Commentary: Considering intrauterine location in a model of fetal growth restriction after maternal titanium dioxide nanoparticle inhalation
- 1Department of Pharmacology and Toxicology, Ernest Mario School of Pharmacy, Rutgers University, Piscataway, NJ, United States
- 2Environmental and Occupational Health Sciences Institute, Piscataway, NJ, United States
Fetal growth restriction (FGR) is a condition with several underlying etiologies including gestational disease (e.g., preeclampsia, gestational diabetes) and xenobiotic exposure (e.g., environmental contaminants, pharmaceuticals, recreational drugs). Rodent models allow study of FGR pathogenesis. However, given the multiparous rodent pregnancy, fetal growth variability within uterine horns may arise. To ascertain whether intrauterine position is a determinant of fetal growth, we redesigned fetal weight analysis to include litter size and maternal weight. Our FGR model is produced by exposing pregnant Sprague Dawley rats to aerosolized titanium dioxide nanoparticles at 9.44 ± 0.26 mg/m3 on gestational day (GD) 4, GD 12 or GD 17 or 9.53 ± 1.01 mg/m3 between GD 4-GD 19. In this study fetal weight data was reorganized by intrauterine location (i.e., right/left uterine horn and ovarian/middle/vaginal position) and normalized by maternal weight and number of feti per uterine horn. A significant difference in fetal weight in the middle location in controls (0.061 g ± 0.001 vs. 0.055 g ± 0.002), GD 4 (0.033 g ± 0.003 vs. 0.049 g ± 0.004), and GD 17 (0.047 g ± 0.002 vs. 0.038 g ± 0.002) exposed animals was identified. Additionally, GD 4 exposure produced significantly smaller feti in the right uterine horn at the ovarian end (0.052 g ± 0.003 vs. 0.029 g ± 0.003) and middle of the right uterine horn (0.060 g ± 0.001 vs. 0.033 g ± 0.003). GD 17 exposure produced significantly smaller feti in the left uterine horn middle location (0.055g ± 0.002 vs. 0.033 ± 0.002). Placental weights were unaffected, and placental efficiency was reduced in the right uterine horn middle location after GD 17 exposure (5.74 g ± 0.16 vs. 5.09 g ± 0.14). These findings identified: (1) differences in fetal weight of controls between the right and left horns in the middle position, and (2) differential effects of single whole-body pulmonary exposure to titanium dioxide nanoparticles on fetal weight by position and window of maternal exposure. In conclusion, these results indicate that consideration for intrauterine position, maternal weight, and number of feti per horn provides a more sensitive assessment of FGR from rodent reproductive and developmental studies.
Introduction
Fetal growth restriction (FGR) is a pathology where the full in utero growth potential for a fetus is not met during the period of gestation (Wollmann, 1998). In the clinical terms, this is identified as birth weight below the 10th percentile on a singleton growth curve (Bamfo and Odibo, 2011). Concerningly, a FGR diagnosis is commonly accompanied by other immediate health concerns of perinatal death and neonatal complications. Surviving newborns face an increased likelihood of developing cardiovascular disease, asthma, type 2 diabetes, and metabolic disorders later in life (Hales et al., 1991; Martyn et al., 1995; Curhan et al., 1996; Leon et al., 1998; Barker, 2006; Xu et al., 2014). Because of these associations with short- and long-term health conditions, FGR is a condition of concern for obstetric, pediatric, and primary care practitioners as well as reproductive and developmental scientists. Although a large body of work has identified several genetic and environmental etiologies of FGR, it is clear that many have yet to be uncovered (Wollmann, 1998; Sharma et al., 2016). Recently, accumulating evidence has suggested that pregnant women environmentally exposed to components of air pollution (e.g., fine/ultrafine fractions of particulate matter) or occupationally exposed to nanoparticles are at increased risk for FGR (Rogers and Dunlop, 2006; Manangama et al., 2019; Bekkar et al., 2020).
Laboratory studies have helped to unveil the molecular mechanisms and potential therapeutics of common gestational diseases and disorders. These may include models of preeclampsia, gestational diabetes, genetic abnormalities, infections, and maternal environmental exposures (e.g., high altitude, phthalates, heavy metals, and ultrafine particles), each likely to result in the development of FGR (Yamashita et al., 2003; Arce et al., 2012; Tunster et al., 2014; Xu et al., 2016; Shen et al., 2017; Bailey et al., 2019; Morales-Rubio et al., 2019; Tachibana et al., 2019; Chen et al., 2020). It is estimated that 95% of the animals used in these studies are multiparous rodents, including mice and rats (Vandamme, 2014). While these models have proven beneficial for understanding FGR and developmental and reproductive science, the nature of rodent uterine anatomy and placentation has inherent differences from humans.
A cornerstone of Developmental and Reproductive Toxicology (DART) studies is fetal weight evaluation. This involves calculating the average weight of a litter per dam, and subsequently averaging litter weights per experimental group, which for our purposes we will refer to as the “traditional” approach (Lazic and Essioux, 2013). This traditional approach may detect a significant impact on fetal body weight, but without consideration for variability between feti in specific uterine locations, right and left uterine horns, litter size, or maternal body weight. Studies utilizing a fetal pig model have shown that there is intra-litter variability in fetal growth, in that implantation in the middle of a uterine horn produces smaller feti than those deposited at the ends of the uterine horn (e.g., near the ovary or vagina) (Perry and Rowell, 1969; Jang et al., 2014). Furthermore, human studies identify that feti which implant on the lateral aspects of the uterus are more likely to be smaller and are at a higher risk for the development of preeclampsia, miscarriage, or preterm birth compared with feti that implant in anterior or posterior positionings (Gonser et al., 1996; Kalanithi et al., 2007; Magann et al., 2007). Particularly hazardous implantation sites that are low in the uterine body can result in placental growth that partially or entirely covers the cervix, a condition known as placenta previa (Balayla et al., 2019). The uterine tissue around the cervix does not have a strong blood supply and is not well-perfused; therefore, fetal implantations diagnosed with placenta previa are at heightened risk for placental ischemia, placental hypoxia, and reduced fetal growth (Harper et al., 2010). This evidence that implantation site is a factor in fetal growth underscores a need to account for intrauterine position in laboratory DART studies, especially when considering pregnancies that result in multiple offspring. Unfortunately, few rodent investigations have examined whether intrauterine position influences fetal body weight at term, thereby contributing to study variability.
The number of feti in a litter is negatively correlated with birth weight (Romero et al., 1992; Ishikawa et al., 2006; McLaurin and Mactutus, 2015). Romero and colleagues reported an inverse relationship between fetal weight and litter size in Sprague Dawley (SD) rats, and emphasized that if this relationship is not taken into account when toxicity on fetal weight is analyzed there is a potential to mask a decrease in fetal weight due to litter size reduction (Romero et al., 1992). Importantly, the number of feti deposited within the right and left uterine horns, respectively, not total litter size, determines the influence of the intrauterine position effect; therefore, experimental outcomes should be evaluated on a per-horn basis (Raz et al., 2012; McLaurin and Mactutus, 2015). Lastly, maternal weight may be an important consideration, as smaller dams may have less energy reserve per fetus to produce feti of a similar weight compared to a larger female (Thame et al., 2015).
The impact of environmental exposure during pregnancy affecting intrauterine positional growth has received little attention. Our model of maternal exposure to titanium dioxide nanoparticle (nano-TiO2) aerosols during gestation has identified impairments in gestational health (Stapleton et al., 2013; Fournier et al., 2019). Utilizing this model, we have previously reported effects on fetal reabsorption and placental and fetal weight depending on the window(s) of gestational exposure (Stapleton et al., 2013; Fournier et al., 2019). Therefore, the purpose of this study was to develop a stepwise method of analysis to organize and normalize fetal growth to more accurately assess FGR in our model. We evaluated fetal weight in a gravid SD rat model under control conditions and after maternal exposure to nano-TiO2 aerosols by position, maternal GD 20 weight, and number of feti per uterine horn. To ascertain whether exposure caused FGR in a particular uterine location and if the timing of the exposure generated a different outcome, the analysis was conducted with data from four different exposure scenarios: single exposure on gestational day (GD) 4, GD 12, or GD 17, and repeat exposures occurring between GD 4 and GD 19. This challenges the traditional methods and serves useful for future investigations of fetal growth.
Methods
Animals
Timed-pregnant SD rats were purchased from Charles River Laboratories (Kingston, NY). Animals were single housed in an AAALAC-approved Rutgers University vivarium where they were allowed access to Purina 5053 chow and water ad libitum. Animals arrived on GD 2 or GD 3. After a 24-h acclamation period, animals were randomly assigned to control (n = 21), filtered-air control (n = 6), or nano-TiO2 exposed groups (n = 6–9). Nano-TiO2 groups received a single exposure on GD 4, GD 12, or GD 17 as described previously (Fournier et al., 2019) or repeat exposures between GD 4 and GD 19 to aerosolized TiO2 nanoparticles via whole-body inhalation. Control animals were exposed to filtered air via whole-body inhalation. Naïve animals were not subjected to the inhalation facility. All procedures were approved by the Institutional Animal Care and Use Committee at Rutgers University.
Particle Characterization
Powdered titanium dioxide was purchased from Evonik (Aeroxide TiO2, Parsippany, NJ). Previous characterization of the material determined a composition to be primarily anatase (80%) and rutile (20%) (Fournier et al., 2019). Primary particle size was determined to be 21 nm with a mean surface area of 48.08 mg2/g (Fournier et al., 2019).
Inhalation Exposure to Aerosolized Titanium Dioxide Nanoparticles
The nano-TiO2 preparation and aerosol exposure has been detailed from one other study previously published (Fournier et al., 2019). Briefly, animals were administered whole-body inhalation exposures in a custom rodent inhalation facility (IEStechno, Morgantown, WV). The size distribution and relative mass of the particle aerosols were monitored in real time with a Scanning Mobility Particle Sizer (SMPS, TSI, Shoreview, MN). Aerosols were collected on a 47-nm PTFE membrane filter for gravimetric sampling to confirm concentration. The exposures were carried out for 4-hr on single days (i.e., GD 4, GD 12, GD 17) or took place repeatedly 5 days/weeks from GD 4 through GD 19. Average aerosol concentrations were measured at 9.44 ± 0.26 mg/m3 and 9.53 ± 1.01 mg/m3 for 4-h single and repeat exposures, respectively.
Cesarean Procedure and Data Collection
On GD 20 animals were anesthetized via isoflurane inhalation (i.e., 5% induction, 3% maintenance) and positioned supine. Surgical scissors were used to create a Y-shaped incision through the abdomen to expose the uterus. The left and right gravid uterine horns were identified, removed, and individually pinned to a dissecting dish positioned with the ovary to the left and the vaginal end to the right.
Using a surgical scissor, the uterine muscle was cut lengthwise to reveal placentas, amniotic sacs, and feti. The fetus from the ovary-most end was designated as fetus number one (Figure 1). Moving toward the vaginal end, each fetus was numbered, removed from its amniotic sac, and weighed. Associated placentas were weighed, and wet weights were recorded.
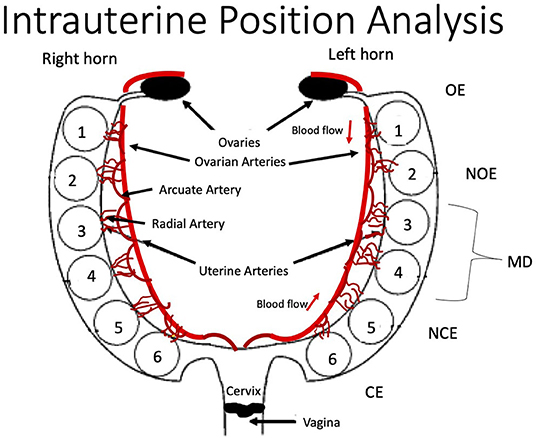
Figure 1. Schematic of rodent uterine horn anatomy from the ventral aspect and acronym key. Feti are numbered within each horn from the ovary end (OE) as fetus one to the cervical end (CE) as fetus six. Anatomy of major uterine and ovarian arteries and associated arcuate and radial artery branching is displayed. OE, Ovarian End; NOE, Next-to Ovarian End; MD, Middle; NCE, Next-to Cervical End; CE, Cervical End.
Protocol for Intrauterine Position Analysis
The inclusion criteria in this analysis required a uterine horn to have at least five feti. Dams that had <5 feti in the litter were excluded from analysis (Table 1). Right or left uterine horns that had <5 feti were also excluded from analysis. Data from horns that met inclusion criteria were organized by intrauterine position [i.e., right/left ovary end (R/L OE), right/left next to ovary end (R/L NOE), right/left middle (R/L MD), right/left next to cervical end (R/L NCE) and right/left cervical end (R/L CE)] (Figure 1). Feti in the middle of uterine horns with more than five feti were averaged for the “middle” category.

Table 1. Reported number of litters and uterine horns excluded from analysis and older analyses of fetal weight demonstrating per dam and per treatment group averages along with percent reduction in fetal weight.
Existing literature has shown that maternal horn size and litter size can influence the intrauterine position effect in the Sprague Dawley rat (Raz et al., 2012; McLaurin and Mactutus, 2015). To correct for differences in maternal weight and litter size, each fetal weight was normalized to the number of feti within that horn by the following equation:
Calculation of Placental Efficiency
Placental efficiency, a ratio of fetal weight to placental weight, is often used as a proxy measurement of placental function in human and laboratory animal studies (Hayward et al., 2016). Placental efficiency was calculated by dividing the raw fetal weight by its respective raw placental weight that were measured on GD 20.
Statistical Analysis
A Grubb's test was employed to remove any outliers from the data set before further analysis. Student's t-test was then used to compare fetal weights using the traditional approach. Fetal weights were also analyzed by arranging and normalizing according to our developed protocol, the individual intrauterine positions from each group were compared between the right and left horn using a two-way ANOVA and Sidak's multiple comparisons test. The nano-TiO2 exposed group was compared with controls by position for the right and left horn using a two-way ANOVA and Sidak's multiple comparison test. Placental weights and placental efficiencies, calculated as a ratio of fetal weight to placental weight, were evaluated with a similar approach using raw unnormalized values. Effect sizes were calculated and included as Supplementary Table 1. Statistical analysis was conducted with GraphPad Prism 8.0 (San Diego, CA, USA). Data is reported as mean ± standard error. Statistical significance was set to p < 0.05 and is indicated with an asterisk (*).
Results
Traditional Analysis of Fetal Weight
Fetal body weight was compared between control dams exposed to filtered air in our inhalation exposure facility and naïve animal that never entered the facility using both the traditional and developed approaches. As in previous assessments, no statistical differences were observed (data not shown) between control filtered air exposed or naïve groups (Fournier et al., 2019). Therefore, in this study, all assessments compared each nano-TiO2 exposed group to a group naïve animals identified as “control.”
Fetal body weights were compared using the traditional approach of averaging fetal weight by litter and then by treatment group (Table 1). No significant differences and thus no FGR was found using this approach.
Intrauterine Position on Fetal Body Weight
Fetal weights were compared using our developed approach by organizing data by intrauterine position and normalizing by maternal weight at necropsy (GD 20) and number of feti per horn. In controls, average of total fetal weights from the left horn (0.053 g ± 0.001) were smaller than those in the right horn (0.058 g ± 0.001) (p = 0.08). When the overall right and left horn were compared for exposure groups, repeat and GD 17 exposure resulted in significantly smaller feti in the left horn (p = 0.02 and 0.01, respectively). GD 4 exposure resulted in significantly smaller feti in the right horn (p < 0.0001) (Figure 2A). GD 4 and GD 17 also produced significantly smaller pups (p < 0.0001 each) in the right horn, as compared to control growth in the right horn (Figure 2B). Comparisons for the left horn produced significantly smaller pups on GD 17 (p < 0.0001) (Figure 2B).
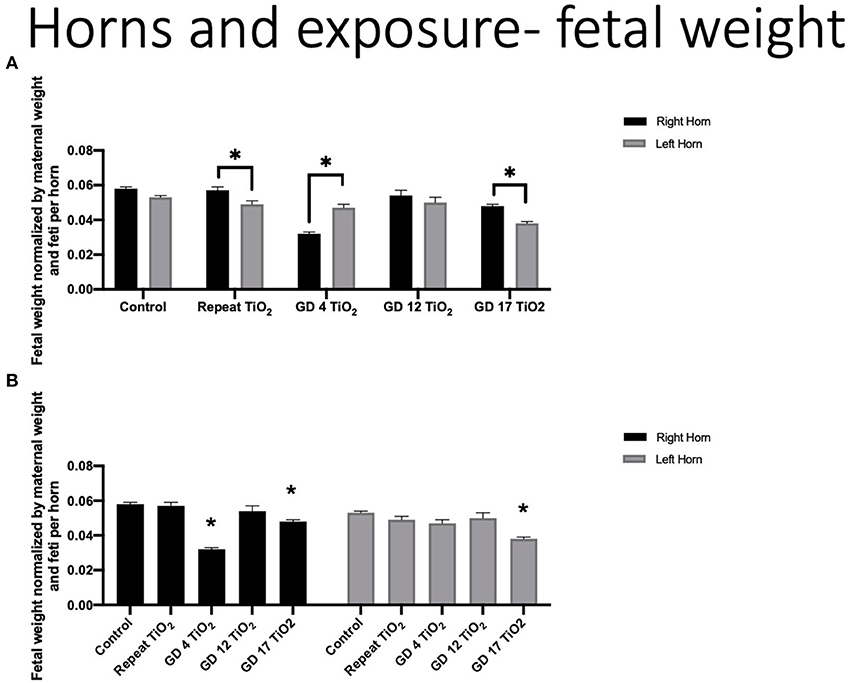
Figure 2. Fetal weight normalized by maternal weight on GD 20 and number of feti per uterine horn. (A) Normalized fetal weights comparing the right and left horn within each exposure timing condition. This analysis identifies differing fetal growth between the uterine horns within the same experimental condition. (B) Normalized fetal weight of the right and left horns for each exposure group compared to control. This analysis identifies fetal growth impaired by maternal exposure to nano-TiO2 during gestation. Significance is set to p < 0.05, indicated as * and values are shown as mean ± SEM.
When positional outcomes (Figure 1) were included in the analyses, fetal weights were significantly smaller in the LMD position compared with the RMD position in controls (LMD 0.054 g ± 0.002 vs. RMD 0.060 g ± 0.001) (Figure 3A). The feti with the lowest weight in control dams were in the LOE and LNOE positions.
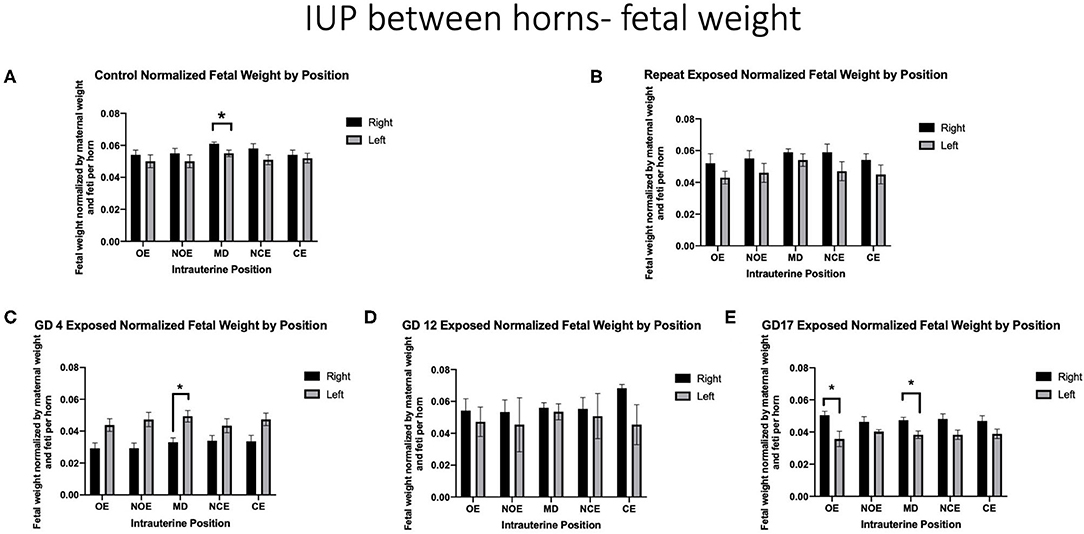
Figure 3. Fetal weight normalized by maternal weight on GD 20 and number of feti per horn. Data is analyzed by anatomical uterine position to identify differences between right and left uterine horns in (A) control and (B–E) exposure groups. Significance is set to p < 0.05, indicated as * and values are shown as mean ± SEM.
When analyzing fetal body weights, exposure to a single inhalation of nano-TiO2 during pregnancy had a differential effect on fetal weight by intrauterine location based on the timing of exposure. Dams that were repeatedly exposed had no position that was significantly impacted (Figure 3B). Dams exposed on GD 4 had significantly smaller fetal weight in the RMD location compared with the LMD location (0.033 g ± 0.003 vs. 0.049 g ± 0.004, respectively) (Figure 3C). Exposure on GD 12 resulted in no significant differences between intrauterine locations within and between right and left horns (Figure 3D). Damns exposed on GD 17 had significantly smaller feti on the LOE (0.036 g ± 0.005 vs. 0.051 g ± 0.002) and the LMD (0.038 g ±0.002 vs. 0.047 g ± 0.002) locations (Figure 3E).
When comparing fetal weights between exposure groups on a per-location basis, exposure on GD 4 had a statistically significant impact on the ROE (0.052 g ± 0.003 vs. 0.029 g ± 0.003), RNOE (0.053 g ± 0.004 vs. 0.029 g ± 0.003), the RMD (0.060 g ± 0.001 vs. 0.033 g ± 0.003) and the RNCE (0.055 g ± 0.004 vs. 0.034 g ± 0.003) locations as compared to control (Figure 4). No significance was found between control and repeated exposed or control and GD 12 exposed groups at any intrauterine location. Exposure on GD 17 had a statistically significant impact, compared to control, on the LMD location (p = 0.003).
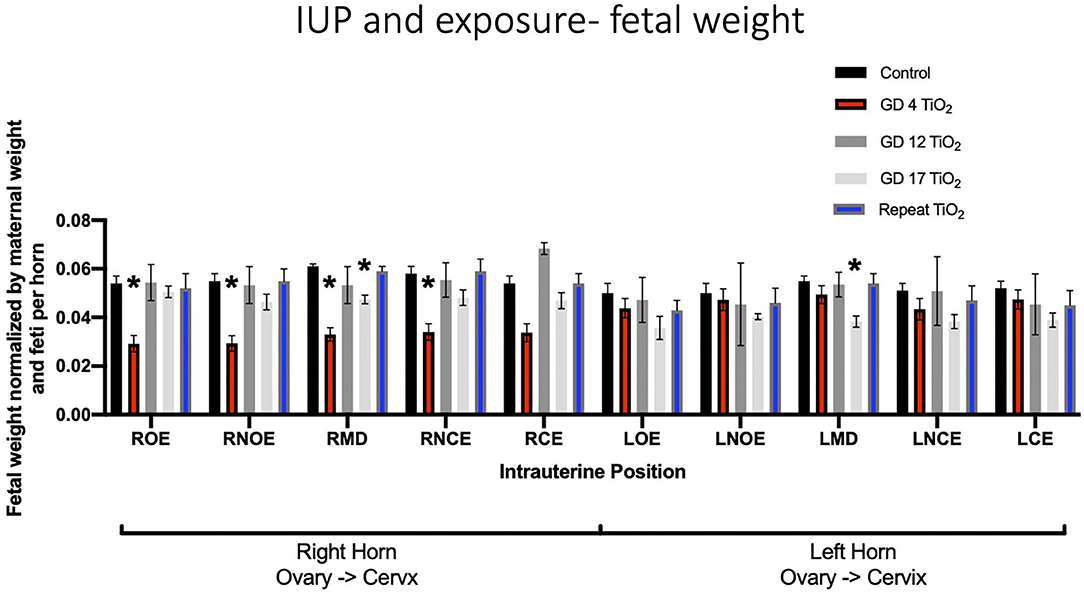
Figure 4. Fetal weight normalized by maternal weight on GD 20 and number of feti per horn. This analysis pinpoints intrauterine positions which are susceptible to growth reduction after certain timing of exposure(s) compared to controls. Significance is set to p < 0.05, indicated as *, and values are shown as mean ± SEM.
Intrauterine Position Effect on Placental Weight
When analyzing for placental weight under control conditions, there was no significant impact on placental weight within and between the right and left uterine horns in control animals (Figure 5A). Placental weights were not impacted within or between intrauterine locations in the right and left uterine horns with repeated or single exposures to nano-TiO2 (Figures 5B–E). Comparison of raw placental weights at each intrauterine location between each experimental group and the control group showed no significance differences (Figure 6).
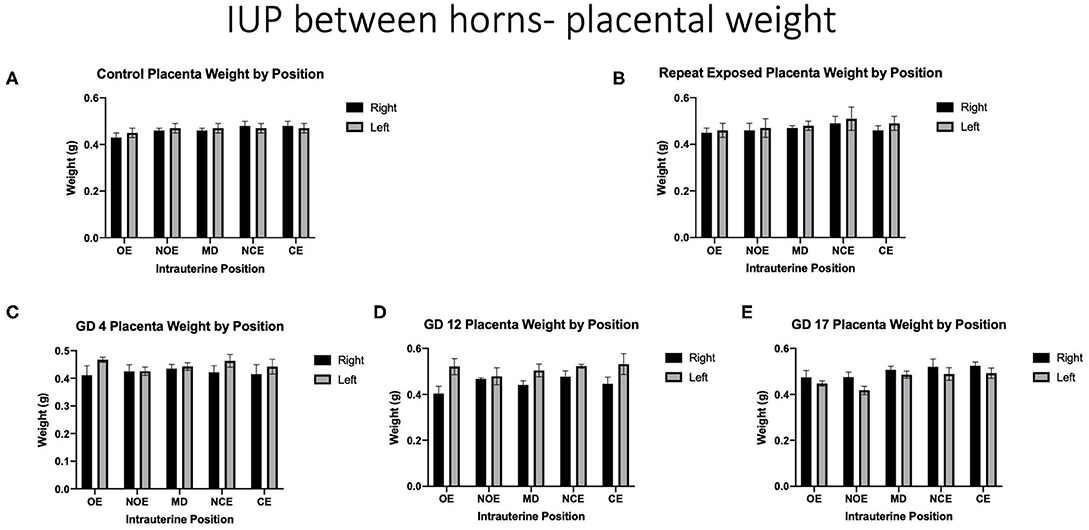
Figure 5. Raw placental weights (g). Data is analyzed by anatomical uterine position to identify differences between right and left uterine horns in (A) control and (B–E) exposure conditions.
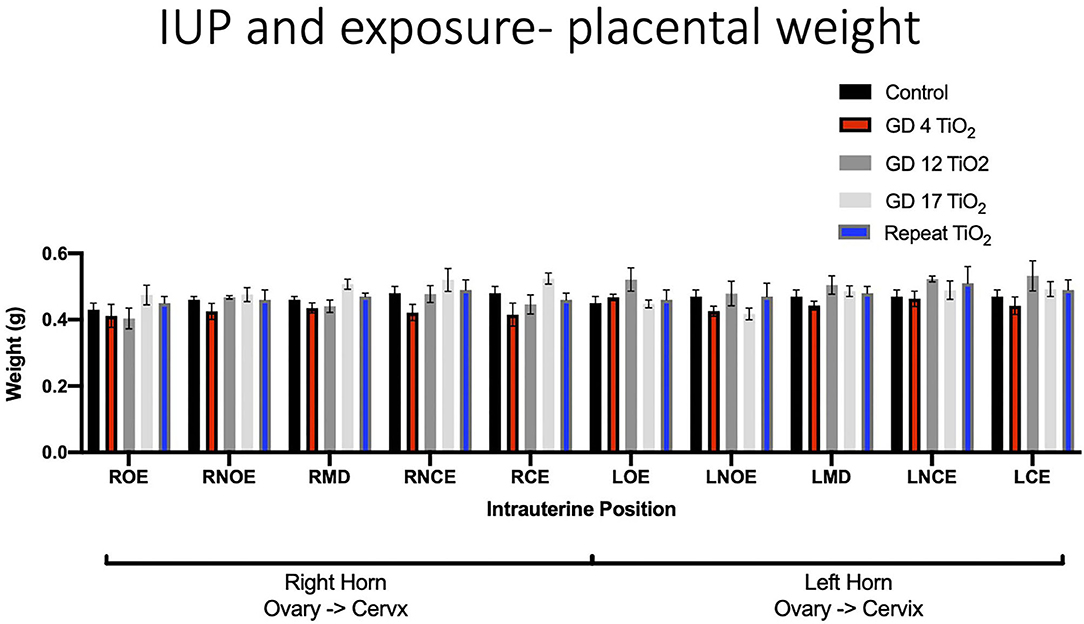
Figure 6. Raw placental weight (g) at each intrauterine position for control and exposure groups. This analysis pinpoints intrauterine positions which are susceptible to placental weight change after certain timing of exposure(s) compared to controls.
Intrauterine Position Effect on Placental Efficiency
When analyzing for placental efficiency, the ratio of fetal weight to placental weight, under control conditions, there was no difference in either horn or at any location (Figure 7A). Placental efficiency was not impacted within and between intrauterine locations in the right and left uterine horns with repeated (Figure 7B) or single (Figures 7C–E) exposures to nano-TiO2 aerosols. When comparing placental efficiency between each experimental group and the control group no significance was found with the exception of a single exposure on GD 17 resulting in a significant reduction in placental efficiency at the RMD location (5.09 ± 0.14 vs. 5.74 ± 0.16) (Figure 8).
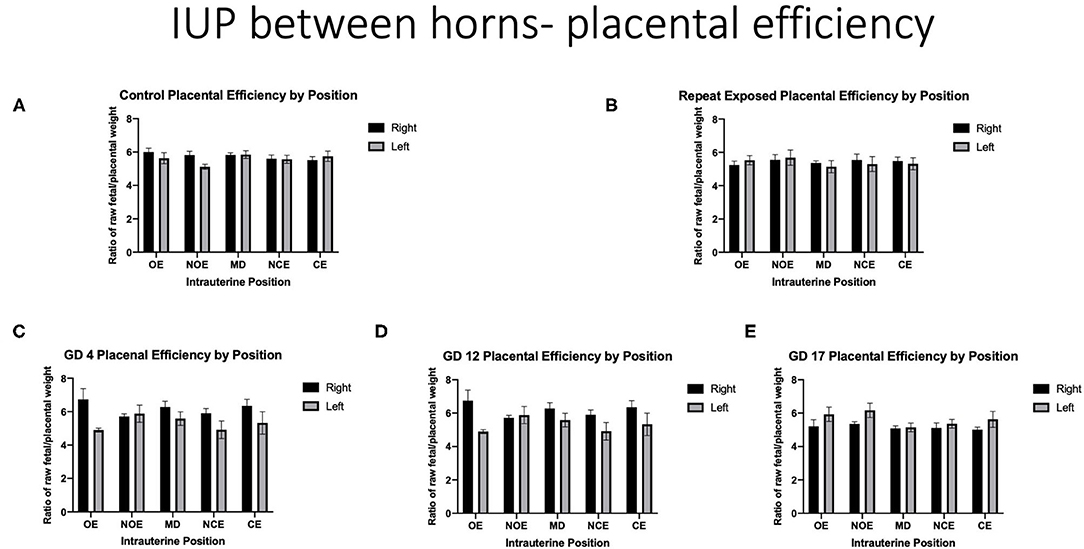
Figure 7. Placental efficiency calculated by fetal weight/placental weight. Data is analyzed by anatomical uterine position to identify differences between right and left uterine horns in control (A) and exposure groups (B–E).
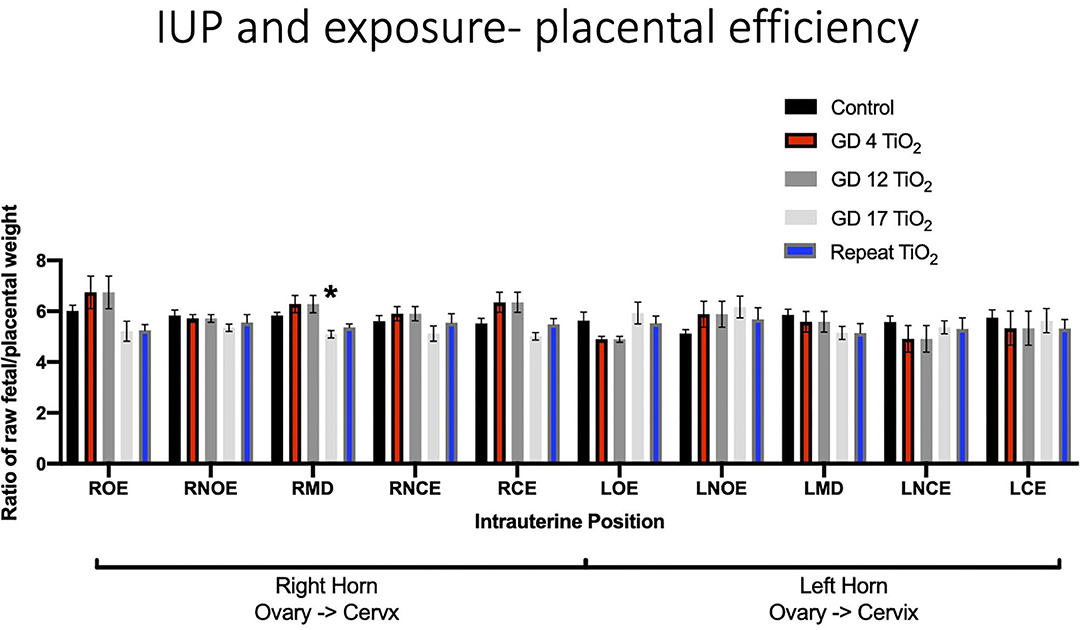
Figure 8. Placental efficiency, calculated by fetal weight/placental weight, for control and exposure groups. This analysis pinpoints intrauterine positions which are susceptible to reduced placental efficiency after certain timing of exposure(s) compared to controls. Significance is set to p < 0.05, indicated as *, and values are shown as mean ± SEM.
Discussion
In this study, data from controls and experimental exposures to nano-TiO2 were evaluated in a manner that challenges the traditional dogma of litter data analysis. Herein, we were able to account for intrauterine position, number of feti per horn and maternal weight. When evaluating this dataset using traditional methods, we did not detect significant differences or more than 10% reduction in fetal growth between treatment groups. However, upon further evaluation using our revised approach we observed that under control conditions, feti implanted in the left uterine horn tended to be smaller in body weight at term compared with feti implanted in the right uterine horn. These analyses also demonstrated significant FGR in exposed animals compared with controls, separated by uterine horn and intrauterine position, outcomes that were lost with traditional approaches. This imbalance of fetal growth between the uterine horns was exacerbated after either repeated maternal exposure to nano-TiO2 aerosols during gestation or a single exposure early (GD 4) or in late (GD 17) pregnancy. The most severe FGR in terms of magnitude of impact and locations affected were apparent from a single maternal exposure on GD 4 in the middle position of the right uterine horn. The middle position of the right uterine horn was also impacted with respect to other endpoints including increased placental weight, reduced fetal weight, and decreased placental efficiency after a single exposure late in gestation, on GD 17. Interestingly, there was no significant positional impact after repeated maternal nano-TiO2 exposure compared to control in this cohort. Overall, this study demonstrates both critical windows of maternal exposure early and late in gestation and the risks associated with anatomical positioning of implanted fetus in the right horn. Moreover, this information was not gleaned using a traditional approach to data analysis, where no impact on fetal weight was detected.
Observations from control conditions are in agreement with findings from other studies, in that despite anatomical similarities, the right horn produced larger feti suggesting a more favorable environment than the left (Wiebold and Becker, 1987; Lan et al., 2010). This observation has also held from human studies, where there is a tendency for right ovary ovulation and implantation on the right side of the reproductive tract (Kawakami et al., 1993; Fukuda et al., 2000). The anatomical or physiological reasons behind this phenomenon are not understood. Moreover, when comparing each designated position between horns, a significant difference was found in the middle position. Others have reported the middle of the uterine horn to be impacted when considering intrauterine position in pigs (Perry and Rowell, 1969; Jang et al., 2014) and mice (Raz et al., 2012). Interestingly, rat studies have a species-specific effect where fetal growth is largest in the middle of each uterine horn (Jensh et al., 1970; Padmanabhan and Singh, 1981). This phenomenon may be explained by middle placentas receiving dual-artery blood supply from ovarian and uterine arteries (Avni et al., 2012). Our findings from the control group of animals are in agreement with these observations. In our experimental groups we observed feti in middle positions were also most susceptible to reduced body weight after exposure on GD 4 and GD 17 (Figures 3C,E). These findings suggest differences in fetal growth between intrauterine implantation positions under control conditions and after maternal exposure that may be applicable to DART studies.
When comparing between right and left horns (Figure 2) and specific intrauterine position within (Figure 3) after a single or repeated nano-TiO2 inhalation, the patterns of fetal growth were dependent on the timing of exposure during pregnancy. After a single exposure on GD 4, smaller feti were found in the right horn compared with the left, oppositional to all other findings (Figure 2A). Additionally, the middle position in GD 4 (Figure 3C) as well as GD 17 (Figure 3E) exposure fetal weights were impacted between horns (Figure 3). GD 17 exposed dams had an additional effect on the body weight of feti on the left horn (Figure 2B), and specifically in the ovarian end position (Figure 3E). Animals exposed repeatedly or on GD 12 did not present significant outcomes. These findings are also similar to other studies evaluating the gestational timing of maternal particulate inhalation exposures on FGR, wherein the outcome is dependent upon the pregnancy window(s) of exposure (Blatt et al., 2015; DeFranco et al., 2015).
The resulting FGR outcomes with maternal inhalation of nano-TiO2 may be associated with vascular dysfunction of the maternal uterine vascular tree. Previous studies have demonstrated a blunted relaxation and increased vascular smooth muscle contractility of the uterine artery following a single maternal exposure during early, mid, and late pregnancy (Fournier et al., 2019). Uterine microvascular dysfunction of the radial arteries has been identified in correlation with reduced fetal growth after repeated maternal nano-TiO2 inhalation in previous studies (Stapleton et al., 2013). Further, impaired basal arteriolar dilation was identified in late-stage pregnancy in vivo 24-hr after a single exposure to nano-TiO2 aerosols (Stapleton et al., 2018), unfortunately fetal growth was not evaluated in this study. The variability in fetal weight by location may be attributed to differences in perfusion of the uterine tissue, as previously demonstrated by arterial spin labeling MRI imaging in mice uterine vasculature (Raz et al., 2012). Dual-perfusion to the placental-fetal unit is delivered cranially from the ovarian artery and caudally from the uterine artery (Figure 1). In theory, there should be no change to blood quality or oxygenation between the ovarian, middle, or vaginal segments of the uterine horn (Burbank, 2012). However, as evidenced by human placental position studies and control animal data, balanced perfusion throughout the uterus may not be the case (Garris et al., 1983; Kalanithi et al., 2007; Zia, 2013; Jang et al., 2014; McLaurin and Mactutus, 2015). Raz et al. suggested feti at extreme ends of a uterine horn receive more nutrient- and volume-rich blood conveying a growth advantage over the middle positions in a normal pregnancy (Raz et al., 2012). They also suggest that this dual perfusion model may provide a survival advantage to fetus implanted in the middle of the uterine horn in cases of arterial perturbation (Raz et al., 2012). Other studies have shown non-uniformity in hematocrit dispersal, pressure gradients, and blood flow at vascular bifurcations, which may also occur at branching along the uterine arteries (Kalsho and Kassab, 2004; Sriram et al., 2014). During disease states, instances of non-uniformity or non-equitable blood distribution are exacerbated (Butcher et al., 2014; Frisbee et al., 2016); this may also be the case after environmental exposure as evidenced in Figure 2A. More research of blood distribution through the uterus and perturbations to uterine perfusion after maternal xenobiotic exposures is needed. Other potential mechanisms of toxicity may include: (1) impaired uterine angiogenesis (Bosquiazzo et al., 2010; Pereira et al., 2015), (2) increased uterine/spiral artery rarefaction (Baykal et al., 2004), (3) decreased quality of blood oxygenation or nutrition (Zamudio et al., 2010), (4) impaired placental function/nutrient transfer (Vrijer et al., 2004), (5) altered placental metabolism (Challis et al., 2000; Vaughan and Fowden, 2016), and (6) nano-TiO2 translocation culminating in physical blockade within the placenta (Ho et al., 2017; Zhang et al., 2018).
The placenta regulates the interface between maternal and fetal systems and is often implicated in cases of FGR as a reduction in placental efficiency. Interestingly, with the exception of GD 17 in the RMD position, neither placental weight nor efficiency were impacted. A change in placental weight may indicate placental stress and/or adaptation to the maternal milieu (Furukawa et al., 2011; Ouyang et al., 2013). Decreased placental efficiency ratios are associated with the development of chronic disease in later life, such as cardiovascular disease, diabetes, and other metabolic diseases (Martyn et al., 1996). Although we did not observe changes in placental weights and placental efficiency ratios that aligned with every pinpointed FGR location, placental oxidative stress balance and nutrient transfer may be perturbed at the molecular level (Huang et al., 2018; Zygula et al., 2020). Other environmental exposures, such as cadmium, are known to cause changes at the transcriptional level to the glucose transport proteins (GLUTs), impairing fetal glucose transfer (Xu et al., 2016). Alternatively, physical sedimentation of particles within the placenta may be causing physical blockage of fetal nutrient transfer (D'Errico et al., 2019; Fournier et al., 2020). Additional studies are required to understand potential placental molecular mechanisms that link exposure to nano-TiO2 to FGR outcomes.
The etiologies, pathogenesis, and potential therapies for FGR pregnancies have yet to be elucidated. Although many maternal factors (e.g., age, BMI, nutritional status, infections, cardiovascular, immune, pulmonary or thyroid disorders), administered medications [e.g., anticoagulants, steroids, beta blockers, anticonvulsants, and tranquilizers (Redmond, 1979)], and social behaviors [e.g., smoking, drinking, and substance abuse (Vogt Isaksen, 2004; Carter et al., 2013; Garrison et al., 2016; Sabra et al., 2017)] have been documented with human FGR studies, it is estimated that a large portion of FGR cases are of an unknown etiology. Domestic, occupational, or environmental exposures may play a large or confounding role in the initiation of FGR.
There are some limitations to our analyses in this study. While we focused on the effect of intrauterine growth positioning on fetal growth, the pathogenesis of FGR may also be influenced by fetal sex, a variable not assessed in these studies. Fetal sex plays a role in macronutrient uptake through the placenta, and thus sex-dependent differences in response to a growth-hindering intrauterine environment may occur (Mukhopadhyay et al., 2018). It has been hypothesized that male feti demonstrate a higher demand for nutrients for a rapid rate of growth; whereas, female feti are more frugal with nutrient uptake from the mother (Alur, 2019). Thus, because of this higher demand male feti may be more vulnerable to reduced growth if the maternal intrauterine condition is perturbed; in contrast, female feti may be more responsive to changes in the intrauterine environment, modifying growth to nutritional availability (Alur, 2019). Some studies that have stratified their fetal growth data by sex identified male-specific growth restriction after maternal conditions, such as gestational phthalate exposure (Zhao et al., 2014) and hypoxia (Thompson et al., 2020), have found evidence to support that FGR may be sex-dependent. Therefore, when conducting any assessment for fetal growth, stratifying data by fetal sex will be important to reveal sex dependent FGR. Future studies should include this variable in their analyses. Second, maternal body weight pre-pregnancy (Zhao et al., 2018) and gestational weight gain (Ludwig and Currie, 2010; Sato and Miyasaka, 2019) have been shown to correlate with fetal growth. Studies show larger females deliver larger feti, reasonably a result of more nutrition available for fetal growth. In this study maternal body weight on GD 20 was recorded and used in normalization calculations. Future studies should utilize maternal weight gain throughout pregnancy (e.g., maternal weight prior to implantation and at sacrifice or delivery) to have a better representation of exposure effects and nutritional concentrations available for fetal growth.
The overall goal of DART is to use information gained from animal studies to identify hazardous substances for a developing fetus. It is of utmost importance that reliable results are generated from these investigations; disregard for disparities associated with intrauterine positioning may lead to future studies riddled with variability. Therefore, clarity on the intrauterine positioning of feti such as those utilized and analyzed in these studies is paramount. Herein, we presented evidence of anatomical intrauterine positional effects after maternal exposure to nano-TiO2 aerosols, thereby challenging common DART dogma. Reconsidering the way in which these data are evaluated may reduce study variability and allow for more refined conclusions of hazard to fetal growth to be drawn.
Data Availability Statement
The raw data supporting the conclusions of this article will be made available by the authors, without undue reservation.
Ethics Statement
The animal study was reviewed and approved by Rutgers University Institutional Animal Care and Use Committee.
Author Contributions
JD'E was responsible for the chronic animal exposures, data organization and analysis, and writing of this manuscript. SF was responsible for single animal exposures and critical review of the manuscript. This manuscript was conceptually attributed to and written under the guidance of PS. All authors contributed to the article and approved the submitted version.
Funding
This work was supported by the National Institute of Environmental Health Sciences (R00-ES024783; R01-ES031285), Rutgers Center for Environmental Exposures and Disease (P30-ES005022), and Rutgers Joint Graduate Program in Toxicology (T32-ES007148).
Conflict of Interest
The authors declare that the research was conducted in the absence of any commercial or financial relationships that could be construed as a potential conflict of interest.
Acknowledgments
We would like to thank Ms. Chelsea Cary and Ms. Talia Seymore for their critical reading and review of the manuscript.
Supplementary Material
The Supplementary Material for this article can be found online at: https://www.frontiersin.org/articles/10.3389/ftox.2021.643804/full#supplementary-material
Supplementary Table 1. Table of effect sizes for traditional analysis approach (T-Test) and for our developed method (2-Way ANOVA).
Abbreviations
DART, Developmental and Reproductive Toxicology; FGR, Fetal Growth Restriction; GD, Gestational Day; GLUT, Glucose Transporter Protein; IUP, Intrauterine Position; Nano TiO2, Titanium Dioxide nanoparticles; R/L CE, Right/Left Cervical End; R/L MD, Right/Left Middle; R/L NCE, Right/Left Next-to Cervical End; R/L NOE, Right/Left Next-to Ovarian End; R/L OE, Right/Left Ovarian End; SD, Sprague Dawley; SMPS, Scanning Mobility Particle Sizer.
References
Alur, P. (2019). Sex differences in nutrition, growth, and metabolism in preterm infants. Front. Pediatrics. 7:22. doi: 10.3389/fped.2019.00022
Arce, R. M., Caron, K. M., Barros, S. P., and Offenbacher, S. (2012). Toll-like receptor 4 mediates intrauterine growth restriction after systemic Campylobacter rectus infection in mice. Mol. Oral Microbiol. 27, 373–381. doi: 10.1111/j.2041-1014.2012.00651.x
Avni, R., Raz, T., Biton, I. E., Kalchenko, V., Garbow, J. R., and Neeman, M. (2012). Unique in utero identification of fetuses in multifetal mouse pregnancies by placental bidirectional arterial spin labeling MRI. Magnet. Resonance Med. 68, 560–570. doi: 10.1002/mrm.23246
Bailey, B. A., Donnelly, M., Bol, K., Moore, L. G., and Julian, C. G. (2019). High altitude continues to reduce birth weights in Colorado. Matern. Child Health J. 23, 1573–1580. doi: 10.1007/s10995-019-02788-3
Balayla, J., Desilets, J., and Shrem, G. (2019). Placenta previa and the risk of intrauterine growth restriction (IUGR): a systematic review and meta-analysis. J. Perinat. Med. 47, 577–584. doi: 10.1515/jpm-2019-0116
Bamfo, J. E. A. K., and Odibo, A. O. (2011). Diagnosis and management of fetal growth restriction. J. Pregnancy. 2011, 640715–640715. doi: 10.1155/2011/640715
Barker, D. J. P. (2006). Adult consequences of fetal growth restriction. Clin. Obstet. Gynecol. 49, 270–283. doi: 10.1097/00003081-200606000-00009
Baykal, C., Sargon, M. F., Esinler, I., Onderoglu, S., and Onderoglu, L. (2004). Placental microcirculation of intrauterine growth retarded fetuses: scanning electron microscopy of placental vascular casts. Arch. Gynecol. Obstet. 270, 99–103. doi: 10.1007/s00404-003-0511-z
Bekkar, B., Pacheco, S., Basu, R., and DeNicola, N. (2020). Association of air pollution and heat exposure with preterm birth, low birth weight, and stillbirth in the US: a systematic review. JAMA Network Open. 3:e208243. doi: 10.1001/jamanetworkopen.2020.8243
Blatt, K., Moore, E., Chen, A., Van Hook, J., and DeFranco, E. A. (2015). Association of reported trimester-specific smoking cessation with fetal growth restriction. Obstet. Gynecol. 125, 1452–1459. doi: 10.1097/AOG.0000000000000679
Bosquiazzo, V. L., Varayoud, J., Muñoz-de-Toro, M., Luque, E. H., and Ramos, J. G. (2010). Effects of neonatal exposure to bisphenol A on steroid regulation of vascular endothelial growth factor expression and endothelial cell proliferation in the adult rat uterus. Biol. Reprod. 82, 86–95. doi: 10.1095/biolreprod.109.078543
Burbank, F. (2012). Hemodynamic Changes in the Uterus and its Blood Vessels in Pregnancy*. London: Sapiens Publishing Ltd.
Butcher, J. T., Stanley, S. C., Brooks, S. D., Chantler, P. D., Wu, F., and Frisbee, J. C. (2014). Impact of increased intramuscular perfusion heterogeneity on skeletal muscle microvascular hematocrit in the metabolic syndrome. Microcirculation 21, 677–687. doi: 10.1111/micc.12146
Carter, R. C., Jacobson, J. L., Sokol, R. J., Avison, M. J., and Jacobson, S. W. (2013). Fetal alcohol-related growth restriction from birth through young adulthood and moderating effects of maternal prepregnancy weight. Alcohol. Clin. Exp. Res. 37, 452–462. doi: 10.1111/j.1530-0277.2012.01940.x
Challis, D. E., Pfarrer, C. D., Ritchie, J. W., Koren, G., and Adamson, S. L. (2000). Glucose metabolism is elevated and vascular resistance and maternofetal transfer is normal in perfused placental cotyledons from severely growth-restricted fetuses. Pediatr. Res. 47, 309–315. doi: 10.1203/00006450-200003000-00005
Chen, B., Hong, W., Yang, P., Tang, Y., Zhao, Y., Aguilar, Z. P., et al. (2020). Nano zinc oxide induced fetal mice growth restriction, based on oxide stress and endoplasmic reticulum stress. Nanomaterials 10:259. doi: 10.3390/nano10020259
Curhan, G. C., Willett, W. C., Rimm, E. B., Spiegelman, D., Ascherio, A. L., and Stampfer, M. J. (1996). Birth weight and adult hypertension, diabetes mellitus, and obesity in US men. Circulation 94, 3246–3250. doi: 10.1161/01.CIR.94.12.3246
DeFranco, E., Hall, E., Hossain, M., Chen, A., Haynes, E. N., Jones, D., et al. (2015). Air pollution and stillbirth risk: exposure to airborne particulate matter during pregnancy is associated with fetal death. PLoS ONE 10:e0120594. doi: 10.1371/journal.pone.0120594
D'Errico, J. N., Doherty, C., Fournier, S. B., Renkel, N., Kallontzi, S., Goedken, M., et al. (2019). Identification and quantification of gold engineered nanomaterials and impaired fluid transfer across the rat placenta via ex vivo perfusion. Biomed. Pharmacother. 117:109148. doi: 10.1016/j.biopha.2019.109148
Fournier, S. B., D'Errico, J. N., Adler, D. S., Kollontzi, S., Goedken, M. J., Fabris, L., et al. (2020). Nanopolystyrene translocation and fetal deposition after acute lung exposure during late-stage pregnancy. Part. Fibre Toxicol. 17:55. doi: 10.1186/s12989-020-00385-9
Fournier, S. B., Kallontzi, S., Fabris, L., Love, C., and Stapleton, P. A. (2019). Effect of gestational age on maternofetal vascular function following single maternal engineered nanoparticle exposure. Cardiovasc Toxicol. 19, 321–333. doi: 10.1007/s12012-019-09505-0
Frisbee, J. C., Goodwill, A. G., Frisbee, S. J., Butcher, J. T., Wu, F., and Chantler, P. D. (2016). Microvascular perfusion heterogeneity contributes to peripheral vascular disease in metabolic syndrome. J. Physiol. 594, 2233–2243. doi: 10.1113/jphysiol.2014.285247
Fukuda, M., Fukuda, K., Andersen, C. Y., and Byskov, A. G. (2000). Right-sided ovulation favours pregnancy more than left-sided ovulation. Hum. Reprod. 15, 1921–1926. doi: 10.1093/humrep/15.9.1921
Furukawa, S., Hayashi, S., Usuda, K., Abe, M., Hagio, S., and Ogawa, I. (2011). Toxicological pathology in the rat placenta. J. Toxicol. Pathol. 24, 95–111. doi: 10.1293/tox.24.95
Garris, D. R., Blankenship, L. T., and Whitehead, D. S. (1983). Regional variations in uterine blood flow in the guinea pig: relationship to pregnancy site distribution. Gynecol. Obstet. Invest. 15, 33–40. doi: 10.1159/000299391
Garrison, L., Leeman, L., Savich, R. D., Gutierrez, H., Rayburn, W. F., and Bakhireva, L. N. (2016). Fetal growth outcomes in a cohort of polydrug- and opioid-dependent patients. J. Reprod. Med. 61, 311–319.
Gonser, M., Tillack, N., Pfeiffer, K. H., and Mielke, G. (1996). [Placental location and incidence of pre-eclampsia]. Ultraschall Med. 17, 236–238. doi: 10.1055/s-2007-1003188
Hales, C. N., Barker, D. J., Clark, P. M., Cox, L. J., Fall, C., Osmond, C., et al. (1991). Fetal and infant growth and impaired glucose tolerance at age 64. BMJ 303, 1019–1022. doi: 10.1136/bmj.303.6809.1019
Harper, L. M., Odibo, A. O., Macones, G. A., Crane, J. P., and Cahill, A. G. (2010). Effect of placenta previa on fetal growth. Am. J. Obstetr. Gynecol. 203, 330.e1-330.e3305. doi: 10.1016/j.ajog.2010.05.014
Hayward, C. E., Lean, S., Sibley, C. P., Jones, R. L., Wareing, M., Greenwood, S. L., et al. (2016). Placental adaptation: what can we learn from birthweight:placental weight ratio? Front. Physiol. 7, 28–28. doi: 10.3389/fphys.2016.00028
Ho, D., Leong, J. W., Crew, R. C., Norret, M., House, M. J., Mark, P. J., et al. (2017). Maternal-placental-fetal biodistribution of multimodal polymeric nanoparticles in a pregnant rat model in mid and late gestation. Sci. Rep. 7, 2866–2866. doi: 10.1038/s41598-017-03128-7
Huang, X., Anderle, P., Hostettler, L., Baumann, M. U., Surbek, D. V., Ontsouka, E. C., et al. (2018). Identification of placental nutrient transporters associated with intrauterine growth restriction and pre-eclampsia. BMC Genomics 19:173. doi: 10.1186/s12864-018-4518-z
Ishikawa, H., Seki, R., Yokonishi, S., Yamauchi, T., and Yokoyama, K. (2006). Relationship between fetal weight, placental growth and litter size in mice from mid- to late-gestation. Reprod. Toxicol. 21, 267–270. doi: 10.1016/j.reprotox.2005.08.002
Jang, Y. D., Ma, Y. L., and Lindemann, M. D. (2014). Intrauterine position affects fetal weight and crown-rump length throughout gestation. J. Anim. Sci. 92, 4400–4406. doi: 10.2527/jas.2014-7762
Jensh, R. P., Brent, R. L., and Barr, M. Jr. (1970). The litter effect as a variable in teratologic studies of the albino rat. Am. J. Anat. 128, 185–191. doi: 10.1002/aja.1001280205
Kalanithi, L. E. G., Illuzzi, J. L., Nossov, V. B., Frisbæk, Y., Abdel-Razeq, S., Copel, J. A., et al. (2007). Intrauterine growth restriction and placental location. J. Ultrasound Med. 26, 1481–1489. doi: 10.7863/jum.2007.26.11.1481
Kalsho, G., and Kassab, G. S. (2004). Bifurcation asymmetry of the porcine coronary vasculature and its implications on coronary flow heterogeneity. Am. J. Physiol. Heart Circul. Physiol. 287, H2493–H2500. doi: 10.1152/ajpheart.00371.2004
Kawakami, Y.-u., Andoh, K., Mizunuma, H., Yamada, K., Itoh, M., and Ibuki, Y. (1993). Assessment of the implantation site by transvaginal ultrasonography. Fertil. Steril. 59, 1003–1006. doi: 10.1016/S0015-0282(16)55918-4
Lan, K. C., Huang, F. J., Lin, Y. C., Kung, F. T., Lan, T. H., and Chang, S. Y. (2010). Significantly superior response in the right ovary compared with the left ovary after stimulation with follicle-stimulating hormone in a pituitary down-regulation regimen. Fertil. Steril. 93, 2269–2273. doi: 10.1016/j.fertnstert.2008.12.123
Lazic, S. E., and Essioux, L. (2013). Improving basic and translational science by accounting for litter-to-litter variation in animal models. BMC Neurosci. 14, 37–37. doi: 10.1186/1471-2202-14-37
Leon, D. A., Lithell, H. O., Vagero, D., Koupilova, I., Mohsen, R., Berglund, L., et al. (1998). Reduced fetal growth rate and increased risk of death from ischaemic heart disease: cohort study of 15 000 Swedish men and women born 1915-29. BMJ 317, 241–245. doi: 10.1136/bmj.317.7153.241
Ludwig, D. S., and Currie, J. (2010). The association between pregnancy weight gain and birthweight: a within-family comparison. Lancet 376, 984–990. doi: 10.1016/S0140-6736(10)60751-9
Magann, E. F., Doherty, D. A., Turner, K., Lanneau, G. S. Jr., Morrison, J. C., and Newnham, J. P. (2007). Second trimester placental location as a predictor of an adverse pregnancy outcome. J. Perinatol. 27, 9–14. doi: 10.1038/sj.jp.7211621
Manangama, G., Migault, L., Audignon-Durand, S., Gramond, C., Zaros, C., Bouvier, G., et al. (2019). Maternal occupational exposures to nanoscale particles and small for gestational age outcome in the French Longitudinal Study of Children. Environ. Int. 122, 322–329. doi: 10.1016/j.envint.2018.11.027
Martyn, C. N., Barker, D. J., Jespersen, S., Greenwald, S., Osmond, C., and Berry, C. (1995). Growth in utero, adult blood pressure, arterial compliance. Br. Heart J. 73, 116–121. doi: 10.1136/hrt.73.2.116
Martyn, C. N. D., Barker, J. P., and Osmond, C. (1996). Mothers' pelvic size, fetal growth, and death from stroke and coronary heart disease in men in the UK. Lancet 348, 1264–1268. doi: 10.1016/S0140-6736(96)04257-2
McLaurin, K. A., and Mactutus, C. F. (2015). Polytocus focus: Uterine position effect is dependent upon horn size. Int. J. Dev. Neurosci. 40, 85–91. doi: 10.1016/j.ijdevneu.2014.11.001
Morales-Rubio, R. A., Alvarado-Cruz, I., Manzano-León, N., Andrade-Oliva, M.-d.-l.-A., Uribe-Ramirez, M., Quintanilla-Vega, B., et al. (2019). In utero exposure to ultrafine particles promotes placental stress-induced programming of renin-angiotensin system-related elements in the offspring results in altered blood pressure in adult mice. Part. Fibre Toxicol. 16:7. doi: 10.1186/s12989-019-0289-1
Mukhopadhyay, A., Thomas, T., Bosch, R. J., Dwarkanath, P., Thomas, A., Duggan, C. P., et al. (2018). Fetal sex modifies the effect of maternal macronutrient intake on the incidence of small-for-gestational-age births: a prospective observational cohort study. Am. J. Clin. Nutr. 108, 814–820. doi: 10.1093/ajcn/nqy161
Ouyang, F., Parker, M., Cerda, S., Pearson, C., Fu, L., Gillman, M. W., et al. (2013). Placental weight mediates the effects of prenatal factors on fetal growth: the extent differs by preterm status. Obesity. 21, 609–620. doi: 10.1002/oby.20254
Padmanabhan, R., and Singh, S. (1981). Effect of intrauterine position on foetal weight in CF rats. Indian J Med Res. 73, 134–139.
Pereira, R. D., De Long, N. E., Wang, R. C., Yazdi, F. T., Holloway, A. C., and Raha, S. (2015). Angiogenesis in the placenta: the role of reactive oxygen species signaling. Biomed. Res. Int. 2015, 814543–814543. doi: 10.1155/2015/814543
Perry, J. S., and Rowell, J. G. (1969). Variations in foetal weight and vascular supply along the uterine horn of the pig. J. Reprod. Fertil. 19, 527–534. doi: 10.1530/jrf.0.0190527
Raz, T., Avni, R., Addadi, Y., Cohen, Y., Jaffa, A. J., Hemmings, B., et al. (2012). The hemodynamic basis for positional- and inter-fetal dependent effects in dual arterial supply of mouse pregnancies. PLoS ONE 7:e52273. doi: 10.1371/journal.pone.0052273
Redmond, G. P. (1979). Effect of drugs on intrauterine growth. Clin. Perinatol. 6, 5–19. doi: 10.1016/S0095-5108(18)31160-6
Rogers, J. F., and Dunlop, A. L. (2006). Air pollution and very low birth weight infants: a target population? Pediatrics 118, 156–164. doi: 10.1542/peds.2005-2432
Romero, A., Villamayor, F., Grau, M. T., Sacristán, A., and Ortiz, J. A. (1992). Relationship between fetal weight and litter size in rats: application to reproductive toxicology studies. Reprod. Toxicol. 6, 453–456. doi: 10.1016/0890-6238(92)90009-I
Sabra, S., Gratacós, E., and Gómez Roig, M. D. (2017). D., Smoking-induced changes in the maternal immune, endocrine, and metabolic pathways and their impact on fetal growth: a topical review. Fetal Diagn. Ther. 41, 241–250. doi: 10.1159/000457123
Sato, N., and Miyasaka, N. (2019). Stratified analysis of the correlation between gestational weight gain and birth weight for gestational age: a retrospective single-center cohort study in Japan. BMC Pregnancy Childbirth 19:402. doi: 10.1186/s12884-019-2563-5
Sharma, D., Shastri, S., and Sharma, P. (2016). Intrauterine Growth Restriction: Antenatal and Postnatal Aspects. Clin. Med. Insights Pediatrics. 10, 67–83. doi: 10.4137/CMPed.S40070
Shen, R., Zhao, L. L., Yu, Z., Zhang, C., Chen, Y. H., Wang, H., et al. (2017). Maternal di-(2-ethylhexyl) phthalate exposure during pregnancy causes fetal growth restriction in a stage-specific but gender-independent manner. Reprod. Toxicol. 67, 117–124. doi: 10.1016/j.reprotox.2016.12.003
Sriram, K., Intaglietta, M., and Tartakovsky, D. M. (2014). Hematocrit dispersion in asymmetrically bifurcating vascular networks. Am. J. Physiol. Heart Circul. Physiol. 307, H1576–H1586. doi: 10.1152/ajpheart.00283.2014
Stapleton, P. A., McBride, C. R., Yi, J., Abukabda, A. B., and Nurkiewicz, T. R. (2018). Estrous cycle-dependent modulation of in vivo microvascular dysfunction after nanomaterial inhalation. Reprod. Toxicol. 78, 20–28. doi: 10.1016/j.reprotox.2018.03.001
Stapleton, P. A., Minarchick, V. C., Yi, J., Engels, K., McBride, C. R., and Nurkiewicz, T. R. (2013). Maternal engineered nanomaterial exposure and fetal microvascular function: does the Barker hypothesis apply? Am. J. Obstet. Gynecol. 209, 227.e1–11. doi: 10.1016/j.ajog.2013.04.036
Tachibana, R., Umekawa, T., Yoshikawa, K., Owa, T., Magawa, S., Furuhashi, F., et al. (2019). Tadalafil treatment in mice for preeclampsia with fetal growth restriction has neuro-benefic effects in offspring through modulating prenatal hypoxic conditions. Sci. Rep. 9, 234–234. doi: 10.1038/s41598-018-36084-x
Thame, M., Osmond, C., and Trotman, H. (2015). Fetal growth and birth size is associated with maternal anthropometry and body composition. Matern. Child Nutr. 11, 574–582. doi: 10.1111/mcn.12027
Thompson, L. P., Turan, S., and Aberdeen, G. W. (2020). Sex differences and the effects of intrauterine hypoxia on growth and in vivo heart function of fetal guinea pigs. Am. J. Physiol. Regul. Integr. Comp. Physiol. 319, R243–R254. doi: 10.1152/ajpregu.00249.2019
Tunster, S. J., Van De Pette, M., and John, R. M. (2014). Isolating the role of elevated Phlda2 in asymmetric late fetal growth restriction in mice. Dis. Model. Mech. 7, 1185–1191. doi: 10.1242/dmm.017079
Vandamme, T. F. (2014). Use of rodents as models of human diseases. J. Pharm. Bioallied Sci. 6, 2–9. doi: 10.4103/0975-7406.124301
Vaughan, O. R., and Fowden, A. L. (2016). Placental metabolism: substrate requirements and the response to stress. Reprod. Domest. Anim. 51(Suppl. 2), 25–35. doi: 10.1111/rda.12797
Vogt Isaksen, C. (2004). Maternal smoking, intrauterine growth restriction, placental apoptosis. Pediatr. Dev. Pathol. 7, 433–42. doi: 10.1007/s10024-004-0105-1
Vrijer, B. D. T., Regnault, R. H., Wilkening, R. B., Meschia, G., and Battaglia, F. C. (2004). Placental uptake and transport of ACP, a neutral nonmetabolizable amino acid, in an ovine model of fetal growth restriction. Am. J. Physiol. Endocrinol. Metab. 287, E1114–E1124. doi: 10.1152/ajpendo.00259.2004
Wiebold, J. L., and Becker, W. C. (1987). Inequality in function of the right and left ovaries and uterine horns of the mouse. J. Reprod. Fertil. 79, 125–134. doi: 10.1530/jrf.0.0790125
Wollmann, H. A. (1998). Intrauterine growth restriction: definition and etiology. Horm. Res. Paediatr. 49, 1–6. doi: 10.1159/000053079
Xu, P., Wu, Z., Xi, Y., and Wang, L. (2016). Epigenetic regulation of placental glucose transporters mediates maternal cadmium-induced fetal growth restriction. Toxicology 372, 34–41. doi: 10.1016/j.tox.2016.10.011
Xu, X.-F. Y., Li, J. Y., Sheng, J. J., Liu, L. L., Tang, F. Z., and Chen, M (2014). Effect of low birth weight on childhood asthma: a meta-analysis. BMC Pediatr. 14, 275–275. doi: 10.1186/1471-2431-14-275
Yamashita, H., Shao, J., Qiao, L., Pagliassotti, M., and Friedman, J. E. (2003). Effect of spontaneous gestational diabetes on fetal and postnatal hepatic insulin resistance in Lepr(db/+) mice. Pediatr. Res. 53, 411–418. doi: 10.1203/01.PDR.0000049667.58071.7D
Zamudio, S., Torricos, T., Fik, E., Oyala, M., Echalar, L., Pullockaran, J., et al. (2010). Hypoglycemia and the origin of hypoxia-induced reduction in human fetal growth. PLoS ONE 5:e8551. doi: 10.1371/journal.pone.0008551
Zhang, B., Tan, L., Yu, Y., Wang, B., Chen, Z., Han, J., et al. (2018). Placenta-specific drug delivery by trophoblast-targeted nanoparticles in mice. Theranostics 8, 2765–2781. doi: 10.7150/thno.22904
Zhao, R., Xu, L., Wu, M. L., Huang, S. H., and Cao, X. J. (2018). Maternal pre-pregnancy body mass index, gestational weight gain influence birth weight. Women Birth. 31, e20–e25. doi: 10.1016/j.wombi.2017.06.003
Zhao, Y., Chen, L., Li, L.-., Xie, C.-., Li, D., Shi, H.-., et al. (2014). Gender-specific relationship between prenatal exposure to phthalates and intrauterine growth restriction. Pediatr. Res. 76, 401–408. doi: 10.1038/pr.2014.103
Zia, S. (2013). Placental location and pregnancy outcome. J. Turkish German Gynecol. Assoc. 14, 190–193. doi: 10.5152/jtgga.2013.92609
Zygula, A., Kosinski, P., Wroczynski, P., Makarewicz-Wujec, M., Pietrzak, B., Wielgos, M., et al. (2020). Oxidative stress markers differ in two placental dysfunction pathologies: pregnancy-induced hypertension and intrauterine growth restriction. Oxid. Med. Cell. Longev. 2020:1323891. doi: 10.1155/2020/1323891
Keywords: fetal growth restriction, intrauterine position, titanium dioxide nanoparticle, spraque-dawley rat, rodent model, maternal exposures
Citation: D'Errico JN, Fournier SB and Stapleton PA (2021) Considering Intrauterine Location in a Model of Fetal Growth Restriction After Maternal Titanium Dioxide Nanoparticle Inhalation. Front. Toxicol. 3:643804. doi: 10.3389/ftox.2021.643804
Received: 18 December 2020; Accepted: 22 February 2021;
Published: 23 March 2021.
Edited by:
Rosaria Meccariello, University of Naples Parthenope, ItalyReviewed by:
Colette Miller, United States Environmental Protection Agency (EPA), United StatesGeertje Lewin, Consultant, Münster, Germany
Copyright © 2021 D'Errico, Fournier and Stapleton. This is an open-access article distributed under the terms of the Creative Commons Attribution License (CC BY). The use, distribution or reproduction in other forums is permitted, provided the original author(s) and the copyright owner(s) are credited and that the original publication in this journal is cited, in accordance with accepted academic practice. No use, distribution or reproduction is permitted which does not comply with these terms.
*Correspondence: P. A. Stapleton, stapleton@eohsi.rutgers.edu