10.74, p = 0.0254] in striatum. Post hoc analysis showed that Gls variant 1, Gria1, Slc1a3, MGLUR1, GAD67, and Slc32a1 mRNA expression increased in the 1 mg ATR/kg group (U´s = 5.00–20.00, p < 0.05) while the mRNA expression of Slc1a3 and Slc32a1 increased also in the 10 mg ATR/kg group (U´s = 15.00 and 18.00, p < 0.05) in comparison to the control group (Figure 3).
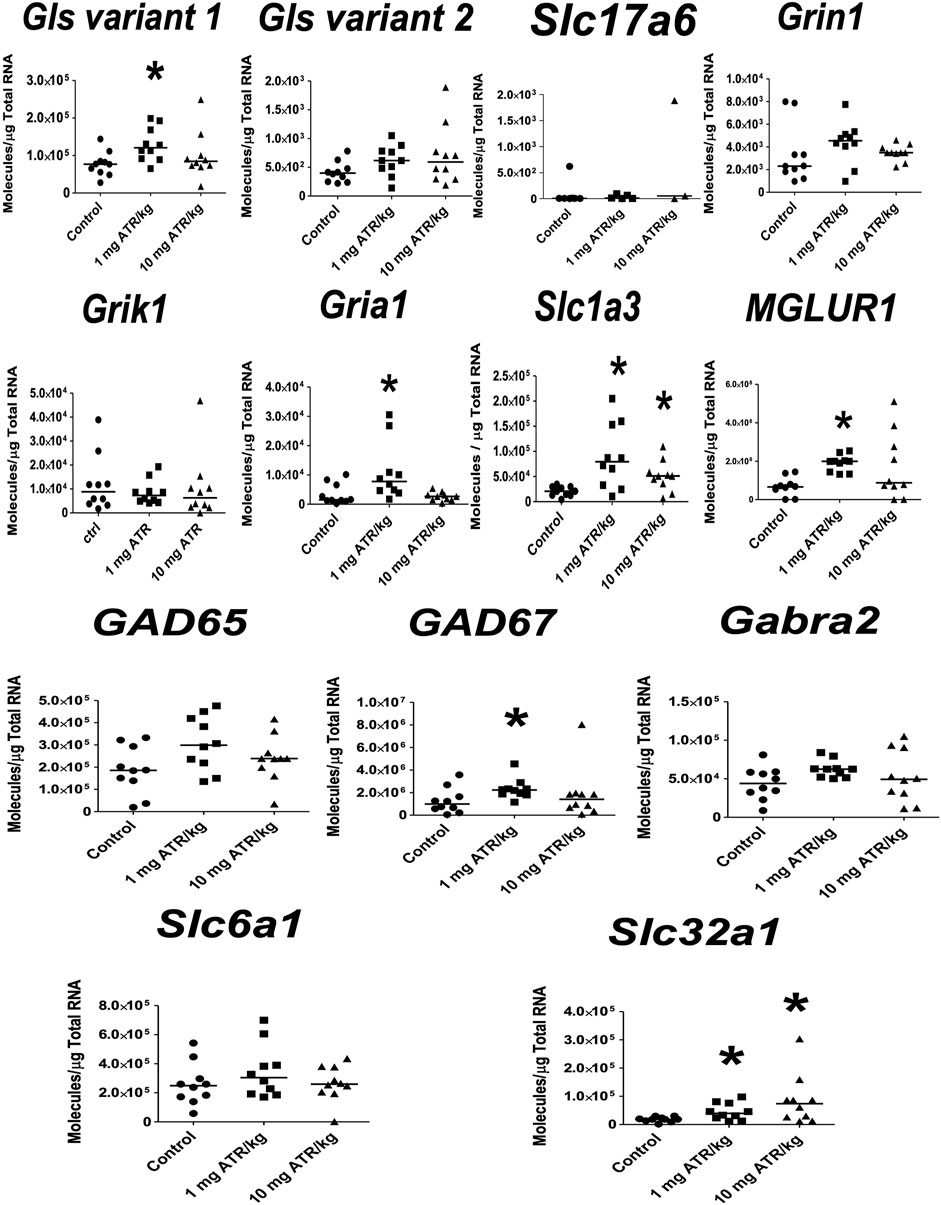
FIGURE 3. Chronic exposure to 1 or 10 mg ATR/kg increased the mRNA expression of glutaminase variant 1 (Gls), N-methyl-D-aspartate receptor (Gria1), excitatory amino acid transporter 1 (Slc1a3), and metabotropic glutamate receptor 1 (MGLUR1) of the glutamatergic system and increased the expression of glutamate decarboxylase 67 (GAD67) and the vesicular GABA transporter (Slc32a1) of the GABAergic system in the striatum. Data were analyzed by Kruskal-Wallis test for overall effects; in case of significant effects, Mann–Whitney U test was used for pairwise comparisons. * Denotes differences between 1 or 10 mg ATR/kg group and the control group, p < 0.05. (n = 10).
In the nucleus accumbens, significant ATR treatment effect was found for Gls variant 1 mRNA expression [H = (2, n = 30) = 14.55, p = 0.007]. Post hoc analysis showed increases in the mRNA expression of Gls variant 1 in the 1 mg ATR/kg group (U = 6.00, p = 0.0003) and the 10 mg ATR/kg group (U = 17.00, p < 0.0115) in comparison to the control group (Figure 4).
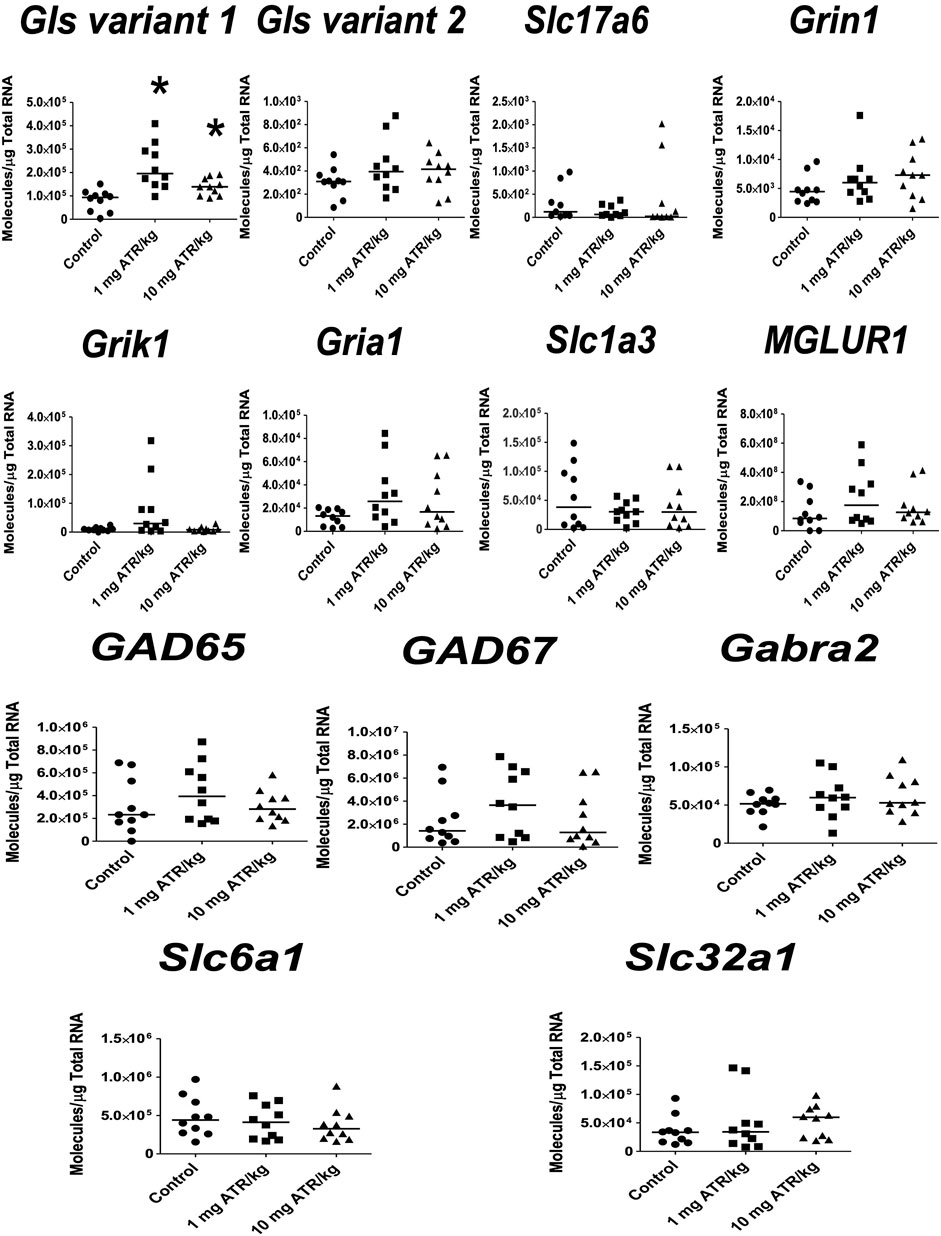
FIGURE 4. Chronic exposure to 1 or 10 mg ATR/kg only increased the mRNA expression of glutaminase variant 1 (Gls) of the glutamatergic system in the nucleus accumbens. Data were analyzed by Kruskal-Wallis test for overall effects; in case of significant effects, Mann–Whitney U test was used for pairwise comparisons. * Denotes differences between 1 or 10 mg ATR/kg group and the control group, p < 0.05. (n = 10).
For the ventral midbrain, only ATR treatment effects were found for Slc32a1 mRNA expression [H = (2, n = 30) = 6.196, p = 0.0451]. Post hoc analysis showed increases in the mRNA expression of Slc32a1 in the 1 mg ATR/kg group (U = 6.00, p = 0.0003) in comparison to the control group (Figure 5).
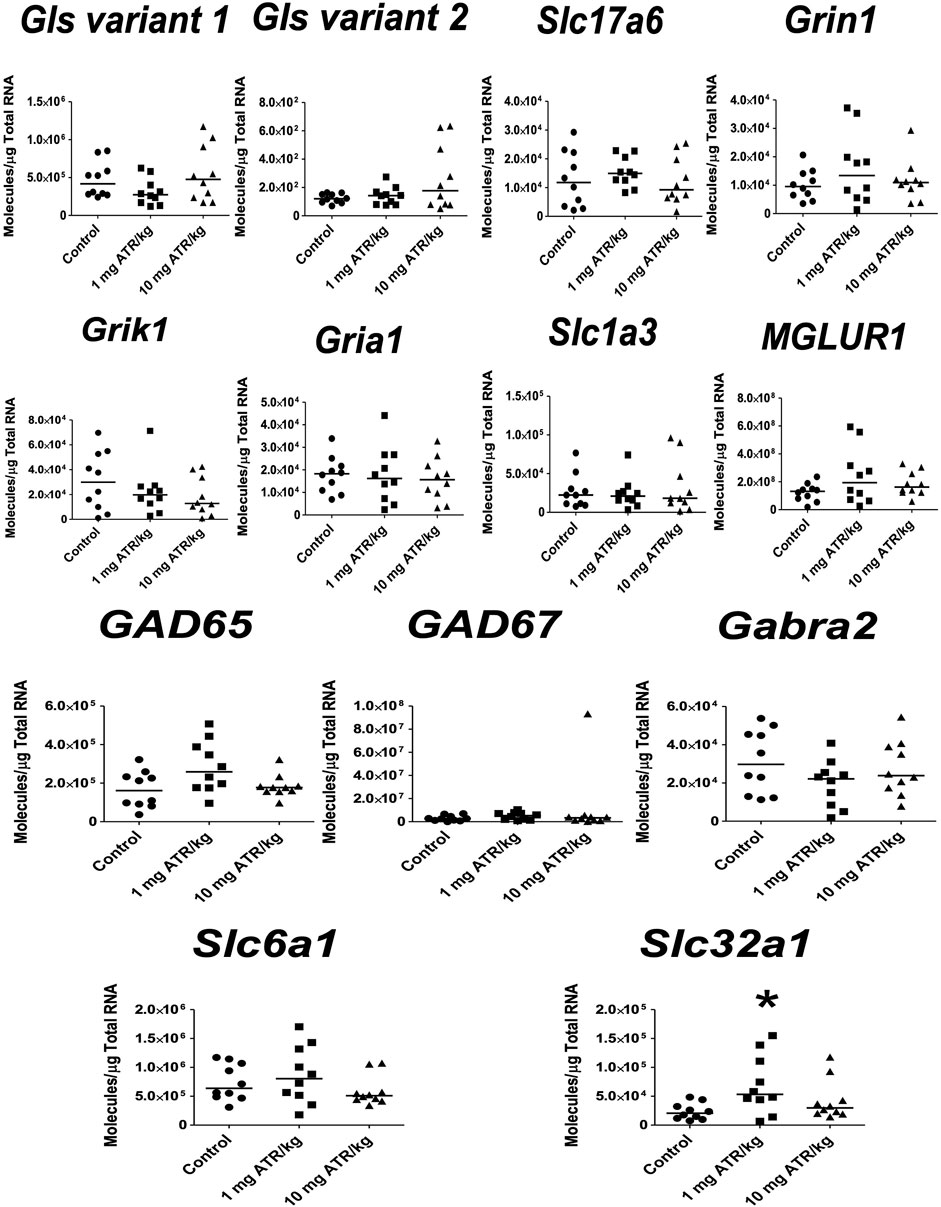
FIGURE 5. Chronic exposure to 1 mg ATR/kg increased the mRNA expression of vesicular GABA transporter (Slc32a1) of the GABAergic system in the ventral midbrain. Data were analyzed by Kruskal-Wallis test for overall effects; in case of significant effects, Mann–Whitney U test was used for pairwise comparisons. * Denotes differences between 1 or 10 mg ATR/kg group and the control group, p < 0.05. (n = 10).
ATR treatment effects were found for Gls variant 2 and Gria1 mRNA expression [H´s = (2, n = 30) = 7.829 and 6.849, p < 0.05] in prefrontal cortex. Post hoc analysis showed that Gls2 and Gria1 mRNA expression increased in the 1 mg ATR/kg group (U´s = 21.00 and 19.00, p < 0.05) while the mRNA expression of Gls variant 2 increased also in the 10 mg ATR/kg group (U = 18.00, p < 0.0171) in comparison to the control group (Figure 6).
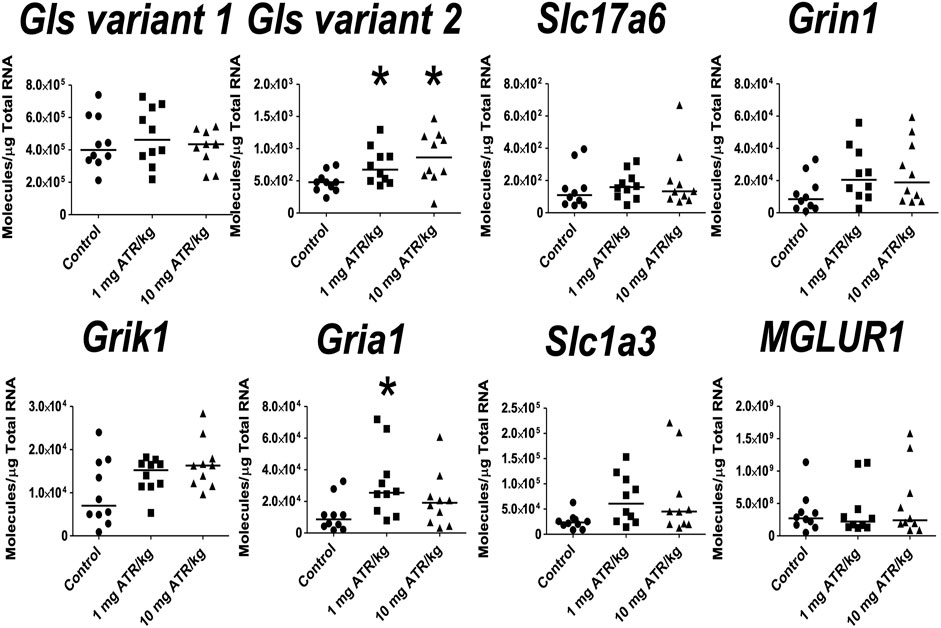
FIGURE 6. Chronic exposure to 1 or 10 mg ATR/kg increased the mRNA expression of glutaminase variant 2 (Gls) and α-amino-3-hydroxy 5 methyl-4-isoxazole propionic acid receptor (Gria1) of the glutamatergic system in the prefrontal cortex. Data were analyzed by Kruskal-Wallis test for overall effects; in case of significant effects, Mann–Whitney U test was used for pairwise comparisons. * Denotes differences between 1 or 10 mg ATR/kg group and the control group, p < 0.05. (n = 10).
In the hippocampus, significant ATR treatment effects were found for Gls variant 2, Slc17a6 and Grin1 mRNA expression [H´s = (2, N = 30) = 8.422–10.54, p < 0.05]. Post hoc analysis showed increase in the mRNA expression of Grin1 in the 1 mg ATR/kg group (U = 13.00, p = 0.0058) and increase of Gls variant 2, Slc17a6 and Grin1 mRNA expression in the 10 mg ATR/kg group (U´s = 13.00–23.00, p < 0.05) in comparison to the control group (Figure 7).
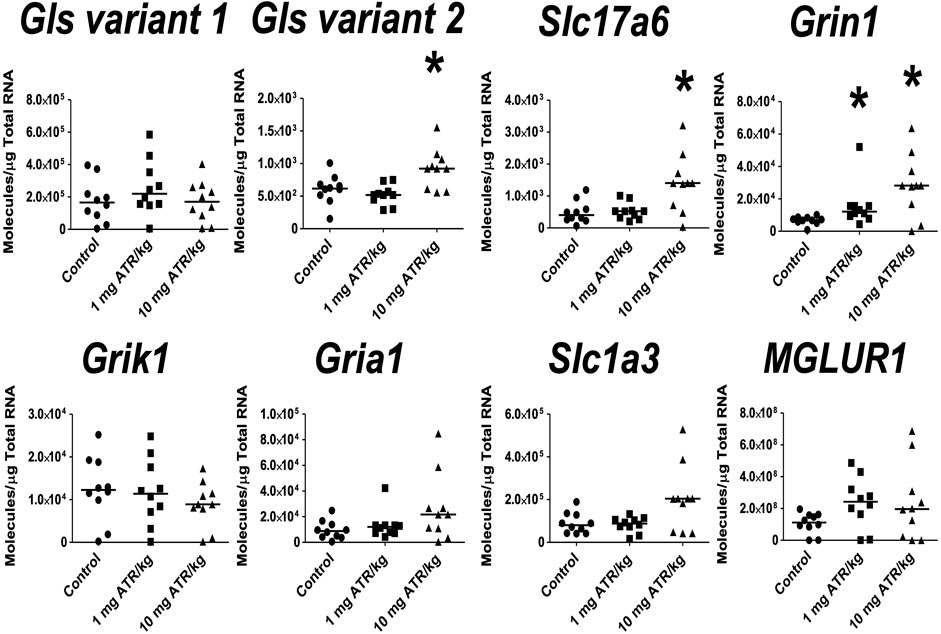
FIGURE 7. Chronic exposure to 1 or 10 mg ATR/kg increased the mRNA expression of glutaminase variant 2 (Gls), vesicular glutamate transporter 2 (Slc17a6), and N-methyl D-aspartate receptor (Grin1) of the glutamatergic system in the hippocampus. Data were analyzed by Kruskal-Wallis test for overall effects; in case of significant effects, Mann–Whitney U test was used for pairwise comparisons. * Denotes differences between 1 or 10 mg ATR/kg group and the control group, p < 0.05. (n = 10).
Discussion
Since 2011 we have been interested in exploring the effect of atrazine on neurotransmitters; we have shown that depending on the dose and length of exposure, there are changes in behavior, activation of brain regions (using the c-Fos expression), and changes in monoamines, GABA, glutamate and glutamine (Bardullas et al., 2011; Bardullas et al., 2013; Rodriguez et al., 2013; Marquez-Ramos et al., 2017; Rodriguez et al., 2017; Chavez-Pichardo et al., 2020). This paper explores the changes at the mRNA levels of several genes involved in the production, vesiculation, reuptake, and receptors of GABA and Glu in the brain of rats chronically exposed to the widely used herbicide atrazine.
Chronic ATR exposure to 1 or 10 mg ATR/kg increased the mRNA expression of some genes of the glutamatergic and GABAergic systems involved in the production, vesiculation, reuptake, and some of its receptors in the prefrontal cortex, nucleus accumbens, striatum, hippocampus, and ventral midbrain. The increased expression of genes related to GABAergic and glutamatergic systems could be related to the hyperactivity found in rats exposed to 10 mg ATR/kg and already reported in this chronic paradigm exposure of ATR (Bardullas et al., 2011; Chavez-Pichardo et al., 2020). These results support our previous study that showed the GABAergic and glutamatergic systems as targets of the chronic ATR exposure and the susceptibility not only of the basal ganglia but other structures such as the hippocampus and the prefrontal cortex.
The striatum is the brain region that presents more changes due to chronic ATR exposure in the mRNA expression of GABAergic and glutamatergic systems. This brain region is composed mainly of GABAergic medium spiny neurons which receive glutamatergic input predominantly from the cortex and the thalamus, and they send GABAergic output to the entopeduncular nucleus (EP), or internal segment of the globus pallidus in primates (Gpi) and/or substantia nigra pars reticulata (SNpr) (Bolam et al., 2000). In the striatum, 1 mg ATR treatment increased the mRNA expression of glutaminase transcript variant 1 (Gls; enzyme present in glutamatergic and GABAergic neurons and astrocytes) (Cardona et al., 2015), its function is to convert glutamine into glutamate and ammonium ions, possible implying the increased production of glutamate in this brain region, which is used as GABA precursor by medium spiny interneurons. The increased mRNA expression of Gls could be associated with a higher demand in glutamate production, which could be associated with the decreased levels of glutamate in the group exposed to 1 mg ATR/kg previously reported by Chavez-Pichardo et al., 2020. In regard to the reuptake of glutamate, we found increased the mRNA expression of the gene of the excitatory amino acid transporter (Slc1a3) (1 and 10 mg ATR), also known as GLAST, present in the glutamatergic neurons, which transports glutamate inside the cell implying the increased reuptake of glutamate by glutamatergic terminals. These results support those from a previous study that reports alterations in glutamate tissue levels and the increased release of basal or evoked glutamate with high potassium in STR (Chavez-Pichardo et al., 2020). Furthermore, there were also increased mRNA expression of subunit 1 of the ionotropic glutamate receptor AMPA (Gria1) (1 mg ATR group) and the glutamate metabotropic receptor 1 (MGLUR1) (1 mg ATR group), which indicates modifications in the fast, as well as slow responses to glutamate in this brain region. Moreover, for the GABAergic system in the striatum, there was increased mRNA expression of the main active enzyme in the brain related to GABA production (GAD67), which catalyzes the decarboxylation of glutamate to GABA in the group chronically exposed to 1 mg ATR. In this respect, GAD67 expression could reflect the increased synthesis of interneuronal GABA within the striatum (Lau and Murthy 2012). Also, increased mRNA expression of the solute carrier family 23 member 1 (Slc32a1) was found, a protein related to vesiculation of GABA in both groups exposed to ATR, implying the increased vesiculation of GABA in the striatum. These alterations in the mRNA expression of these genes could be related to the neurochemical disruptions already reported by Chavez-Pichardo et al. (2020). The increased expression of genes associated with the reuptake of glutamate (Slc1a3), vesiculation of GABA (Slcl32a1), and glutamate receptors (Gria1 and MGLUR1) in both the glutamatergic and GABAergic systems show the susceptibility of these neurotransmitter systems to this herbicide. It is important to note that ATR has been reported to alter striatal dopamine production, reuptake, and vesiculation. In this respect, Hossain and Filipov (2008) found a dose-dependent (1–250 uM) decrease in DA vesiculation using a preparation of synaptic vesicles from striata of Sprague-Dawley rat. These data show that not only the dopaminergic system is affected after exposure to ATR (Coban and Filipov 2007; Bardullas et al., 2011; Bardullas et al., 2013; Lin et al., 2013; Rodriguez et al., 2013; Marquez-Ramos et al., 2017), but that several proteins related to the metabolism of GABAergic and glutamatergic systems are targets of this herbicide.
The NAcc or ventral striatum is a brain region like the striatum composed of GABAergic neurons that receive glutamatergic input from the amygdala, hippocampus, prefrontal cortex, and dopaminergic input from the ventral tegmental area. The NAcc sends GABAergic projections to globus pallidus, pedunculopontine tegmentum, and substantia nigra. It has been assumed that NAcc is a limbic–motor interface that incorporates context information and drives reward to influence motivated behavior (Mogenson et al., 1980). In this region, the chronic exposure to 1 or 10 mg ATR increased mRNA expression of the Gls variant 1, which could be associated with a higher demand for glutamate to produce GABA. A previous study reported increased GABA levels in nucleus accumbens in the group exposed to 10 mg ATR (Chavez-Pichardo et al., 2020). Thus, the increased levels of GABA in NAcc could be contributing to hyperactivity presented by the 10 mg ATR/kg group in this and a previous report (Chavez-Pichardo et al., 2020).
The ventral midbrain is composed of GABAergic, glutamatergic, dopaminergic cells, among other cell types. In this brain region, we found increased mRNA expression of the solute carrier family 23 member 1 (Slc32a1), a protein related to vesiculation of GABA in both groups exposed ATR, possibly implying the increased vesiculation of GABA in the ventral midbrain. This result could be associated with the increased content of GABA in this brain region of rats exposed to 10 mg ATR as reported in a previous study by our group (Chavez-Pichardo et al., 2020). Furthermore, Rodriguez et al. (2013) reported this brain region as a target of ATR exposure when they reported decreased mRNA expression of dopamine transporter (DAT) and tyrosine hydroxylase (th) and increased mRNA expression of the vesicular monoamine transporter-2 (vmat-2) in substantia nigra of Sprague-Dawley rats repeatedly exposed to 100 mg ATR. Together these data could indicate alterations at the vesiculation stage of dopamine and GABA in the ventral midbrain.
The prefrontal cortex has excitatory (glutamatergic) neurons and inhibitory (GABAergic) interneurons expressing several peptides (Szilagyi et al., 2011). PFC plays a vital role in executive functions (goal-directed behavior) such as the decision to execute actions in working memory, depression, among others (Warden et al., 2012). The increased mRNA expression of glutaminase transcript variant 2, also known as Gls-encoded alternative-spliced short transcript glutaminase variant or GAC (Cardona et al., 2015), could be associated with the decreases in glutamine levels found in the prefrontal cortex of rats chronically treated with 1 or 10 mg ATR for 14 months (Chavez-Pichardo et al., 2020). Also, we found increased mRNA expression of subunit 1 of the ionotropic glutamate receptor AMPA (Gria1) (1 mg ATR group), which could indicate alterations in the fast response to glutamate in this brain region. These alterations could be associated with a report by Bardullas et al. (2011), who in rats chronically treated with 10 mg ATR/kg found disruptions on behavioral tasks such as the spontaneous alternation task and in the non-delayed random foraging paradigm, these behavioral tasks are strongly dependent on the integrity of the PFC (Robbins 2005).
Hippocampus is related to learning and memory formation. Like the cortex, the hippocampus is composed of pyramidal cells (glutamatergic cells) and interneurons (GABAergic cells) (Szilagyi et al., 2011). And similar to the prefrontal cortex, chronic ATR exposure to 10 mg ATR/kg increased mRNA expression of glutaminase transcript variant 2, which could be associated with glutamine metabolism alterations. However, there are no reports regarding the effects of ATR exposure on GABA or glutamate metabolism in the literature for comparison. Also, the increased mRNA expression of Slc17a6 implies possible alterations in glutamate vesiculation and consequent glutamate release. Regarding the effects of ATR exposure on glutamate receptors, the increased mRNA expression of glutamate ionotropic receptor NMDA type subunit 1 (Grin1) could indicate modifications in the fast response to glutamate in both groups exposed to ATR. In this respect, some studies have reported increased oxidative stress on this brain region after the oral exposure to 200 mg/kg body weight/day for 30 successive days (Ahmed et al., 2022). Also, maternal or developmental ATR exposure impairs spatial learning and memory, damages hippocampal morphology, and reduces expression of genes and proteins associated with mechanisms of learning and memory (Li et al., 2018; Wang et al., 2019).
Interestingly, in some cases, the 1 mg ATR/kg group but not the 10 mg ATR/kg group showed increases the mRNA expression of particular genes. This finding indicates the possibility of a non-linear relationship between ATR dose and mRNA expression response, a type of response, that is, commonly observed after the exposure to other environmental toxicants, such as lead and arsenic, as well as to endocrine disruptors (Calabrese & Baldwin, 2002; Hirsch et al., 2003; Yang et al., 2007; Lagarde et al., 2015). However, more information is needed regarding the effects of chronic exposure to small doses of ATR to clarify this observation.
These data support the notion that chronic ATR exposure produces alterations in the GABAergic and glutamatergic systems and suggests possible molecular targets in PFC, STR, NAcc, ventral midbrain and the hippocampus, regions involved in movement, addiction, memory and learning, decision making, habituation, among others brain functions. However, further studies are necessary to unravel the risk of exposure to the herbicide atrazine and its association to neurodegenerative disorders.
Data availability statement
The original contributions presented in the study are included in the article/supplementary materials, further inquiries can be directed to the corresponding author.
Ethics statement
The protocol was reviewed and approved by the local Committee on Research Ethics and carried out per the Official Mexican Standard NOM- 062-ZOO-1999 titled “Especificaciones tecnicas para la produccion, cuidado y uso de los animales de laboratorio,” which agrees with the guidelines of the Institutional Animal Care and Use Committee Guidebook (NIH Publication 80‐23, Bethesda, MD, United States, 1996).
Author contributions
DR-B and PV-A performed the gene expression analysis, collected and analyzed the data, and prepared some figures. JA-Z treated the rats and performed behavioral studies. MM-T helped with data and tissue collection. AO and MG contributed to interpreting the results and revised the manuscript. VR designed the experiment, supervised the study, analyzed the data, and wrote the manuscript draft. All authors contributed to the article and approved the submitted version.
Funding
This work was supported by the grants UNAM-DGAPA-PAPIIT 208119 and CONACYT 251510 to VMR. DR-B (628655), and JA-Z (626201) received a fellowship from CONACYT.
Acknowledgments
The authors would like to thank Jorge Humberto Limón-Pacheco for his comments relating to this manuscript.
Conflict of interest
The authors declare that the research was conducted in the absence of any commercial or financial relationships that could be construed as a potential conflict of interest.
Publisher’s note
All claims expressed in this article are solely those of the authors and do not necessarily represent those of their affiliated organizations, or those of the publisher, the editors and the reviewers. Any product that may be evaluated in this article, or claim that may be made by its manufacturer, is not guaranteed or endorsed by the publisher.
References
Ahmed, Y. H., AbuBakr, H. O., Ahmad, I. M., and Ahmed, Z. S. O. (2022). Histopathological, immunohistochemical, and molecular alterations in brain tissue and submandibular salivary gland of atrazine-induced toxicity in male rats. Environ. Sci. Pollut. Res. Int. 29, 30697–30711. doi:10.1007/s11356-021-18399-x
Bardullas, U., Giordano, M., and Rodriguez, V. M. (2013). Atrazine is primarily responsible for the toxicity of long-term exposure to a combination of atrazine and inorganic arsenic in the nigrostriatal system of the albino rat. Neurotoxicol. Teratol. 40, 59–66. doi:10.1016/j.ntt.2013.10.003
Bardullas, U., Giordano, M., and Rodriguez, V. M. (2011). Chronic atrazine exposure causes disruption of the spontaneous locomotor activity and alters the striatal dopaminergic system of the male Sprague-Dawley rat. Neurotoxicol. Teratol. 33 (2), 263–272. doi:10.1016/j.ntt.2010.09.001
Bolam, J. P., Hanley, J. J., Booth, P. A., and Bevan, M. D. (2000). Synaptic organisation of the basal ganglia. J. Anat. 196, 527–542. doi:10.1046/j.1469-7580.2000.19640527.x
Calabrese, E. J., and Baldwin, L. A. (2002). Defining hormesis. Hum. Exp. Toxicol. 21, 91–97. doi:10.1191/0960327102ht217oa
Cardona, C., Sanchez-Mejias, E., Davila, J. C., Martin-Rufian, M., Campos-Sandoval, J. A., Vitorica, J., et al. (2015). Expression of Gls and Gls2 glutaminase isoforms in astrocytes. Glia 63 (3), 365–382. doi:10.1002/glia.22758
Chavez-Pichardo, M. E., Reyes-Bravo, D. Y., Mendoza-Trejo, M. S., Marin-Lopez, A. G., Giordano, M., Hernandez-Chan, , et al. (2020). Brain alterations in GABA, glutamate and glutamine markers after chronic atrazine exposure in the male albino rat. Arch. Toxicol. 94 (9), 3217–3230. doi:10.1007/s00204-020-02806-2
Coban, A., and Filipov, N. M. (2007). Dopaminergic toxicity associated with oral exposure to the herbicide atrazine in juvenile male C57BL/6 mice. J. Neurochem. 100 (5), 1177–1187. doi:10.1111/j.1471-4159.2006.04294.x
Cooper, J. R., Bloom, F. E., and Roth, R. H. (2003). The biochemical basis of neuropharmacology. 8th edn, 1. New York Oxford: University Press.
Hirsch, H. V., Mercer, J., Sambaziotis, H., Huber, M., Stark, D. T., Torno-Morley, T., et al. (2003). Behavioral effects of chronic exposure to low levels of lead in Drosophila melanogaster. Neurotoxicology 24, 435–442. doi:10.1016/S0161-813X(03)00021-4
Hossain, M. M., and Filipov, N. M. (2008). Alteration of dopamine uptake into rat striatal vesicles and synaptosomes caused by an in vitro exposure to atrazine and some of its metabolites. Toxicology 248 (1), 52–58. doi:10.1016/j.tox.2008.03.007
Jablonowski, N. D., Schaffer, A., and Burauel, P. (2011). Still present after all these years: Persistence plus potential toxicity raise questions about the use of atrazine. Environ. Sci. Pollut. Res. Int. 18 (2), 328–331. doi:10.1007/s11356-010-0431-y
Lagarde, F., Beausoleil, C., Belcher, S. M., Belzunces, L. P., Emond, C., Guerbet, M., et al. (2015). Non-monotonic dose-response relationships and endocrine disruptors: a qualitative method of assessment. Environ. Health 11 (14), 13. doi:10.1186/1476-069X-14-13
Lau, C. G., and Murthy, V. N. (2012). Activity-dependent regulation of inhibition via GAD67. J. Neurosci. 32 (25), 8521–8531. doi:10.1523/JNEUROSCI.1245-12.2012
Lau, J. C., Kroes, R. A., Moskal, J. R., and Linsenmeier, R. A. (2013). Diabetes changes expression of genes related to glutamate neurotransmission and transport in the Long-Evans rat retina. Mol. Vis. 19, 1538–1553.
Li, J., Li, X., Bi, H., Ma, K., and Li, B. (2018). Developmental exposure to atrazine impairs spatial memory and downregulates the hippocampal D1 dopamine receptor and cAMP-dependent signaling pathway in rats. Int. J. Mol. Sci. 19 (8), E2241. doi:10.3390/ijms19082241
Lin, Z., Dodd, C. A., and Filipov, N. M. (2013). Short-term atrazine exposure causes behavioral deficits and disrupts monoaminergic systems in male C57BL/6 mice. Neurotoxicol. Teratol. 39, 26–35. doi:10.1016/j.ntt.2013.06.002
Marquez-Ramos, J. A., Hernandez-Plata, I., Diaz-Munoz, M., and Rodriguez, V. M. (2017). The hypoactivity associated with the repeated exposure to atrazine is related to decreases in the specific binding to D1-DA receptors in the striatum of rats. J. Toxicol. 2017, 2169212. doi:10.1155/2017/2169212
Merrill, C. B., McNeil, M., Williamson, R. C., Poole, B. R., Nelson, B., SudweekS, S., et al. (2012). Identification of mRNA for endocannabinoid biosynthetic enzymes within hippocampal pyramidal cells and CA1 stratum radiatum interneuron subtypes using quantitative real-time polymerase chain reaction. Neuroscience 218, 89–99. doi:10.1016/j.neuroscience.2012.05.012
Mogenson, G. J., Jones, D. L., and Yim, C. Y. (1980). From motivation to action: functional interface between the limbic system and the motor system. Prog. Neurobiol. 14 (2-3), 69–97. doi:10.1016/0301-0082(80)90018-0
Robbins, T. W. (2005). Chemistry of the mind: neurochemical modulation of prefrontal cortical function. J. Comp. Neurol. 493 (1), 140–146. doi:10.1002/cne.20717
Rodriguez, V. M., Limon-Pacheco, J. H., Mendoza-Trejo, M. S., Gonzalez-Gallardo, A., Hernandez-Plata, I., and Giordano, M. (2013). Repeated exposure to the herbicide atrazine alters locomotor activity and the nigrostriatal dopaminergic system of the albino rat. Neurotoxicology 34C, 82–94. doi:10.1016/j.neuro.2012.10.012
Rodriguez, V. M., Mendoza-Trejo, M. S., Hernandez-Plata, I., and Giordano, M. (2017). Behavioral effects and neuroanatomical targets of acute atrazine exposure in the male Sprague-Dawley rat. Neurotoxicology 58, 161–170. doi:10.1016/j.neuro.2016.12.006
Rowe, A. M., Brundage, K. M., Schafer, R., and Barnett, J. B. (2006). Immunomodulatory effects of maternal atrazine exposure on male Balb/c mice. Toxicol. Appl. Pharmacol. 214 (1), 69–77. doi:10.1016/j.taap.2005.12.006
Shafer, T. J., Ward, T. R., Meacham, C. A., and Cooper, R. L. (1999). Effects of the chlorotriazine herbicide, cyanazine, on GABA(A) receptors in cortical tissue from rat brain. Toxicology 142 (1), 57–68. doi:10.1016/s0300-483x(99)00133-x
Sun, J. T., Pan, L. L., Zhan, Y., Tsang, D. C., Zhu, L. Z., and Li, X. D. (2017). Atrazine contamination in agricultural soils from the Yangtze River Delta of China and associated health risks. Environ. Geochem. Health 39 (2), 369–378. doi:10.1007/s10653-016-9853-x
Szilagyi, T., Orban-Kis, K., Horvath, E., Metz, J., Pap, Z., and Pavai, Z. (2011). Morphological identification of neuron types in the rat hippocampus. Rom. J. Morphol. Embryol. 52 (1), 15–20.
Wang, D., Li, B., Wu, Y., and Li, B. (2019). The effects of maternal atrazine exposure and swimming training on spatial learning memory and hippocampal morphology in offspring male rats via PSD95/NR2B signaling pathway. Cell. Mol. Neurobiol. 39 (7), 1003–1015. doi:10.1007/s10571-019-00695-3
Warden, M. R., Selimbeyoglu, A., Mirzabekov, J. J., Lo, M., Thompson, K. R., Kim, S. Y., et al. (2012). A prefrontal cortex-brainstem neuronal projection that controls response to behavioural challenge. Nature 492 (7429), 428–432. doi:10.1038/nature11617
Wearne, T. A., Parker, L. M., Franklin, J. L., Goodchild, A. K., and Cornish, J. L. (2016). GABAergic mRNA expression is differentially expressed across the prelimbic and orbitofrontal cortices of rats sensitized to methamphetamine: Relevance to psychosis. Neuropharmacology 111, 107–118. doi:10.1016/j.neuropharm.2016.08.038
Yang, P., He, X. Q., Peng, L., Li, A. P., Wang, X. R., Zhou, J. W., et al. (2007). The role of oxidative stress in hormesis induced by sodium arsenite in human embryo lung fibroblast (HELF) cellular proliferation model. oxidative stress in hormesis induced by sodium arsenite in human embryo lung fibroblast (HELF) cellular proliferation model. J. Toxicol. Environ. Health. A 70 (11), 976–983. doi:10.1080/15287390701290832
Keywords: herbicides, GABA, glutamate, albino rat, neurodegenenerative diseases, mRNA expression
Citation: Reyes-Bravo DY, Villalobos-Aguilera P, Almonte-Zepeda JT, Mendoza-Trejo MS, Giordano M, Orozco A and Rodríguez VM (2022) Chronic atrazine exposure increases the expression of genes associated with GABAergic and glutamatergic systems in the brain of male albino rat. Front. Toxicol. 4:933300. doi: 10.3389/ftox.2022.933300
Received: 30 April 2022; Accepted: 19 July 2022;
Published: 22 August 2022.
Edited by:
Alice Villalobos, Texas Tech University Health Sciences Center, United StatesReviewed by:
Marcia H. Ratner, Boston University School of Medicine, United StatesTauqeerunnisa Syeda, Purdue University, United States
Copyright © 2022 Reyes-Bravo, Villalobos-Aguilera, Almonte-Zepeda, Mendoza-Trejo, Giordano, Orozco and Rodríguez. This is an open-access article distributed under the terms of the Creative Commons Attribution License (CC BY). The use, distribution or reproduction in other forums is permitted, provided the original author(s) and the copyright owner(s) are credited and that the original publication in this journal is cited, in accordance with accepted academic practice. No use, distribution or reproduction is permitted which does not comply with these terms.
*Correspondence: V. M. Rodríguez, dm1yb2RyaWd1ZXpAY29tdW5pZGFkLnVuYW0ubXg=