- 1 Chemical and Biochemical Engineering Department, Rutgers University, Piscataway, NJ, United States
- 2 Biomedical Engineering Department, Rutgers University, Piscataway, NJ, United States
The central circadian pacemaker in the suprachiasmatic nuclei (SCN) aligns the phase and period of autonomous molecular oscillators in peripheral cells to daily light/dark cycles via physiological, neuronal, hormonal, and metabolic signals. Among different entrainment factors, temperature entrainment has been proposed as an essential alternative for inducing and sustaining circadian rhythms in vitro. While the synchronization mechanisms for hormones such as glucocorticoids have been widely studied, little is known about the crucial role of body temperature as a systemic cue. In this work, we develop a semi-mechanistic mathematical model describing the entrainment of peripheral clocks to temperature rhythms. The model incorporates a temperature sensing-transduction cascade involving a heat shock transcription factor-1 (HSF1) and heat shock response (HSR) pathway to simulate the entrainment of clock genes. The model is used to investigate the mammalian temperature entrainment and synchronization of cells subject to temperature oscillations of different amplitudes and magnitudes and examine the effects of transitioning between temperature schedules. Our computational analyses of the system’s dynamic responses reveal that 1) individual cells gradually synchronize to the rhythmic temperature signal by resetting their intrinsic phases to achieve coherent dynamics while oscillations are abolished in the absence of temperature rhythmicity; 2) alterations in the amplitude and period of temperature rhythms impact the peripheral synchronization behavior; 3) personalized synchronization strategies allow for differential, adaptive responses to temperature rhythms. Our results demonstrate that temperature can be a potent entrainer of circadian rhythms. Therefore, in vitro systems subjected to temperature modulation can serve as a potential tool for studying the adjustment or disruption of circadian rhythms.
Introduction
The circadian timing system enables organisms to anticipate daily environmental variations and adapt their physiological activities and behaviors correspondingly. In mammals, virtually every cell contains a clock with a period of
These individual cells are driven by cues that synchronize their oscillations (Balsalobre et al., 2000; Damiola et al., 2000; Yamazaki et al., 2000; Stokkan et al., 2001). The cues are centrally coordinated by the master circadian clock located in the hypothalamus’s suprachiasmatic nucleus (SCN), which is primarily entrained to external light/dark cycles (Li and Androulakis, 2022). While the master clock is fairly robust, peripheral clocks are more prone to desynchronization, leading to diverse pathological conditions (Nudell et al., 2019). Several factors, including steroid hormones and (core body) temperature, have been considered potent internal entrainers in resetting peripheral clocks (Balsalobre et al., 2000; Kornmann et al., 2007; Buhr et al., 2010).
Experimental systems that can maintain or disrupt circadian rhythms are essential for studies aiming at understanding the pathophysiology of circadian disruption (Ndikung et al., 2020). While in vivo systems primarily use light and feeding as the key entrainers of circadian rhythms, few in vitro systems demonstrate the ability to sustain circadian oscillations. Circadian clocks in cultured cells can be synchronized, albeit transiently (Yamazaki et al., 2000), with a variety of signals, including serum, glucocorticoid hormones, synthetic glucocorticoids (e.g., dexamethasone), retinoic acid, Ca2+ ionophores, tumor promoters, and growth factors (Stratmann and Schibler, 2006) However, experimental studies have demonstrated that peripheral clocks in astrocytes, fibroblasts, liver, kidney, and lung, can be stably entrained to temperature pulses that mimic body temperature rhythms (Brown et al., 2002; Prolo et al., 2005; Abraham et al., 2010; Buhr et al., 2010; Saini et al., 2012). This offers the possibility of using temperature modulation as an in vitro stimulus to facilitate studies of circadian rhythms (Nudell et al., 2019). Unlike biochemical signals, external temperature rhythms can be modulcated at will and provide a more consistent way of generating circadian rhythms. In particular, this motivates the development of both experimental and theoretical systems that use temperature as a global entrainer to induce and sustain circadian expression patterns in cells.
The daily fluctuation of mammalian core body temperature lies within a narrow physiological range. It arises from a continuous interplay between the central circadian clock, the thermoregulatory center located in the preoptic hypothalamus, and heat-modulating tissues/organs (Morf and Schibler, 2013). Temperature variations are sensed by temperature-sensitive sensors throughout the body and brain (Dhaka et al., 2006; Benarroch, 2007; Romanovsky, 2007). Studies have also demonstrated the rhythmic accumulation of heat shock protein (Hsp) mRNA (Kornmann et al., 2007) resulting from a rhythmic activation of the transcription activator heat shock transcription factor-1 (HSF1) (Reinke et al., 2008) in response to temperature cycles. While total Hsf1 mRNA and HSF1 protein levels do not vary diurnally, the fraction of activated nuclear HSF1 during the active phase, when body temperature is elevated, is increased, pointing to a critical regulatory role (Saini et al., 2012).
Given that HSF1 acts as the regulatory output of a wide range of cellular signaling pathways (Akerfelt et al., 2010), it likely represents a point of convergence of several regulatory pathways, including the temperature sensing-transduction (Mohawk et al., 2012). Furthermore, the resetting of Per2 expression by circadian temperature pulses in tissue explants is blunted by the blockage of an HSF1 inhibitor (Buhr et al., 2010) and in HSF1-deficient fibroblasts (Tamaru et al., 2011). These observations suggest a signaling cascade involved in the entrainment of peripheral circadian clocks by (rhythmic) temperature signals.
In this work, we propose a mathematical model accounting for temperature as a zeitgeber, acting on a temperature signaling cascade entraining a population of cells. By conducting in silico experiments with the model, we reproduce several key experimental observations indicating that 1) the temperature rhythmicity induces the synchronization of peripheral cell clocks in an amplitude- and period-dependent manner, 2) desynchronization and synchronization of peripheral cells can be modulated by implementing appropriate temperature schedules, and 3) the ability of the system to adapt to temperature schedules depends on individual properties. Our model predicts a possible connection between a system’s abilities to maintain inherent rhythmicity and to attune to new circadian schedules. Taken together, these results demonstrate that temperature-entrained in vitro systems can replicate circadian dynamics observed in vivo, thus advancing the idea of developing more physiologically realistic in vitro systems for studying circadian rhythms.
Materials and methods
To capture the mammalian peripheral entrainment of the temperature rhythm, we propose a semi-mechanistic, ordinary differential equation (ODE)-based model consisting of two major components: a temperature signaling cascade and a prototypical peripheral clock gene network (Figure 1).
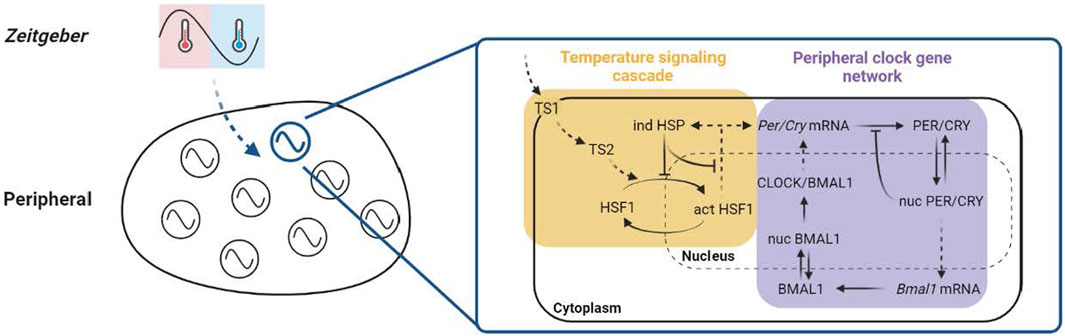
Figure 1. Schematic of the model. The ODE-based model is built on two sections, the temperature signaling cascade and the peripheral clock gene network using the indirect response model (IRM). In the temperature signaling cascade (yellow panel), a generalized thermosensor-transductor dynamics (TS1, TS2 ) is assumed to amplify the relative intensity of the temperature rhythm, as denoted by the ratio of the amplitude to the average of the component oscillation. This ampled temperature signal is then propagated to a downstream effector, the HSF1 -mediated HSR pathway. The temperature sensing process is hypothesized to be activated by integrating the differential sensing of temperature magnitude (represented by the average) and variation (representedby the amplitude). In addition, a classical HSR is assumed to be partly involved in the temperature entrainment by considering only the stimulatory effect of active HSF1 on the HSP production and inhibitory feedbacks of the HSF1 -induced HSP. In the peripheral clock gene network (purple panel), a gene regulatory network model consisting of positive and negative limbs is used to describe the intracellular circadian dynamics of clock genes and proteins. The figure is created with BioRender.com.
Temperature sensing and transduction
Temperature rhythms are systemic cues that efficiently entrain individual clocks in cultured cells and tissue explants (Morf and Schibler, 2013). A possible mechanism involves the post-transcriptional regulation of the cytoplasmic abundance of Clock mRNA by cold-inducible RNA-binding protein (CIRBP) (Morf et al., 2012) and the transcriptional induction of the nuclear Per2 mRNA by heat shock factor 1 (HSF1) (Saini et al., 2012). Experimental evidence shows that cold and heat exposure can activate HSF1 (Cullen and Sarge, 1997) and upregulate the nuclear Per2 transcript (Chappuis et al., 2013; Fischl et al., 2020), so we focus on the HSF1-associated mechanism in our model.
Without loss of generality, temperature rhythms are modeled as square wave functions. Such an approximation enables us to capture the essential oscillation characteristics such as the phase, period, and peak-to-trough amplitude (Eq. 1). Thermosensing and thermoregulation are closely interconnected (Xiao and Xu, 2021). Since temperature regulates nearly all the physiological processes in living organisms, thermosensors are ubiquitous and indispensable in sensing, transducing, and processing external and internal temperature fluctuations (Xiao and Xu, 2021). Thermosensitive transient receptor potential channels (thermo-TRPs) are molecular thermosensors shown to be present in the plasma membranes of both central and peripheral cells and participate in detecting temperature changes (Wang and Siemens, 2015; Kashio, 2021), thus playing a critical role in the entrainment of peripheral clocks (Bromberg et al., 2013; Poletini et al., 2015). Temperature is one of the gating forces to the thermo-TRPs activation (Matta and Ahern, 2007; Yao et al., 2011; Xiao and Xu, 2021), which means that they can be fully activated only by temperature changes that either fall within a specific temperature range or exceed a particular temperature threshold. This implies that both magnitude and changes in temperature are detected. Such temperature sensing process is expected to be essential for temperature entrainment and is assumed to be the first step of the system’s response to a temperature input. Using an indirect response modeling (IRM) approach (Foteinou et al., 2009; Foteinou et al., 2011), we hypothesize two intermediate signals, denoted as TS 1 and TS 2 , to represent the essential components involved in this cascade. Eqs 1, 2 describe their kinetics.
By using the average to represent, for simplicity, the magnitude of the input temperature oscillation, Eq. 2 encapsulates the temperature-induced thermo-TRPs activation upon detecting both temperature magnitude and change as well as their inactivation as a result of the homeostatic regulation. This transient activation of thermo-TRPs in the membrane in response to the temperature rhythm might enable the influx of cation ions and the change of membrane fluidity, which then initiate other downstream intracellular events (Vriens et al., 2014; Wang and Siemens, 2015; Guihur et al., 2022). Eq. 3 generalizes the surge (denoted by a Hill coefficient) and the vanishment of the effector of the thermosensor’s activation.
HSF1 is hypothesized to be involved in the mammalian temperature entrainment through the HSF1-induced heat shock response (HSR) pathway (Buhr et al., 2010). When there is no stress in the canonical HSR pathway, heat shock proteins (HSPs) sequester HSF1 monomers and suppress HSF1 transcriptional activity. Upon heat stress, the denaturation of specific thermolabile proteins leads to the dissociation of HSP chaperones from inactive HSF1/HSP complexes. Then, hyperphosphorylation, trimerization, and translocation of HSF1 occur sequentially (Rieger et al., 2005; Reinke et al., 2008; Scheff et al., 2015; Mazaira et al., 2018; Dudziuk et al., 2019; Masser et al., 2020; Guihur et al., 2022), inducing transcriptional activity of HSF1. The active HSF1 trans-activators stimulate the production of HSPs by binding the heat shock elements (HSEs) on the promotor. The resulting excessive HSPs in turn engage in the termination of HSF1 activation with the same sequestration strategy (Gomez-Pastor et al., 2018; Dudziuk et al., 2019). Independent of this molecular chaperone displacement theory, HSF1 can also be activated through different mechanisms, such as temperature-induced intrinsic structural response and other inter-molecular interactions (Hentze et al., 2016; Gomez-Pastor et al., 2018; Mazaira et al., 2018). However, exactly how HSF1 is activated by temperature variations within the physiological range and how HSR participates in the entrainment process remains unclear.
Therefore, by considering the contribution of thermosensors to thermoregulation and focusing on the HSF1-induced excessive HSPs that play an essential role in recovering HSF1 to its inactivated state, we assume that the HSF1 responds to a temperature input (TS 2 ) as is transduced by the thermosensing process while being inhibited by the excessive HSPs. The mechanisms are expressed via Eq. 4, which delineates the transduction of the temperature signal leading to the HSPs-mediated activation and the homeostatic inactivation of HSF1. Besides this canonical pathway, HSPs are also found to promote the noncanonical dissociation of active trimer HSF1 from the HSEs on target DNA (Kijima et al., 2018). This is described by HSPs inhibiting their HSF1-induced production in Eq. 5 along with a 1st-order protein degradation of HSPs.
Moreover, studies suggest that the HSF1 activity, such as its nuclear level, phosphorylation degree, and HSE-binding, exhibits circadian rhythm (Reinke et al., 2008; Hirota et al., 2016). In contrast, the Hsf1 mRNA and total HSF1 protein levels remain constant throughout the day (Buhr et al., 2010; Hirota et al., 2016). As such, the transcription dynamics of Hsf1 mRNA are not included.
The active HSF1 transduces the circadian oscillations of the temperature signal to the peripheral clock genes by inducing Per2 transcription (Saini et al., 2012). In addition, an active HSF1:CLOCK/BMAL1 interaction on the Per2 promotor was observed after a heat shock pulse and speculated to be involved in the temperature resetting process (Tamaru et al., 2011). Thus, This interaction is hypothesized to stabilize the HSF1:HSE binding and be competitive with the HSP noncanonical function. These mechanisms are included in the HSF1 entraining term in Eq. 6, which models the induction effect of the active HSF1 trans-activator on the Per/Cry expression as an indirect response.
Peripheral clock gene dynamics
The intrinsic dynamics of the peripheral clock gene network (Eqs 6–12) are modeled based on previous works (Becker-Weimann et al., 2004; Geier et al., 2005; Mavroudis et al., 2012; Mavroudis et al., 2014; Li and Androulakis, 2021). The network consists of transcriptional translational feedback loops, incorporating intertwined positive and negative feedback loops (Becker-Weimann et al., 2004; Geier et al., 2005). Through a positive feedback loop, the nuclear PER/CRY protein indirectly activates the transcription of Bmal1 mRNA. The translation to cytoplasmic BMAL1 protein and its translocation to the nucleus (nucBMAL1) lead to an increase in the production of CLOCK/BMAL1 heterodimer. On the other hand, the nuclear PER/CRY inhibits the stimulation of the transcription of Per/Cry by CLOCK/BMAL1.
Parameter estimation
Fifteen newly introduced parameters associated with the temperature signaling cascade and entrainment process were estimated, while the remaining parameters were set to the values determined earlier (Mavroudis et al., 2012). The parameter estimation aimed to satisfy the following criteria at the single-cell level: 1) the concentrations of TS
1
, TS
2, and activated HSF1 remain positive; 2) when subject to a 12-h warm/12-h cold (W12/C12
In silico population and quantification of synchronicity
A population of cells and individuals is simulated in the in silico experiments in this study. The cell population is used to understand the ensemble (average) behavior of peripheral cell oscillators, representative of tissue behavior (Yamazaki et al., 2002; Liu et al., 2007; Abraham et al., 2010), while members of the ensemble of individuals are utilized to approximate personalized responses (intra- and inter-individual variability). We generate 1,000 cells for the cell population by sampling the parameters associated with the temperature signaling cascade and peripheral clock gene network. To simulate inter-individual variability, we assume subjects to have individualized temperature sensing ability, which is attained by sampling the associated parameters (
To explore how the circadian rhythm of the temperature signal affects the state of clock genes at the peripheral tissue level, we evaluate the synchronicity of the cell population. In particular, the synchronization, defined as R
syn,j
, is assessed by quantifying cell oscillators’ deviations from mean levels (in terms of the clock gene component
where
In Eq. 13,
Modeling “temperature shift”
The circadian patterns of core body temperature are controlled by the mammalian master clock in the SCN, which is entrained by light/dark cycles (Edery, 2010; Morf and Schibler, 2013; Coiffard et al., 2021). Changes in the phase and period of the light/dark cycles may affect the circadian body temperature rhythm’s phase, period, and amplitude. This is manifested clearly in shift work, a type of circadian disruption, and has been recorded in many in vivo experiments (Knauth et al., 1978; Reinberg et al., 1980; Knauth et al., 1981; Reinberg et al., 1988; Logan and McClung, 2019). Since such mechanisms are absent in cell cultures, we hypothesize that imposed temperature rhythms can play the same zeitgeber role in cultured cells, offering the opportunity to mimic circadian disruption in vitro (Abraham et al., 2010; Stowie et al., 2019).
By applying persistent perturbations to the imposed temperature rhythm, permanent or alternating “temperature shift” are simulated in our study to examine the long-term effect of circadian disruption on peripheral cell synchronization and the inter-individual variability of responses to circadian disruption. As mentioned earlier, the temperature pattern is simulated as a series of Heaviside step functions (Eq. 1). In other words, during the warm period, the temperature is set at its maximum, whereas during the cold period, the temperature is set at its minimum. Combining different patterns results in various zeitgeber schedules. Thus, an alternating shift schedule (ASS) consists of a combination of successive inverted patterns (hereinafter termed “normal” and “reversed”, respectively):
The following temperature schedules are examined: 1) 5-day reversed (R) and 2-day normal (N) shift, 2) 3-day reversed and 4-day normal shift, 3) 6-day reversed and 1-day normal shift every 7 days (essentially simulating, in shorthand, 7–5:2/−3:4/−6:1 (R:N) ASS); 4) 20-day reversed, and 8-day normal shift, 5) 12-day reversed and 16-day normal shift every 28 days (in shorthand, a 28–5:2/−3:4 (R:N) ASS). Schedules (i)-(iii) and (iv)-(v) hold the same rotation periods, respectively, whereas (i) and (iv), (ii), and (v) have the same shift windows, respectively. A detailed depiction of
The modeling and computational analyses were implemented in MATLAB R2020b, and the codes are available in GitHub (https://github.com/IPAndroulakis/Temperature-induced-CR).
Results
Model calibration and validation
To simulate the peripheral entrainment subject to an imposed temperature rhythm, we constructed the model with two parts: (a) temperature signaling cascade and (b) peripheral clock gene network (Figure 1; see details in Materials and Methods). In the temperature signaling cascade, we consider a generalized thermosensor-transductor dynamic model and the downstream effector represented by an HSF1-mediated HSR pathway. The peripheral clock gene network is built upon a gene regulatory network model (Jong, 2002) as our earlier works (Mavroudis et al., 2012; Mavroudis et al., 2014; Mavroudis et al., 2015), while temperature rhythms are modeled as earlier described, with a 12 h warm (inactive) period between zeitgeber time (ZT) 0 h–12 h and the cold (active) period between ZT 12 h–24 h (nocturnal animals).
We hypothesize that temperature rhythms drive the phase and period of the peripheral cell-autonomous oscillators. Consequently, all the clock components’ rhythms are expected to be entrained and maintain stable temperature-phase relations (Abraham et al., 2018). Figure 2A reflects the temporal dynamics of representative components (temperature signal (TS
2
), HSF1 activity, clock genes’ mRNA, and their cytoplasmic proteins) when cells are entrained to a nominal W12/C12
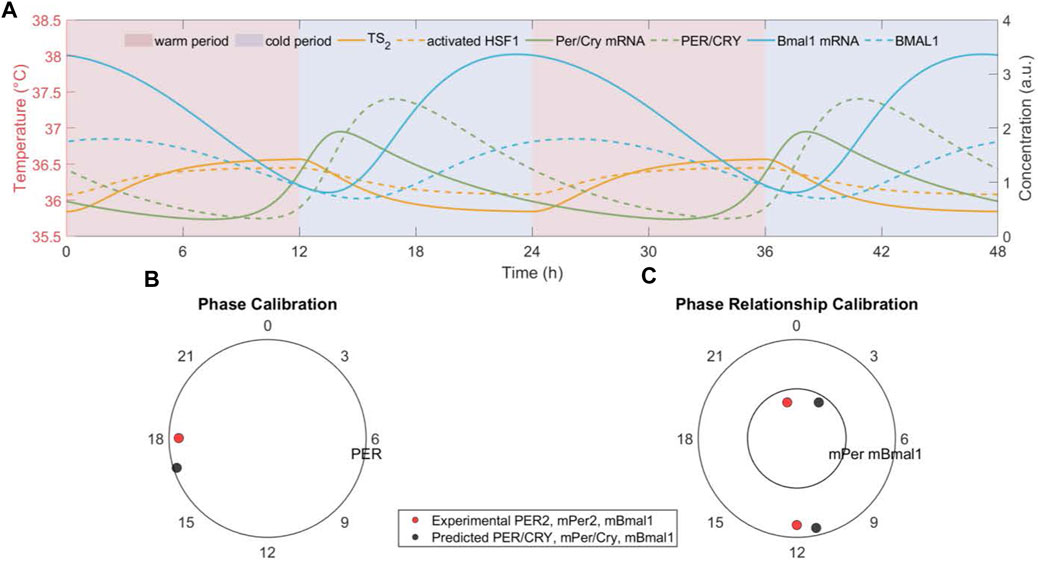
Figure 2. The peripheral cell dock gets phase and period entrained to the imposed temperature rhythm. (A) The temporal dynamics of transduced temperature signal (TS2 ), activate HSF1, clock genes (Per/Cry and Bmal1) mRNAs and cytoplasmic proteins, (B) the phase of clock gene (Per/Cry) cytoplasmatic protein, and (C) the phase relationship between clock genes (Per/Cry and Bmal1) mRNAs generated by the model with nominal parameters indicated in Table 1 and subject to a nominal W12/C12 37°C ± 1.5°C temperature rhythm. All components oscillate with a period of 24 h and maintain a stable phase relationship with the onset of the cold phase in the temperature rhythm. In addition, the predicted Per/Cry phase and nearly antiphase relationship between Per/Cry and Bmal1 mRNAs are experimentally consistent (Becker-Weimann et al., 2004; Li and Androulakis, 2021). Red/blue patterns denote warm/cold settings.
Our model further captures the entraining and resetting effect of temperature rhythm on the synchronization of a population of cells. Figure 3A depicts the ensemble dynamics of Per/Cry mRNA of a cell population subject to a switch between introduction and removal of temperature rhythm. In the absence of temperature rhythm
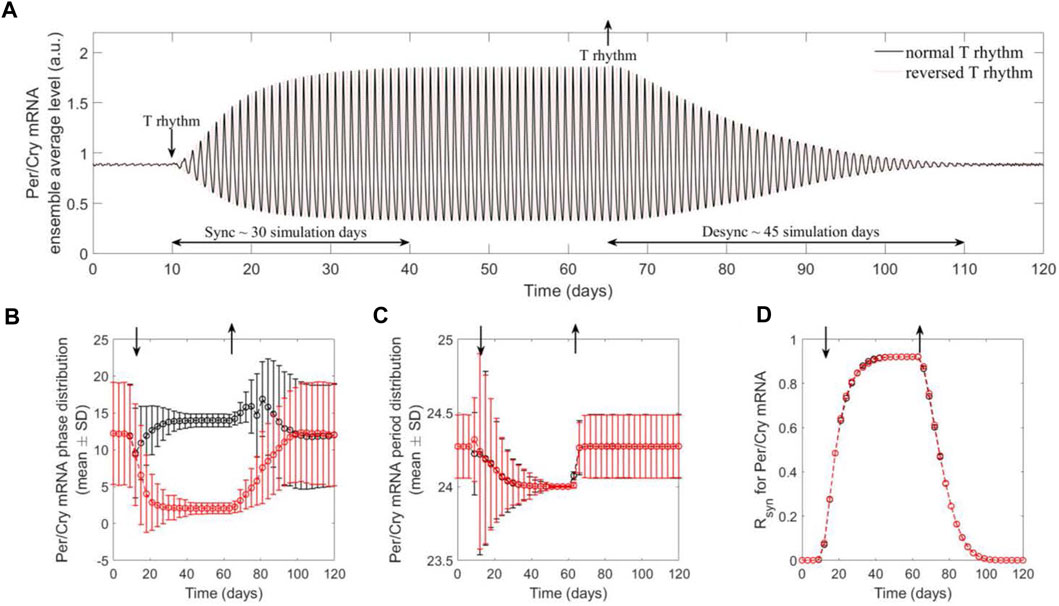
Figure 3. The population of peripheral cell clocks gets entrained and reset to the present rhythms of temperature signals. The population behavior was characterized by the ensemble average of cell clocks. Two temperature schedules were imposed, which are comprised of a consecutive absence (constant temperature at 37°C) and presence (W12/C12 37°C ± 1.5°C with 12 h phase difference) of the rhythms. The Per/Cry mRNA ensemble average circadian profile (A), single-cell phase (B) and period (C) distributions, and synchrony degree (Rsyn ) dynamics (D) were generated to analyze synchronization and desynchronization properties of component cell clocks. By preventing cell clocks from maintaining their original intrinsic/incoherent phases and periods, the presence of rhythm drives the synchronization of these individual oscillators, as indicated by shifted, narrowly distributed phases and periods, and high synchrony. This leads to the enhanced ensemble average amplitude and the adaptation of ensemble average phase and period to temperature rhythms. These results demonstrate efficient entraining and resetting effects of temperature rhythm on a population of cells. In addition, the difference in the dynamics of synchronization and desynchronization may be attributed to the inference of external signals in the intrinsic interactions between clock genes with interlocking feedback loops.
Interestingly, the qualitative differences in the dynamics of synchronization and desynchronization are apparent: the system requires a substantially shorter time to synchronize than desynchronize. This model prediction can be explained by Per2’s property of immediate early gene (Saini et al., 2012), suggesting that during the period following receiving an input signal, Per/Cry in each cell oscillator functions as an immediate early regulator. However, once the driving force is removed, Per/Cry functions solely as a core clock gene, leading the network to relax more gradually via its intrinsic mutual interactions between components and interlocking feedback loops.
The effects of temperature characteristics on peripheral clock synchronization
Since zeitgeber’s strength is critical for entrainment and synchronization, we evaluated the synchronization response to variations in the temperature amplitude. By varying the peak-to-trough values, i.e., amplitude (
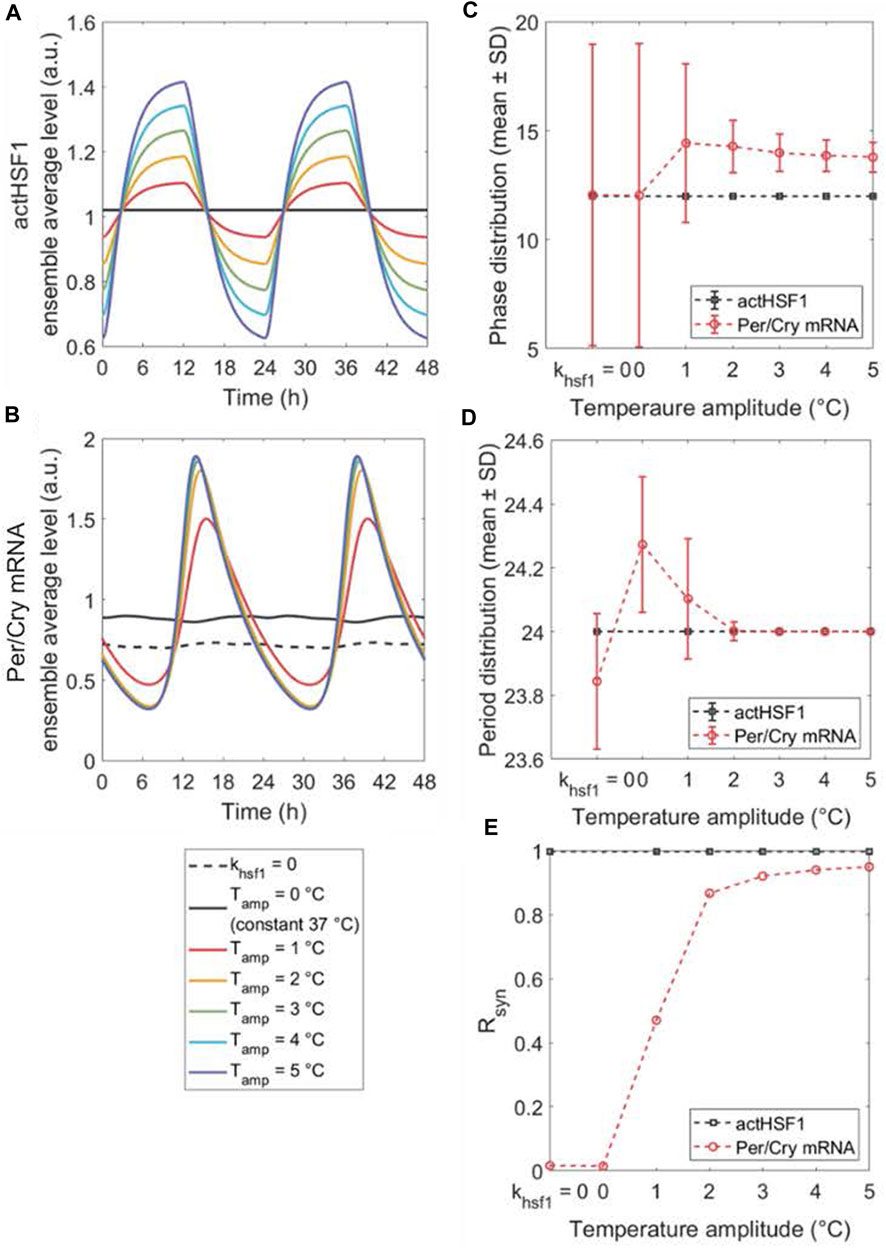
Figure 4. The peripheral synchronization changes in response to amplitude-varying temperature rhythms. The dynamics of ensemble average (A,B), single-cell phase (C) and period (D) distributions, and synchronization degree Rsyn (E) of actHSF1 and Per/Cry mRNA were obtained when peripheral cells are either insusceptible to (by setting khsf1 = 0 in the model) or susceptible to temperature rhythms with amplitudes varying from 0°C to 5°C. The loss of entraining agent (khsf1 = 0) and the elimination of the entrainer rhythm (constant temperature) result in a similar response of cell population, with the only difference in the magnitude of Per/Cry mRNA ensemble average and period. The increase in the amplitude of temperature rhythm enhances the Per/Cry mRNA ensemble average amplitude by more efficiently synchronizing the peripheral cells. This enhancement relies on both the amplification of rhythmic actHSF1 inputs and the inherent oscillating property of clocks.
Similarly, by altering the zeitgeber’s average (
To further elucidate how the period of temperature oscillations influences the synchronization of peripheral clocks, we analyzed the effect of period mismatch between the ensemble average of peripheral cell oscillators (intrinsic period
Internal responses to alternating temperature schedules
The previous results established relations between the synchronization behavior and dynamics of peripheral clocks and unperturbed temperature oscillations. In this subsection, we will investigate and analyze the response of a system exposed to alternating temperature schedules (i.e., perturbed temperature oscillations), which we describe as “alternating shift schedules (ASSs).”
Our first in silico experiments mimic the equivalence to “shift work,” where the system is repeatedly exposed to one zeitgeber schedule followed by a different schedule, each lasting for a certain period of time. Figure 5 shows the dynamics of ensemble phases and synchronization level of peripheral clocks subject to different ASSs (Figure A1). Two parameters define the ASS: a) the total period of the minimum non-repeating unit of schedule and b) the internal breakdown. For example, “7–5:2” implies that we consider a 7-day period during which the system is exposed to one oscillatory pattern for 5 days, and then the pattern is inverted for 2 days. Then, the 7-day cycle repeats in the schedule. Similarly, “7–4:3” implies a 7-day period with 4 days in one pattern and 3 days in the inverted. On the other hand, “28–5:2” means that we consider a 28-day period during which the system is exposed to one temperature pattern for 20 days and then an inverted pattern for 8 days (5:2 is the ratio of days in each pattern). Equivalently, “28–3:4” implies a 28-day non-repeating unit, with 12 days in one pattern and 16 days in the inverted. Specifically, in the simulations that follow, the system is first exposed to a “normal” temperature pattern (
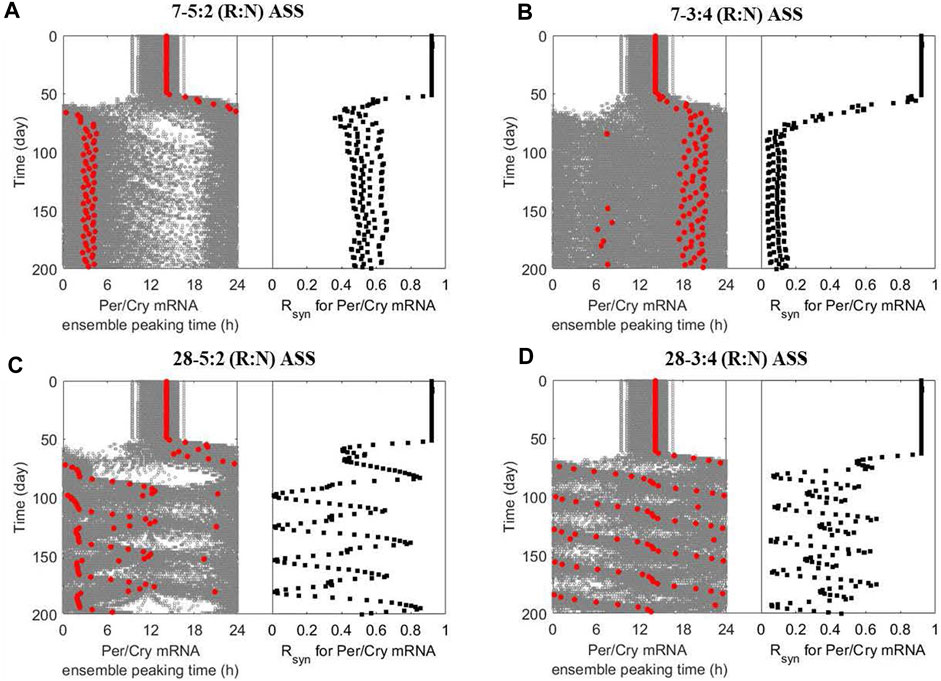
Figure 5. The multicellular system responds to alternating shift schedules (ASSs) in a schedule-dependent manner. The dynamics of ensemble phases (left panel; single-cell phases and their ensemble average denoted by grey and red circles, respectively) and synchronization level (right panel) of peripheral cell clocks were generated when the multicellular system was subject to different ASSs after 50 simulation days: (A) 5-day reversed and 2-day normal (7–5:2 (R:N)), (B) 3-day reversed and 4-day normal (7–3:4 (R:N)), (C) 20-day reversed and 8-day normal (28–5:2 (R:N)) and (D) 12-day reversed and 16-day normal (28–3:4 (R:N)). The system exhibits better circadian adaptation under the 7–5:2 (R:N) than the 7–3:4 (R:N) ASS, as indicated by eventually adopting oscillatory properties (phase and period) of the dominating temperature pattern and maintaining a higher internal synchronization. The slowly rotating schedules (28–5:2 and −3:4 (R:N) ASSs) generally damage the system’s circadian adaptive ability by inducing severe fluctuation in its dynamic phase entrainment and synchronization, implying an underlying destabilization compared to frequently rotating schedules (7–5:2 and - 3:4 (R:N) ASSs).
Figure 5A indicates that the ensemble average of peripheral clocks in a system following a 7–5:2 (R:N) schedule eventually adopts oscillatory characteristics resembling the dominating temperature pattern with a phase advance of −12 h while maintaining robust synchronization in the long run. The manifestation is quite different under the 7–4:3 (R:N) schedule (Figure 5B), where the system never manages to attain a clear phase and achieve synchronization. Thus, this schedule induces significant desynchrony. Circadian misalignment and desynchronization of cellular clocks are major contributing factors to chronic disease prevalence. Our observations are consistent with the experimental finding showing the three-shift workers are more vulnerable (probably by having a more dysregulated immune system (Castanon-Cervantes et al., 2010)) than the five-shift workers, resulting in a higher prevalence of common infections among members of the first category (Mohren et al., 2002).
We then considered slowly rotating schedules (i.e., longer time on each pattern before a change happens), generating more extended periods for the minimum non-repeating units. The peripheral ensemble average phase (Per/Cry mRNA peaking time) fluctuates significantly upon exposure to a 28–5:2 (R:N) schedule (Figure 5C) and effectively loses rhythmicity under the 28–3:4 (R:N) schedule (Figure 5D). Compared with the alternating schedules with a higher frequency, slowly rotating schedules allow the system to achieve a higher synchronization level during the adaptation process while leading to an internal destabilization, despite allowing the system to achieve a higher maximal R syn during the adaptation process. The destabilization can potentially bring about an allostatic accumulation of the consequent internal adverse effects of the shift schedules (Burgess, 2007).
Our early in silico experiments identified temperature amplitude and period as critical factors that can be manipulated to affect the circadian alignment of the system. To analyze the impact of amplitude further, we examined it in conjunction with ASSs. In Figure 6, the dynamics of ensemble average phases and R
syn
of peripheral clocks are reevaluated under the 7–5:2 and 7–3:4 schedules as a function of
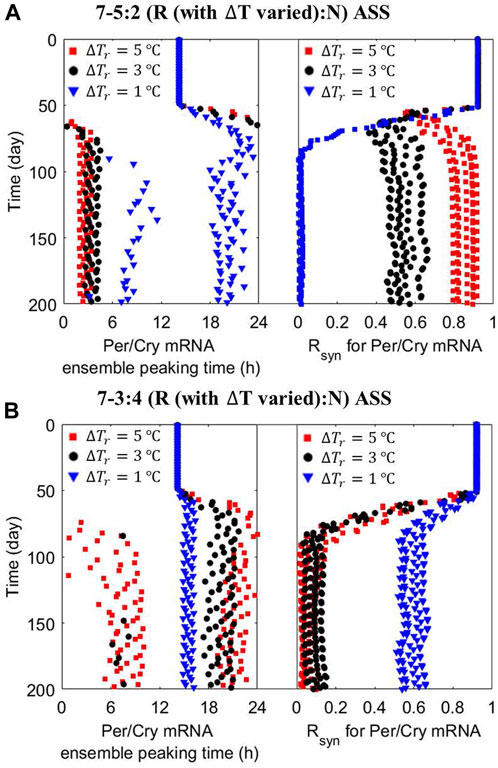
Figure 6. Zeitgeber’s amplitude has a schedule-dependent effect on peripheral cells’ nominal ensemble average response to ASSs. The dynamics of the ensemble average phase (left panel) and synchronization level (right panel) of peripheral cell clocks were reevaluated under the (A) 7–5:2 (R:N) and (B) 7–3:4 (R:N) ASSs with different zeitgeber’s amplitude (ΔTr, = 5 (red squares) and 1 (blue triangles) °C) during the reversed temperature pattern. A “good” state of circadian adaptation is characterized by more consistent and robust phase entrainment and higher internal synchrony. Under the 7–5:2 (R:N) ASS, an increase in the amplitude of dominant zeitgeber pattern further improves the system’s circadian adaptation, while a decrease destroys it. However, during the 7–3:4 (R:N) ASS, the multicellular system is strongly improved by a decrease in the amplitude of Tr pattern, reducing the inherent desynchronizing incline of the system caused by this schedule.
Individualized responses to alternating temperature schedules
Recent evidence (Rajaratnam et al., 2013; Rao and Androulakis, 2019; Scherholz et al., 2020) suggests that adaptation to zeitgeber characteristics strongly depends on an individual’s ability to sense and be influenced by them. Therefore, we hypothesize that individual peripheral synchronization is driven by, among others, differences in personalized temperature sensing as indicated by
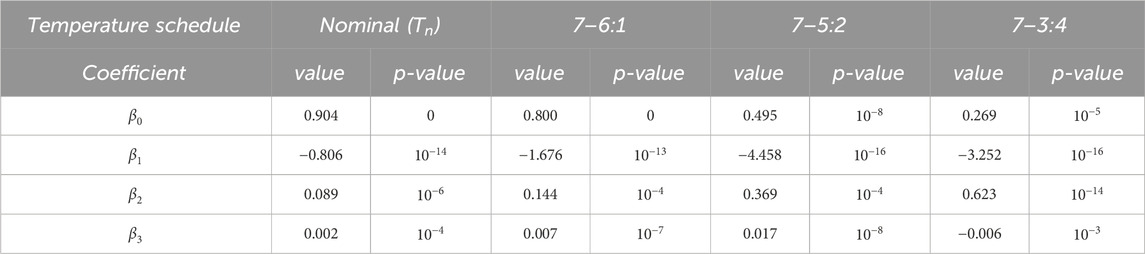
Table 2. Multi-linear regression analysis on the impact of individualized factors (vact0,ts1 , vact1,ts1 , kb,ts2 ) on peripheral synchronization degree under different ASSs.
We then evaluated the individual’s ability to remain entrained under an ASS as a function of the robustness of its circadian rhythms under the nominal temperature schedule by plotting the
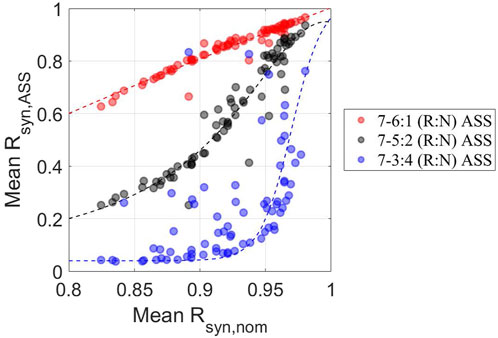
Figure 7. Individualized adaptive performance to ASSs depends on the schedule and the initial homeostatic state The synchrony degree under ASSs (mean Rsyn,ASS ) was plotted against the homeostatic synchronization state (mean Rsyn,nom ) for 7–6:1 (red circles), −5:2 (black circles), and −3:4 (blue circles) (R:N) schedules. Consistent with previous observations, individuals tend to exhibit better adaptation to circadian disruptive circumstances, as reflected by overall higher mean Rsyn, Ass when subject to 7–6:1, −5:2 than −3:4 (R:N) schedules. On the other hand, individuals with higher mean Rsyn, nom have higher mean Rsyn,ASS , indicating a critical and positive role of the pre-existing level of personalized internal synchronization in determining the personalized performance of circadian adaptation to ASSs.
Discussion
The synchronization of the internal physiological environment by zeitgebers is essential to maintaining homeostasis in living organisms. Although mammalian circadian entrainment/alignment has been predominantly studied both experimentally (in vivo, ex vivo, in vitro) and computationally (in silico) using light and feeding as zeitgebers, the role of temperature rhythms has received less attention. Temperature rhythms convey entrainment signals to peripheral cells, and their disturbance may lead to phase and period disruptions. Earlier works have presented mathematical models describing temperature entrainment of a single circadian clock in Neurospora crassa (Burt et al., 2021). In our study, we proposed a mathematical model representing the sensing and transduction of temperature and its role in entraining an ensemble of peripheral clocks in mammals through a simplified HSR pathway (Figure 1). Our model aims to provide insights into in vitro temperature entrainment.
Earlier experimental studies have reported that simulated body temperature rhythms can sustain or reset oscillations of circadian gene expression in cultured fibroblasts (Brown et al., 2002; Saini et al., 2012). In accordance with experimental findings, our model demonstrates that temperature oscillations not only induce and maintain the ensemble average oscillation of a virtual population of cells (Figure 2) but can also act as signals whose strengths determine the onset of circadian rhythms (Figure 3). Our results provide computational support to the potential of temperature cycles for inducing and sustaining periodic oscillation of cellular function in cultured cells or tissues (Abraham et al., 2010; Abraham et al., 2018; Stowie et al., 2019).
The entrainment of oscillatory systems generally depends on both the characteristics of the entraining signal and the intrinsic properties of the entrainee (Burt et al., 2021). To decipher the individual contributions, we first systematically examined the effects of amplitude, magnitude (average), and period of the entraining temperature signal on the synchronization properties of an ensemble of entrained clocks in peripheral cells. Since the synchronization of endogenous rhythms to zeitgeber cycles is fundamental for the adaptive function of circadian clocks (Abraham et al., 2010), we characterized both ensemble average phase distribution and synchronization (R
syn
). The absence of a periodic stimulus or its rhythm (
Circadian disruptions induced by alternating zeitgeber schedules such as irregular feeding, shift work, and jetlag are associated with an increased risk of chronic diseases, including depression, cancer, cardiovascular disease, metabolic syndrome, and diabetes (Wang et al., 2011). It is hypothesized that circadian misalignment leads to the desynchronization of peripheral clocks and eventually to the dysregulation of downstream processes mediated or regulated by clock genes (Wang et al., 2011; Rajaratnam et al., 2013; Kervezee et al., 2020). Although the alternating schedules have been primarily studied in the context of shift work, the implications of imposing temperature alternating shift schedules (ASSs) are lacking.
In order to better understand the effects of temperature ASSs (alternating frequency between and overall duration of alternative patterns), we computationally determined them with respect to the entrainment of a population of peripheral cells. We simulated the ASSs by repeatedly imposing alternative temperature patterns and denote them as “N-n1:n2”, meaning the overall period is “N” days, of which “n1:n2” is the relative time spent in each pattern (Figure A1). For example, “7–5:2” implies that the system experiences the overall pattern repeating every 7 days, during which it is first under one temperature pattern for 5 days and then under the inverted pattern for the next 2 days. The pattern “28–5:2” means that the overall pattern repeats every 28 days, and during each 28-day period, the system spends 20 days in one temperature pattern and 8 days in the inverted (20:8 = 5:2). The inversion of the temperature patterns is motivated by the classic definition of shift work, where the active phase becomes the rest and vice versa.
One of the key negative implications of alternating patterns of zeitgebers (for example, shift work) is that the circadian system never fully adjusts (Haus and Smolensky, 2006; Goldbeter and Leloup, 2021; Boivin et al., 2022). Qualitatively consistent with “shift work” experience, our results indicate that the system will tend to adopt the phase behavior of the pattern it spends most time in. Figure 5A suggests that, in the long run, the system will adopt the phase corresponding to the pattern presented the longest and be better entrained (i.e., internally synchronized). However, if the ASS does not have a dominating pattern and the alterations appear frequently, the system will lose its ability to maintain the underlying coherency and synchronization (Figure 5B). The situation can be exacerbated if the system spends more (total) time under each alteration in the schedule. In Figures 5C,D, even though the relative amount of time spent in each pattern is the same, because the absolute time is longer, the system is forced to oscillate between different entrainment states and thus never gets to readjust to a new coherent phase.
The nature of circadian adaptation under ASSs depends not only on the phase difference and time spent in each pattern but also on the strength of the entrainer. Interestingly, the strength of the entrainer emphasizes the effect of the ASS. Figure 6A depicts the situation where for the 7–5:2 schedule—a schedule expected to lead to coherent long-term response—the elicited long-term synchronized behavior is accentuated by increasing the amplitude of the dominant temperature pattern. On the other hand, the 7–4:3 ASS, which is expected to drive desynchronization, accentuates this effect as the zeitgeber’s strength in any pattern decreases (Figure 6B).
Finally, we aimed to assess individualized homeostatic entrainment and responses to ASSs in general. We concentrated on key control points of sensing and transduction of temperature. By sampling the corresponding parameters, we determined the relations between those critical parameters and entertainment responses under different zeitgeber schedules, as well as the abilities of individuals to be entrained during different ASSs given their baseline responses. The parameter associated with an individual’s sensing sensitivity to the magnitude (average) of temperature rhythm (
Admittedly, the mathematical model developed in this study has several limitations. Our simplified models do not account, among others, for 1) the tissue-specific temperature compensation that might be regulated by a phosphoswitch mechanism controlling the stability of PER/CRY (Narasimamurthy and Virshup, 2017), and 2) mediators like temperature-sensitive proteins other than HSF members that may contribute to the observed residual synchronization of HSF1-deficient cells to temperature rhythms (Tamaru et al., 2011). Nevertheless, it is essential to recognize that the purpose of this model is neither to recapitulate the regulation of core body temperature through thermoregulatory centers in the hypothalamus and the nearly unidirectional control of the circadian component by the SCN (Buhr et al., 2010), nor to use the examined alternating temperature schedules as equivalent to (human) shifwork. Instead, it aims to consider temperature as a likely entrainer of in vitro systems and determine whether such a zeitgeber could produce cellular responses that mimic circadian disruption’s effects. Our model provides a detailed theoretical framework to describe the circadian entrainment mechanisms of body temperature. The model qualitatively recapitulates critical observations and offers a blueprint for subsequent experiments to validate the induction and disruption of circadian rhythms in vitro or ex vivo by temperture rhythms. Our work contributes to the development of cell culture systems that can beter represent circadian biology (Mihelakis et al., 2022).
Conclusion
The early pioneering works of Goodwin, Goldbeter, Tyson, and others demonstrated how autonomous oscillations can result from well-defined networks of mutually regulated components. When subjected to zeitgebers, the entrainment of these “peripheral clocks” follows from the basic mathematical concepts describing forced oscillators. The question, however, concerns how zeitgeber information is transduced via appropriate signaling mechanisms to engage, entrain, or disrupt the innate circadian rhythms. We aimed to express how “generalized” structures materialize in a specific context to convert findings into actions. This work had two broader aims: 1) it is an attempt to shed some light on how T exerts its entraining actions. The fact that T can act as a zeitgeber, endowed with all the well-established properties of a zeitgeber, is expected. However, how this materializes is still being explored. Knowing this would be vital as we move to the next step, which is understanding how multiple zeitgebers convey their entraining signals; 2) one of the main issues is the lack of tunable in vitro systems that would enable to reproduce conditions of circadian disruption in a well-controlled environment. Motivated by early experimental observations, we further argue the importance of T to induce sustained and tunable oscillations. This would be critical to assess the role of circadian rhythms in physiology and pharmacology in a controlled environment. Cell culture systems are limited in generating, sustaining, and disrupting circadian rhythms at will to study their broader physiological implications in a controlled environment. The continuous efforts to assess the role of circadian rhythms and the need to reduce animal testing necessitates the development of appropriate surrogates to provide a more relevant environment. Recent work has demonstrated that temperature is a potent in vitro entrainer of peripheral clocks. In this direction, we developed a mathematical model that integrates temperature sensing, transduction, and an HSF1-mediated signaling component of the HSR pathway asnd peripheral clock genes to describe the temperature entrainment process in a multicellular system.
By investigating unperturbed and perturbed circadian conditions and considering individualized temperature signaling function, our model suggests an amplitude and/or period-dependent temperature effect on the entrainment of cellular clocks. We further showed how alternative temperature rhythms could mimic conditions of circadian disruption and their implications. Our results demonstrate that temperature rhythm can serve as an in vitro analog of circadian entrainment and an experimental tool to study the implications of circadian disruption. Since our model incorporates essential components and possible pathways of the temperature entrainment process, it provides the foundations for further experimental system and model development.
Data availability statement
The original contributions presented in the study are included in the article/Supplementary Material, further inquiries can be directed to the corresponding author.
Author contributions
LL: Conceptualization, Writing–review and editing, Formal Analysis, Investigation, Methodology, Validation, Visualization, Writing–original draft. YL: Methodology, Writing–review and editing. RS: Writing–review and editing. IA: Conceptualization, Formal Analysis, Funding acquisition, Investigation, Supervision, Writing–review and editing.
Funding
The author(s) declare financial support was received for the research, authorship, and/or publication of this article. LL, YL, and IPA acknowledge financial support from NIH GM 131800.
Conflict of interest
The authors declare that the research was conducted in the absence of any commercial or financial relationships that could be construed as a potential conflict of interest.
The author(s) declared that they were an editorial board member of Frontiers, at the time of submission. This had no impact on the peer review process and the final decision.
Publisher’s note
All claims expressed in this article are solely those of the authors and do not necessarily represent those of their affiliated organizations, or those of the publisher, the editors and the reviewers. Any product that may be evaluated in this article, or claim that may be made by its manufacturer, is not guaranteed or endorsed by the publisher.
Supplementary material
The Supplementary Material for this article can be found online at: https://www.frontiersin.org/articles/10.3389/fsysb.2024.1256398/full#supplementary-material
References
Abraham, U., Granada, A. E., Westermark, P. O., Heine, M., Kramer, A., and Herzel, H. (2010). Coupling governs entrainment range of circadian clocks. Mol. Syst. Biol. 6, 438. doi:10.1038/msb.2010.92
Abraham, U., Schlichting, J. K., Kramer, A., and Herzel, H. (2018). Quantitative analysis of circadian single cell oscillations in response to temperature. PLoS One 13, e0190004. doi:10.1371/journal.pone.0190004
Akerfelt, M., Morimoto, R. I., and Sistonen, L. (2010). Heat shock factors: integrators of cell stress, development and lifespan. Nat. Rev. Mol. Cell Biol. 11, 545–555. doi:10.1038/nrm2938
Balsalobre, A., Brown, S. A., Marcacci, L., Tronche, F., Kellendonk, C., Reichardt, H. M., et al. (2000). Resetting of circadian time in peripheral tissues by glucocorticoid signaling. Science 289, 2344–2347. doi:10.1126/science.289.5488.2344
Balsalobre, A., Damiola, F., and Schibler, U. (1998). A serum shock induces circadian gene expression in mammalian tissue culture cells. Cell 93, 929–937. doi:10.1016/s0092-8674(00)81199-x
Becker-Weimann, S., Wolf, J., Herzel, H., and Kramer, A. (2004). Modeling feedback loops of the Mammalian circadian oscillator. Biophys. J. 87, 3023–3034. doi:10.1529/biophysj.104.040824
Benarroch, E. E. (2007). Thermoregulation: recent concepts and remaining questions. Neurology 69, 1293–1297. doi:10.1212/01.wnl.0000275537.71623.8e
Boivin, D. B., Boudreau, P., and Kosmadopoulos, A. (2022). Disturbance of the circadian system in shift work and its health impact. J. Biol. Rhythms 37, 3–28. doi:10.1177/07487304211064218
Bromberg, Z., Goloubinoff, P., Saidi, Y., and Weiss, Y. G. (2013). The membrane-associated transient receptor potential vanilloid channel is the central heat shock receptor controlling the cellular heat shock response in epithelial cells. PLoS One 8, e57149. doi:10.1371/journal.pone.0057149
Brown, S. A., Zumbrunn, G., Fleury-Olela, F., Preitner, N., and Schibler, U. (2002). Rhythms of mammalian body temperature can sustain peripheral circadian clocks. Curr. Biol. 12, 1574–1583. doi:10.1016/s0960-9822(02)01145-4
Buhr, E. D., Yoo, S.-H., and Takahashi, J. S. (2010). Temperature as a universal resetting cue for mammalian circadian oscillators. Science 330, 379–385. doi:10.1126/science.1195262
Burgess, P. A. (2007). Optimal shift duration and sequence: recommended approach for short-term emergency response activations for public health and emergency management. Am. J. Public Health 97, S88–S92. doi:10.2105/AJPH.2005.078782
Burt, P., Grabe, S., Madeti, C., Upadhyay, A., Merrow, M., Roenneberg, T., et al. (2021). Principles underlying the complex dynamics of temperature entrainment by a circadian clock. iScience 24, 103370. doi:10.1016/j.isci.2021.103370
Castanon-Cervantes, O., Wu, M., Ehlen, J. C., Paul, K., Gamble, K. L., Johnson, R. L., et al. (2010). Dysregulation of inflammatory responses by chronic circadian disruption. J. Immunol. 185, 5796–5805. doi:10.4049/jimmunol.1001026
Chappuis, S., Ripperger, J. A., Schnell, A., Rando, G., Jud, C., Wahli, W., et al. (2013). Role of the circadian clock gene Per2 in adaptation to cold temperature. Mol. Metab. 2, 184–193. doi:10.1016/j.molmet.2013.05.002
Coiffard, B., Diallo, A. B., Mezouar, S., Leone, M., and Mege, J. L. (2021). A tangled threesome: circadian rhythm, body temperature variations, and the immune system. Biol. (Basel) 10, 65. doi:10.3390/biology10010065
Cullen, K. E., and Sarge, K. D. (1997). Characterization of hypothermia-induced cellular stress response in mouse tissues. J. Biol. Chem. 272, 1742–1746. doi:10.1074/jbc.272.3.1742
Damiola, F., Le Minh, N., Preitner, N., Kornmann, B., Fleury-Olela, F., and Schibler, U. (2000). Restricted feeding uncouples circadian oscillators in peripheral tissues from the central pacemaker in the suprachiasmatic nucleus. Genes Dev. 14, 2950–2961. doi:10.1101/gad.183500
Dhaka, A., Viswanath, V., and Patapoutian, A. (2006). Trp ion channels and temperature sensation. Annu. Rev. Neurosci. 29, 135–161. doi:10.1146/annurev.neuro.29.051605.112958
Dudziuk, G., Wronowska, W., Gambin, A., Szymanska, Z., and Rybinski, M. (2019). Biologically sound formal model of Hsp70 heat induction. J. Theor. Biol. 478, 74–101. doi:10.1016/j.jtbi.2019.05.022
Duffy, J. F., Rimmer, D. W., and Czeisler, C. A. (2001). Association of intrinsic circadian period with morningness-eveningness, usual wake time, and circadian phase. Behav. Neurosci. 115, 895–899. doi:10.1037//0735-7044.115.4.895
Edery, I. (2010). Circadian rhythms. Temperatures to communicate by. Science 330, 329–330. doi:10.1126/science.1197747
Fischl, H., McManus, D., Oldenkamp, R., Schermelleh, L., Mellor, J., Jagannath, A., et al. (2020). Cold-induced chromatin compaction and nuclear retention of clock mRNAs resets the circadian rhythm. EMBO J. 39, e105604. doi:10.15252/embj.2020105604
Foteinou, P. T., Calvano, S. E., Lowry, S. F., and Androulakis, I. P. (2009). Translational potential of systems-based models of inflammation. Clin. Transl. Sci. 2, 85–89. doi:10.1111/j.1752-8062.2008.00051.x
Foteinou, P. T., Calvano, S. E., Lowry, S. F., and Androulakis, I. P. (2011). A physiological model for autonomic heart rate regulation in human endotoxemia. Shock 35, 229–239. doi:10.1097/SHK.0b013e318200032b
Gachon, F., Nagoshi, E., Brown, S. A., Ripperger, J., and Schibler, U. (2004). The mammalian circadian timing system: from gene expression to physiology. Chromosoma 113, 103–112. doi:10.1007/s00412-004-0296-2
Geier, F., Becker-Weimann, S., Kramer, A., and Herzel, H. (2005). Entrainment in a model of the mammalian circadian oscillator. J. Biol. Rhythms 20, 83–93. doi:10.1177/0748730404269309
Goldbeter, A., and Leloup, J. C. (2021). From circadian clock mechanism to sleep disorders and jet lag: insights from a computational approach. Biochem. Pharmacol. 191, 114482. doi:10.1016/j.bcp.2021.114482
Gomez-Pastor, R., Burchfiel, E. T., and Thiele, D. J. (2018). Regulation of heat shock transcription factors and their roles in physiology and disease. Nat. Rev. Mol. Cell Biol. 19, 4–19. doi:10.1038/nrm.2017.73
Guihur, A., Rebeaud, M. E., Bourgine, B., and Goloubinoff, P. (2022). How do humans and plants feel the heat? Trends Plant Sci. 27, 630–632. doi:10.1016/j.tplants.2022.03.006
Haus, E., and Smolensky, M. (2006). Biological clocks and shift work: circadian dysregulation and potential long-term effects. Cancer Causes Control 17, 489–500. doi:10.1007/s10552-005-9015-4
Hentze, N., Le Breton, L., Wiesner, J., Kempf, G., and Mayer, M. P. (2016). Molecular mechanism of thermosensory function of human heat shock transcription factor Hsf1. Elife 5, e11576. doi:10.7554/eLife.11576
Hirota, T., and Fukada, Y. (2016). “Heat shock factors modulate circadian rhythms,” in Heat shock factor. Editor A. Nakai (Japan: Springer), 197–209.
Jong, H. d. (2002). Modeling and simulation of genetic regulatory systems: a literature review. J. Comput. Biol. 9, 67–103. doi:10.1089/10665270252833208
Kaneko, H., Kaitsuka, T., and Tomizawa, K. (2020). Response to stimulations inducing circadian rhythm in human induced pluripotent stem cells. Cells 9, 620. doi:10.3390/cells9030620
Kashio, M. (2021). Thermosensation involving thermo-TRPs. Mol. Cell Endocrinol. 520, 111089. doi:10.1016/j.mce.2020.111089
Kervezee, L., Kosmadopoulos, A., and Boivin, D. B. (2020). Metabolic and cardiovascular consequences of shift work: the role of circadian disruption and sleep disturbances. Eur. J. Neurosci. 51, 396–412. doi:10.1111/ejn.14216
Kijima, T., Prince, T. L., Tigue, M. L., Yim, K. H., Schwartz, H., Beebe, K., et al. (2018). HSP90 inhibitors disrupt a transient HSP90-HSF1 interaction and identify a noncanonical model of HSP90-mediated HSF1 regulation. Sci. Rep. 8, 6976. doi:10.1038/s41598-018-25404-w
Knauth, P., Emde, E., Rutenfranz, J., Kiesswetter, E., and Smith, P. (1981). Re-Entrainment of body temperature in field studies of shiftwork. Int. Archives Occup. Environ. Health 49, 137–149. doi:10.1007/bf00377667
Knauth, P., Rutenfranz, J., Herrmann, G., and Poeppl, S. J. (1978). Re-entrainment of body temperature in experimental shift-work studies. Ergonomics 21, 775–783. doi:10.1080/00140137808931781
Kornmann, B., Schaad, O., Bujard, H., Takahashi, J. S., and Schibler, U. (2007). System-driven and oscillator-dependent circadian transcription in mice with a conditionally active liver clock. PLoS Biol. 5, e34. doi:10.1371/journal.pbio.0050034
Lell, B., Brandts, C. H., Graninger, W., and Kremsner, P. G. (2000). The circadian rhythm of body temperature is preserved during malarial fever. Wien Klin. Wochenschr 112, 1014–1015.
Li, Y., and Androulakis, I. P. (2021). Light entrainment of the SCN circadian clock and implications for personalized alterations of corticosterone rhythms in shift work and jet lag. Sci. Rep. 11, 17929. doi:10.1038/s41598-021-97019-7
Li, Y., and Androulakis, I. P. (2022). Light-induced synchronization of the SCN coupled oscillators and implications for entraining the HPA axis. Front. Endocrinol. 13, 960351. doi:10.3389/fendo.2022.960351
Liu, A. C., Welsh, D. K., Ko, C. H., Tran, H. G., Zhang, E. E., Priest, A. A., et al. (2007). Intercellular coupling confers robustness against mutations in the SCN circadian clock network. Cell 129, 605–616. doi:10.1016/j.cell.2007.02.047
Logan, R. W., and McClung, C. A. (2019). Rhythms of life: circadian disruption and brain disorders across the lifespan. Nat. Rev. Neurosci. 20, 49–65. doi:10.1038/s41583-018-0088-y
Masser, A. E., Ciccarelli, M., and Andreasson, C. (2020). Hsf1 on a leash - controlling the heat shock response by chaperone titration. Exp. Cell Res. 396, 112246. doi:10.1016/j.yexcr.2020.112246
Matta, J. A., and Ahern, G. P. (2007). Voltage is a partial activator of rat thermosensitive TRP channels. J. Physiol. 585, 469–482. doi:10.1113/jphysiol.2007.144287
Mavroudis, P. D., Corbett, S. A., Calvano, S. E., and Androulakis, I. P. (2014). Mathematical modeling of light-mediated HPA axis activity and downstream implications on the entrainment of peripheral clock genes. Physiol. Genomics 46, 766–778. doi:10.1152/physiolgenomics.00026.2014
Mavroudis, P. D., Corbett, S. A., Calvano, S. E., and Androulakis, I. P. (2015). Circadian characteristics of permissive and suppressive effects of cortisol and their role in homeostasis and the acute inflammatory response. Math. Biosci. 260, 54–64. doi:10.1016/j.mbs.2014.10.006
Mavroudis, P. D., Scheff, J. D., Calvano, S. E., Lowry, S. F., and Androulakis, I. P. (2012). Entrainment of peripheral clock genes by cortisol. Physiol. Genomics 44, 607–621. doi:10.1152/physiolgenomics.00001.2012
Mazaira, G. I., Daneri-Becerra, C., Zgajnar, N. R., Lotufo, C. M., and Galigniana, M. D. (2018). Gene expression regulation by heat-shock proteins: the cardinal roles of HSF1 and Hsp90. Biochem. Soc. Trans. 46, 51–65. doi:10.1042/BST20170335
McGowan, N. M., and Coogan, A. N. (2013). Circadian and behavioural responses to shift work-like schedules of light/dark in the mouse. J. Mol. Psychiatry 1, 7. doi:10.1186/2049-9256-1-7
Mihelakis, M., Ndikung, J., Oelgeschlager, M., and Ertych, N. (2022). The 4th dimension of in vitro systems - time to level up. Environ. Int. 164, 107256. doi:10.1016/j.envint.2022.107256
Mohawk, J. A., Green, C. B., and Takahashi, J. S. (2012). Central and peripheral circadian clocks in mammals. Annu. Rev. Neurosci. 35, 445–462. doi:10.1146/annurev-neuro-060909-153128
Mohren, D. C., Jansen, N. W., Kant, I. J., Galama, J., van den Brandt, P. A., and Swaen, G. M. (2002). Prevalence of common infections among employees in different work schedules. J. Occup. Environ. Med. 44, 1003–1011. doi:10.1097/00043764-200211000-00005
Morf, J., Rey, G., Schneider, K., Stratmann, M., Fujita, J., Naef, F., et al. (2012). Cold-inducible RNA-binding protein modulates circadian gene expression posttranscriptionally. Science 338, 379–383. doi:10.1126/science.1217726
Morf, J., and Schibler, U. (2013). Body temperature cycles: gatekeepers of circadian clocks. Cell Cycle 12, 539–540. doi:10.4161/cc.23670
Nagoshi, E., Saini, C., Bauer, C., Laroche, T., Naef, F., and Schibler, U. (2004). Circadian gene expression in individual fibroblasts: cell-autonomous and self-sustained oscillators pass time to daughter cells. Cell 119, 693–705. doi:10.1016/j.cell.2004.11.015
Narasimamurthy, R., and Virshup, D. M. (2017). Molecular mechanisms regulating temperature compensation of the circadian clock. Front. Neurol. 8, 161. doi:10.3389/fneur.2017.00161
Ndikung, J., Storm, D., Violet, N., Kramer, A., Schonfelder, G., Ertych, N., et al. (2020). Restoring circadian synchrony in vitro facilitates physiological responses to environmental chemicals. Environ. Int. 134, 105265. doi:10.1016/j.envint.2019.105265
Nudell, V., Wei, H., Nievergelt, C., Maihofer, A. X., Shilling, P., Alda, M., et al. (2019). Entrainment of circadian rhythms to temperature reveals amplitude deficits in fibroblasts from patients with bipolar disorder and possible links to calcium channels. Mol. Neuropsychiatry 5, 115–124. doi:10.1159/000497354
Pierre, K., Schlesinger, N., and Androulakis, I. P. (2016). The role of the hypothalamic-pituitary-adrenal axis in modulating seasonal changes in immunity. Physiol. Genomics 48, 719–738. doi:10.1152/physiolgenomics.00006.2016
Poletini, M. O., Moraes, M. N., Ramos, B. C., Jeronimo, R., and Castrucci, A. M. (2015). TRP channels: a missing bond in the entrainment mechanism of peripheral clocks throughout evolution. Temp. (Austin) 2, 522–534. doi:10.1080/23328940.2015.1115803
Prolo, L. M., Takahashi, J. S., and Herzog, E. D. (2005). Circadian rhythm generation and entrainment in astrocytes. J. Neurosci. 25, 404–408. doi:10.1523/JNEUROSCI.4133-04.2005
Rajaratnam, S. M., Howard, M. E., and Grunstein, R. R. (2013). Sleep loss and circadian disruption in shift work: health burden and management. Med. J. Aust. 199, S11–S15. doi:10.5694/mja13.10561
Rao, R., and Androulakis, I. P. (2019). Allostatic adaptation and personalized physiological trade-offs in the circadian regulation of the HPA axis: a mathematical modeling approach. Sci. Rep. 9, 11212. doi:10.1038/s41598-019-47605-7
Rao, R. T., and Androulakis, I. P. (2017). Modeling the sex differences and interindividual variability in the activity of the hypothalamic-pituitary-adrenal Axis. Endocrinology 158, 4017–4037. doi:10.1210/en.2017-00544
Refinetti, R. (2020). Circadian rhythmicity of body temperature and metabolism. Temp. (Austin) 7, 321–362. doi:10.1080/23328940.2020.1743605
Reinberg, A., Andlauer, P., Guillet, P., Nicolai, A., Vieux, N., and Laporte, A. (1980). Oral temperature, circadian rhythm amplitude, ageing and tolerance to shift-work. Ergonomics 23, 55–64. doi:10.1080/00140138008924718
Reinberg, A., Motohashi, Y., Bourdeleau, P., Andlauer, P., Levi, F., and Bicakova-Rocher, A. (1988). Alteration of period and amplitude of circadian rhythms in shift workers. With special reference to temperature, right and left hand grip strength. Eur. Joumal Appl. Physiology 57, 15–25. doi:10.1007/BF00691232
Reinke, H., Saini, C., Fleury-Olela, F., Dibner, C., Benjamin, I. J., and Schibler, U. (2008). Differential display of DNA-binding proteins reveals heat-shock factor 1 as a circadian transcription factor. Genes Dev. 22, 331–345. doi:10.1101/gad.453808
Reppert, S. M., and Weaver, D. R. (2001). Molecular analysis of mammalian circadian rhythms. Annu. Rev. Physiology 63, 647–676. doi:10.1146/annurev.physiol.63.1.647
Rieger, T. R., Morimoto, R. I., and Hatzimanikatis, V. (2005). Mathematical modeling of the eukaryotic heat-shock response: dynamics of the hsp70 promoter. Biophys. J. 88, 1646–1658. doi:10.1529/biophysj.104.055301
Romanovsky, A. A. (2007). Thermoregulation: some concepts have changed. Functional architecture of the thermoregulatory system. Am. J. Physiol. Regul. Integr. Comp. Physiol. 292, R37–R46. doi:10.1152/ajpregu.00668.2006
Saini, C., Morf, J., Stratmann, M., Gos, P., and Schibler, U. (2012). Simulated body temperature rhythms reveal the phase-shifting behavior and plasticity of mammalian circadian oscillators. Genes Dev. 26, 567–580. doi:10.1101/gad.183251.111
Scheff, J. D., Stallings, J. D., Reifman, J., and Rakesh, V. (2015). Mathematical modeling of the heat-shock response in HeLa cells. Biophys. J. 109, 182–193. doi:10.1016/j.bpj.2015.06.027
Scherholz, M. L., Rao, R. T., and Androulakis, I. P. (2020). Modeling inter-sex and inter-individual variability in response to chronopharmacological administration of synthetic glucocorticoids. Chronobiology Int. 37, 281–296. doi:10.1080/07420528.2019.1660357
Schmal, C., Myung, J., Herzel, H., and Bordyugov, G. (2015). A theoretical study on seasonality. Front. Neurol. 6, 94. doi:10.3389/fneur.2015.00094
Stokkan, K.-A., Yamazaki, S., Tei, H., Sakaki, Y., and Menaker, M. (2001). Entrainment of the circadian clock in the liver by feeding. Science 291, 490–493. doi:10.1126/science.291.5503.490
Stowie, A., Ellis, I., Adams, K., Castanon-Cervantes, O., and Davidson, A. J. (2019). A reductionist, in vitro model of environmental circadian disruption demonstrates SCN-independent and tissue-specific dysregulation of inflammatory responses. PLoS One 14, e0217368. doi:10.1371/journal.pone.0217368
Stratmann, M., and Schibler, U. (2006). Properties, entrainment, and physiological functions of mammalian peripheral oscillators. J. Biol. Rhythms 21, 494–506. doi:10.1177/0748730406293889
Tamaru, T., Hattori, M., Honda, K., Benjamin, I., Ozawa, T., and Takamatsu, K. (2011). Synchronization of circadian Per2 rhythms and HSF1-BMAL1:CLOCK interaction in mouse fibroblasts after short-term heat shock pulse. PLoS One 6, e24521. doi:10.1371/journal.pone.0024521
Vriens, J., Nilius, B., and Voets, T. (2014). Peripheral thermosensation in mammals. Nat. Rev. Neurosci. 15, 573–589. doi:10.1038/nrn3784
Wang, H., and Siemens, J. (2015). TRP ion channels in thermosensation, thermoregulation and metabolism. Temp. (Austin) 2, 178–187. doi:10.1080/23328940.2015.1040604
Wang, X. S., Armstrong, M. E., Cairns, B. J., Key, T. J., and Travis, R. C. (2011). Shift work and chronic disease: the epidemiological evidence. Occup. Med. (Lond) 61, 78–89. doi:10.1093/occmed/kqr001
Welsh, D. K., Yoo, S. H., Liu, A. C., Takahashi, J. S., and Kay, S. A. (2004). Bioluminescence imaging of individual fibroblasts reveals persistent, independently phased circadian rhythms of clock gene expression. Curr. Biol. 14, 2289–2295. doi:10.1016/j.cub.2004.11.057
Xiao, R., and Xu, X. Z. S. (2021). Temperature sensation: from molecular thermosensors to neural circuits and coding principles. Annu. Rev. Physiol. 83, 205–230. doi:10.1146/annurev-physiol-031220-095215
Yamazaki, S., Numano, R., Abe, M., Hida, A., Takahashi, R.-i., Ueda, M., et al. (2000). Resetting central and peripheral circadian oscillators in transgenic rats. Science 288, 682–685. doi:10.1126/science.288.5466.682
Yamazaki, S., Straume, M., Tei, H., Sakaki, Y., Menaker, M., and Block, G. D. (2002). Effects of aging on central and peripheral mammalian clocks. Proc. Natl. Acad. Sci. U. S. A. 99, 10801–10806. doi:10.1073/pnas.152318499
Yao, J., Liu, B., and Qin, F. (2011). Modular thermal sensors in temperature-gated transient receptor potential (TRP) channels. Proc. Natl. Acad. Sci. U. S. A. 108, 11109–11114. doi:10.1073/pnas.1105196108
Appendix A
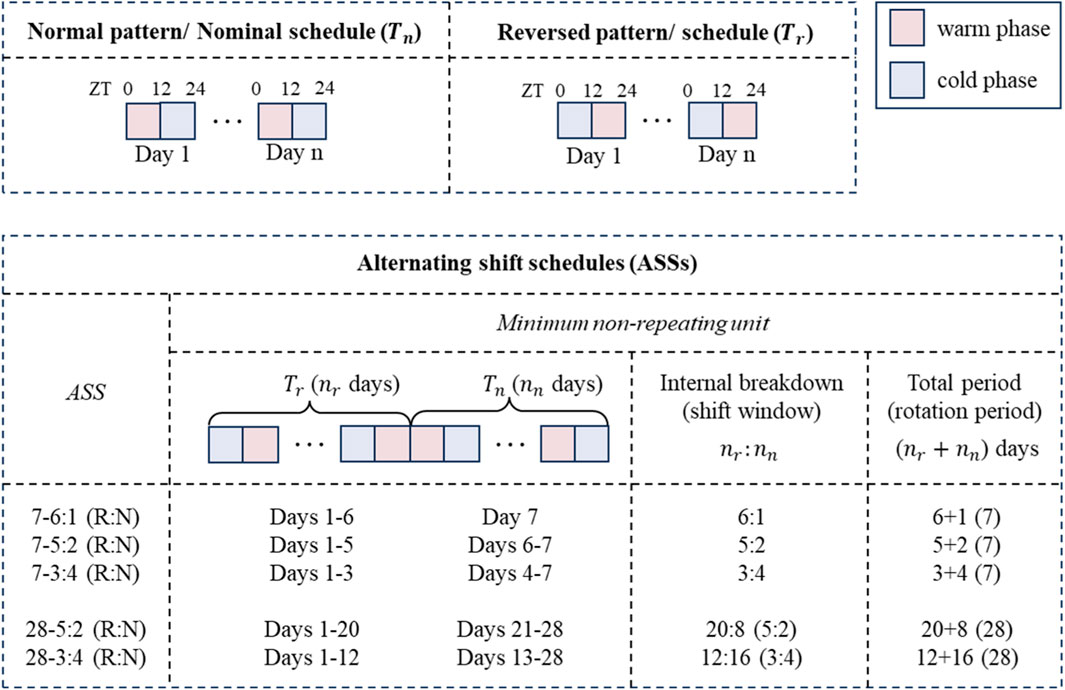
FIGURE A1. Schematic of temperature schedules. The nominal temperature schedule comprises recurring normal temperature patterns (
Keywords: circadian, systems biology, temperature, shiftwork, circadian disruption (CD)
Citation: Lu L, Li Y, Schloss R and Androulakis IP (2024) Mathematical modeling of temperature-induced circadian rhythms. Front. Syst. Biol. 4:1256398. doi: 10.3389/fsysb.2024.1256398
Received: 10 July 2023; Accepted: 05 March 2024;
Published: 25 March 2024.
Edited by:
Yoram Vodovotz, University of Pittsburgh, United StatesReviewed by:
Takeshi Hase, Tokyo Medical and Dental University, JapanAshok Prasad, Colorado State University, United States
Copyright © 2024 Lu, Li, Schloss and Androulakis. This is an open-access article distributed under the terms of the Creative Commons Attribution License (CC BY). The use, distribution or reproduction in other forums is permitted, provided the original author(s) and the copyright owner(s) are credited and that the original publication in this journal is cited, in accordance with accepted academic practice. No use, distribution or reproduction is permitted which does not comply with these terms.
*Correspondence: Ioannis P. Androulakis, eWFubmlzQHNvZS5ydXRnZXJzLmVkdQ==