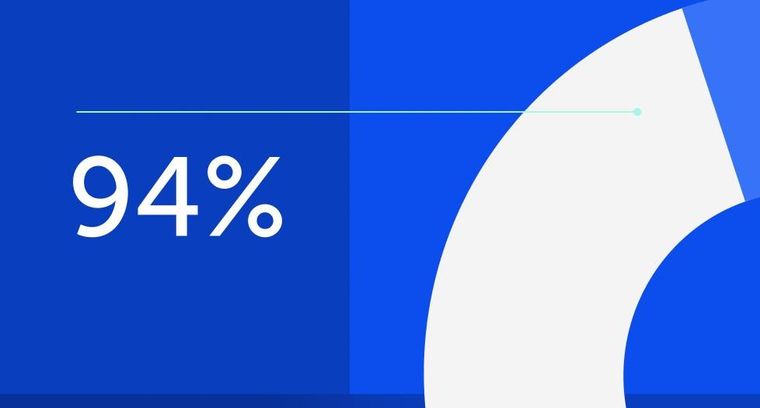
94% of researchers rate our articles as excellent or good
Learn more about the work of our research integrity team to safeguard the quality of each article we publish.
Find out more
SPECIALTY GRAND CHALLENGE article
Front. Therm. Eng., 24 October 2023
Sec. Heat Engines
Volume 3 - 2023 | https://doi.org/10.3389/fther.2023.1301616
In 2022 I was honored by Frontiers in Thermal Engineering Journal with the possibility of being Speciality Chief Editor of the Heat Engines Section, which of course I accepted immediately. Heat Engines are by far the most worldwide used engineering systems to convert heat energy, which is easy to generate but hard to use, into mechanical energy, that is not so easy to generate (at least in great numbers) but much easily converted into other forms of energy tailored for different applications.
The historical impact of Heat Engines on society cannot be discussed. Despite being invented shortly earlier, the relevance of Heat Engines started with the development of the Steam Engine and its further mass adoption, as it was undoubtedly one, if not the most important, driver enabling the Industrial Revolution that changed the means of production until our days (Sandfort, 1979). Looking to the present, after centuries of intense research and development, Heat Engines are in general extremely optimized, offering a wide portfolio of power ranging from Watts to gigaWatts, together with high flexibility in terms of technological solutions and associated costs, making them extremely suitable for the power generation and transportation sectors, among other domestic and industrial applications.
From a scientific and engineering perspective, Heat Engines are both fascinating and challenging systems altogether. Their design and analysis comprise the most prominent engineering fields, including (but not limited to) chemical, thermal, fluid-dynamics, mechanical, electrical and automation/control-related aspects. However, Heat Engines are under a lot of pressure since they have to fulfill not only the expectations of the customers, but also the increasing demands of society to reduce their environmental impact while increasing their sustainability, and these two aspects are opposite in most cases. Thus, aside from the constant need to increase their performance, the most critical challenges of Heat Engines focus on reducing their pollutant emissions, increasing their thermal efficiency (reducing fuel consumption) and their decarbonization by replacing partially or totally conventional fossil fuels.
In a general sense, a broad set of actions are being investigated to update Heat Engines in order to satisfy the previous expectations and demands from different angles. These actions include developing and integrating new engine hardware and components, advanced combustion concepts, optimized exhaust gas after treatment systems, or even new engine architectures aside from those with historical industrial success. A different but complementary trend consists of the electrification of the Heat Engine or its combination with an additional power source, usually electrical-based, to generate a hybrid system.
In addition, research activities dedicated to the further development of Heat Engines usually require close cooperation between the scientific community and the industry as they provide complementary skills and competencies, together with an intelligent combination of advanced experimental techniques and computational methods, assuring the right framework to generate beyond the state-of-the-art knowledge.
Now, it is time to review the key challenges faced by the Heat Engines research community that were briefly introduced in the previous section. It is important to remark that, of course, the list is not exhaustive and probably the reader can identify other non-discussed aspects where the Heat Engines should be or are already being developed, but in general, the final impact of those aspects will result in benefits and/or drawbacks in one or more of the grand challenges included here.
Heat Engines are widely used in various modes of transportation and industrial applications. However, they are also a significant source of air pollution, contributing to global warming and causing severe health problems (Fayyazbakhsh et al., 2022; Joshi, 2022; Rupcic et al., 2023). The most relevant specific pollutants emitted by Heat Engines include:
• Carbon Monoxide (CO): Carbon monoxide is a colorless and odorless gas produced by the incomplete combustion of carbon-based fuels. It is toxic to humans and can lead to carbon monoxide poisoning, which can be fatal in moderate-to-high concentrations.
• Nitrogen Oxides (NOx): Nitrogen oxides are a group of gases, including nitrogen oxide (NO) and nitrogen dioxide (NO2), formed during the combustion of fuels due to the combination of oxygen availability and high temperatures. They contribute to the formation of smog, acid rain, and can have harmful effects on human health, such as respiratory system problems.
• Particulate Matter (PM): Particulate matter refers to tiny solid or liquid particles suspended in the air. In the context of Heat Engines, PM mainly consists of soot, ash, and other combustion byproducts in general also including soluble organic compounds like fuel and lubricating oil. These particles can vary in size and composition and have adverse health effects when inhaled, particularly fine particulate matter (PM2.5) which can penetrate deep into the lungs.
• Volatile Organic Compounds (VOCs): VOCs are a diverse group of chemicals that vaporize at room temperature. They are emitted during the combustion process as well as from fuel evaporation. VOCs contribute to the formation of ground-level ozone and smog, and some VOCs are classified as hazardous air pollutants due to their toxicity.
• Sulfur Dioxide (SO2): Sulfur dioxide is produced when fuels containing sulfur, such as coal and oil, are burned. It is a major contributor to acid rain and can cause respiratory problems in humans, particularly in individuals with asthma or other respiratory conditions. The SO2 generation in Heat Engines is being decreased by imposing stringent regulations on the sulfur content in the fuel.
• Greenhouse Gases (GHGs): Heat Engines are significant sources of greenhouse gas emissions, primarily carbon dioxide (CO2), which is a major contributor to climate change. Other greenhouse gases, such as methane (CH4) and nitrous oxide (N2O), may also be emitted in smaller amounts.
• Hazardous Air Pollutants (HAPs): Heat Engines can emit a range of hazardous air pollutants, which are chemicals known to have adverse effects on human health or the environment. Examples of HAPs include benzene, formaldehyde, acrolein, and polycyclic aromatic hydrocarbons (PAHs). These pollutants are often regulated due to their toxicity and potential long-term health impacts.
• Ozone-Depleting Substances (ODS): While not as prevalent as in the past, some heat engines, such as older models of air conditioning and refrigeration systems, may still emit ozone-depleting substances. These substances, such as chlorofluorocarbons (CFCs) and hydrochlorofluorocarbons (HCFCs), can lead to the destruction of the ozone layer and contribute to the greenhouse effect.
• Black Carbon: Also known as soot, black carbon is a type of fine particulate matter composed of carbonaceous material. It is a byproduct of incomplete combustion, particularly in diesel engines and biomass burning. Black carbon absorbs sunlight, leading to warming effects and the reduction of albedo when deposited on snow and ice surfaces, accelerating their melting.
• Heavy Metals: Some Heat Engines, especially those using fossil fuels like coal or oil, can release heavy metals into the environment. These metals, including mercury, lead, arsenic, and cadmium, are toxic and can bioaccumulate in ecosystems, posing risks to human and animal health. Efforts are made to reduce the emissions of heavy metals through emission control technologies and cleaner fuel sources.
• Ammonia (NH3): Heat Engines powered by ammonia-based fuels, such as certain types of internal combustion engines, may release ammonia emissions. While ammonia itself is not considered a direct greenhouse gas, it can contribute to the formation of particulate matter and nitrogen deposition, impacting air and water quality.
It is important to note that the extent and composition of pollutant emissions from Heat Engines can vary depending on several factors, including the engine design, fuel quality, combustion efficiency, maintenance, and emission control technologies employed (Johnson and Joshi, 2018; Leach et al., 2020). Regulations and standards play a crucial role in reducing pollutant emissions from heat engines, with emission limits and guidelines established by governmental bodies to promote cleaner and more efficient technologies. Continued research and development efforts focus on improving engine thermal efficiency, exploring alternative fuels, and implementing advanced emission control systems to mitigate the environmental impact of Heat Engines emissions. Additionally, developing alternative power systems, such as hybrid or electric vehicles, aims to reduce or eliminate the emissions associated with Heat Engines.
The increase in energy conversion efficiency in Heat Engines has always been of interest to the scientific community (Johnson and Joshi, 2018; Leach et al., 2020; Mu et al., 2022). However, the need to improve thermal efficiency has recently become more relevant due to the urgent request to maximize the use of resources and control global warming by minimizing CO2 emissions.
In general, the main strategies to increase thermal efficiency in Heat Engines include:
• Adaptation of the thermodynamic cycle: Increasing the compression ratio is a well-known strategy widely applied to different Heat Engines, such as reciprocating internal combustion engines (RICE) or gas turbines (GT) (Benajes et al., 2019; Bontempo and Manna, 2019; Xing et al., 2023). Other less extended option consists of switching from the standard cycle to other more efficient alternatives, such as Atkinson/Miller cycles in the case of RICE or even Stirling/Ericsson cycles, which are external combustion engine versions of the conventional RICE and GT engines theoretically able to reach the Carnot efficiency limit. Modifying the working fluid is also an attractive alternative since its properties play a significant role in the thermal efficiency of Heat Engines, being the specific heat ratio the most relevant between them, and a good example is the Argon cycle under investigation for its applications in RICE.
• Development of the combustion process: The standard combustion process nowadays widely implemented in Heat Engines presents well-known limitations in terms of efficiency. In RICE the premixed combustion characteristic of Spark-Ignition (SI) engines limits this efficiency by operating in stoichiometric conditions (high heat losses due to the high temperatures and low specific heat ratio of the combustion products), while the diffusive combustion that develops in Compression-Ignition (CI) engines also faces efficiency problems (high local heat losses due to the flame-wall interactions and long duration). Thus, advanced combustion processes are being investigated with the aim of solving these problems by operating SI engines in lean conditions (Ziyaei et al., 2023) or CI engines in partially/fully premixed conditions (Dimitrakopoulos and Tunér, 2020), among others. In GT the implementation of lean combustion concepts is also under intense investigation to improve their efficiency by decreasing heat transfer losses (Haque et al., 2020).
• Technological development of systems: Since their inception, the technological evolution of Heat Engines has been really impressive. The systems integrated into these engines have evolved in all aspects, but probably the most relevant are the increase of the thermo-mechanic specifications of the engine materials and the optimization of the performance and efficiency of the engine subsystems. Thus, the increase in the maximum pressure and temperature attainable during the combustion process has a positive impact on engine efficiency, and also the development of more advanced subsystems that allow to improve the adjustment of some key engine settings, such as the injection or the boost pressures.
• Reduction of mechanical losses: This aspect is being largely investigated in the field of Heat Engines due to its positive impact on their thermal efficiency and fuel consumption. The technological solutions under evaluation include, but are not limited to, the use of dedicated low-friction materials, low-viscosity oils or intelligent management of the engine auxiliary systems. In general, these concepts are associated with some important drawbacks, such as a higher engine complexity, a decrease in its reliability or an increase in the total cost of ownership, so further research and development efforts are still required to maximize their benefits.
• Integration of Waste Heat Recovery (WHR) systems: The conversion of the energy rejected by Heat Engines into mechanical or electrical power is a very attractive alternative to increase the efficiency of the complete system. In this direction, The use of turbocompounding (Leng et al., 2023; Zhang et al., 2022), bottoming organic Rankine cycles (Xu et al., 2019; Varshil and Deshmukh, 2021) or thermoelectric systems (Rodriguez et al., 2019; Burnete et al., 2022) to recover the energy still available in the engine exhaust gas flow (high exergy) and/or in the coolant flow (low exergy) are alternatives gaining attention in RICE. Additionally, regeneration is a traditional option in GT, while the implementation of bottoming power cycles are recent alternatives being under investigation due to their potential (Li et al., 2021; Sun et al., 2021).
An additional extended option nowadays consists of the electrification of the engine by integrating components such as electrical superchargers (Baek et al., 2021) or turbochargers (Baek et al., 2019) in the case of RICE, which usually does not improve the intrinsic thermal efficiency of the engine but results in an increase in the global efficiency of the powerplant.
The key strategy enabling the decarbonization of Heat Engines consists of switching from conventional fossil fuels to alternative Fuels, which are usually classified as biofuels or e-fuels.
Biofuels are a category of fuels that are derived from organic materials, known as biomass, rather than from fossil fuels like coal, oil, and natural gas. They are considered a renewable energy source because they can be produced from various types of biological matter, such as plants, algae, and animal waste (Mat Aron et al., 2020; Jung et al., 2021; Osman et al., 2021). Biofuels have gained significant attention in recent years due to their potential to reduce greenhouse gas emissions and dependence on finite fossil fuel reserves.
Ethanol is the most widely used biofuel worldwide. It is an alcohol-based fuel primarily produced from corn, sugarcane, and wheat crops. The production process typically involves fermenting the sugar or starch present in these crops and then distilling it to produce ethanol. Ethanol is commonly blended with gasoline to create ethanol-gasoline blends like E10 (10% ethanol, 90% gasoline) or E85 (85% ethanol, 15% gasoline). Biodiesel is a renewable fuel derived from vegetable oils, animal fats, or recycled cooking oil. The production process involves a chemical reaction called transesterification, which converts these feedstocks into biodiesel. Biodiesel can be used in diesel engines in its pure form (B100) or blended with petroleum diesel at different ratios (e.g., B20, which contains 20% biodiesel and 80% diesel). Biogas is a gaseous biofuel produced through the anaerobic digestion of organic materials such as agricultural waste, sewage sludge, and food waste. The decomposition of these materials by bacteria in the absence of oxygen generates biogas, primarily consisting of methane and carbon dioxide. Biogas can be used directly for heating or electricity generation or upgraded to biomethane, a pipeline-quality gas similar to natural gas. Hydrocarbon biofuels are synthesized from biomass through processes such as pyrolysis, gasification, or Fischer-Tropsch synthesis. These biofuels are designed to be chemically similar to petroleum-based fuels and can be used as drop-in replacements in existing transportation infrastructure. Examples include renewable diesel and renewable jet fuel.
Biofuels offer several potential advantages. They can help reduce greenhouse gas emissions because the CO2 released during their combustion is offset by that absorbed by the plants during their growth. Additionally, biofuels can help diversify energy sources, promote rural development, and decrease dependence on foreign oil. However, biofuel production also faces challenges. It competes with food production for land and resources, impacting food prices and availability. Additionally, some biofuel feedstocks require significant amounts of water and fertilizer, which may have environmental implications. It is important to ensure sustainable and responsible practices in biofuel production to mitigate these potential drawbacks.
Researchers continue to explore advanced biofuel technologies, such as algae-based biofuels and cellulosic biofuels made from non-food crops or agricultural residues. These developments aim to improve the efficiency, sustainability, and economic viability of biofuels as a renewable energy option.
E-fuels, also known as electrofuels or synthetic fuels, are a type of renewable fuel that is produced by combining CO2 captured from the atmosphere with hydrogen generated through electrolysis, using electricity from renewable sources (Ueckerdt et al., 2021; Brynolf et al., 2022; Singh et al., 2022). E-fuels are distinct from biofuels because they are not derived from biomass but are instead created through a chemical process.
The first step involves capturing carbon dioxide from the atmosphere or from industrial processes, such as power plants. Various technologies, including chemical absorption and direct air capture, can be used to extract CO2. Renewable electricity is then used to power the process of electrolysis, where water is split into hydrogen and oxygen. This electrolysis process requires significant amounts of electricity, making it crucial for the power source to be renewable to ensure the sustainability of e-fuels. The captured CO2 is combined with H2 to produce synthetic hydrocarbon fuels, such as methane or liquid hydrocarbons like methanol or dimethyl ether. These fuels can serve as drop-in replacements for traditional fossil fuels and can be used in existing infrastructure without requiring major modifications.
E-fuels have several potential benefits. The production of e-fuels can be designed to be carbon-neutral or even carbon-negative. By capturing CO2 from the atmosphere and combining it with renewable hydrogen, the process can effectively recycle and reuse CO2 emissions that would otherwise contribute to global warming. E-fuels can serve as a form of energy storage for renewable electricity. When there is excess electricity generated from renewable sources, it can be used to produce e-fuels. These fuels can then be stored and utilized later when electricity demand is high or when renewable energy generation is low. E-fuels can be used in existing vehicles, aircraft, and infrastructure without significant modifications. This characteristic makes them an attractive option for decarbonizing transportation sectors that are currently reliant on fossil fuels.
However, e-fuels also face some challenges. The production of e-fuels is energy-intensive, and the overall process has lower energy efficiency than directly using renewable electricity. The efficiency losses occur during the steps of CO2 capture, electrolysis, and fuel synthesis. Currently, e-fuels are more expensive to produce than fossil fuels, primarily due to the high cost of renewable electricity and the additional energy required for the production process (Nemmour et al., 2023). As renewable energy costs continue to decline, the cost of e-fuels is expected to become more competitive. Scaling up the production of e-fuels to meet significant energy demands is a challenge. Large-scale production requires substantial amounts of CO2, renewable electricity, and hydrogen, which may strain available resources.
E-fuels are considered one of the key alternatives for decarbonizing sectors that are difficult to electrify, such as aviation, heavy-duty transportation, and certain industrial processes. While they offer potential benefits, further research, technological advancements, and cost reductions are needed to make e-fuels more economically viable and environmentally sustainable on a larger scale.
The key research and development grand challenges faced by modern Heat Engines, common to most if not all of them, have been briefly described with the aim of giving direct hints on these topics that are of primary interest to the Heat Engines Section of the Frontiers in Thermal Engineering Journal.
Thus, high-quality research papers reporting interesting scientific or technological results that push the knowledge beyond its current level, showing a positive impact on these topics are very welcome. Nonetheless, research activities targeting other potentially critical aspects related to Heat Engines are also under the scope of this Section, even if they don’t directly target the previous topics.
As a last remark, the envision of the Editorial Team behind the Heat Engines Section is to provide the most suitable forum for the researchers and engineers community to share their advances in these fascinating energy conversion systems, with the common mission of making them part of the future.
RN: Writing–original draft, Writing–review and editing.
The author declared that no financial support was received for the research, authorship, and/or publication of this article.
The author RN declared that they were an editorial board member of Frontiers at the time of submission. This had no impact on the peer review process and the final decision.
All claims expressed in this article are solely those of the authors and do not necessarily represent those of their affiliated organizations, or those of the publisher, the editors and the reviewers. Any product that may be evaluated in this article, or claim that may be made by its manufacturer, is not guaranteed or endorsed by the publisher.
Baek, S., Lee, H., and Lee, K. (2021). Fuel efficiency and exhaust characteristics of turbocharged diesel engine equipped with an electric supercharger. Energy 214, 119049. doi:10.1016/j.energy.2020.119049
Baek, S., Woo, S., Kim, Y., and Lee, K. (2019). Prediction of turbocharged diesel engine performance equipped with an electric supercharger using 1d simulation. Energy 185, 213–228. doi:10.1016/j.energy.2019.07.060
Benajes, J., Novella, R., Gómez-Soriano, J., Martínez-Hernándiz, P. J., Libert, C., and Dabiri, M. (2019). Evaluation of the passive pre-chamber ignition concept for future high compression ratio turbocharged spark-ignition engines. Appl. Energy 248, 576–588. doi:10.1016/j.apenergy.2019.04.131
Bontempo, R., and Manna, M. (2019). Work and efficiency optimization of advanced gas turbine cycles. Energy Convers. Manag. 195, 1255–1279. doi:10.1016/j.enconman.2019.03.087
Brynolf, S., Hansson, J., Anderson, J. E., Skov, I. R., Wallington, T. J., Grahn, M., et al. (2022). Review of electrofuel feasibility—prospects for road, ocean, and air transport. Prog. Energy 4, 042007. doi:10.1088/2516-1083/ac8097
Burnete, N. V., Mariasiu, F., Depcik, C., Barabas, I., and Moldovanu, D. (2022). Review of thermoelectric generation for internal combustion engine waste heat recovery. Prog. Energy Combust. Sci. 91, 101009. doi:10.1016/j.pecs.2022.101009
Dimitrakopoulos, N., and Tunér, M. (2020). Evaluation of engine efficiency, emissions and load range of a ppc concept engine, with higher octane and alkylate gasoline. Fuel 275, 117955. doi:10.1016/j.fuel.2020.117955
Fayyazbakhsh, A., Bell, M. L., Zhu, X., Mei, X., Koutný, M., Hajinajaf, N., et al. (2022). Engine emissions with air pollutants and greenhouse gases and their control technologies. J. Clean. Prod. 376, 134260. doi:10.1016/j.jclepro.2022.134260
Haque, M. A., Nemitallah, M. A., Abdelhafez, A., Mansir, I. B., and Habib, M. A. (2020). Review of fuel/oxidizer-flexible combustion in gas turbines. Energy & Fuels 34, 10459–10485. doi:10.1021/acs.energyfuels.0c02097
Johnson, T., and Joshi, A. (2018). Review of vehicle engine efficiency and emissions. SAE Int. J. Engines 11, 1307–1330. doi:10.4271/2018-01-0329
Joshi, A. (2022). Review of vehicle engine efficiency and emissions. SAE Int. J. Adv. Curr. Pract. Mobil. 4, 1704–1733. doi:10.4271/2022-01-0540
Jung, S., Shetti, N. P., Reddy, K. R., Nadagouda, M. N., Park, Y.-K., Aminabhavi, T. M., et al. (2021). Synthesis of different biofuels from livestock waste materials and their potential as sustainable feedstocks–a review. Energy Convers. Manag. 236, 114038. doi:10.1016/j.enconman.2021.114038
Leach, F., Kalghatgi, G., Stone, R., and Miles, P. (2020). The scope for improving the efficiency and environmental impact of internal combustion engines. Transp. Eng. 1, 100005. doi:10.1016/j.treng.2020.100005
Leng, L., Ma, Z., Cheng, J., Shi, L., and Deng, K. (2023). Research on exhaust energy distribution regulation for fuel economy improvement of turbocompound diesel engine. Appl. Therm. Eng. 220, 119708. doi:10.1016/j.applthermaleng.2022.119708
Li, B., Wang, S.-s., Wang, K., and Song, L. (2021). Comparative investigation on the supercritical carbon dioxide power cycle for waste heat recovery of gas turbine. Energy Convers. Manag. 228, 113670. doi:10.1016/j.enconman.2020.113670
Mat Aron, N. S., Khoo, K. S., Chew, K. W., Show, P. L., Chen, W.-H., and Nguyen, T. H. P. (2020). Sustainability of the four generations of biofuels–a review. Int. J. Energy Res. 44, 9266–9282. doi:10.1002/er.5557
Mu, H., Wang, Y., Yang, C., Teng, H., Zhao, X., Lu, H., et al. (2022). Technical research on improving engine thermal efficiency. Adv. Mech. Eng. 14, 168781322211250. doi:10.1177/16878132221125032
Nemmour, A., Inayat, A., Janajreh, I., and Ghenai, C. (2023). Green hydrogen-based e-fuels (e-methane, e-methanol, e-ammonia) to support clean energy transition: a literature review. Int. J. Hydrogen Energy 48, 29011–29033. doi:10.1016/j.ijhydene.2023.03.240
Osman, A. I., Mehta, N., Elgarahy, A. M., Al-Hinai, A., Al-Muhtaseb, A. H., and Rooney, D. W. (2021). Conversion of biomass to biofuels and life cycle assessment: a review. Environ. Chem. Lett. 19, 4075–4118. doi:10.1007/s10311-021-01273-0
Rodriguez, R., Preindl, M., Cotton, J. S., and Emadi, A. (2019). Review and trends of thermoelectric generator heat recovery in automotive applications. IEEE Trans. Veh. Technol. 68, 5366–5378. doi:10.1109/tvt.2019.2908150
Rupcic, L., Pierrat, E., Saavedra-Rubio, K., Thonemann, N., Ogugua, C., and Laurent, A. (2023). Environmental impacts in the civil aviation sector: current state and guidance. Transp. Res. Part D Transp. Environ. 119, 103717. doi:10.1016/j.trd.2023.103717
Singh, H., Li, C., Cheng, P., Wang, X., and Liu, Q. (2022). A critical review of technologies, costs, and projects for production of carbon-neutral liquid e-fuels from hydrogen and captured co 2. Energy Adv. 1, 580–605. doi:10.1039/d2ya00173j
Sun, L., Wang, D., and Xie, Y. (2021). Energy, exergy and exergoeconomic analysis of two supercritical co2 cycles for waste heat recovery of gas turbine. Appl. Therm. Eng. 196, 117337. doi:10.1016/j.applthermaleng.2021.117337
Ueckerdt, F., Bauer, C., Dirnaichner, A., Everall, J., Sacchi, R., and Luderer, G. (2021). Potential and risks of hydrogen-based e-fuels in climate change mitigation. Nat. Clim. Change 11, 384–393. doi:10.1038/s41558-021-01032-7
Varshil, P., and Deshmukh, D. (2021). A comprehensive review of waste heat recovery from a diesel engine using organic rankine cycle. Energy Rep. 7, 3951–3970. doi:10.1016/j.egyr.2021.06.081
Xing, K., Guan, W., Guo, X., Wang, Y., Tu, Z., and Huang, H. (2023). Potential of high compression ratio combined with knock suppression strategy for improving thermal efficiency of spark ignition stoichiometric natural gas engine. Energy Convers. Manag. 276, 116544. doi:10.1016/j.enconman.2022.116544
Xu, B., Rathod, D., Yebi, A., Filipi, Z., Onori, S., and Hoffman, M. (2019). A comprehensive review of organic rankine cycle waste heat recovery systems in heavy-duty diesel engine applications. Renew. Sustain. Energy Rev. 107, 145–170. doi:10.1016/j.rser.2019.03.012
Zhang, Z., Liu, Q., Zhao, R., Chen, Y., and Qin, Q. (2022). Research on in-cylinder steam injection in a turbocompound diesel engine for fuel savings. Energy 238, 121799. doi:10.1016/j.energy.2021.121799
Keywords: thermal engineering, energy conversion, use of resources, environmental impact, sustainability
Citation: Novella R (2023) Grand challenges in heat engines. Front. Therm. Eng. 3:1301616. doi: 10.3389/fther.2023.1301616
Received: 25 September 2023; Accepted: 03 October 2023;
Published: 24 October 2023.
Edited and reviewed by:
Xianguo Li, University of Waterloo, CanadaCopyright © 2023 Novella. This is an open-access article distributed under the terms of the Creative Commons Attribution License (CC BY). The use, distribution or reproduction in other forums is permitted, provided the original author(s) and the copyright owner(s) are credited and that the original publication in this journal is cited, in accordance with accepted academic practice. No use, distribution or reproduction is permitted which does not comply with these terms.
*Correspondence: Ricardo Novella, cmlub3JvQG1vdC51cHYuZXM=
Disclaimer: All claims expressed in this article are solely those of the authors and do not necessarily represent those of their affiliated organizations, or those of the publisher, the editors and the reviewers. Any product that may be evaluated in this article or claim that may be made by its manufacturer is not guaranteed or endorsed by the publisher.
Research integrity at Frontiers
Learn more about the work of our research integrity team to safeguard the quality of each article we publish.