- 1College of Health Solutions and School of Molecular Sciences, Arizona State University, Phoenix, AZ, United States
- 2Department of Chemical and Life Science Engineering, Virginia Commonwealth University, Richmond, VA, United States
- 3School of Sustainable Engineering and the Built Environment, Ira A. Fulton School of Engineering, Arizona State University, Tempe, AZ, United States
More than 90% of all the roads in the United States are covered with asphalt, despite hundreds of scientific studies demonstrating the detrimental effect of asphalt on human health. Asphalt is a complex mixture of thousands of compounds. Here, we not only review studies of the effects of asphalt on human health, but go a step further by taking a novel view of these health effects from a systems biology perspective. In particular, we propose an analogy to protein-protein interaction networks, which can be within species and across species when looking at host-pathogen interactions. While in the former, all nodes are of the same type (e.g., human proteins), in the latter nodes can be of different types, such as human proteins and pathogen proteins. To build a corresponding network of interactions between different nodes for asphalt, we retrieved the literature studying the molecular targets of identified components in asphalt and their corresponding cellular biomarkers. Using this approach, we show that a complex trans pollutant-human target network appears in which multiple health effects can be triggered through interactions of multiple pollutant molecules with multiple human targets. We envision that the insights gained from this analysis may assist future efforts at regulating the use of asphalt.
1 Introduction
Asphalt is used in roofing, paving, crack filling, sealing, water proofing, and many other applications. It generally refers to a mixture of largely rocks and a binder, an adhesive which keeps the rocks together. Bitumen is typically used as binder in asphalt. This review investigates the effects of asphalt exposure on human health from a systems biology perspective. When we think of systems biology, we think of the biological system and its complexity, where a birds-eye view is needed to understand the response of an organism to a specific disease or environmental stress. Comparative analysis has shown that there is extensive overlap in these responses to disparate stresses. In the case of asphalt, even the stressor is complex, requiring its own birds-eye view. This type of scenario has been studied extensively previously for a different stressor, namely infectious disease. In that scenario, we have a complex organism, a pathogen such as bacteria, e.g., Salmonella, or virus, e.g., Sars-CoV-2 or HIV, and the human host organism. Much work has been carried out in understanding such complex networks [e.g., (Qi et al., 2006; Tastan et al., 2009; Kshirsagar et al., 2015)]. With the development of -omics experimental technologies, it became quickly clear that identification of the full complement of all interacting proteins in an organism requires help from computer science. Computational models using machine learning approaches were created to predict new interactions from previously known interactions. The emphasis was initially on intraspecies protein-protein interactions, where the goal was to identify all edges (representing physical interactions) connecting nodes (representing proteins) in a single organism, such as human [e.g., (Qi et al., 2006)]. The concept was then extended to identification of cross-species protein-protein interactions, where one type of node was the human proteins whereas a second type of node, the pathogen protein was introduced [e.g., (Tastan et al., 2009)]. This trans-network prediction task comes with challenges, because most data sources are only available for nodes of the same types. The first step in creating such a network is the identification of edges that are connecting nodes of different types, e.g., the edges connecting human with pathogen proteins. This step is essential when developing reliable models, although transfer learning approaches can then be used to extrapolate from well-studied organisms to less well studied organisms (Kshirsagar et al., 2015).
We here propose that we can view the exposure to asphalt in direct analogy to host-pathogen interaction networks. Asphalt used in road and roofing materials contains tens of thousands of different chemicals (Khare et al., 2020; Breuer et al., 2011). Thus, the components of asphalt are one type of node, and they connect directly to asphalt as a parent mixture. Only a fraction of those chemicals has been studied in isolation for the impacts on human health, and of those, only a small selection has regulated exposure limits. The complexity of the pollutant (asphalt fumes) has made demonstrating direct correlations between exposure and potentially harmful health effects challenging. As a result, the current basis for protecting people from harm by asphalt exposure rests on a single or small subset of chemicals being below a given exposure limit. Cumulative effects of multiple toxic compounds are currently neglected. This review aims to argue that understanding the harmful effects of complex pollutants on health requires a systems-level view of the pollutant as well as the biological responses to its exposure. The effects on human health are studied by identification of biomarkers of exposure, and this forms a different type of node in the network, that of type “biomarker.” Within this type of node, we can (but do not have to) define additional subclasses, such as proteins vs. small molecules. Given, that only few biomarkers have been directly linked to specific pollutants is highly analogous to the host-pathogen interaction network which are usually also very sparse. In that field, machine learning made a huge impact by taking the small set of identified nodes and edges as a gold standard and developing models that are able to predict previously unknown interactions. What if we can develop computational models in the future that can take these few known interactions and predict a complex network of interactions? We here develop the first step towards this long-term goal, by creating such a “gold standard” dataset of linked interactions between pollutants and biomarkers of exposure. Using such a systems level approach to advance human health is at the heart of translational systems biology.
In the field of understanding the effects of complex pollutants such as asphalt on human health, translational systems biology may thus be able to support the global emphasis toward emission-curbing roads to drive environmental health, and some approaches are briefly discussed here. The translational goal of our proposed systems view of asphalt is to further the dialogue on improving sensing methods, exposure thresholds, quantification of biological consequences and removal of those compounds that cause greatest harm for the protection of public health. The study outcomes provide insights to road authorities and practitioners to include emission limits among design criteria to complement existing performance-driven criteria. Our review is based on the analysis of a PubMed search conducted on 1 March 2022 of “asphalt” as supplementary concept, and which yielded 827 publications, and search term (“asphalt fume” and “bitumen”) and filters (English, Human, Other Animals) which yielded 387 papers and the corresponding OR logic which yielded 748 papers (Supplementary File S1). An overview of the review showing the complexity of asphalt’s chemical composition and its dependence on environmental conditions and additives, the effects on emissions and consequently on human health are shown in Figure 1.
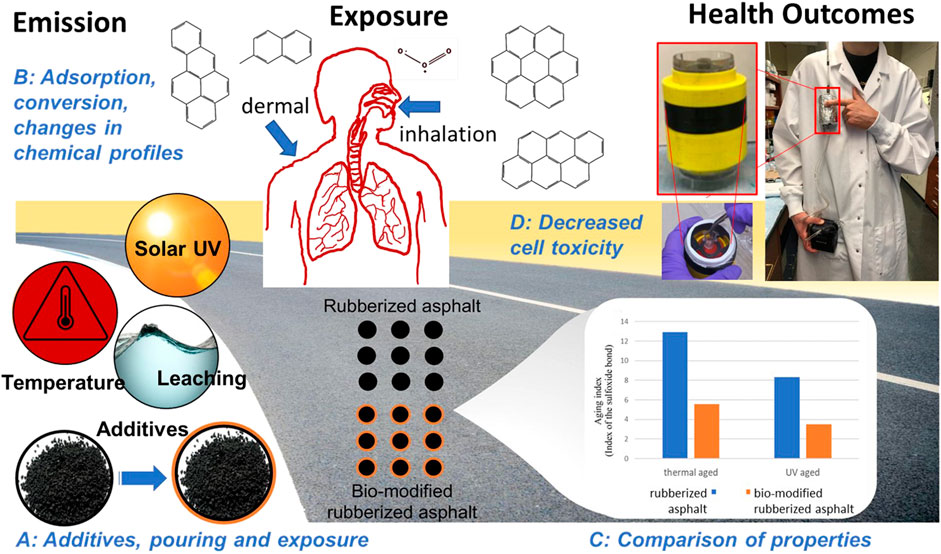
FIGURE 1. Overview of asphalt research: From modifications of asphalt materials and resulting changes in emission (Rajib and Fini, 2020; Mousavi et al., 2021; Lyu et al., 2022) to studying the health effects of these modifications (Secondo et al., 2019; Secondo et al., 2022). Understanding health effects of asphalt requires investigation of emission, exposure and health outcomes, and interventions at the following stages, A–D. Stage (A) Additives, pouring and exposure: Modifications to asphalt compositions as well as subsequent changes aggravated by environmental conditions such as light and heat can affect the emissions of asphalt throughout its life-cycle. For example, bio-modified rubberized asphalt binder is shown as an example in this figure. Traditionally, the physicochemical properties of bio-modified vs. non-modified rubberized asphalt was compared. Similar arguments can be made for other modifications to asphalt, e.g., the addition of inherently functionalized carbon from lipid and protein-rich biomass such as biowaste or algae originally studied to reduce ultraviolet-induced damages in bituminous materials (Lyu et al., 2022). Stage (B): Adsorption, conversion and changes in chemical profiles: The effects of the modifications made in (A) on emissions needs to be quantified. For example, addition of functionalized carbon adsorbs precursors of secondary organic aerosols and thus directly affect emissions. Stage (C): Comparison of properties. Traditionally, changes in performance criteria of the material itself are studied. What is needed, is to couple the changes in properties to health effects. This is Stage (D): Decreased cell toxicity. A new portable in vitro exposure cassette for aerosol sampling will allow quantifying the changes made to emissions to health effects, because the cassette for the first time allows real-time monitoring of cellular oxidative stress (Secondo et al., 2019; Secondo et al., 2022).
2 Effects of asphalt on general health
What are the health concerns with asphalt emissions? The carcinogenicity of complex carbonaceous materials, such as coal tars, bitumens, and incomplete combustion products, have been researched and recorded for hundreds of years, with the first human evidence documented by Percival Pott who identified the scrotum cancer of chimney sweeps to be due to their occupational exposure to soot in chimneys in 1775 (Pott, 1775; Abboud, 2017). Soot and asphalt have much in common, e.g., Benzo [a] pyrene (BaP) is a significant component of both soot and asphalt and is listed as a “potent carcinogen” by the International Agency for Research on Cancer (IARC) (World Health Organization WHO : International Agency for Research on Cancer IARC, 1973). In an effort to reduce occupational exposure risks, the 1907 Workers Compensation Act in Britain made employers liable for workplace exposures to tar or pitch substances leading to skin cancer (World Health Organization WHO : International Agency for Research on Cancer IARC, 1973). Shamberg in 1910 found that the control measures implemented in Britain to reduce the exposure to soot by chimney sweeps resulted in a corresponding decrease in scrotal cancer (World Health Organization WHO : International Agency for Research on Cancer IARC, 1973).
Conclusion as to the carcinogenicity of complex carbonaceous materials such as asphalt have been confounded by the variations in its composition throughout the efforts in the 1900s to improve workplace exposures. Bitumen is known to have significantly lower volatile organic compound (VOC), including polycyclic aromatic hydrocarbons (PAHs), emissions than coal tar (Burstyn et al., 2002). While this knowledge led some countries to start phasing out the use of coal tar in asphalt prior to the 1970s, coal tar was still added to bitumen through the 1990s in some countries (Riala et al., 1998). This difference in real exposure by age and location means that asphalt workers cannot be considered as a single population for epidemiological studies. Further, in areas where petroleum-based bitumen is typically used, workers were still at risk of exposure to coal tar when repairing and replacing roads that were paved prior to the change. However, the carcinogenicity of bitumen was unclear in part because of the co-exposure of asphalt workers to both coal tar and bitumen asphalt products (Burstyn et al., 2002). Going a step further, Boffetta et al. (2003) reported that workers exposed to bitumen fumes had a reduced risk of lung cancer compared to those workers who were also exposed to coal tar fumes. However, criticisms of the 2001 IARC epidemiological study suggested that the cancer risks of bitumens were underestimated because the PAHs analyzed were not the fractions of bitumen fume condensates (BFC) that are known to have major genotoxic effects (Binet et al., 2002).
Additionally, lifestyle choices and location are frequent confounding factors. The “Healthy Worker Effect,” which is a selection bias that workers in a particular field are healthier than persons in the general population that are not employed, may mask the epidemiologically identifiable risk associated with asphalt work through an undercount of mortality (Chowdhury et al., 2017). In the 1950s it was reported that non-smokers living in cities had twice the risk of death from lung cancer as those living in rural areas (Merlo et al., 1998). This further confounds the evidence available to link asphalt with disease as it becomes necessary to parse out the effects of smoking with a higher concentration of roads and other emission sources. Further, multiple exposure routes can influence measured biomarkers and metabolic products. For example, dietary exposures, occupational exposures and smoking can all increase the urinary concentration of the polycyclic aromatic hydrocarbons (PAH) exposure marker, 1-hydroxypyrene (1-OHPY) (Jongeneelen et al., 1988; Tolos et al., 1990; Buckley and Lioy, 1992; Merlo et al., 1998; Väänänen et al., 2005; Väänänen et al., 2006; Sobus et al., 2009a). Therefore, measurements of 1-OHPY cannot differentiate between BaP exposure from asphalt or from other lifestyle exposures in food or smoking. Finally, there may also be a genetic component to the physiologic response and susceptibility to health effects from environmental exposures (Zanobetti et al., 2011).
The connection between asphalt and cancer remains to this date controversial. An epidemiological study from 2002 reported that the lung cancer risk to bitumen fume exposed workers was comparable to workers without bitumen fume exposure (Boffetta et al., 2003). A separate epidemiology study by Randem et al. (2003), in 2003 identified an increased risk of lung cancer, but that the increase in risk could be explained by smoking habits or exposures to coal tar earlier in the asphalt worker’s career. Additionally, a literature review by Kriech et al. (2018), in 2018, funded in part by the Asphalt Institute, concluded that the available research did not provide robust conclusions as to the carcinogenicity of bitumen fume exposure in most of the research reviewed when applying modified quality ratings designed by Klimisch. They pointed to the differences in composition of bitumen used, exposure risk is higher at higher temperatures that are not experienced by the vast majority of asphalt workers, and that markers of inflammation and genotoxicity in vivo and in vitro studies did not correlate with evidence from animal and human studies (Kriech et al., 2018). Other reviews published at this time, including Mundt et al. (2018) and Rhomberg et al. (2018) also came to the conclusion that there is not a statistically significant increase in risk of cancer when aggregating the evidence from human studies on asphalt exposure published to date. These discrepancies are likely due to the complexity of the composition of asphalt as well as the complexity of the human body reacting to the thousands of compounds in asphalt when exposed.
Cancer has been linked to genotoxicity, a term used to describe the DNA damage or chromosomal damage caused by a chemical agent. The DNA damage induced by genotoxins can result in somatic mutation, which can ultimately lead to the development of cancer in the cells (Phillips et al., 2009). Genotoxicity can be determined in a variety of ways, including the comet assay (% DNA in tail), PCR-based tests, immunoassays, DNA stand breaks, and oxidative processes. For example, DNA breaks in human lung epithelial (BEAS-2B) cells have been observed when adding waste plastic and a tall oil pitch stone-mastic asphalt (SMA) mixture (Lindberg et al., 2008). On the other hand, there was no noticeable difference in DNA damage in buccal leukocytes between exposed and unexposed employees (Lindberg et al., 2008). A 2004 animal in vivo study examined DNA damage in pathogen-free female Sprague–Dawley rats using the comet assay. The rats were subjected to various concentrations of road paving asphalt fume and compared to rats exposed to air at the same concentrations to illustrate the clear effect of asphalt exposure on DNA damage. According to the results of this experiment, asphalt exposure caused DNA damage in alveolar macrophages and lung tissue, and the higher the amount of asphalt fume, the more DNA damage occurs. On the other hand, increasing air levels seemed to have no effect on DNA damage in the same way as asphalt exposure (Zhao et al., 2004). Thus, the results on genotoxicity of asphalt are inconclusive.
3 Molecular responses to asphalt exposure by route
Asphalt toxicity studies can also be classified based on the route of exposure, dermal and inhalation.
3.1 Dermal exposure
Dermal exposure to asphalt fumes was initially investigated in rodents with later studies conducted in humans through biomonitoring of workers plus in vitro and ex vivo tests. The rodent studies focused on measuring carcinogenicity of bitumen by applying undiluted bitumen to the skin of the animals as a single exposure (Hueper and Payne, 1960; Booth et al., 1998), daily for several weeks (Poon et al., 1994; Poon et al., 1996; Freeman et al., 2011) or multiple times per week over 2 years or their entire lifetime (Mckee et al., 1986; Sivak et al., 1997; Clark et al., 2011). Despite the high dosage and prolonged exposure duration, few animals developed tumors when exposed to undiluted bitumen or raw asphalt, whereas higher tumor incidence rates in mice exposed to neat asphalt fume daily for over a year were observed. While body weight was unaffected in the mice studies, a reduction in food intake and consequently a reduction in weight gain was observed in rats exposed daily for 4–13 weeks (Poon et al., 1994; Poon et al., 1996). Other endpoints such as changes in liver and spleen weights and blood parameters, such as hemoglobin, cholesterol, and iron binding capacity, demonstrate systemic effects of dermal exposure to bitumen and asphalt fumes. Although few tumors were observed, bitumen and asphalt fume are considered tumor initiators and skin irritants (Clark et al., 2011; Freeman et al., 2011). Biomonitoring studies corroborate the symptom of skin irritation which was reported in high percentages for roofers and road pavers (Riala et al., 1998). A study taking punch biopsies from road pavers revealed activation of apoptosis with chronic bitumen skin exposure, as determined from measuring overexpression of cytokeratin, bax, DR5, caspase-3, and TRAIL, underexpression of bcl-2, TUNEL positive cells, and epidermal thinning (Loreto et al., 2007; Rapisarda et al., 2009). Studies measuring dermal exposure either by observation (Agostini et al., 2011) or dermal patches/samplers (Jongeneelen et al., 1988; Burstyn et al., 2002; Väänänen et al., 2005; McClean et al., 2007; Sobus et al., 2009b; Fustinoni et al., 2010; Cavallari et al., 2011) revealed high concentrations of polyaromatic compounds, including phenanthrene, pyrene and BaP. Workers’ hands and wrists were most exposed as compared to the rest of the body due to limited use of gloves. Dermal exposure consistently correlated with urinary metabolite measurements. Rates of dermal penetration have been determined through ex vivo skin studies and range from .02 to 120 ng/cm2/h depending on the compound measured and the test conditions (Roy et al., 2007; Hopf et al., 2018). An increase in permeation and p53 expression was measured in skin exposed to UV-S as compared to those without UV-S exposure (Hopf et al., 2018). In vitro studies measuring cytotoxicity and carcinogenic potential revealed reduction in DNA content, inhibition of gap junction intercellular communication, and loss in cell viability at concentrations greater than 25 μg/ml (Wey et al., 1992; Ma et al., 2003a). AP-1 and PI3K activation was measured at asphalt fume concentration of 20 μg/ml, which correlated with observed anchorage independent growth. Thus, dermal application of asphalt in animal models and humans clearly causes skin irritation and molecular reactions to the exposure.
3.2 Inhalation exposure
While initial research on asphalt hazards focused on skin exposure due to prevalence of burns, inhalation is the route of exposure of most concern when considering vapor and aerosol emissions from asphalt. An early case report demonstrated an instance of hydrogen sulfide poisoning in workers exposed to roofing asphalt fumes when inspecting an asphalt tank without a respirator. Another study on exposure of road workers to PAHs from spraying bitumen stated the need for respiratory protection and work patterns which reduced exposure (Darby et al., 1986). With the development of inhalation exposure systems, rodent studies revealed changes in CYP1A1 and CYP1B1 levels and gene expression. These changes were measured in rats exposed from 6 h per day for 5 days (Ma et al., 2003b; Gate et al., 2006) to up to 2 years (Halter et al., 2007). CYP1A1 and CYP1B1 are members of the cytochrome P450 family and are involved in the metabolism of PAHs as well as the cell response to oxidative stress. In the 2-year chronic toxicity and carcinogenicity study with bitumen condensate, rats were exposed by nose only inhalation to paving bitumen for 6 h per day, 5 days per week to concentrations of 6.8, 34.4, and 172.5 mg/m3 of total hydrocarbons (both vapor and aerosol). Data collected from bronchiolar lavage, immunohistochemistry, and histopathology revealed local irritation but no systemic irritation effects (Fuhst et al., 2007). Additional endpoints included micronuclei formation, urinary metabolites, DNA adduct formation, oxidative DNA damage, and altered gene expression in the lungs (Halter et al., 2007). Here measurement of urinary metabolites of naphthalene and phenanthrene confirmed systemic uptake of bitumen emissions. No evidence of micronuclei formation or oxidative DNA damage were found, but DNA adducts were measured with a dose and time dependence. In a human study, nasal lavage fluid (NALF), induced sputum, and urine was collected from 74 asphalt workers and construction workers before and after their shifts over several days (Raulf-Heimsoth et al., 2007). The results indicated that the workers with exposures >10 mg/m3 reported increased coughing and eye irritation post shift that correlated with increased urinary PAH metabolites, increased neutrophil counts and cytokine concentrations in sputum, and a moderate decline in lung function. The decline in lung function was consistent over the measurement days compared to the reference population and indicated that the decline in function was an effect of a chronic exposure to asphalt fumes (Raulf-Heimsoth et al., 2007). This was further supported by a later study published by the same group with a larger cohort of 320 bitumen workers (Raulf-Heimsoth et al., 2011a). This group also compared the exposure of asphalt workers in tunnel construction where they used paving asphalt which is handled at 180°C then applied mastic asphalt which is handled at 250°C (Raulf-Heimsoth et al., 2011b). The higher temperature of mastic asphalt correlated with higher emissions and higher exposure concentrations; however, there were no measured differences in lung function, urinary metabolite excretion, or genotoxic markers. Risk of lung diseases other than cancer from occupational exposure to asphalt emissions has also been studied. Higher adverse respiratory symptoms, such as asthma and chronic obstructive pulmonary disease (COPD), were reported in asphalt workers as compared to outdoor construction workers (Randem et al., 2004). More recently, data analysis of a large public health survey further corroborates this finding with workers exposed to particles from asphalt/bitumen fumes found to have a higher risk of developing COPD when adjusted for smoking (Grahn et al., 2021).
4 Whose health is affected the most by asphalt emissions?
Asphalt workers are exposed to the highest concentrations of asphalt emissions since they are involved in the production and handling of hot asphalt. Occupational exposure studies have demonstrated that asphalt workers are exposed to different concentrations of emissions while performing different tasks such as paving, rolling, raking, and leveling. Higher exposures occur in confined spaces where emissions can accumulate. Engineering controls like ventilating tunnels where paving is occurring can reduce exposure risks significantly. Additionally, instead of the typically used “hot mix asphalt” where mixing temperatures are in the range of 140–190°C, there is also “warm mix asphalt,” where temperatures are lowered to 20–40°C (Rathore et al., 2021). The drop in temperature is intended to reduce asphalt emission and workers’ exposure during the compaction and placement (Farshidi et al., 2011).
Asphalt emissions also affect the general public because emissions continue during the service life of asphalt regardless of mixing temperature used during pavement. The severity of emissions largely depends on the combination of temperature and solar exposure during service (Figures 1A,B). For example, the loss of volatile compounds is increased by 300% due to UV light, and 70% for every 20°C increase in ambient temperature (Khare et al., 2020). The magnitude of these emissions during service life is substantial. Asphalt-related emissions account for more secondary organic aerosols (SOAs) than the combined emissions of gas and diesel on-road vehicles (Khare et al., 2020). Emissions were shown to include hydrocarbons and the particularly toxic sulfur/oxygen-containing compounds, the concentration of which increased sharply with increasing UV radiation (Khare et al., 2020). Therefore, the general population continues to be impacted by asphalt exposure during the service life of the road. Lastly, asphalt production facilities are also located within cities allowing for non-occupational exposures to asphalt emissions during production and transport.
Leachate of asphalt compounds via water does not appear to be an acute exposure risk to the general public. Bowen et al. (2000) for example reported that the leachate from nine bitumens studied was less than the limits for surface water runoff and more than ten times less than the limits for potable water in many European countries. Additionally, Kumpiene et al. (2021) reported that a detailed analysis needed to be made for the increased risk of disturbing the underlying road when removing layers made with coal tar for disposal as hazardous waste. They found that the leachate of the intact roads had low bioavailability and it was difficult to parse out the impact of automobile traffic from the impact of the leachate (Kumpiene et al., 2021). An earlier study conducted by Andersson-Sköld et al. (2007) considered the total risks of removal including leaching from temporary storage, leaching from landfills, the production of virgin materials, transportation of both the waste and virgin road materials, among other factors. They found that from a lifecycle perspective that included environmental concerns, the reuse of the material in place outweighed the low leachate risk from the coal tar containing roads of the leachate from roads with coal tar (Andersson-Sköld et al., 2007). However, this analysis focuses predominately on the measurement of the 16 priority pollutants identified by the United States Environmental Protection Service (EPA), which are only a fraction of the emissions from asphalt. Further, these analyses do not consider the accumulative nature of toxicity of the individual compounds and combined exposure effects to occupational workers exposed during the road work and the general public. Therefore, leachate during service life and on removal for disposal or recycling pose an additional risk for occupational and non-occupational exposures.
5 Composition of asphalt and its emissions: A complex system
In most studies of the adverse health outcomes of asphalt described above, asphalt was treated as a “black box” of undefined composition. Recent work has begun to unravel the chemical composition of asphalt. Asphalt is the name of a collection of products that is generally composed of mineral aggregates (rocks, 90%–95% by weight) and a binder (adhesive, 5%–10% by weight) which keeps the aggregates together. Historically, both bitumen and coal tar have been used as the binder in asphalt. Bitumen can be naturally occurring or obtained as a byproduct of oil refining. Coal tar is a byproduct of coal and petroleum production. To enhance performance of asphalt, liquid and solid modifiers are typically introduced to the mixture (as indicated in Figure 1A). Among those have been wax, coal, lime, rubber, polyphosphoric acid, various polymers and plastics, plus siliceous, calcareous and carbonaceous particles to name a few.
Different bitumen grades have different chemical profiles which further change when additives and modifiers are added to bitumen in the blending terminal or at the asphalt plant (Khare et al., 2020; Boffetta et al., 2003; Farshidi et al., 2011). During the preparation, deployment, and throughout its lifetime, asphalt emits volatile compounds, as indicated in Figure 1B. Some of these compounds are listed in Table 1, including but not limited to PAH (Khare et al., 2020; Breuer et al., 2011; Burstyn et al., 2002; Boffetta et al., 2003; Väänänen et al., 2005; Bowen et al., 2000; Kumpiene et al., 2021; Andersson-Sköld et al., 2007). Recently, the full complexity of these emissions has been highlighted in a comprehensive mass spectrometric analysis of asphalt fumes in situ (Khare et al., 2020). Adding to the complexity further, emissions from asphalt change with the age, use, temperature and UV exposure of the asphalt, not only in the total amount emitted, but also in the types of chemicals emitted (Rühl et al., 2006; Hung et al., 2019; Hung and Fini, 2020). As a result, researchers tend toward measuring the 16 priority polycyclic aromatic hydrocarbons (PAH) published by the EPA when reporting the chemical constituents of bitumen (Tolos et al., 1990; Bowen et al., 2000; Wang et al., 2001; Burstyn et al., 2002; Väänänen et al., 2005; Breuer et al., 2011; Schreiner, 2011; Rhomberg et al., 2018).
Consequently, recreating the fumes experienced in situ in a laboratory environment is challenging. It is even more difficult to recreate the seasonal variation of emissions experienced by road users. Every batch of asphalt and bitumen is different because the focus is on performance-based grading criteria such as the SuperPave specification and Balanced Mix Design to avoid fatigue and rutting cracking while not accounting for emissions during construction or later in service life of asphalt surfaced areas (New Report Provides Guidelines on Constructing Superpave Pavements [Internet], 1998; Balanced Mix Design, 2021). These commonly used grading systems are blind to chemical composition as it pertains to health, and are not included as a design criterion. The total emission is usually accounted for by placing a limit on total allowable mass loss; mass loss should not exceed .01 percent of the total sample (Colorado Procedure - Laboratory, 2020). Therefore, the mass loss composition and health effect of each constituent contained in this total mass loss are not accounted for. Further, different applications of asphalt products require different temperatures and different tools which can change a worker’s proximity to the fumes and the composition of the fumes (Programme international sur la sécurité, 2004).
Due to the complexity of asphalt composition and the challenges of collecting volatile samples as a function of time and exposure conditions, there are few labs that are capable of performing a comprehensive, let alone complete, mass spectrometric analysis of the aerosols and vapors. Instead, the EPA has created in 1977 a list of 16 PAHs as environmental contaminants of priority concern (Keith, 2015), which has since been used as a proxy for PAH contamination as a whole. This list includes naphthalene, acenaphthylene, acenaphthene, fluorene, phenanthrene, anthracene, fluoranthene, pyrene, benzo [a] anthracene, chrysene, benzo [b] fluoranthene, benzo [k] fluoranthene, benzo [a] pyrene (BaP), benzo [g,h,i] perylene, indeno [1,2,3-c,d] pyrene, and dibenz [a,h] anthracene, the structures of which are also provided in reference (Keith, 2015). While these 16 compounds have been identified in bitumen fumes, they are not the chemicals of greatest threat to human health in bitumen condensate fumes (see more details below). In particular, oxygen, nitrogen, and sulfur analogues of PAHs, are known to have greater genotoxic effects than some of the 16 PAH (Binet et al., 2002; Andersson-Sköld et al., 2007; Samburova et al., 2017). The concentrations of these analogues depend on composition, for example they are greater in bitumen fumes than in coal tar fumes (Binet et al., 2002). The original list of 16 published nearly half a century ago (Keith, 2015) was a regulatory compromise considering the analytical abilities at the time and practical considerations, early studies of health effects, existing regulatory exposure limits, and pervasiveness in environmental samples (Keith, 2015). Specifically, naphthalene, acenaphthene, and fluoranthene were in an earlier list of 65 toxic pollutants from the settlement agreement between the EPA and environmental advocacy organizations (Keith, 2015). Benzo [a] anthracene, chrysene, benzo [b] fluoranthene, benzo [k] fluoranthene, BaP, indeno [1,2,3-c,d] pyrene, and dibenz [a,h] anthracene were selected because they occurred frequently in water in an 1976 EPA database, were manufactured in high quantities, and were commercially available so that a representative sample was available for GC-MS identification. Acenaphthylene, fluorene, and phenanthrene were chosen because they were described in a 1975 EPA report on “Suspect Carcinogens in Water Supplies” (Keith, 2015). Anthracene and pyrene were selected because they are common industrial chemicals produced from coal tar. Finally, benzo [g,h,i] perylene was chosen to represent a PAH with a 6-membered ring (Keith, 2015). Suggestions have been made to expand the list now that the analytical techniques have improved and the exposure effects of more chemicals are known (Andersson and Achten, 2015). Demonstrating the inadequacy of the list of 16 compounds, the airborne toxicity measured using only these 16 PAHs accounted for .2%–41.9% of the measured airborne toxicity using 88 PAHs (Samburova et al., 2017). Despite these shortcomings, the value of the continued use of the 16 PAHs as a measurement of air quality is that it allows for standardization of measurement and ease of comparison between studies in scientific research as shown in Table 1. The selection of studies represents international efforts, including asphalt research from Norway, Netherlands, Finland, Germany, and the United States of America. Because of the standardization of the 16 PAH, the research can be readily compared. While the inclusion of additional compounds varies across these studies (see bottom half of Table 1), they are referenced by the inclusion of the 16 PAH (upper half of Table 1).
6 Exposure limits
Despite the crucial role of the 16 PAH in standardization, the exposure limits for coal tar fumes, asphalt, bitumen, and some of the chemical components that are measured in the emitted vapors and aerosols cannot be compared directly between countries or regulatory agencies. This is because the exposure limit is tied to the method of measurement of the material. For instance, BGIA method No. 6305 in Germany measures the vapors and the aerosols emitted from asphalt, capturing more of the emissions than the United States National Institute for Occupational Safety and Health (NIOSH) measurement method No. 5042 that collects only the aerosols (Breuer et al., 2011). To account for the difference in method, the German exposure limit in 2006 was 20 times greater than the United States (Rühl et al., 2006). The NIOSH exposure limit of 5 mg/m3 for 15 min, was originally proposed in 1977 to protect against irritation of the eyes and respiratory tract and not as a protection from other health consequences of exposure to vapors and aerosols. At the time, NIOSH had determined that the research results did not provide sufficient evidence for proposing an exposure limit based on potential biological effects of asphalt fumes including cancer risks (Tepper et al., 2006). NIOSH recommended exposure limits and OSHA permissible exposure limits are listed in Table 2 for all 16 PAH and other pollutants with regulated exposure limits. Some are not regulated as individual exposures but instead as cumulative exposures (denoted by * in Table 2). The additional compounds listed in Table 2 reflect additional compounds measured in relation to asphalt research reflected in Table 1 above and Figure 3 in the subsequent sections.
To consider the fact that multiple compounds are present in asphalt simultaneously, “toxicity equivalency factors” (TEF) have been developed for 88 PAHs to find the combined “carcinogenic potency” in a given sample (Samburova et al., 2017). The TEF is a means of comparing the toxicity of a chemical to a reference chemical, which in this case is BaP. Because much of the research on toxicity focused on the 16 priority PAHs, there were only TEFs for them and one other PAH so the TEFs for other compounds was based on the molecular similarity to one of the compounds with a known TEF (Samburova et al., 2017). While the relative exposure risks of the sample environments measured by Samburova et al. (2017), which included urban air, biomass burning, tunnel engine emissions, meat cooking and mining, are comparable to each other, it is likely an underestimate of the exposure risk. This is because the method employed by Samburova et al. (2017) to determine approximate TEF for compounds without known TEFs identified 60 of the 88 constituents as having a TEF of .001, the lowest TEF value. Additionally, dibenzo [a,i] pyrene, which is present in asphalt but does not have an exposure limit, is about 10 times more carcinogenically potent than BaP (Andersson and Achten, 2015). Finally, NIOSH method No. 5042 for measuring asphalt fumes is non-specific and cannot resolve beyond total particulate and benzene-soluble fraction of total particulate. NIOSH method No. 5042 directs the user to NIOSH method No. 5800 for the measurement of Total Polycyclic Aromatic Compounds (NIOSH Manual of Analytical Methods (NMAM TM ) [Internet], 1994). However, the procedure cannot be used to compare the results to the regulatory exposure limits as it provides an indication of exposure and is not selective to the 16 PAHs (NIOSH Manual of Analytical Methods (NMAM TM ) [Internet], 1994). Therefore, current testing methods employed by regulators are not granular enough to measure whether the limit has been exceeded, and using only the 16 priority pollutants does not capture the true risks of adverse health effects afflicting people who have been exposed.
7 Mechanisms of toxicity: Systems biology of health effects
The availability of compositional information now allows us to begin to detail the contributions of individual chemicals emitted from asphalt and understand potentially synergistic effects. Both asphalt mixtures, as well as individual components have been tested extensively on laboratory animals and mammalian cell cultures. Numerous biological assays and biomarkers have been investigated (see Supplementary File S2). A subset of the best studied biomarker effects are depicted in Figure 2 as a network graph, highlighting those studies where a direct link to individual components within asphalt mixtures was established.
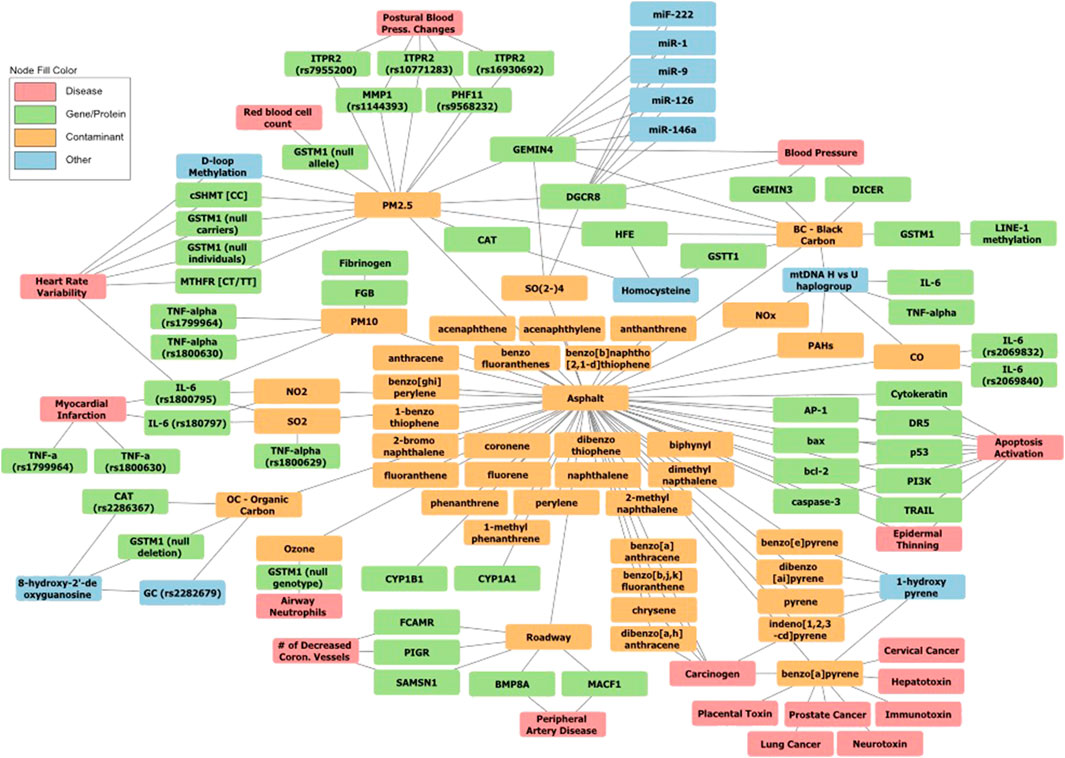
FIGURE 2. Network graph of pollutants, biomolecular targets and health effects. Nodes are color labeled by type. Orange nodes indicate pollutants identified as being emitted by asphalt. Green nodes are proteins or genes that encode proteins that are affected by exposure to asphalt constituents. Dark blue nodes are diseases or health effects associated asphalt exposure. Light blue nodes are small molecule biomarkers, posttranslational modifications impacted by specific emissions from asphalt, and mitochondrial DNA that do not fall neatly into another group (Khare et al., 2020; Breuer et al., 2011; Burstyn et al., 2002; Väänänen et al., 2005; Loreto et al., 2007; Rapisarda et al., 2009; Hopf et al., 2018; Wey et al., 1992; Ma et al., 2003a; Ma et al., 2003b; Gate et al., 2006; Halter et al., 2007; Bowen et al., 2000; Wang et al., 2001; Schreiner, 2011; Keith, 2015; Ward-Caviness, 2019; Verma et al., 2012).
Asphalt emissions, biomolecular targets and associated health effects are shown in Figure 2. Nodes refer to pollutants, biomarkers of exposure (proteins and other biomolecules such as RNA), as well as diseases or health effects associated with specific emissions from asphalt. The figure was created using Cytoscape (Shannon et al., 2003), an open source tool used for visualizing networks, after extracting the underlying data and conclusions from a selection of literature review papers and IARC Monographs. The information was added to spreadsheets, based on categories such as “Chem to Asphalt,” “Pollutant to Illness,” “Pollutant to Molecule,” “Molecule to Symptom,” “Symptom to Disease,” and “Color Categories.” This allowed us to create the lines between the nodes and organize the information for data entry in a standardized way. Predominantly review articles were chosen for inclusion to capture a broad set of exposure effects related to asphalt. Not all papers had information that could be added to every sheet. For instance, papers detailing the constituents of asphalt did not include the proteins impacted by the exposures and vice versa. Papers referencing air pollution effects may not specifically indicate asphalt as the source of pollutant but included compounds that are also found in asphalt. Additionally, papers were included in the figure that were used as references in the dermal and inhalation exposure sections. Nodes were arranged manually to optimize legibility. The majority of nodes reflect contaminants, proteins and diseases. Biomolecules such as micro RNAs and mitochondrial DNA were grouped as “other.”
Of the 33 specific contaminants shown in this graph that are documented to be present in asphalt (Bowen et al., 2000; Burstyn et al., 2002; Väänänen et al., 2005; Breuer et al., 2011), only 22 have permissible exposure limits regulated by OSHA (Figure 3). Of those 22, 17 are regulated as a sum of exposure to PAHs, effectively reducing the thousands of constituents of asphalt to 17 measured representatives. This proves problematic for non-occupational exposures that are not regularly measured and motivates identifying biomarkers that may be able to augment medical care for persons with non-acute chronic exposures. Our network graph shows that when different studies are integrated through edges at different levels of granularity (e.g., asphalt vs. its constituent pollutants, and diseases vs. its associated biomolecular mechanisms) a level of connectivity is obtained that indicates mixtures need to be considered as a whole to estimate potentially adverse health effects.
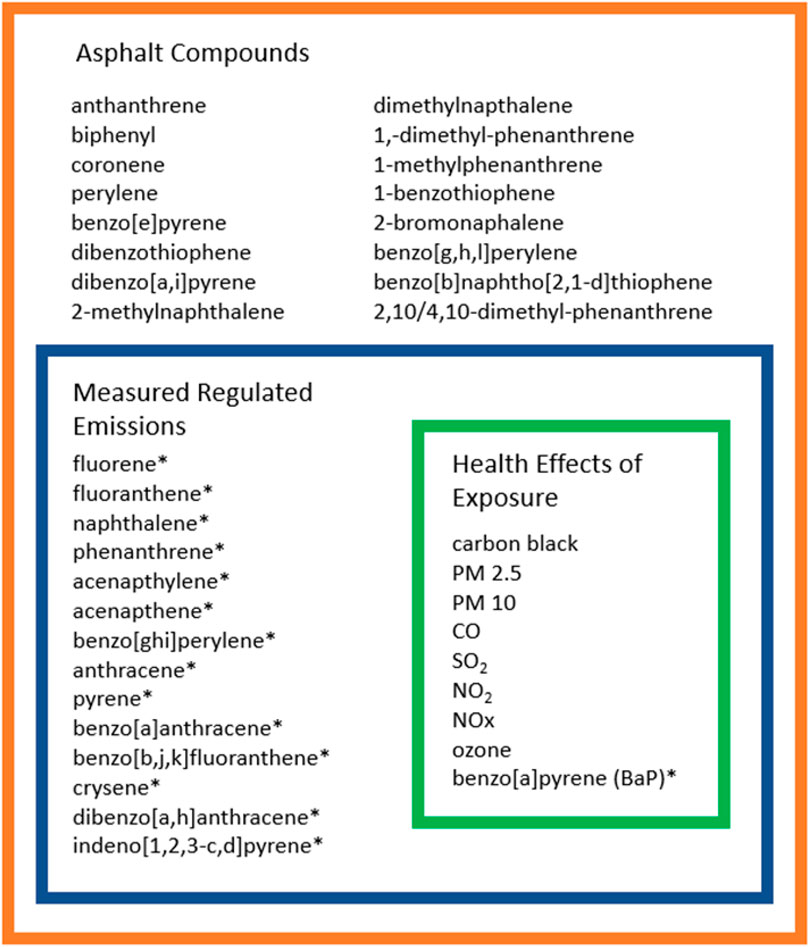
FIGURE 3. Venn diagram of asphalt compounds by regulation and health effects. The figure is compiled from the tables and information presented on the previous pages. The Health Effects of Exposure are the compounds associated with exposure effects. The Measured Regulated emissions box that encompasses the Health Effects of Exposure are compounds in asphalt that have regulated exposure limits. The orange Asphalt Compounds box contains the rest of the compounds that do not have exposure limit regulations as well as the compounds in the blue and green boxes. * = Compounds listed as part of the EPA list of 16 priority PAHs.
Figure 3 summarizes the information about the chemical compounds in asphalt as presented in this paper. The green box around Health Effects of Exposure encompasses the specific compounds that were presented in this review as having health effects beyond an associated cancer risk. The blue box encompassing Measured Regulated Emissions and the green Health Effects of Exposure box and contains those asphalt chemicals that have regulated exposure limits. The outer orange box contains all of the asphalt compounds specifically discussed in this report. The restatement highlights the research gap between the existing regulations, known health effects, and additional compounds in need of evaluation in the exposure environment.
8 Modulating emissions: Impacts of additives in bitumen
Understanding the composition of asphalt and deciphering the relative contribution of these constituents to adverse health effects also opens the way to target classes of compounds to limit their emission and/or make them less toxic. One way to limit the potential risks of exposure prior to a complete understanding of exposure effects is to reduce the emissions from asphalt (Figure 1A). As mentioned previously, the composition of asphalt is chemistry blind and focuses primarily on the mechanical properties of the product. Volatilization of asphalt hydrocarbons contributes to mass loss and aging of the material, leading to changes in bitumen’s colloidal structure as well as rheological and performance characteristics. This in turn makes asphalt stiffer and more prone to crack (Oldham et al., 2021). Economic factors have encouraged the recycling of asphalt products into new surfacing of roads (Kumpiene et al., 2021; Andersson-Sköld et al., 2007; Resource Responsible Use of Reclaimed Asphalt Pavement in Asphalt Mixtures [tech brief], 2021). The percentage of recycled asphalt material in the final product contributes brittleness to the final product and can cause premature cracking (Resource Responsible Use of Reclaimed Asphalt Pavement in Asphalt Mixtures [tech brief], 2021). Thus, the percentages of recycled material are based on the balance between economic cost of the new material incorporated and the mechanical performance of the resulting road (Kumpiene et al., 2021; Andersson-Sköld et al., 2007; Resource Responsible Use of Reclaimed Asphalt Pavement in Asphalt Mixtures [tech brief], 2021). Regardless, recycling of material increases the risks of occupational exposures to coal tar asphalts that are under the surface layers of many older roads (Andersson-Sköld et al., 2007; Kumpiene et al., 2021). Introduction of various additives to bitumen changes the bitumen composition which may alter its emission profile. Liquid additives with high content of volatile compounds can exacerbate the vapor emissions from bitumen. However, solid additives may not promote emission and even in some cases they may reduce total emission by retaining select volatile compounds (Mousavi et al., 2020; Mousavi et al., 2021). The addition of coal fly ash to recycled asphalt during the recycling and repaving process does not increase worker exposure to PAHs (Väänänen et al., 2005). While crumb rubber is considered a solid addition, a NIOSH report on the exposure differences for asphalt workers using conventional asphalt and crumb rubber modified (CRM) asphalt showed an increase in eye, nose and throat irritation during CRM asphalt application. Additionally, the measured benzene soluble particulate and polycyclic aromatic compounds in the personal breathing zone were higher during CRM asphalt application (Burr et al., 2001). Waste plastic and tall oil pitch (WPT) have also been used as fillers and subsequently studied for their health effects. Unlike CRM asphalt, exposure to PAHs and particulates were the same. However, in WPT application, exposure to aldehydes and resin acids was increased leading to increased reporting of eye and throat irritation in exposed workers (Väänänen et al., 2006).
As motor vehicles have increased in efficiency, heat and UV exposure effects on asphalt emissions have increased beyond the values for motor vehicle emissions (Khare et al., 2020). Therefore, there is an urgent need for methods to reduce loss of volatiles associated with asphalt surfaced areas (Mousavi and Fini, 2022). Having strict thresholds would motivate accurate measurements and incentivize innovation toward zero emission asphalt roads. Researchers looking to decrease the emissions from asphalt, or to change the mechanical properties of the material have mixed different additives into asphalt mixtures. Mousavi et al. (2021) added functionalized carbon to bitumen and found that the new mixture was able to adsorb specific chemicals known to be emitted from bitumen. In the same lab, a different research team identified that the addition of functionalized carbon to bitumen reduced the diffusion of hydrogen peroxide radicals that contributed to aging thus potentially reducing emissions (Ghaseme et al., 2022).
9 Conclusion
In this review we consider a link between aging in asphalt, the loss of volatile organic compounds in asphalt, environmental pollution, and human health at a systems level taking the complexity of both, individual constituents of asphalt and their effects on a multitude of biological targets into account. Reduction of emissions from asphalt surfaced area is well in-line with industry and road authorities’ visions. To achieve such goals, emission limits should be factored in design criteria alongside application and in-service performance criteria. In terms of asphalt emission, there is a need to study the trajectory and fate of selected toxic volatile compounds emitted from bitumen during production, placement, and service life of asphalt as relates to various production and application practices. In addition, there is a need for innovative approaches to minimize emission. In term of health impacts, there is a need to clearly demonstrate the positive health outcomes of making these modifications. Certainly, changes in the ways that asphalt is produced, reducing or eliminating coal tar, and improving workplace exposure controls have significantly decreased the long-term risks of health effects from asphalt products.
We described a new systems biology view of asphalt pollution akin to the field of host-pathogen interactions in protein-protein interactions networks. Similar to this task, there are two different types of nodes, host proteins vs. pathogen proteins, here host proteins vs. pollutant molecules. This analogy opens the doors to apply the tools of systems biology to this novel application area of pollutant-host response networks. For example, we have created a small gold standard set of interactions between pollutants and biomarkers of pollution from review of the current literature (depicted visually in Figure 2). This network can be expanded, for example by virtue of the fact that many of the biomarkers of exposure are proteins, and protein-protein interaction network data is widely available, allowing us to couple these existing networks to the new pollutant-biomarker network. The availability of a gold standard also opens the door to apply machine learning approaches to expanding the network. For example, we can define a new classification task, that of predicting the full network of all possible edges connecting pollutants to human biomarkers. These can be created as specific disease networks, similar to how protein-protein interaction networks have been created not only for all proteins in an organism such as human, but also for specific interaction subnetworks tied to a specific disease. Research is needed to identify what are the types of features that will be predictive for this new task. For example, transcriptomic changes upon stress, gene ontology and protein structural features may be very informative, alongside structural and physicochemical property descriptions of the pollutants and proteins.
We hope that this article has convinced the reader that translational systems biology provides a new option for identifying cumulative chronic exposure risks, and evaluation of new risks posed by novel modifications of asphalt, through an integrated view of asphalt constituents and biological targets. The outcomes of this study can contribute to the understanding of the impacts of asphalt emissions on public health encouraging incorporation of emission thresholds and consequences for health into the design of asphalt surfaced areas.
Author contributions
JK-S invented the proposed systems view of pollutants and health effects, devised the concept, wrote the paper; ER surveyed the literature and created the cytoscape graph and tables, wrote the paper; OT collected relevant publications; EF wrote the section on additives; NL reviewed literature, organized and wrote the paper.
Conflict of interest
The authors declare that the research was conducted in the absence of any commercial or financial relationships that could be construed as a potential conflict of interest.
The handling editor declared a past co-authorship with the author JK-S.
Publisher’s note
All claims expressed in this article are solely those of the authors and do not necessarily represent those of their affiliated organizations, or those of the publisher, the editors and the reviewers. Any product that may be evaluated in this article, or claim that may be made by its manufacturer, is not guaranteed or endorsed by the publisher.
Acknowledgments
We would like to thank the National Science Foundation for supporting I-BREATHE, award number 2124284, for enabling this collaboration.
Supplementary material
The Supplementary Material for this article can be found online at: https://www.frontiersin.org/articles/10.3389/fsysb.2022.928962/full#supplementary-material
References
Abboud, C. (2017). percivall Pott (1714-1788). Embryo Proj. Encycl. 24 (5), 287–292. doi:10.1038/sc.1986.40
Agostini, M., Fransman, W., De Vocht, F., Van Wendel De Joode, B., and Kromhout, H. (2011). Assessment of dermal exposure to bitumen condensate among road paving and mastic crews with an observational method. Ann. Occup. Hyg. 55 (6), 578–590. doi:10.1093/annhyg/mer026
Andersson, J. T., and Achten, C. (2015). Time to say goodbye to the 16 EPA PAHs? Toward an up-to-date use of PACs for environmental purposes. Polycycl. Aromat. Compd. 35 (2–4), 330–354. doi:10.1080/10406638.2014.991042
Andersson-Sköld, Y., Andersson, K., Lind, B., Claesson, A. N., Larsson, L., Suer, P., et al. (2007). Coal tar-containing asphalt Resource or hazardous waste? J. Ind. Ecol. 11 (4), 99–116. doi:10.1162/jiec.2007.1106
Balanced Mix Design (2021). Balanced mix design. Greenbelt, MD: National Asphalt Pavement Association. Available from: https://www.asphaltpavement.org/expertise/engineering/resources/bmd-resource-guide.
Bennert, T., Reinke, G., Mogawer, W., and Mooney, K. (2010). Assessment of workability and compactability of warm-mix asphalt. Transp. Res. Rec. J. Transp. Res. Board 2180 (1), 36–47. doi:10.3141/2180-05
Binet, S., Pfohl-Leszkowicz, A., Brandt, H., Lafontaine, M., and Castegnaro, M. (2002). Bitumen fumes: Review of work on the potential risk to workers and the present knowledge on its origin. Sci. Total Environ. 300, 37–49. doi:10.1016/s0048-9697(02)00279-6
Boffetta, P., Burstyn, I., Partanen, T., Kromhout, H., Svane, O., Langård, S., et al. (2003). Cancer mortality among European asphalt workers: An international epidemiological study. II. Exposure to bitumen fume and other agents. Am. J. Ind. Med. 43 (1), 28–39. doi:10.1002/ajim.10182
Booth, E. D., Brandt, H. C. A., Loose, R. W., and Watson, W. P. (1998). Correlation of 32 P-postlabelling-detection of DNA adducts in mouse skin in vivo with the polycyclic aromatic compound content and mutagenicity in Salmonella typhimurium of a range of oil products. Arch. Toxicol. 72 (8), 505–513. doi:10.1007/s002040050535
Bowen, C., de Groot, P., and Brandt, C. A. Health safety and the environment-aqueous leaching of PAC’s from bitumen. (2000) [cited 2022 Feb 8]. p. 754–761. Proc.0083uk.
Breuer, D., Hahn, J. U., Höber, D., Emmel, C., Musanke, U., Rühl, R., et al. (2011). Air sampling and determination of vapours and aerosols of bitumen and polycyclic aromatic hydrocarbons in the Human Bitumen Study. Arch. Toxicol. 85 (S1), 11–20. doi:10.1007/s00204-011-0678-1
Buckley, T. J., and Lioy, P. J. (1992). An examination of the time course from human dietary exposure to polycyclic aromatic hydrocarbons to urinary elimination of 1-hydroxypyrene. Occup. Environ. Med. 49 (2), 113–124. doi:10.1136/oem.49.2.113
Burr, G., Tepper, A., Feng, A., Olsen, L., and Miller, A. (2001). Crumb-rubber modified asphalt paving: Occupational exposures and acute health effects [internet]. (HETA 2001-0536-2864). Cincinnati, Ohio: U.S. Department of Health and Human Services, Public Health Service, Centers for Disease Control and Prevention, National Institute for Occupational Safety and Health. Available from: https://www.cdc.gov/niosh/hhe/reports/pdfs/2001-0536-2864.pdf.
Burstyn, I., Randem, B., Lien, J. E., Langard, S., and Kromhout, H. (2002). Bitumen, polycyclic aromatic hydrocarbons and vehicle exhaust: Exposure levels and controls among Norwegian asphalt workers. Ann. Occup. Hyg. 46 (1), 79–87. doi:10.1093/annhyg/mef023
Cavallari, J. M., Osborn, L. V., Snawder, J. E., Kriech, A. J., Olsen, L. D., Herrick, R. F., et al. (2011). Predictors of dermal exposures to polycyclic aromatic compounds among hot-mix asphalt paving workers. Ann. Occup. Hyg. 56 (2), 125–137. doi:10.1093/annhyg/mer108
Chowdhury, R., Shah, D., and Payal, A. (2017). Healthy worker effect phenomenon: Revisited with emphasis on statistical methods – a review. Indian J. Occup. Environ. Med. 21 (1), 2–8. doi:10.4103/ijoem.IJOEM_53_16
Clark, C. R., Burnett, D. M., Parker, C. M., Arp, E. W., Swanson, M. S., Minsavage, G. D., et al. (2011). Asphalt fume dermal carcinogenicity potential: I. Dermal carcinogenicity evaluation of asphalt (bitumen) fume condensates. Regul. Toxicol. Pharmacol. 61 (1), 9–16. doi:10.1016/j.yrtph.2011.04.003
Colorado Procedure - Laboratory (2020). Colorado procedure - laboratory 5120-20 standard method of test for determination of the asphalt binder content of bituminous mixtures by the ignition method [internet]. Denver Colorado: Colorado Department of Transportation. Available from: https://www.codot.gov/business/designsupport/materials-and-geotechnical/manuals/2020-laboratory-manual-of-test-procedures/cpls/cp-ls-5100/cpl-5120-20.
Darby, F. W., Willis, A. F., and Winchester, R. V. (1986). Occupational health hazards from road construction and sealing work. Ann. Occup. Hyg. 30 (4), 445–454. doi:10.1093/annhyg/30.4.445
Farshidi, F., Jones, D., Kumar, A., Green, P. G., and Harvey, J. T. (2011). Direct measurements of volatile and semivolatile organic compounds from hot- and warm-mix asphalt. Transp. Res. Rec. J. Transp. Res. Board 2207 (1), 1–10. doi:10.3141/2207-01
Freeman, J. J., Schreiner, C. A., Beazley, S., Burnett, D. M., Clark, C. R., Mahagaokar, S., et al. (2011). Asphalt fume dermal carcinogenicity potential: II. Initiation–promotion assay of type III built-up roofing asphalt. Regul. Toxicol. Pharmacol. 61 (1), 17–22. doi:10.1016/j.yrtph.2011.05.008
Fuhst, R., Creutzenberg, O., Ernst, H., Hansen, T., Pohlmann, G., Preiss, A., et al. (2007). 24 Months inhalation carcinogenicity study of bitumen fumes in wistar (Wu) rats. J. Occup. Environ. Hyg. 4 (1), 20–43. doi:10.1080/15459620701326257
Fustinoni, S., Campo, L., Cirla, P. E., Martinotti, I., Buratti, M., Longhi, O., et al. (2010). Dermal exposure to polycyclic aromatic hydrocarbons in asphalt workers. Occup. Environ. Med. 67 (7), 456–463. doi:10.1136/oem.2009.050344
Gate, L., Langlais, C., Micillino, J. C., Nunge, H., Bottin, M. C., Wrobel, R., et al. (2006). Bitumen fume-induced gene expression profile in rat lung. Toxicol. Appl. Pharmacol. 215 (1), 83–92. doi:10.1016/j.taap.2006.01.012
Ghaseme, H., Yazdani, A., Rajib, A., and Fini, E. H. (2022). Toward carbon-negative and emission-curbing roads to drive environmental health. ACS Sustain Chem. Eng.
Grahn, K., Gustavsson, P., Andersson, T., Lindén, A., Hemmingsson, T., Selander, J., et al. (2021). Occupational exposure to particles and increased risk of developing chronic obstructive pulmonary disease (COPD): A population-based cohort study in stockholm, Sweden. Environ. Res. 200, 111739. doi:10.1016/j.envres.2021.111739
Halter, R., Hansen, T., Seidel, A., Ziemann, C., and Borlak, J. (2007). Importance of DNA-adduct formation and gene expression profiling of disease candidate genes in rats exposed to bitumen fumes. J. Occup. Environ. Hyg. 4 (1), 44–64. doi:10.1080/15459620701337528
Hopf, N. B., Spring, P., Hirt-Burri, N., Jimenez, S., Sutter, B., Vernez, D., et al. (2018). Polycyclic aromatic hydrocarbons (PAHs) skin permeation rates change with simultaneous exposures to solar ultraviolet radiation (UV-S). Toxicol. Lett. 287, 122–130. doi:10.1016/j.toxlet.2018.01.024
Hueper, W. C., and Payne, W. W. (1960). Carcinogenic studies on petroleum asphalt, cooling oil, and coal tar. Arch. Pathol. 70, 372–384.
Hung, A., and Fini, E. H. (2020). Surface morphology and chemical mapping of UV-aged thin films of bitumen. ACS Sustain Chem. Eng. 8 (31), 11764–11771. doi:10.1021/acssuschemeng.0c03877
Hung, A. M., Kazembeyki, M., Hoover, C. G., and Fini, E. H. (2019). Evolution of morphological and nanomechanical properties of bitumen thin films as a result of compositional changes due to ultraviolet radiation. ACS Sustain Chem. Eng. 7 (21), 18005–18014. doi:10.1021/acssuschemeng.9b04846
Jongeneelen, F. J., Scheepers, P. T. J., Groenendijk, A., Aerts, L. A. G. J. M. V., Anzion, R. B. M., Bos, R. P., et al. (1988). Airborne concentrations, skin contamination, and urinary metabolite excretion of polycyclic aromatic hydrocarbons among paving workers exposed to coal tar derived road tars. Am. Ind. Hyg. Assoc. J. 49 (12), 600–607. doi:10.1080/15298668891380312
Keith, L. H. (2015). The source of U.S. EPA’s sixteen PAH priority pollutants. Polycycl. Aromat. Compd. 35 (2–4), 147–160. doi:10.1080/10406638.2014.892886
Khare, P., Machesky, J., Soto, R., He, M., Presto, A. A., and Gentner, D. R. (2020), Asphalt-related emissions are a major missing nontraditional source of secondary organic aerosol precursors. Sci. Adv. 6 (36), eabb9785. doi:10.1126/sciadv.abb9785
Kriech, A. J., Schreiner, C. A., Osborn, L. V., and Riley, A. J. (2018). Assessing cancer hazards of bitumen emissions – A case study for complex petroleum substances. Crit. Rev. Toxicol. 48 (2), 121–142. doi:10.1080/10408444.2017.1391170
Kshirsagar, M., Schleker, S., Carbonell, J., and Klein-Seetharaman, J. (2015). Techniques for transferring host-pathogen protein interactions knowledge to new tasks. Front. Microbiol. 6, 36. doi:10.3389/fmicb.2015.00036
Kumpiene, J., Larsson, M. O., Carabante, I., and Arp, H. P. H. (2021). Roads with underlying tar asphalt - spreading, bioavailability and toxicity of their polycyclic aromatic hydrocarbons. Environ. Pollut. 289, 117828. doi:10.1016/j.envpol.2021.117828
Lindberg, H. K., Väänänen, V., Järventaus, H., Suhonen, S., Nygren, J., Hämeilä, M., et al. (2008). Genotoxic effects of fumes from asphalt modified with waste plastic and tall oil pitch. Mutat. Res. Toxicol. Environ. Mutagen 653 (1–2), 82–90. doi:10.1016/j.mrgentox.2008.03.009
Loreto, C., Rapisarda, V., Carnazza, M. L., Musumeci, G., D’Agata, V., Valentino, M., et al. (2007). Bitumen products alter bax, bcl-2 and cytokeratin expression: An in vivo study of chronically exposed road pavers. J. Cutan. Pathol. 34 (9), 699–704. doi:10.1111/j.1600-0560.2006.00687.x
Lyu, L., Pei, J., Hu, D., Sun, G., and Fini, E. H. (2022). Bio-modified rubberized asphalt binder: A clean, sustainable approach to recycle rubber into construction. J. Clean. Prod. 345, 131151. doi:10.1016/j.jclepro.2022.131151
Ma, C., Wang, J., and Luo, J. (2003). Exposure to asphalt fumes activates activator protein-1 through the phosphatidylinositol 3-kinase/akt signaling pathway in mouse epidermal cells. J. Biol. Chem. 278 (45), 44265–44272. doi:10.1074/jbc.M309023200
Ma, J. Y. C., Rengasamy, A., Frazer, D., Barger, M. W., Hubbs, A. F., Battelli, L., et al. (2003). Inhalation exposure of rats to asphalt fumes generated at paving temperatures alters pulmonary xenobiotic metabolism pathways without lung injury. Environ. Health Perspect. 111 (9), 1215–1221. doi:10.1289/ehp.5740
McClean, M. D., Rinehart, R. D., Sapkota, A., Cavallari, J. M., and Herrick, R. F. (2007). Dermal exposure and urinary 1-hydroxypyrene among asphalt roofing workers. J. Occup. Environ. Hyg. 4 (1), 118–126. doi:10.1080/15459620701334756
Mckee, R., Stubblefield, W. A., Lewis, S. C., Scala, R. A., Simon, G. S., and DePass, L. R. (1986). Evaluation of the dermal carcinogenic potential of tar sands bitumen-derived liquids. Fundam. Appl. Toxicol. 7 (2), 228–235. doi:10.1016/0272-0590(86)90152-1
Merlo, F., Andreassen, A., Weston, A., Pan, C. F., Haugen, A., Valerio, F., et al. (1998). Urinary excretion of 1-hydroxypyrene as a marker for exposure to urban air levels of polycyclic aromatic hydrocarbons. Cancer Epidemiol. BiomarkersPrevention 7, 147–155.
Mousavi, M., and Fini, E. H. (2022). Preventing emissions of hazardous organic compounds from bituminous composites. J. Clean. Prod. 344, 131067. doi:10.1016/j.jclepro.2022.131067
Mousavi, M., Hung, A., and Fini, E. H. (2020). Multifunctional zeolite nanorods as wax carriers and acid scavengers in asphalt binder. Constr. Build. Mater 264, 120249. doi:10.1016/j.conbuildmat.2020.120249
Mousavi, M., Martis, V., and Fini, E. H. (2021). Inherently functionalized carbon from algae to adsorb precursors of secondary organic aerosols in noncombustion sources. ACS Sustain Chem. Eng. 9 (43), 14375–14384. doi:10.1021/acssuschemeng.1c03827
Mundt, K. A., Dell, L. D., Crawford, L., Sax, S. N., and Boffetta, P. (2018). Cancer risk associated with exposure to bitumen and bitumen fumes: An updated systematic review and meta-analysis. J. Occup. Environ. Med. 60 (1), e6–e54. doi:10.1097/JOM.0000000000001202
New Report Provides Guidelines on Constructing Superpave Pavements [Internet] (1998). FOCUS: Using products of the Strategic Highway Research Program to build better, safer roads. Available from https://library.unt.edu/gpo/OTA/pubs/focus/fcs398/pavemnts.htm.
NIOSH Manual of Analytical Methods (NMAMTM) [Internet] (1994). Publication 94-113. DHHS (NIOSH). 4th ed. Available from: https://www.cdc.gov/niosh/docs/2003-154/default.html.
Occupational Chemical Database [Internet] (xxxx). United States department of labor. Available from: https://www.osha.gov/chemicaldatabase (Accessed 2022).
Oldham, D. J., Obando, C. J., Mousavi, M., Kaloush, K. E., and Fini, E. H. (2021). Introducing the critical aging point (CAP) of asphalt based on its restoration capacity. Constr. Build. Mater 278, 122379. doi:10.1016/j.conbuildmat.2021.122379
Phillips, D. H., and Arlt, V. M. Genotoxicity: Damage to DNA and its consequences. In: A. Luch, editor. Molecular, clinical and environmental toxicology [internet]. Basel: Birkhäuser Basel; 2009 [cited 2022 Apr 24]. p. 87–110. (Experientia Supplementum;vol. 99). Available from: http://link.springer.com/10.1007/978-3-7643-8336-7_4.
Poon, R., Chu, I., Davis, H., Yagminas, A. P., and Valli, V. E. (1996). Systemic toxicity of a bitumen upgrading product in the rat following subchronic dermal exposure. Toxicology 109 (2–3), 129–146. doi:10.1016/0300-483x(96)03326-4
Poon, R., Chu, I., Villeneuve, D. C., and Valli, V. E. (1994). Short-term toxicity of bitumen upgrading products in the rat following repeated dermal exposure. Fundam. Appl. Toxicol. 23 (2), 237–250. doi:10.1006/faat.1994.1102
Pott, P. (1775). Chirurgical observations relative to the cataract, the polypus of the nose, the cancer of the scrotum, the different kinds of ruptures, and the mortification of the toes and feet. London: T.J. Carnegy.
Programme international sur la sécurité (2004), Programme international sur la sécurité des substances chimiques. Concise international chemical assessment document. Asphalt (bitumen). Geneva: World health organization.
Qi, Y., Bar-Joseph, Z., and Klein-Seetharaman, J. (2006). Evaluation of different biological data and computational classification methods for use in protein interaction prediction. Proteins Struct. Funct. Bioinforma. 63 (3), 490–500. doi:10.1002/prot.20865
Rajib, A., and Fini, E. H. (2020). Inherently functionalized carbon from lipid and protein-rich biomass to reduce ultraviolet-induced damages in bituminous materials. ACS Omega 5 (39), 25273–25280. doi:10.1021/acsomega.0c03514
Randem, B. G., Langård, S., Dale, I., Kongerud, J., Martinsen, J. I., and Andersen, A. (2003). Cancer incidence among male Norwegian asphalt workers: Male Norwegian Asphalt Workers. Am. J. Ind. Med. 43 (1), 88–95. doi:10.1002/ajim.10169
Randem, B. G., Ulvestad, B., Burstyn, I., and Kongerud, J. (2004). Respiratory symptoms and airflow limitation in asphalt workers. Occup. Environ. Med. 61 (4), 367–369. doi:10.1136/oem.2002.006114
Rapisarda, V., Carnazza, M. L., Caltabiano, C., Loreto, C., Musumeci, G., Valentino, M., et al. (2009). Bitumen products induce skin cell apoptosis in chronically exposed road pavers. J. Cutan. Pathol. 36 (7), 781–787. doi:10.1111/j.1600-0560.2008.01140.x
Rathore, M., Haritonovs, V., and Zaumanis, M. (2021). Performance evaluation of warm asphalt mixtures containing chemical additive and effect of incorporating high reclaimed asphalt content. Materials 14 (14), 3793. doi:10.3390/ma14143793
Raulf-Heimsoth, M., Marczynski, B., Spickenheuer, A., Pesch, B., Welge, P., Rühl, R., et al. (2011). Bitumen workers handling mastic versus rolled asphalt in a tunnel: Assessment of exposure and biomarkers of irritation and genotoxicity. Arch. Toxicol. 85 (S1), 81–87. doi:10.1007/s00204-011-0685-2
Raulf-Heimsoth, M., Pesch, B., Kendzia, B., Spickenheuer, A., Bramer, R., Marczynski, B., et al. (2011). Irritative effects of vapours and aerosols of bitumen on the airways assessed by non-invasive methods. Arch. Toxicol. 85 (S1), 41–52. doi:10.1007/s00204-011-0681-6
Raulf-Heimsoth, M., Pesch, B., Schott, K., Kappler, M., Preuss, R., Marczynski, B., et al. (2007). Irritative effects of fumes and aerosols of bitumen on the airways: Results of a cross-shift study. Arch. Toxicol. 81 (1), 35–44. doi:10.1007/s00204-006-0115-z
Resource Responsible Use of Reclaimed Asphalt Pavement in Asphalt Mixtures [tech brief] (2021). Resource responsible use of reclaimed asphalt pavement in asphalt mixtures [tech brief] [internet]. Sep. Report No.: FHWA-HIF-22-003. Available from: https://rosap.ntl.bts.gov/view/dot/59939.
Rhomberg, L. R., Mayfield, D. B., Prueitt, R. L., and Rice, J. W. (2018). A bounding quantitative cancer risk assessment for occupational exposures to asphalt emissions during road paving operations. Crit. Rev. Toxicol. 48 (9), 713–737. doi:10.1080/10408444.2018.1528208
Riala, R., Heikkilä, P., and Kanerva, L. (1998). A questionnaire study of road pavers’ and roofers’ work related skin symptoms and bitumen exposure. Int. J. Dermatol 37, 27–30. doi:10.1046/j.1365-4362.1998.00226.x
Roy, T. A., Kriech, A. J., and Mackerer, C. R. (2007). Percutaneous absorption of polycyclic aromatic compounds from bitumen fume condensate. J. Occup. Environ. Hyg. 4 (1), 137–143. doi:10.1080/15459620701334814
Rühl, R., Musanke, U., Kolmsee, K., Priess, R., Zoubek, G., and Breuer, D. (2006). Vapours and aerosols of bitumen: Exposure data obtained by the German bitumen forum. Ann. Occup. Hyg. 50 (5), 459–468. doi:10.1093/annhyg/mel001
Samburova, V., Zielinska, B., and Khlystov, A. (2017). Do 16 polycyclic aromatic hydrocarbons represent PAH air toxicity? Toxics 5 (3), 17. doi:10.3390/toxics5030017
Schreiner, C. A. (2011). Review of mechanistic studies relevant to the potential carcinogenicity of asphalts. Regul. Toxicol. Pharmacol. 59 (2), 270–284. doi:10.1016/j.yrtph.2010.10.010
Secondo, L. E., Avrutin, V., Ozgur, U., Topsakal, E., and Lewinski, N. A. (2022). Real-time monitoring of cellular oxidative stress during aerosol sampling: A proof of concept study. Drug Chem. Toxicol. 45 (2), 767–774. doi:10.1080/01480545.2020.1774774
Secondo, L. E., Wygal, N. J., and Lewinski, N. A. (2019). A new portable in vitro exposure cassette for aerosol sampling. J. Vis. Exp. (144), 58916. doi:10.3791/58916
Shannon, P., Markiel, A., Ozier, O., Baliga, N. S., Wang, J. T., Ramage, D., et al. (2003). Cytoscape: A software environment for integrated models of biomolecular interaction networks. Genome Res. 13 (11), 2498–2504. doi:10.1101/gr.1239303
Sivak, A., Niemeier, R., Lynch, D., Beltis, K., Simon, S., Salomon, R., et al. (1997). Skin carcinogenicity of condensed asphalt roofing fumes and their fractions following dermal application to mice. Cancer Lett. 117 (1), 113–123. doi:10.1016/s0304-3835(97)00214-0
Sobus, J. R., McClean, M. D., Herrick, R. F., Waidyanatha, S., Nylander-French, L. A., Kupper, L. L., et al. (2009). Comparing urinary biomarkers of airborne and dermal exposure to polycyclic aromatic compounds in asphalt-exposed workers. Ann. Occup. Hyg. 53 (6), 561–571. doi:10.1093/annhyg/mep042
Sobus, J. R., McClean, M. D., Herrick, R. F., Waidyanatha, S., Onyemauwa, F., Kupper, L. L., et al. (2009). Investigation of PAH biomarkers in the urine of workers exposed to hot asphalt. Ann. Occup. Hyg. 53 (6), 551–560. doi:10.1093/annhyg/mep041
Tastan, O., Qi, Y., Carbonell, J. G., and Klein-Seetharaman, J. (2009). Prediction of interactions between HIV-1 and human proteins by information integration. Pac Symp. Biocomput Pac Symp. Biocomput, 516–527. doi:10.1142/9789812836939_0049
Tepper, A. L., Burr, G. A., Feng, H. A., Singal, M., Miller, A. K., Hanley, K. W., et al. (2006). Acute symptoms associated with asphalt fume exposure among road pavers. Am. J. Ind. Med. 49 (9), 728–739. doi:10.1002/ajim.20346
Tolos, W. P., Shaw, P. B., Lowry, L. K., MacKenzie, B. A., Deng, J. F., and Markel, H. L. (1990). 1-Pyrenol: A biomarker for occupational exposure to polycyclic aromatic hydrocarbons. Appl. Occup. Environ. Hyg. 5 (5), 303–309. doi:10.1080/1047322x.1990.10389643
Väänänen, V., Elovaara, E., Nykyri, E., Santonen, T., and Heikkilä, P. (2006). Road pavers’ occupational exposure to asphalt containing waste plastic and tall oil pitch. J. Env. Monit. 8 (1), 89–99. doi:10.1039/b513505b
Väänänen, V., Hämeilä, M., Kalliokoski, P., Nykyri, E., and Heikkilä, P. (2005). Dermal exposure to polycyclic aromatic hydrocarbons among road pavers. Ann. Occup. Hyg. 49 (2), 167–178. doi:10.1093/annhyg/meh094
Verma, N., Pink, M., Rettenmeier, A. W., and Schmitz-Spanke, S. (2012). Review on proteomic analyses of benzo[a]pyrene toxicity. Proteomics 12 (11), 1731–1755. doi:10.1002/pmic.201100466
Wang, J., Lewis, D. M., Castranova, V., Frazer, D. G., Goldsmith, T., Tomblyn, S., et al. (2001). Characterization of asphalt fume composition under simulated road paving conditions by GC/MS and microflow LC/quadrupole time-of-flight MS. Anal. Chem. 73 (15), 3691–3700. doi:10.1021/ac010334m
Ward-Caviness, C. K. (2019). A review of gene-by-air pollution interactions for cardiovascular disease, risk factors, and biomarkers. Hum. Genet. 138 (6), 547–561. doi:10.1007/s00439-019-02004-w
Wey, H. E., Breitenstein, M. J., and Toraason, M. A. (1992). Inhibition of intercellular communication in human keratinocytes by fractionated asphalt fume condensates. Carcinogenesis 13 (6), 1047–1050. doi:10.1093/carcin/13.6.1047
World Health Organization WHO : International Agency for Research on Cancer IARC (1973). Certain polycyclic aromatic hydrocarbons and heterocyclic compounds. Lyon: IARC.
Zanobetti, A., Baccarelli, A., and Schwartz, J. (2011). Gene–air pollution interaction and cardiovascular disease: A review. Prog. Cardiovasc Dis. 53 (5), 344–352. doi:10.1016/j.pcad.2011.01.001
Keywords: biomarkers of pollutants, exposure routes, review, network analysis, bitumen
Citation: Rozewski E, Taqi O, Fini EH, Lewinski NA and Klein-Seetharaman J (2023) Systems biology of asphalt pollutants and their human molecular targets. Front. Syst. Biol. 2:928962. doi: 10.3389/fsysb.2022.928962
Received: 26 April 2022; Accepted: 19 December 2022;
Published: 10 January 2023.
Edited by:
Naveena Yanamala, The State University of New Jersey, United StatesReviewed by:
Viktors Haritonovs, Riga Technical University, LatviaAugusto Cannone Falchetto, Aalto University, Finland
Copyright © 2023 Rozewski, Taqi, Fini, Lewinski and Klein-Seetharaman. This is an open-access article distributed under the terms of the Creative Commons Attribution License (CC BY). The use, distribution or reproduction in other forums is permitted, provided the original author(s) and the copyright owner(s) are credited and that the original publication in this journal is cited, in accordance with accepted academic practice. No use, distribution or reproduction is permitted which does not comply with these terms.
*Correspondence: Judith Klein-Seetharaman, amtsZWluc2VAYXN1LmVkdQ==