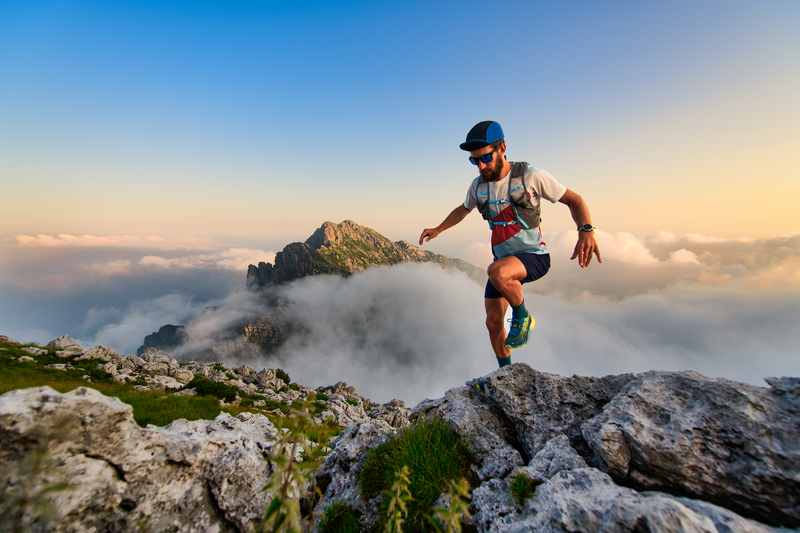
95% of researchers rate our articles as excellent or good
Learn more about the work of our research integrity team to safeguard the quality of each article we publish.
Find out more
REVIEW article
Front. Surg. , 07 March 2024
Sec. Neurosurgery
Volume 11 - 2024 | https://doi.org/10.3389/fsurg.2024.1298709
This article is part of the Research Topic Applications of Fluorescence in Surgery and Diagnostics Volume II: Evolution and Breakthroughs View all 6 articles
Surgical resection is considered for most brain tumors to obtain tissue diagnosis and to eradicate or debulk the tumor. Glioma, the most common primary malignant brain tumor, generally has a poor prognosis despite the multidisciplinary treatments with radical resection and chemoradiotherapy. Surgical resection of glioma is often complicated by the obscure border between the tumor and the adjacent brain tissues and by the tumor's infiltration into the eloquent brain. 5-aminolevulinic acid is frequently used for tumor visualization, as it exhibits high fluorescence in high-grade glioma. Here, we provide an overview of the fluorescent probes currently used for brain tumors, as well as those under development for other cancers, including HMRG-based probes, 2MeSiR-based probes, and other aminopeptidase probes. We describe our recently developed HMRG-based probes in brain tumors, such as PR-HMRG, combined with the existing diagnosis approach. These probes are remarkably effective for cancer cell recognition. Thus, they can be potentially integrated into surgical treatment for intraoperative detection of cancers.
Surgical resection is the primary treatment for brain tumors, complemented by radiotherapy or chemotherapy for malignant types (1–3). Total resection is often challenging, especially for gliomas, due to their infiltrative nature and difficulty in distinguishing from surrounding tissues (4–7). Fluorescence imaging, rapidly adopted in neurosurgery, addresses these challenges. It offers low-cost, high-resolution visualization of tumors, clearly differentiating them from adjacent brain tissue and aiding in the identification of ill-defined boundaries. This method is particularly crucial in reducing residual tumors and the associated risk of regrowth or relapse (8–11).
Panel diagnostics using next-generation sequencing have advanced the identification of oncogenes in solid cancers, such as lung and breast cancers, paving the way for precision medicine (12, 13). While these omics analyses provide comprehensive insights, they lack spatiotemporal data after cell homogenization. In contrast, fluorescence imaging in surgery offers a non-invasive, real-time, and high-resolution method for observing and quantitatively analyzing biomolecules within tissues (8, 9).
Fluorescent probes can be broadly categorized based on their features (14). The “always on” probes continuously exhibit fluorescence, whereas “activatable” probes only become fluorescent upon interaction with a specific target. Today, “always on” probes such as indocyanine green (15) and fluorescein sodium are used in the neurosurgical field (9, 16). However, these probes are not always accumulating in tumor tissues and have tendency to emit high background from the effect of “always on” (9). On the other hand, 5-aminolevulinic acid (5-ALA) serves as an “activatable” probe, distinguishing the tumor from the non-tumor tissues based on metabolic activity variations (8).
This review highlights advancements in fluorescent aminopeptidase probes, especially Hydroxymethyl Rhodamine Green (HMRG), 2-Methyl silicon rhodamine (2MeSiR), and 2-O-Methyl silicon rhodamine (2OMeSiR). These probes are expected to enhance surgical precision and rapid cancer detection. Their interaction with tumor enzymes allows accurate differentiation between tumor and surrounding tissues. We will explore their benefits and applications in fluorescence-guided surgery.
Miura et al. reported a rational design principle, Photoinduced Electron Transfer, for modulating the fluorescence properties of probes using fluorescein (17). Building upon this theory, Urano et al. synthesized probes with 2,6-dicarboxyethyl-1,3,5,7-tetramethyl boron-dipyrromethene (BODIPY) as a fluorescent scaffold, enabling the detection of acidic pH changes in vitro with HER2-positive cells. Significantly, these probes possessed the added advantage of reversibility, making them suitable for in vivo applications in lung cancer detection (18). To address the slower reaction rate of endo-peptidase detectable probes that hydrolyze non-terminal peptides, we employed intramolecular spirocyclization (19). This concept facilitated rapid responsiveness and precise molecular design, targeting the amino-terminal or carboxyl-terminal ends (19–21). Furthermore, Kuriki et al. established HMRG-based probe libraries that potentially targeted different enzymes achieved by substituting the acetyl group of Ac-HMRG with various amino acids (22) (Figure 1).
Figure 1. Chemical structure and reaction principle of HMRG probe. When dipeptidyl-HMRG reacts with the enzyme in tumor, it becomes HMRG and gets fluorescent. HMRG distinguishes tumor from the non-tumor tissue as an “activatable” probe.
Urano et al. applied gGlu-HMRG to human ovarian cancer cell lines (SHIN-3) and normal human umbilical vein endothelial cells, observing high fluorescence intensity and γ-glutamyltransferase (GGT) activity exclusively in SHIN-3 cells (23). gGlu-HMRG, reacting with GGT expressed on tumor cells, produces a highly fluorescent reaction product (24, 25). In experiments with mice bearing SHIN-3 tumors, intraperitoneal injection of gGlu-HMRG led to distinct high fluorescence in tumor areas, visible to the naked eye, while normal mice without tumors showed no such fluorescence and had low GGT background activity. This fluorescence was also confirmed in vitro with many human ovarian cancer cell lines tested. Spraying gGlu-HMRG on the peritoneal surface of mice injected with six of these cell types resulted in strong fluorescence in four types. In further studies, SHIN-3 cells transfected with red fluorescent protein (RFP) and injected into mice revealed complete overlapping fluorescence with gGlu-HMRG 10 min post-injection. Notably, the detection of SHIN3-RFP cells using gGlu-HMRG showed 100% sensitivity and specificity (23).
Dipeptidyl peptidase IV (DPP-IV), a ubiquitous enzyme found in the kidney, liver, intestine, and other organs, is implicated in various cancers including prostate adenocarcinoma, thyroid cancer, and esophageal cancer (26–29). It can cleave EP-HMRG, resulting in fluorescence emission (30).
Onoyama et al. developed peptidase probes for esophageal squamous cell cancer (30). Screening was performed using a series of HMRG-based aminopeptidase-activatable fluorescence probes such as γ-glutamyltranspeptidase, DPP-IV, fibroblast activation protein (AcGP-HMRG), cathepsin H (Arg-HMRG), against fresh biopsy samples. They discovered that glycine-prolyl-HMRG (GP-HMRG), targeting DPP-IV, exhibited a rapid, substantial, and specific increase in fluorescence in tumor cells. After assessing DPP-IV expression levels and enzymatic activity, they synthesized HMRG probes with various N-terminal amino acids, such as Glutamic acid, Lysine, Tyrosine, Leucine, and Proline, to determine their affinity. The EP-HMRG probe, showing the highest affinity and lowest Michaelis constant (Km) for DPP-IV, was selected. Validation of EP-HMRG involved measuring the increase in fluorescence intensity in tumor and normal samples over time, revealing a significant increase in tumor samples.
Recently, we published our research on the development of fluorescent probes designed for glioblastoma, relying on enzymatic activity (31). We initially screened 320 fluorescent probes using homogenized tumor lysates from patients, selecting the top 10% of promising probes based on their ability to differentiate between glioblastoma and surrounded tissues. We further narrowed down these candidates in a secondary screening with fresh surgical specimens, identifying the top three probes that demonstrated the highest differential fluorescence intensities for glioblastoma detection. These results were comprehensively analyzed, and a tertiary screening involved computational, mathematical, and pathological analysis. The proline-arginine-HMRG (PR-HMRG) probe showed the highest reactivity with 79.4% accuracy for detecting glioblastoma. We also attempted to identify the enzyme-cleaving PR-HMRG using Diced Electrophoresis Gel (DEG) assay, followed by Liquid Chromatography/Tandem Mass Spectrometry (LC/MS) (32). Through LC/MS, we identified four potential enzymes, with calpain 1 (CAPN1) as the responsible one, confirmed by enzyme inhibition experiments and CAPN1 RNA expression analysis. In U87 glioblastoma cells, CAPN1 knockdown reduced PR-HMRG fluorescence (31). In a U87 orthotopic xenograft model, PR-HMRG displayed higher fluorescence in tumor areas, consistent with CAPN1 expression. Human surgical specimens also showed elevated CAPN1 expression by both immunohistochemistry and western blotting, indicating the potential of this probe for glioblastoma detection during the surgery in the future (31). PR-HMRG probe showed early fluorescence onset within 5 min of application (31) (Figure 2).
Figure 2. Fluorescence-guided resection using 5-ALA and PR-HMRG in glioma surgery. (A) Corticotomy site visualized under white light. (B) Visualization of the tumor core and margins using 5-ALA-induced fluorescence at an emission wavelength of approximately 630 nm. (C) Post-resection view showing no residual fluorescence, suggesting complete removal of the tumor. (D) Schematic representation of PR-HMRG probe activation, depicting the transition from a colorless state to fluorescence upon enzymic reaction with the tumor.
Kuriki et al. found promising probes lysine-histidine-HMRG (KH-HMRG) for gastric cancer (negative staining) and lysine-lysine-HMRG (KK-HMRG) for lung cancer from among 380 types of HMRG-based fluorescent probes, which detect tumor tissues with high expression of puromycin-sensitive aminopeptidase (PSA) and aminopeptidase N (APN), respectively (22).
Takahashi et al. selected the promising fluorescent probe GP-HMRG for pancreatic cancer from our probe library (33). Dipeptidyl peptidase, or DPP-IV-like enzyme, was identified as the target enzyme.
Ac-lysine-glutamine-leucine-arginine-HMRG (Ac-KQLR-HMRG) is a fluorescent probe for visualizing prostate cancer. Yogo et al. synthesized Ac-KQLR-HMRG, which is activated by hepsin and matriptase. This probe showed specific fluorescence of various prostate cancer cell lines in vitro (34).
Yamamoto et al. synthesized an avidin-conjugated fluorescent probe, the Avidin-Leu-HMRG (35). Avidin is a protein which has a high affinity for lectin on cancer cells. In a mouse model of peritoneal ovarian metastasis, this probe demonstrated high fluorescence intensity at tumor locations, attributable to the fluorescence activity of lysosomal leucine aminopeptidase.
HMRG-based fluorescent probes may be useful for various diseases other than cancers. Yamashita et al. evaluated the fluorescence intensity in pancreatic juice and intestinal juice discharged after the pancreatic ventricle or central pancreatectomy using glutamyl-phenylalanine-HMRG (gPhe-HMRG) (36). They showed that it is possible to measure protease (chymotrypsin) activity in drained pancreatic fluid samples.
Challenges with green HMRG probes include interference from tissue autofluorescence and attenuation related to blood absorption (37). To circumvent these limitations, researchers have identified alternative scaffolds that emit at longer wavelengths. Kushida et al. demonstrated that 2MeSiR600, a red fluorescent scaffold, could be used to design activatable probes targeting proteases, although it exhibited high background fluorescence due to its relatively high fluorescence quantum yield (38). Addressing this, Ogasawara et al. modified 2MeSiR600 to reduce background signals and synthesized 2OMeSiR600 probes for aminopeptidase activity detection, controlled by photoinduced electron transfer (39).
Takahashi et al. developed a fluorescence imaging technique to identify the extrahepatic biliary tree (eCCA) using an enzyme-activated probe for diagnostic imaging of liver cancer (40). They selected the most specific probe for eCCA from 384 HMRG-based fluorescent probes, 400 2MeSiR-based probes, and 16 types of Hydroxymethyl N-(2,2,2-trifluoroethyl) rhodol (HMRef) -based fluorescence probes for glycosidases that they had synthesized (41). PM-2MeSiR emerged as the most specific fluorophore for eCCA, targeting a puromycin-sensitive aminopeptidase (40).
QA-2MeSiR and QA-2OMeSiR are probes developed for detecting tumors in lung cancer. Kawashima et al. screened these probes, selecting those with the highest fluorescence intensity for lung cancer (42). They found QA-2OMeSiR to have a lower background than QA-2MeSiR, targeting enzymes like DPP-IV and PSA (42).
Table 1 shows a summary of the HMRG-based and 2MeSiR-based fluorescent probes.
Leucine aminopeptidase (LAP) is an enzyme that cleaves a type of amino acid from the end of a peptide. It has been confirmed that LAP's blood concentration increases due to bile stagnation, and LAP is present in various cancer cells (43). Gong Q et al. developed the fluorescent probe of the incorporating L-leucine into the skeleton of cresyl violet as a recognition moiety using confocal fluorescence imaging (44). They analyzed changes in LAP concentration using human liver cancer-derived HepG2 and human lung cancer-derived A549 cells under cisplatin treatment. A higher concentration increase of LAP was found in HepG2 cells. Inhibitor experiments of LAP expression with siRNA further reduced cell viability. This result indicated that LAP was highly resistant to cisplatin. LAP is known to be involved in detoxifying cisplatin in hepatoma cells and contributes to inherent drug resistance (44). He X et al. developed a specific and sensitive near-infrared fluorescent probe (HCAC) for in vivo imaging of LAP activity in liver disease models. HCAC showed acetaminophen-induced liver injury and upregulation of LAP in tumor mouse models (45).
Pyroglutamate aminopeptidase-1 (PGP-1) enzyme plays an important role in inflammation involving immune cells, blood vessels, and molecular mediators (46). Cao et al. designed a red-emitting ratiometric fluorescence sensor (DP-1) that specifically detects PGP-1. They showed that PGP-1 expression was associated with inflammation using human liver cancer-derived HepG2 and mouse macrophage-like cell line RAW264 cells by imaging of the DP-1. Furthermore, imaging of mouse tumor models has shown that PGP-1 is closely associated with some inflammation and tumor disease (46).
Prolyl aminopeptidases (PAP) are often present in infectious disease bacteria, which is a potential biomarker and therapeutic target for pathogen infection (47). Liu X. et al. developed a near-infrared fluorescent “turn-on” probe (NIR-PAP) for detecting and imaging the activity of PAP in vivo. They indicated that this probe exhibited high specificity and reactivity to PAP under physiological pH and temperature conditions in vitro (47).
APN is expressed in ovarian carcinoma cells and is an important biomarker for cancers such as osteosarcoma and hematopoietic tumors (48–50). NIR fluorescent probes have been developed for detecting APN activity. He X et al. have developed an NIP fluorescent probe detecting APN (51). Using confocal microscopy, they showed that hepatoma cells had higher APN content than normal cells. Additionally, APNs were imaged in cells and mice in vivo. CD3/aminopeptidase N is an ectoenzyme with multiple functions, including tumor growth, migration, angiogenesis, and metastasis. LiH et al. have developed the first two-photon NIR fluorescent probe for in vitro and in vivo tracking of APN (52). Hydrolysis of the amino group of the N-terminal alanyl moiety restored the intramolecular charge transfer effect, resulting in strong fluorescence. In addition, the probe DCM-APN distinguished normal cells (LD2 cells) from cancer cells (human liver cancer-derived HepG-2 and malignant melanoma B16/BL6 cells).
The standard treatment for most brain tumors is surgical resection under a microscope, often accompanied by adjuvant radiotherapy and/or chemotherapy for malignant types (1–3). Maximal resection is attempted for prolonged tumor control and improved patient survival in most cases, except for certain tumors like malignant lymphoma and germinoma, which are sensitive to either radiotherapy or chemotherapy (53, 54). The utilization of fluorescent probes in surgical procedures offers a significant advantage by enabling surgeons to accurately differentiate tumor from normal or surrounding tissues in real-time (55–57). This enhanced visualization, provided by the fluorescence of these probes, leads to increased resection rates—a critical factor in surgical success. Importantly, achieving a higher extent of resection, especially Gross Total Resection (GTR), has been independently associated with improved progression-free survival (PFS) and overall survival (OS) in patients with high-grade and supratentorial low-grade gliomas. Therefore, by facilitating more precise and extensive tumor resections, fluorescent probes have the potential to further improve PFS and OS outcomes in brain tumor patients.
The development of aminopeptidase probes, particularly HMRG-based and 2MeSiR-based probes, presents promising advancements in cancer detection and monitoring as biomarkers. These probes offer unique advantages, such as rapid activation and reduced background signals. The HMRG probes react quickly, yielding results within minutes, a benefit already confirmed in esophageal cancer and brain tumor studies (30, 31). In their studies, probes that target enzymes like g-glu, DPP4, CAPN1, LAP, PGP-1, and APN hold significant promise for detecting a variety of diseases, encompassing both cancer and infections (58, 59). Present, three fluorescent agents that have been studied and utilized widely in human neurosurgical fields are fluorescein sodium, ICG, and 5-ALA (8, 9).
ICG is a water-soluble molecule that is excited at a wavelength of approximately 780 nm and emits fluorescent within the 700–850 nm range, making it detectable only with a filtered scope. ICG is observed a few seconds after 0.2–0.5 mg/kg IV administration, reaching its peak around 10 min (15, 60, 61). ICG is widely used to confirm blood flow and patency in vascular surgeries for aneurysms, AVMs, and anastomoses. It can also be beneficial in assessing the circulatory status when tumors compress or infiltrate cerebral circulation (62, 63). The Second Window ICG technique uses tumors' vascular permeability and poor clearance. Delivering substantial quantities of ICG allows neurosurgeons to locate tumors during surgery. However, it takes 19–30 h to visualize and does not accumulate in a tumor-specific manner (64, 65).
Fluorescein demonstrates fluorescence, peaking at around 530 nm when excited at approximately 480 nm with high detection rate for glioma in multicentric prospective phase II trial (66). For lower concentrations, observation through a 560 nm filter is typically required to detect its fluorescence (67). Notably, at higher doses, specifically 20 mg/kg, fluorescein's fluorescence becomes visible to the naked eye (68). The technique of confocal endoscopy and endomicroscopy, which has been employed with fluorescein, is notable for its application in brain tumor imaging (69, 70). ICG and fluorescein does not selectively activate fluorescence in malignant glioma cells. Instead, it tends to concentrate in areas where the blood-brain barrier is disrupted, a common characteristic of tumor sites (67, 71).
5-ALA can be used to visually distinguish tumor tissues from normal ones (72, 73). 5-ALA transforms into protoporphyrin IX (PpIX), which is a photosensitizer and precursor in heme synthesis. PpIX excites and emits at 405 nm (violet) and 633 nm, enabling broad-spectrum activation (74). PpIX accumulation results from increased 5-ALA levels, elevated 5-ALA synthase activity, or malfunctioning ferrochelatase (FECH) enzyme, facilitating its conversion into heme (75). Glioblastoma exhibits reduced FECH expression compared to normal brain tissue, contributing to PpIX accumulation (76). Instances of ventricular wall fluorescence, indicating false positives, are observed even in cases where magnetic resonance imaging (MRI) or macroscopic observation show no evidence of tumor involvement (77). Stummer et al. noted that 5-ALA was effective in increasing tumor resection rates to 65% and enhancing six-month progression-free survival to 41%, as opposed to lower rates without it. However, its fluorescence is stronger in high-grade gliomas but weaker in low-grade ones. The compound becomes fluorescent six hours after intake but loses potency over time as it is metabolized. Other disadvantages include the potential for false positives in cases of radio-necrosis or inflammation, and false negatives in low-density areas (78–82).
Recent advancements have led to the development of both flexible and rigid endoscopic systems that utilize 5-ALA fluorescence, thereby enhancing surgeons' capabilities in the diagnosis and resection of brain tumors. The flexible endoscope system is particularly adept at observing 5-ALA fluorescence, aiding in the accurate identification of tumor margins (83). Conversely, the rigid endoscope system, which has been commercially available and widely reported, demonstrates effectiveness in 5-ALA fluorescence-guided surgery, significantly contributing to surgical outcomes (84, 85). However, despite these significant advancements, the diagnostic utility of these endoscopic systems as adjuncts to microsurgery remains somewhat limited. The integration of confocal endomicroscopy with 5-ALA is proposed as a promising approach to overcome these limitations. This integration potentially allows for a more detailed and nuanced observation of brain tumors at the microstructural level, which could be particularly beneficial in cases of suspected low-grade gliomas (86, 87).
Economically, PR-HMRG, as a fluorescent-guided surgery, may provide a cost-effective alternative compared to the acquisition of other supportive equipment such as navigation systems, intraoperative MRI, or intraoperative ultrasound sonography (88, 89). This makes the initial cost relatively low, especially when integrated into existing systems designed for 5-ALA, leading to avoidance of the substantial initial investments associated with other advanced diagnostic imaging methods (88, 89). These microscopes are already fitted with the necessary light source and fluorescence display monitors. Utilizing the existing setup with a filter exchange avoids the significant costs associated with major equipment modifications. Integrating HMRG and 2MeSiR probes into neurosurgical microscopes equipped with 5-ALA systems involves switching the microscope's internal filters to match the specific excitation and emission profiles of these probes. HMRG requires blue light excitation at 488 nm for its green fluorescence emission at 524 nm, while 2MeSiR needs an excitation filter at 593 nm to enable its 613 nm red fluorescence emission (31, 39).
Recent advancements in aminopeptidase probes, particularly those based on HMRG and 2MeSiR, are showing significant promise in improving cancer detection and monitoring as disease biomarkers (90, 91). These probes offer distinctive advantages, such as rapid activation and reduced background signals. Probes targeting enzymes like GGT, DPP-IV, CAPN1, LAP, and APN demonstrate potential in detecting various cancers and infections. Ongoing research aimed at enhancing their accuracy and minimizing false results is crucial. Systematic reviews and meta-analyses will likely play a key role in evaluating these newer probes as they transition from preclinical to clinical applications. In summary, fluorescent aminopeptidase probes represent a promising advancement in tumor visualization and image-guided surgery.
TS: Data curation, Conceptualization, Writing – original draft. STan: Writing – review & editing, Supervision, Funding acquisition, Conceptualization, Writing – original draft. YK: Writing – review & editing, Supervision, Data curation, Writing – original draft. YS: Writing – review & editing. MK: Writing – review & editing. STak: Writing – review & editing. HT: Writing – review & editing. YU: Supervision, Writing – review & editing. NS: Supervision, Writing – review & editing.
The author(s) declare that financial support was received for the research, authorship, and/or publication of this article.
This research was supported by Japan Agency for Medical Research and Development (AMED) Practical Research for Innovative Cancer Control (23808448; to ST, YU, NS), and Grant-in-Aid for Scientific Research (B) from Japan Society for the Promotion of Science (23H03017; to ST, YU, K. Maruyama, Y. Suzuki, NS).
The authors thank laboratory members of the Department of Neurosurgery and the Urano laboratory of the University of Tokyo for their helpful comments and assistance.
The authors declare that the research was conducted in the absence of any commercial or financial relationships that could be construed as a potential conflict of interest.
The author(s) declared that they were an editorial board member of Frontiers, at the time of submission. This had no impact on the peer review process and the final decision.
All claims expressed in this article are solely those of the authors and do not necessarily represent those of their affiliated organizations, or those of the publisher, the editors and the reviewers. Any product that may be evaluated in this article, or claim that may be made by its manufacturer, is not guaranteed or endorsed by the publisher.
1. Yang K, Wu Z, Zhang H, Zhang N, Wu W, Wang Z, et al. Glioma targeted therapy: insight into future of molecular approaches. Mol Cancer. (2022) 21:39. doi: 10.1186/s12943-022-01513-z
2. Suh JH, Kotecha R, Chao ST, Ahluwalia MS, Sahgal A, Chang EL. Current approaches to the management of brain metastases. Nat Rev Clin Oncol. (2020) 17:279–99. doi: 10.1038/s41571-019-0320-3
3. Ogasawara C, Philbrick BD, Adamson DC. Meningioma: a review of epidemiology, pathology, diagnosis, treatment, and future directions. Biomedicines. (2021) 9:319. doi: 10.3390/biomedicines9030319
4. Wallner KE, Galicich JH, Krol G, Arbit E, Malkin MG. Patterns of failure following treatment for glioblastoma multiforme and anaplastic astrocytoma. Int J Radiat Oncol Biol Phys. (1989) 16:1405–9. doi: 10.1016/0360-3016(89)90941-3
5. Silbergeld DL, Chicoine MR. Isolation and characterization of human malignant glioma cells from histologically normal brain. J Neurosurg. (1997) 86:525–31. doi: 10.3171/jns.1997.86.3.0525
6. Vollmann-Zwerenz A, Leidgens V, Feliciello G, Klein CA, Hau P. Tumor cell invasion in glioblastoma. Int J Mol Sci. (2020) 21:1932. doi: 10.3390/ijms21061932
7. Cuddapah VA, Robel S, Watkins S, Sontheimer H. A neurocentric perspective on glioma invasion. Nat Rev Neurosci. (2014) 15:455–65. doi: 10.1038/nrn3765
8. Kitagawa Y, Tanaka S, Kuriki Y, Yamamoto K, Ogasawara A, Nejo T, et al. Spray fluorescent probes for fluorescence-guided neurosurgery. Front Oncol. (2019) 9:727. doi: 10.3389/fonc.2019.00727
9. Belykh E, Martirosyan NL, Yagmurlu K, Miller EJ, Eschbacher JM, Izadyyazdanabadi M, et al. Intraoperative fluorescence imaging for personalized brain tumor resection: current state and future directions. Front Surg. (2016) 3:55. doi: 10.3389/fsurg.2016.00055
10. Iwaki H, Kamiya M, Kawatani M, Kojima R, Yamasoba T, Urano Y. Fluorescence probes for imaging basic carboxypeptidase activity in living cells with high intracellular retention. Anal Chem. (2021) 93:3470–6. doi: 10.1021/acs.analchem.0c04793
11. Fujita K, Kamiya M, Urano Y. Rapid and sensitive detection of cancer cells with activatable fluorescent probes for enzyme activity. Methods Mol Biol. (2021) 2274:193–206. doi: 10.1007/978-1-0716-1258-3_17
12. Sholl L. Molecular diagnostics of lung cancer in the clinic. Transl Lung Cancer Res. (2017) 6:560–9. doi: 10.21037/tlcr.2017.08.03
14. Kobayashi H, Longmire MR, Ogawa M, Choyke PL. Rational chemical design of the next generation of molecular imaging probes based on physics and biology: mixing modalities, colors and signals. Chem Soc Rev. (2011) 40:4626–48. doi: 10.1039/c1cs15077d
15. Norat P, Soldozy S, Elsarrag M, Sokolowski J, Yagmurlu K, Park MS, et al. Application of indocyanine green videoangiography in aneurysm surgery: evidence, techniques, practical tips. Front Surg. (2019) 6:34. doi: 10.3389/fsurg.2019.00034
16. Kobayashi H, Ogawa M, Alford R, Choyke PL, Urano Y. New strategies for fluorescent probe design in medical diagnostic imaging. Chem Rev. (2010) 110:2620–40. doi: 10.1021/cr900263j
17. Miura T, Urano Y, Tanaka K, Nagano T, Ohkubo K, Fukuzumi S. Rational design principle for modulating fluorescence properties of fluorescein-based probes by photoinduced electron transfer. J Am Chem Soc. (2003) 125:8666–71. doi: 10.1021/ja035282s
18. Urano Y, Asanuma D, Hama Y, Koyama Y, Barrett T, Kamiya M, et al. Selective molecular imaging of viable cancer cells with pH-activatable fluorescence probes. Nat Med. (2009) 15:104–9. doi: 10.1038/nm.1854
19. Sakabe M, Asanuma D, Kamiya M, Iwatate RJ, Hanaoka K, Terai T, et al. Rational design of highly sensitive fluorescence probes for protease and glycosidase based on precisely controlled spirocyclization. J Am Chem Soc. (2013) 135:409–14. doi: 10.1021/ja309688m
20. Kuriki Y, Kamiya M, Kubo H, Komatsu T, Ueno T, Tachibana R, et al. Establishment of molecular design strategy to obtain activatable fluorescent probes for carboxypeptidases. J Am Chem Soc. (2018) 140:1767–73. doi: 10.1021/jacs.7b11014
21. Kawatani M, Yamamoto K, Yamada D, Kamiya M, Miyakawa J, Miyama Y, et al. Fluorescence detection of prostate cancer by an activatable fluorescence probe for PSMA carboxypeptidase activity. J Am Chem Soc. (2019) 141:10409–16. doi: 10.1021/jacs.9b04412
22. Kuriki Y, Yoshioka T, Kamiya M, Komatsu T, Takamaru H, Fujita K, et al. Development of a fluorescent probe library enabling efficient screening of tumour-imaging probes based on discovery of biomarker enzymatic activities. Chem Sci. (2022) 13:4474–81. doi: 10.1039/d1sc06889j
23. Urano Y, Sakabe M, Kosaka N, Ogawa M, Mitsunaga M, Asanuma D, et al. Rapid cancer detection by topically spraying a gamma-glutamyltranspeptidase-activated fluorescent probe. Sci Transl Med. (2011) 3:110–9. doi: 10.1126/scitranslmed.3002823
24. Miyata Y, Ishizawa T, Kamiya M, Yamashita S, Hasegawa K, Ushiku A, et al. Intraoperative imaging of hepatic cancers using gamma-glutamyltranspeptidase-specific fluorophore enabling real-time identification and estimation of recurrence. Sci Rep. (2017) 7:3542. doi: 10.1038/s41598-017-03760-3
25. Mizushima T, Ohnishi S, Shimizu Y, Hatanaka Y, Hatanaka KC, Hosono H, et al. Fluorescent imaging of superficial head and neck squamous cell carcinoma using a gamma-glutamyltranspeptidase-activated targeting agent: a pilot study. BMC Cancer. (2016) 16:411. doi: 10.1186/s12885-016-2421-z
26. Kikkawa F, Kajiyama H, Shibata K, Ino K, Nomura S, Mizutani S. Dipeptidyl peptidase IV in tumor progression. Biochim Biophys Acta. (2005) 1751:45–51. doi: 10.1016/j.bbapap.2004.09.028
27. Deacon CF. Physiology and pharmacology of DPP-4 in glucose homeostasis and the treatment of type 2 diabetes. Front Endocrinol (Lausanne). (2019) 10:80. doi: 10.3389/fendo.2019.00080
28. Goscinski MA, Suo ZH, Nesland JM, Florenes VA, Giercksky KE. Dipeptidyl peptidase IV expression in cancer and stromal cells of human esophageal squamous cell carcinomas, adenocarcinomas and squamous cell carcinoma cell lines. APMIS. (2008) 116:823–31. doi: 10.1111/j.1600-0463.2008.01029.x
29. Tanaka T, Umeki K, Yamamoto I, Sakamoto F, Noguchi S, Ohtaki S. CD26 (dipeptidyl peptidase IV/DPP IV) as a novel molecular marker for differentiated thyroid carcinoma. Int J Cancer. (1995) 64:326–31. doi: 10.1002/ijc.2910640508
30. Onoyama H, Kamiya M, Kuriki Y, Komatsu T, Abe H, Tsuji Y, et al. Rapid and sensitive detection of early esophageal squamous cell carcinoma with fluorescence probe targeting dipeptidylpeptidase IV. Sci Rep. (2016) 6:26399. doi: 10.1038/srep26399
31. Kitagawa Y, Tanaka S, Kamiya M, Kuriki Y, Yamamoto K, Shimizu T, et al. A novel topical fluorescent probe for detection of glioblastoma. Clin Cancer Res. (2021) 27:3936–47. doi: 10.1158/1078-0432.CCR-20-4518
32. Komatsu T, Hanaoka K, Adibekian A, Yoshioka K, Terai T, Ueno T, et al. Diced electrophoresis gel assay for screening enzymes with specified activities. J Am Chem Soc. (2013) 135:6002–5. doi: 10.1021/ja401792d
33. Takahashi R, Ishizawa T, Sato M, Inagaki Y, Takanka M, Kuriki Y, et al. Fluorescence imaging using enzyme-activatable probes for real-time identification of pancreatic cancer. Front Oncol. (2021) 11:714527. doi: 10.3389/fonc.2021.714527
34. Yogo T, Umezawa K, Kamiya M, Hino R, Urano Y. Development of an activatable fluorescent probe for prostate cancer imaging. Bioconjug Chem. (2017) 28:2069–76. doi: 10.1021/acs.bioconjchem.7b00233
35. Yamamoto K, Kamiya M, Urano Y. Highly sensitive fluorescence imaging of cancer with avidin-protease probe conjugate. Bioorg Med Chem Lett. (2019) 29:126663. doi: 10.1016/j.bmcl.2019.126663
36. Yamashita S, Sakabe M, Ishizawa T, Hasegawa K, Urano Y, Kokudo N. Visualization of the leakage of pancreatic juice using a chymotrypsin-activated fluorescent probe. Br J Surg. (2013) 100:1220–8. doi: 10.1002/bjs.9185
37. Weissleder R. A clearer vision for in vivo imaging. Nat Biotechnol. (2001) 19:316–7. doi: 10.1038/86684
38. Kushida Y, Hanaoka K, Komatsu T, Terai T, Ueno T, Yoshida K, et al. Red fluorescent scaffold for highly sensitive protease activity probes. Bioorg Med Chem Lett. (2012) 22:3908–11. doi: 10.1016/j.bmcl.2012.04.114
39. Ogasawara A, Kamiya M, Sakamoto K, Kuriki Y, Fujita K, Komatsu T, et al. Red fluorescence probe targeted to dipeptidylpeptidase-IV for highly sensitive detection of esophageal cancer. Bioconjug Chem. (2019) 30:1055–60. doi: 10.1021/acs.bioconjchem.9b00198
40. Takahashi S, Ishizawa T, Inagaki Y, Tanaka M, Ogasawara A, Kuriki Y, et al. Real-time fluorescence imaging to identify cholangiocarcinoma in the extrahepatic biliary tree using an enzyme-activatable probe. Liver Cancer. (2023) 12:590–602. doi: 10.1159/000530645
41. Fujita K, Kamiya M, Yoshioka T, Ogasawara A, Hino R, Kojima R, et al. Rapid and accurate visualization of breast tumors with a fluorescent probe targeting α-mannosidase 2C1. ACS Cent Sci. (2020) 6:2217–27. doi: 10.1021/acscentsci.0c01189
42. Kawashima S, Yoshida D, Yoshioka T, Ogasawara A, Fujita K, Yanagiya M, et al. Rapid imaging of lung cancer using a red fluorescent probe to detect dipeptidyl peptidase 4 and puromycin-sensitive aminopeptidase activities. Sci Rep. (2022) 12:9100. doi: 10.1038/s41598-022-12665-9
43. Kondo C, Shibata K, Terauchi M, Kajiyama H, Ino K, Nomura S, et al. A novel role for placental leucine aminopeptidase (P-LAP) as a determinant of chemoresistance in endometrial carcinoma cells. Int J Cancer. (2006) 118:1390–4. doi: 10.1002/ijc.21509
44. Gong Q, Shi W, Li L, Ma H. Leucine aminopeptidase may contribute to the intrinsic resistance of cancer cells toward cisplatin as revealed by an ultrasensitive fluorescent probe. Chem Sci. (2016) 7:788–92. doi: 10.1039/c5sc03600c
45. He X, Li L, Fang Y, Shi W, Li X, Ma H. In vivo imaging of leucine aminopeptidase activity in drug-induced liver injury and liver cancer via a near-infrared fluorescent probe. Chem Sci. (2017) 8:3479–83. doi: 10.1039/c6sc05712h
46. Cao T, Zhang L, Zheng L, Qian J, Iqbal A, Iqbal K, et al. A ratiometric fluorescent sensor for rapid detection of the pyroglutamate aminopeptidase-1 in mouse tumors. J Mater Chem B. (2021) 9:4546–54. doi: 10.1039/d1tb00372k
47. Liu X, Zhang QY, Wang F, Jiang JH. A near infrared fluorescent probe for the detection and imaging of prolyl aminopeptidase activity in living cells. Analyst. (2019) 144:5980–5. doi: 10.1039/c9an01303b
48. Terauchi M, Kajiyama H, Shibata K, Ino K, Nawa A, Mizutani S, et al. Inhibition of APN/CD13 leads to suppressed progressive potential in ovarian carcinoma cells. BMC Cancer. (2007) 7:140. doi: 10.1186/1471-2407-7-140
49. Kido A, Krueger S, Haeckel C, Roessner A. Inhibitory effect of antisense aminopeptidase N (APN/CD13) cDNA transfection on the invasive potential of osteosarcoma cells. Clin Exp Metastasis. (2003) 20:585–92. doi: 10.1023/a:1027383729767
50. Wex T, Lendeckel U, Reinhold D, Kähne T, Arndt M, Frank K, et al. Antisense-mediated inhibition of aminopeptidase N (CD13) markedly decreases growth rates of hematopoietic tumour cells. Adv Exp Med Biol. (1997) 421:67–73. doi: 10.1007/978-1-4757-9613-1_9
51. He X, Hu Y, Shi W, Li X, Ma H. Design, synthesis and application of a near-infrared fluorescent probe for in vivo imaging of aminopeptidase N. Chem Commun (Camb). (2017) 53:9438–41. doi: 10.1039/c7cc05142e
52. Li H, Li Y, Yao Q, Fan J, Sun W, Long S, et al. In situ imaging of aminopeptidase N activity in hepatocellular carcinoma: a migration model for tumour using an activatable two-photon NIR fluorescent probe. Chem Sci. (2019) 10:1619–25. doi: 10.1039/c8sc04685a
53. Schaff LR, Grommes C. Update on novel therapeutics for primary CNS lymphoma. Cancers (Basel). (2021) 13:5372. doi: 10.3390/cancers13215372
54. Koh KN, Wong RX, Lee DE, Han JW, Byun HK, Yoon HI, et al. Outcomes of intracranial germinoma-A retrospective multinational Asian study on effect of clinical presentation and differential treatment strategies. Neuro Oncol. (2022) 24:1389–99. doi: 10.1093/neuonc/noab295
55. Brown TJ, Brennan MC, Li M, Church EW, Brandmeir NJ, Rakszawski KL, et al. Association of the extent of resection with survival in glioblastoma: a systematic review and meta-analysis. JAMA Oncol. (2016) 2:1460–9. doi: 10.1001/jamaoncol.2016.1373
56. McGirt MJ, Chaichana KL, Attenello FJ, Weingart JD, Than K, Burger PC, et al. Extent of surgical resection is independently associated with survival in patients with hemispheric infiltrating low-grade gliomas. Neurosurgery. (2008) 63:700–7. doi: 10.1227/01.NEU.0000325729.41085.73
57. Nagaya T, Nakamura YA, Choyke PL, Kobayashi H. Fluorescence-guided surgery. Front Oncol. (2017) 7:314. doi: 10.3389/fonc.2017.00314
58. Moore GE, Peyton WT, French LA, Walker WW. The clinical use of fluorescein in neurosurgery. J Neurosurg. (1948) 5:392–8. doi: 10.3171/jns.1948.5.4.0392
59. Sun R, Cuthbert H, Watts C. Fluorescence-Guided surgery in the surgical treatment of gliomas: past, present and future. Cancers (Basel). (2021) 13:3508. doi: 10.3390/cancers13143508
60. Hwang SW, Malek AM, Schapiro R, Wu JK. Intraoperative use of indocyanine green fluorescence videography for resection of a spinal cord hemangioblastoma. Neurosurgery. (2010) 67:300–3. doi: 10.1227/01.NEU.0000383876.72704.7B
61. Hojo M, Arakawa Y, Funaki T, Yoshida K, Kikuchi T, Takagi Y, et al. Usefulness of tumor blood flow imaging by intraoperative indocyanine green videoangiography in hemangioblastoma surgery. World Neurosurg. (2014) 82:e495–501. doi: 10.1016/j.wneu.2013.02.009
62. Ferroli P, Acerbi F, Albanese E, Tringali G, Broggi M, Franzini A, et al. Application of intraoperative indocyanine green angiography for CNS tumors: results on the first 100 cases. Acta Neurochir Suppl. (2011) 109:251–7. doi: 10.1007/978-3-211-99651-5_40
63. Takami H, Tanaka S, Takayanagi S, Nakatomi H, Saito N. Indocyanine green illuminates the way to cut the tentorium in occipital transtentorial approach: technical note. Br J Neurosurg. (2021) 6:1–3. doi: 10.1080/02688697.2021.1927982
64. Zeh R, Sheikh S, Xia L, Pierce J, Newton A, Predina J, et al. The second window ICG technique demonstrates a broad plateau period for near infrared fluorescence tumor contrast in glioblastoma. PLoS One. (2017) 12:e0182034. doi: 10.1371/journal.pone.0182034
65. Belykh E, Shaffer KV, Lin C, Byvaltsev VA, Preul MC, Chen L. Blood-brain barrier, blood-brain tumor barrier, and fluorescence-guided neurosurgical oncology: delivering optical labels to brain tumors. Front Oncol. (2020) 10:739. doi: 10.3389/fonc.2020.00739
66. Acerbi F, Broggi M, Schebesch KM, Höhne J, Cavallo C, De Laurentis C, et al. Fluorescein-guided surgery for resection of high-grade gliomas: a multicentric prospective phase II study (FLUOGLIO). Clin Cancer Res. (2018) 24:52–61. doi: 10.1158/1078-0432.Ccr-17-1184
67. Diaz RJ, Dios RR, Hattab EM, Burrell K, Rakopoulos P, Sabha N, et al. Study of the biodistribution of fluorescein in glioma-infiltrated mouse brain and histopathological correlation of intraoperative findings in high-grade gliomas resected under fluorescein fluorescence guidance. J Neurosurg. (2015) 122:1360–9. doi: 10.3171/2015.2.Jns132507
68. Smith EJ, Gohil K, Thompson CM, Naik A, Hassaneen W. Fluorescein-guided resection of high grade gliomas: a meta-analysis. World Neurosurg. (2021) 155:181–8 e7. doi: 10.1016/j.wneu.2021.08.126
69. Ziebart A, Stadniczuk D, Roos V, Ratliff M, von Deimling A, Hänggi D, et al. Deep neural network for differentiation of brain tumor tissue displayed by confocal laser endomicroscopy. Front Oncol. (2021) 11:668273. doi: 10.3389/fonc.2021.668273
70. Acerbi F, Pollo B, De Laurentis C, Restelli F, Falco J, Vetrano IG, et al. Ex vivo fluorescein-assisted confocal laser endomicroscopy (CONVIVO® system) in patients with glioblastoma: results from a prospective study. Front Oncol. (2020) 10:606574. doi: 10.3389/fonc.2020.606574
71. Folaron M, Strawbridge R, Samkoe KS, Filan C, Roberts DW, Davis SC. Elucidating the kinetics of sodium fluorescein for fluorescence-guided surgery of glioma. J Neurosurg. (2018) 131:724–34. doi: 10.3171/2018.4.JNS172644
72. Iinuma S, Farshi SS, Ortel B, Hasan T. A mechanistic study of cellular photodestruction with 5-aminolaevulinic acid-induced porphyrin. Br J Cancer. (1994) 70:21–8. doi: 10.1038/bjc.1994.244
73. Stummer W, Stocker S, Novotny A, Heimann A, Sauer O, Kempski O, et al. In vitro and in vivo porphyrin accumulation by C6 glioma cells after exposure to 5-aminolevulinic acid. J Photochem Photobiol B. (1998) 45:160–9. doi: 10.1016/s1011-1344(98)00176-6
74. Markwardt NA, Haj-Hosseini N, Hollnburger B, Stepp H, Zelenkov P, Rühm A. 405 nm versus 633 nm for protoporphyrin IX excitation in fluorescence-guided stereotactic biopsy of brain tumors. J Biophotonics. (2016) 9:901–12. doi: 10.1002/jbio.201500195
75. Fratz EJ, Hunter GA, Ferreira GC. Expression of murine 5-aminolevulinate synthase variants causes protoporphyrin IX accumulation and light-induced mammalian cell death. PLoS One. (2014) 9:e93078. doi: 10.1371/journal.pone.0093078
76. Stepp H, Stummer W. 5-ALA In the management of malignant glioma. Lasers Surg Med. (2018) 50:399–419. doi: 10.1002/lsm.22933
77. Moon JH, Kim SH, Shim JK, Roh TH, Sung KS, Lee JH, et al. Histopathological implications of ventricle wall 5-aminolevulinic acid-induced fluorescence in the absence of tumor involvement on magnetic resonance images. Oncol Rep. (2016) 36:837–44. doi: 10.3892/or.2016.4881
78. Stummer W, Novotny A, Stepp H, Goetz C, Bise K, Reulen HJ. Fluorescence-guided resection of glioblastoma multiforme by using 5-aminolevulinic acid-induced porphyrins: a prospective study in 52 consecutive patients. J Neurosurg. (2000) 93:1003–13. doi: 10.3171/jns.2000.93.6.1003
79. Stummer W, Pichlmeier U, Meinel T, Wiestler OD, Zanella F, Reulen HJ, et al. Fluorescence-guided surgery with 5-aminolevulinic acid for resection of malignant glioma: a randomised controlled multicentre phase III trial. Lancet Oncol. (2006) 7:392–401. doi: 10.1016/S1470-2045(06)70665-9
80. La Rocca G, Sabatino G, Menna G, Altieri R, Ius T, Marchese E, et al. 5-Aminolevulinic acid false positives in cerebral neuro-oncology: not all that is fluorescent is tumor. A case-based update and literature review. World Neurosurg. (2020) 137:187–93. doi: 10.1016/j.wneu.2020.01.238
81. Omoto K, Matsuda R, Nakagawa I, Motoyama Y, Nakase H. False-positive inflammatory change mimicking glioblastoma multiforme under 5-aminolevulinic acid-guided surgery: a case report. Surg Neurol Int. (2018) 9:49. doi: 10.4103/sni.sni_473_17
82. de Laurentis C, Del Bene M, Fociani P, Tonello C, Pollo B, DiMeco F. 5-ALA Fluorescence in case of brain abscess by aggregatibacter mimicking glioblastoma. World Neurosurg. (2019) 125:175–8. doi: 10.1016/j.wneu.2019.01.190
83. Tamura Y, Kuroiwa T, Kajimoto Y, Miki Y, Miyatake S, Tsuji M. Endoscopic identification and biopsy sampling of an intraventricular malignant glioma using a 5-aminolevulinic acid-induced protoporphyrin IX fluorescence imaging system. Technical note. J Neurosurg. (2007) 106:507–10. doi: 10.3171/jns.2007.106.3.507
84. Rapp M, Kamp M, Steiger HJ, Sabel M. Endoscopic-assisted visualization of 5-aminolevulinic acid-induced fluorescence in malignant glioma surgery: a technical note. World Neurosurg. (2014) 82:e277–9. doi: 10.1016/j.wneu.2013.07.002
85. Bettag C, Hussein A, Behme D, Maragkou T, Rohde V, Mielke D. Endoscopic fluorescence-guided resection increases radicality in glioblastoma surgery. Oper Neurosurg (Hagerstown). (2020) 18:41–6. doi: 10.1093/ons/opz082
86. Kiesel B, Freund J, Reichert D, Wadiura L, Erkkilae MT, Woehrer A, et al. 5-ALA in suspected low-grade gliomas: current role, limitations, and new approaches. Front Oncol. (2021) 11:699301. doi: 10.3389/fonc.2021.699301
87. Sanai N, Snyder LA, Honea NJ, Coons SW, Eschbacher JM, Smith KA, et al. Intraoperative confocal microscopy in the visualization of 5-aminolevulinic acid fluorescence in low-grade gliomas. J Neurosurg. (2011) 115:740–8. doi: 10.3171/2011.6.Jns11252
88. Eljamel MS, Mahboob SO. The effectiveness and cost-effectiveness of intraoperative imaging in high-grade glioma resection; a comparative review of intraoperative ALA, fluorescein, ultrasound and MRI. Photodiagnosis Photodyn Ther. (2016) 16:35–43. doi: 10.1016/j.pdpdt.2016.07.012
89. Abraham P, Sarkar R, Brandel MG, Wali AR, Rennert RC, Lopez Ramos C, et al. Cost-effectiveness of intraoperative MRI for treatment of high-grade gliomas. Radiology. (2019) 291:689–97. doi: 10.1148/radiol.2019182095
90. Iwatate RJ, Kamiya M, Umezawa K, Kashima H, Nakadate M, Kojima R, et al. Silicon rhodamine-based near-infrared fluorescent probe for γ-glutamyltransferase. Bioconjug Chem. (2018) 29:241–4. doi: 10.1021/acs.bioconjchem.7b00776
Keywords: hydroxymethyl rhodamine green, γ-glutamyl-HMRG, glutamylprolyl-HMRG, glioblastoma, glioma, 5-aminolevulinic acid
Citation: Shimizu T, Tanaka S, Kitagawa Y, Sakaguchi Y, Kamiya M, Takayanagi S, Takami H, Urano Y and Saito N (2024) Advancement of fluorescent aminopeptidase probes for rapid cancer detection–current uses and neurosurgical applications. Front. Surg. 11:1298709. doi: 10.3389/fsurg.2024.1298709
Received: 22 September 2023; Accepted: 22 February 2024;
Published: 7 March 2024.
Edited by:
Mark Preul, Barrow Neurological Institute (BNI), United StatesReviewed by:
Evgenii Belykh, Rutgers University, United States© 2024 Shimizu, Tanaka, Kitagawa, Sakaguchi, Kamiya, Takayanagi, Takami, Urano and Saito. This is an open-access article distributed under the terms of the Creative Commons Attribution License (CC BY). The use, distribution or reproduction in other forums is permitted, provided the original author(s) and the copyright owner(s) are credited and that the original publication in this journal is cited, in accordance with accepted academic practice. No use, distribution or reproduction is permitted which does not comply with these terms.
*Correspondence: Yosuke Kitagawa eW9raXRhZ2F3YS10a3lAdW1pbi5hYy5qcA==
†These authors have contributed equally to this work
Disclaimer: All claims expressed in this article are solely those of the authors and do not necessarily represent those of their affiliated organizations, or those of the publisher, the editors and the reviewers. Any product that may be evaluated in this article or claim that may be made by its manufacturer is not guaranteed or endorsed by the publisher.
Research integrity at Frontiers
Learn more about the work of our research integrity team to safeguard the quality of each article we publish.