- Department of the Second Plastic and Aesthetic Surgery, the First People’s Hospital of Foshan, Foshan, China
Skin tissue regeneration and repair is a complex process involving multiple cell types, and current therapies are limited to promoting skin wound healing. Mesenchymal stromal cells (MSCs) have been proven to enhance skin tissue repair through their multidifferentiation and paracrine effects. However, there are still difficulties, such as the limited proliferative potential and the biological processes that need to be strengthened for MSCs in wound healing. Recently, three-dimensional (3D) bioprinting has been applied as a promising technology for tissue regeneration. 3D-bioprinted MSCs could maintain a better cell ability for proliferation and expression of biological factors to promote skin wound healing. It has been reported that 3D-bioprinted MSCs could enhance skin tissue repair through anti-inflammatory, cell proliferation and migration, angiogenesis, and extracellular matrix remodeling. In this review, we will discuss the progress on the effect of MSCs and 3D bioprinting on the treatment of skin tissue regeneration, as well as the perspective and limitations of current research.
Introduction
Skin is the first barrier to protect us from invading pathogens and environmental challenges. However, skin tissue injury is common due to trauma and pathological situations such as diabetes mellitus and vascular disorder (1). Wound healing is a complex process which approximately may divide into three phases: inflammation, proliferation, and extracellular matrix (ECM) remodeling (2–4). Multiple cell types, such as platelets, neutrophils, macrophages, fibroblasts (FBs), and myofibroblasts, take part in the skin wound healing process, regulated by biological factors (5). As a result of various reasons, including physiological inflammatory, infection, or systemic diseases, it turns into a chronic wound, which brings an enormous economic burden and influences the population's health (6, 7). Over the decades, the field of skin tissue regeneration has made progress in acute and chronic wound healing. However, there is not enough solid evidence to support general therapeutic modalities that noticeably improve wound healing, including negative pressure or hyperbaric oxygen therapy. Further studies are needed to figure out a superior therapeutic method of skin wound healing (8).
Mesenchymal stromal cells (MSCs) make a novel and effective contribution to wound healing. They can be obtained from bone marrow, adipose, umbilical cord tissue (9–11), etc. Unlike embryonic stromal cells (ESCs) or induced pluripotent stromal cells (iPSCs), MSCs are easy to isolate from original tissues with less severe ethical issues and a lower risk of teratoma formation (12, 13). Both clinical and preclinical studies suggested that MSCs can accelerate re-epithelization, neovascularization, and collagen deposition to promote wound healing (14, 15). Recently, MSCs have been introduced as a new treatment for wound healing because of their biological characteristics and paracrine function, which could secrete various bioactive factors, such as vascular endothelial growth factor (VEGF), hepatocyte growth factor (HGF), transforming growth factor β1 (TGF-β1), KGF, IL-8, and IL-6 (16, 17). Moreover, as a cell-free therapy, extracellular vesicles (EVs) derived from MSCs have become a promising wound healing treatment. Extracellular vesicles contain abundant messenger RNA (mRNA), microRNAs (miRNAs), and long noncoding RNA (LncRNA) to regulate the activity of host cells and promote wound healing, avoiding risks related to cell transplant (18). Exosomes derived from MSCs have been confirmed that remarkably promote angiogenesis (19, 20), increase collagen synthesis (21), and expression of growth factors (22) in wound healing. Despite all the advantages of MSCs therapy, there are still difficulties, such as the limited proliferative potential and the biological processes that need to be strengthened.
Recently, three-dimensional (3D) bioprinting as additive manufacturing technology has been applied to fabricate tissues/organs to achieve the controllable spatial distribution of living cells and biological materials (23–25). With biocompatibility biomaterials, such as decellularized extracellular matrix (dECM), alginate and hyaluronic acid, 3D-bioprinted cells have been used for tissue repair (26). In the field of skin tissue regeneration, 3D-bioprinted bioengineered skin grafts containing FBs, endothelial cells (ECs), or human dermal fibroblasts (HDFs), have been proved could enhance skin wound repair (27, 28). In recent years, a variety of research explored the potential of MSCs as effective seed cells for the treatment of skin wound healing. 3D-bioprinted MSCs maintained a better ability to proliferate increased biological factors to promote skin wound healing (29, 30). With their ability to differentiate and paracrine effect, MSCs show infinite potentialities based on 3D bioprinting technology (31).
Accordingly, in this review, we will discuss current knowledge about the role of MSCs and 3D bioprinting in the treatment of skin wound healing, as well as the perspective and limitations of recent research.
The process of skin wound repair
Following injury, mammalian wound healing is traditionally divided into three phases: inflammation, proliferation, and ECM remodeling (32). The first stage of wound healing, inflammation, will happen from the moment of tissue damage. Platelets, neutrophils, macrophages, and fibrin matrices work together to prevent ongoing blood and fluid losses as well as infection. In contrast, macrophages are considered vital for coordinating other issues in the wound healing process (33). The second stage, proliferation, is attributed to the proliferation and migration of fibroblasts, myofibroblasts, and keratinocytes. These different cell types are associated with angiogenesis and re-epithelialization to restore the barrier function of the epidermis (2, 34). In the third stage, ECM remodeling, all the activities after injury cease. Macrophages and myofibroblasts will undergo apoptosis, leaving collagen and other extracellular matrix proteins (35). Matrix metalloproteinases secreted by macrophages, fibroblasts, and endothelial cells strengthen the repaired tissue (36). However, the incongruity of any stage will occur in chronic wounds or keloid scar formation (37).
Chronic wounds or impaired wound healing are defects in the skin for more than 6 weeks (38). Typically, chronic wounds may be divided into three types: vascular dysfunction, diabetes, and pressure ulcers (39). Inappropriate physiological inflammatory reactions, underlying systemic diseases, such as diabetes mellitus and vascular disorders, and infections will lead to impairments of cell proliferation and migration and extracellular matrix damage (6, 8). The formation of myofibroblasts is compromised, partly due to hypoxic conditions or vascular insufficiency, which will contribute to the lack of granulation tissue and delayed wound healing (40).
Unfortunately, damaged tissue could not regain the properties of unwounded tissue (41). Importantly, nonhealing and dysfunctional healing cause lifelong disability and a significant economic burden (42). Therefore, the emphasis of research should be on enhancing wound healing and regeneration of original tissues in the future.
MSCs and skin wound healing
Recently, MSCs have become a promising therapy in the field of regenerative medicine because of their pluripotency, self-renewal, and paracrine of biological factors (43). MSCs, possibly derived from the mesoderm, could differentiate into various mesenchymal tissue lineages, such as chondrocytes, adipocytes, osteoblasts, and even myoblasts (44). The most MSCs used in skin regeneration are adult stromal cells because of less ethical controversy and substantial legal restrictions (45). MSCs would accelerate skin wound healing by regulating multiple phases of wound reconstruction, including inflammatory response, cell proliferation, wound angiogenesis, and wound remodeling (46). Fifty years ago, Friedenstein et al. have been isolated bone marrow stromal cells (BMSCs) from bone marrow (47). In the last few decades, adipose-derived stromal cells (ADSCs) have been a new source of MSCs introduced to wound healing because they are obtained from adipose tissues with less invasive methods and less ethical concerns (16). Including human umbilical cord-derived mesenchymal stromal cells (HUC-MSCs), MSCs play an important role in wound healing (Figure 1).
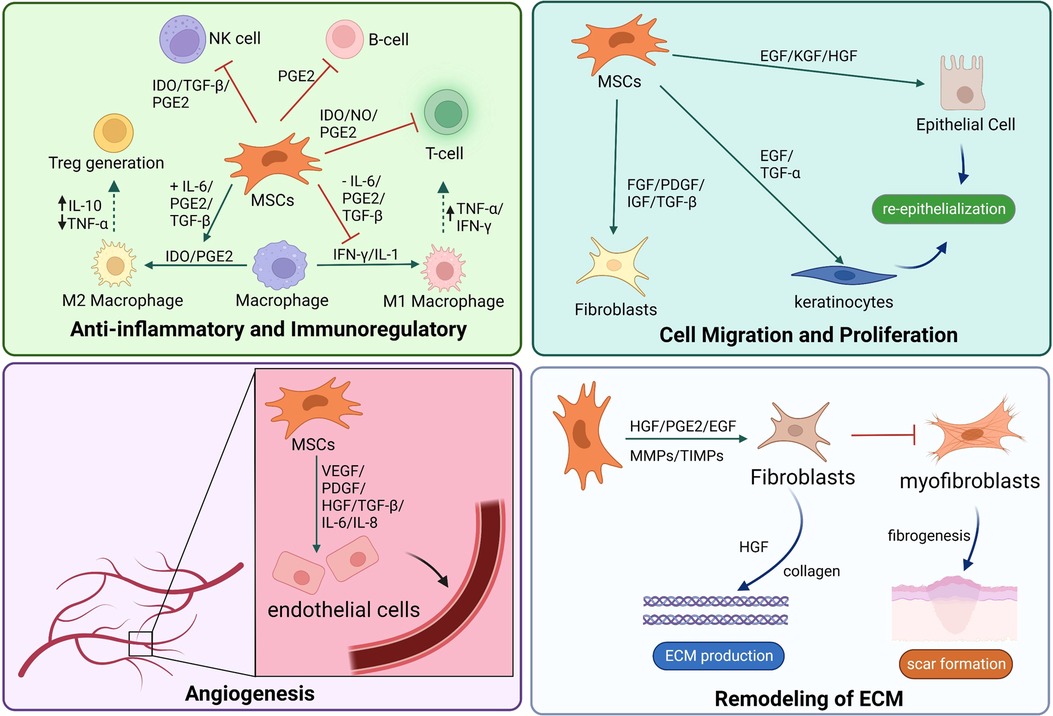
Figure 1. MSCs promote skin tissue regeneration through anti-inflammatory and immunoregulatory, cell migration and proliferation, angiogenesis, and ECM remodeling. MSCs, mesenchymal stromal cells; ECM, extracellular matrix.
MSCs and anti-inflammatory/immunoregulatory
There is an amount of literature about skin wound healing, pointing out that skin wound healing is a complex process that depends on many cell types. Molecular and cellular mechanisms are critical for the process of cutaneous wound healing. At the early stage of wound healing, keratinocytes and inflammatory cells seem necessary. First, leukocytes, especially neutrophil granulocytes, transmigrate to the injury site to initiate and perpetuate inflammation (48, 49). Inflammation is a self-defense mechanism against noxious stimuli in the early stages of wound healing, with a significant objective of removing necrotic debris and pathogenic microorganisms from the wound bed and controlling local area damage (50). MSCs can cooperate with various immune cells to modulate inflammatory responses such as B cells, T cells, natural killer (NK) cells, neutrophils, and macrophages (51, 52). Moreover, MSCs promote the polarization of macrophages to an M2-like phenotype, which reduces inflammation and immunosuppressive function through a prostaglandin E2-dependent mechanism (53). Recent studies have demonstrated that MSCs encourage the polarization of macrophages toward an anti-inflammatory, reparative M2 phenotype by a paracrine mechanism. It has been reported that MSCs could secrete transforming growth factor beta (TGF-β) (54), C-X-C motif chemokine ligand 12 (CXCL12) (55), tumor necrosis factor-α-induced gene/protein-6 (TSG-6) (56), and prostaglandin E2 (53) to induce macrophage M2 polarization (53, 57). The cell–cell interaction between MSCs and macrophages in the progress of skin wound healing can accelerate skin tissue regeneration (58).
Meanwhile, MSCs also inhibit the proliferation of activated helper T (Th) cells (59). Mo et al. found that MSCs suppressed Th2 inflammation by regulating macrophage activation via soluble mediators rather than direct cell-to-cell contact (60). Besides, the extracts of MSCs could suppress Th2 cells and reduce the expression of IL-17 and IFN-γ, which further demonstrate that MSCs inhibit Th2 cells through paracrine factors (61, 62). In clinical, the immunomodulatory ability of MSCs could reverse the ratio of Th1 cells to Th2 cells, with an increase in Th1 and a decrease in Th2 achieving a new balance (63). This interaction could decrease the production of interferon γ (IFN-γ) and interleukin (IL)-17 and increase the production of IL-4 secreted by Th cells, thus leading to T cells polarizing from a pro-inflammatory to an anti-inflammatory phenotype (64).
MSC sheet technology enables cultured MSC harvest without enzymatic treatment or cell or protein disruption using temperature-responsive cell culture dishes (65). With this application, changes in culture temperature cause oscillation between hydrophilic and hydrophobic states. Cells adhere to and proliferate on the surface of the culture dish at 37 °C, and a monolayer cell sheet with ECM detach spontaneously at temperatures below 32 °C without enzymatic digestion (66). Application of MSCs sheet also brings preferable wound healing and less scar formation, believed that it can suppress macrophage infiltration and chemotactic response of macrophages (67, 68). In clinical research, MSCs could reduce the expression of inflammation and oxidative stress-related proteins to improve diabetic foot ulcers (DFU) healing by Nrf2 (69). It has been reported that MSCs therapy may reduce inflammation and has been applied in acute and chronic liver injury, which suggests MSCs improve tissue regeneration using their anti-inflammatory properties (70).
MSCs and proliferation/migration
The intermediate stage of wound healing contains proliferation and migration of keratinocytes, the proliferation of fibroblasts, matrix deposition, and angiogenesis. Stationary keratinocytes are converted to flat migratory keratinocytes to start re-epithelialization (27). In this stage, fibroblasts stimulate wound healing by proliferating and synthesizing a large amount of ECM components such as collagen and elastic fibers under the stimulation of trauma (71). MSCs stimulate the migration and proliferation of keratinocytes by the expression of epidermal growth factor (EGF) and transforming growth factor-α (TGF-α) (72). The treatment of MSCs improves the survival rate of fibroblasts and enhances the healing effects (73). Evidence shows that BMSCs improve the proliferation and migration of dermal fibroblasts (74). In vivo, MSCs increased the expression of CK19 and proliferating cell nuclear antigen (PCNA) and promoted the regeneration of dermal tissue (75, 76). Specifically, PCNA participates in cell proliferation by mediating DNA polymerase while blocking the PCNA production in cells severely affects cell division, which takes a significant part in the synthesis of DNA and its repair, cell proliferation, and progression of the cell cycle (77, 78). Studies show treatment of MSCs could elevate the expression of PCNA, promote wound healing, and enhance re-epithelialization (79–81).
Animals treated with MSCs show improving wound healing with no detectable side effects related to increasing viability, proliferation, and migration of epithelial cells (82). In vivo, the application of MSCs could promote re-epithelialization by enhancing the proliferation of epidermal keratinocytes (83). Moreover, MSCs strengthened the dermal and epidermal cell proliferation ability in a dose-dependent manner and positively impacted oxidative stress injury, which could improve cutaneous wound healing (84). All the evidence indicates that MSCs have a positive effect on wound healing.
MSCs and angiogenesis
Angiogenesis plays an essential role in the process of wound healing. Creating new capillaries will bring oxygen and nutrients to growing tissues and remove catabolic wastes. Therefore, angiogenesis contributes to the repair of wound tissue (85). Angiogenesis is strictly regulated by a variety of factors, mainly through the secretion of proangiogenic factors, such as VEGF and platelet-derived growth factor (PDGF), leading to stimulating endothelial cell proliferation and migration and angiogenesis (86). Rehman et al. found that MSCs secreted synergistic proangiogenic growth factors, such as VEGF and HGF, which enhance angiogenesis (87). The previous review reported that MSCs could promote re-epithelialization, angiogenesis, collagen synthesis, and neovascularization by secretion of multiple growth factors including VEGF, HGF, TGF-β1, KGF, IL-8, and IL-6 during the progress of wound healing (88). MSCs improved cell viability, migration, and angiogenesis of the high glucose-damaged human umbilical vein endothelial cells (HUVECs) through paracrine, increasing the expression of IL-6, TNF-α, ICAM-1, VCAM-1, BAX, P16, P53, and ET-1(89). Different experimental models mimic the effect of MSCs in wound healing, performed preferentially in rodents. Subcutaneously injecting MSCs into the full-thickness wounds of mice will result in more angiogenesis and promote wound healing (90). With treatment of MSCs, the density of neovascularization in wound bed was increased, also with the expression of VEGF, while the expression of IL-10, IL-6, IL-1β, and TNF-α were significantly decreased (91). Meanwhile, MSCs could enhance angiogenesis and improve the survival rate of graft skin in vivo (92). MSCs also positively impacted vascular regeneration and endothelial leukocyte adhesion modulation in critical ischemic skin (93).
MSCs and ECM remodeling
Late-stage healing involves remodeling of ECM, resulting in scar formation and barrier restoration (28). During the wound remodeling stage, fibroblasts differentiate into myofibroblasts, and the granulation tissue gradually becomes fibrotic; collagen gradually increases; the wound begins to contract, and eventually, scar tissue is formed (94). MSCs would release plenty of cytokines and growth factors with anti-fibrotic properties (95, 96). In the early progress, MSCs improve collagen remodeling through synthesizing collagen types I and III of wound healing while reducing scarring in the late stage by inhibiting collagen formation (97). Treated with MSCs, fibroblasts secret more HGF and increase collagen production. With the inhibition of excessive fibrogenesis, fibroblast proliferation, and α-smooth muscle actin expression, MSCs can reduce scar formation during wound healing (98). MSCs also inhibited fibrosis by decreasing the expression of profibrotic genes and protein, promoting extracellular matrix regeneration, inhibiting fibroblast contraction, and reversing myofibroblast activation (99). It has been reported that MSCs were capable of myofibroblast suppression and anti-scar formation in vivo and in vitro (100). MSCs have been considered significant in skin wound repair. However, further research is still needed to increase the biological regulation of wound healing.
MSC-EVs and skin wound healing
Although mesenchymal stem cells could regulate inflammation, promote cell proliferation and migration, and improve angiogenesis during skin wound healing, a few disadvantages limit its wide application. Mesenchymal stem cell therapy may have a problem with storage and transportation, as well as the risk of cancer and deformities. As a result, it is not a definitive treatment without a long-term study on safety (101). EVs, including exosomes, have taken part in various pathological physiology processes and significantly contributed to MSCs (102). It has been reported that EVs derived from MSC carried a large number of regulatory factors, such as active protein, miRNA, and lncRNA (103). EVs play a key role in several biological processes that activate downstream target cells through a paracrine effect (104). Like MSCs, researchers have discovered that EVs derived from MSCs could modulate the inflammatory response, accelerate cell proliferation, promote angiogenesis, and regulate ECM remodeling during wound healing (105).
In the early stage, exosome derived from MSCs (MSCs-exo) helps monocytes translate into M1 macrophages through many immunomodulatory proteins released, such as tumor necrosis factor-alpha (TNF-α), macrophage colony-stimulating factor (MCSF) and retinol-binding protein 4(RBP-4) (106). The expression level of oxidative stress-related proteins and inflammatory cytokines is reduced (69). MSC-EVs upregulate the expression of monocyte chemoattractant protein-1and macrophage inflammatory protein-1α reduces early inflammation and oxidative stress (69, 107). In the proliferation stage, MSC-EVs are internalized by fibroblasts as well as epidermal keratinocytes and promote cell migration and proliferation by expression of N-cadherin, cyclin 1, proliferating cell nuclear antigen, collagen type I and III (22). Besides, MSC-EVs are enriched in vascular endothelial growth factor A (VEGF-A), platelet-derived growth factor BB (PDGF-BB), and noncoding RNAs, which promote proliferation and angiogenesis of vascular endothelial cells (19, 108). In the late stage, MSC-EVs prevented fibroblast-to-myofibroblast differentiation by increasing the ratio of collagen III to collagen I and the ratio of TGF-β3 to TGF-β1 to reduce scar formation (109). Therefore, MSC-EVs have become a hot topic in the field of skin wound regeneration as a cell-free therapy.
Three-dimensional (3D) bioprinting
Inspired by traditional inkjet printer technology, Thomas Boland directly printed viable mammalian cells onto hydrogel-bases papers with a cell printer, successfully exploring the field of 3D bioprinting technology used in tissue engineering (110). Bioink is a biomaterial composed of living cells, biological factors, and biological glue (111). With sufficient mechanical properties and biocompatibility, bioink can provide a stable and harmless environment of proliferation and differentiation for cells (112). Depending on the type of cell deposition, 3D bioprinting technology can be classified into three main strategies: drop-based, filament-based, and plane-based (113) (Figure 2).
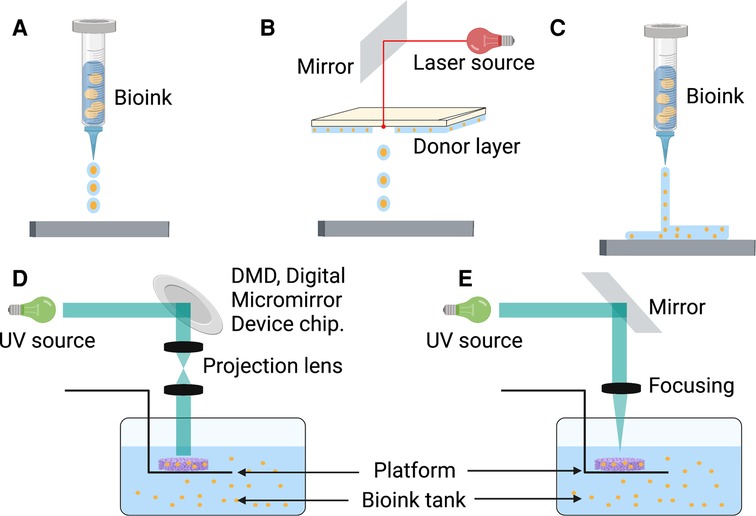
Figure 2. There are multiple strategies of 3D bioprinting, including inkjet bioprinting (A), laser bioprinting (B), extrusion-based bioprinting (C), SLA-based bioprinting (D), and DLP-based bioprinting (E). DLP, digital light processing; SLA, stereolithography.
Drop-based bioprinting
Inkjet or laser bioprinting are two commonly used strategies of drop-based 3D bioprinting. In inkjet-based bioprinting, inkjet bioprinters utilize heat or mechanical compression to create and eject drops. In this bioprinting process, various volumes of ink drops are created based on computer control, in which each drop of bioink contains 104–304 cells (114). Laser-based bioprinting utilizes the laser-induced forward transfer (LIFT) effect to print different biomaterials and living cells (115). During the process, the incident laser light causes the ejection of bioink droplets, which are subsequently received on a receiving substrate (116, 117). It has been reported that inkjet printing can be combined with gene delivery to effectively control stromal cell differentiation while bioprinting neural stromal cells (NSCs) (118). Sorkio et al. used laser-based bioprinting to demonstrate tissues mimicking the structure of the corneal tissue with stromal cells (119). A separate study indicated that MSCs could keep the predefined structure and maintain cell competency for tissue repair (120). Inkjet and laser bioprinting allow the printing of cells, materials, and protein molecules rapidly and inexpensively. However, the smooth printing process would frequently disrupt by the clogging of nozzles because of bioink gelation and the unequal-sized drops in inkjet bioprinting (121). Meanwhile, long-fabrication times and gravitational settling of cells in solution are other challenges in drop-based bioprinting (122).
Filament-based bioprinting
Extrusion-based bioprinting is the most popular approach in the research of filament-based bioprinting strategy. Based on mechanical driven force (displacement driven) or pneumatic driven force (pressure driven), extrusion bioprinting mechanisms can directly dispense the higher viscosity bioinks out of the biomaterial cartridge (123). During the development of extrusion-based bioprinting, the scaffold of acellular polymers, such as polycaprolactone (PCL), polylactic acid (PLA), and poly lactic-co-glycolic acid (PLGA), was used for 3D cell culture by extrusion printing (124–126). With the different applications of bioinert hydrogel materials such as sodium alginate, extrusion bioprinting can print bioinks of living cells to a particular flow out as seamless circular cylindrical filaments with computer manufacturing (127, 128). Extrusion-based bioprinting device creates a scaffold of adipose-derived mesenchymal stromal cells (ADSCs) in alginate-gelatin (Alg-Gel) hydrogel for tissue repair and regeneration (129). Moreover, this technology can provide a kidney organoid with highly reproducible cell numbers and viability (130). Extrusion-based bioprinting brings a promising future of producing rapid and high-throughput organoids for drug screening, disease modeling, and tissue repair. However, rapid speed and high pressure can enhance shear stress, which decreases cell viability (131). The current research focuses on extrusion bioprinting study intensively on cell-instructive hydrogels as bioinks to provide a cell-friendly microenvironment (132).
Plane-based bioprinting
Compared with other 3D bioprinting strategies, plane-based bioprinting technology, such as digital light processing (DLP) and stereolithography (SLA) bioprinting, has significant advantages in efficiency, printing resolution, and working conditions (133). DLP-based bioprinting employs projection light to biomaterials to obtain the predesigned structures. In contrast, SLA-based bioprinting achieves photo polymerization by a light pencil scanning the surface of liquid bioink (133, 134). With light-based techniques, DLP/SLA-based bioprinting can print the entire layer with higher accuracy and speed (135). Through a DLP-based 3D printer, Ma et al. printed a 3D triculture hepatic model encapsulated kind of cells, including induced iPSCs and ADSCs. The microstructure can promote maturation and maintain the functions of cells (136). With SLA-based bioprinting technology, the 3D cell-laden hydrogel scaffolds represent high cell viability and cell adhesion (137). Although this technique has shown the characteristics of high precision, fast speed, and mild condition, its running cost, and the lack of compatible bioinks limit its broader applicability (138).
3D-bioprinted MSCs in skin tissue regeneration
Traditional 2D cell cultures cannot recreate the native three-dimensional (3D) cell microenvironment, which provides cell–matrix and cell–cell interactions that readjust cell morphology and gene expression (139–141). 3D bioprinting is a promising biofabrication strategy, using living cells and biomaterials as bioink to create artificial multicellular tissues (142). In the field of skin tissue repair, 3D bioprinting is currently being explored in developing more complex synthetic skin models (143, 144). Nowadays, various biomaterials have been widely investigated as scaffolds for bioprinting in tissue engineering and skin wound healing (145, 146). Usually, hydrogel containing FBs, keratinocytes (KCs), and HUVECs are bioprinted as scaffolds directly applied on the wound bed (147). Jin et al. had taken advantage of the acellular dermal matrix (ADM) and HUVECs as bioink to 3D-bioprinted functional skin model, which maintained the ECM components to promote cell viability and form the vascular network and framework (148). Recently, 3D bioprinting has been successfully performed using multiple mesenchymal stromal cell types for tissue repair, including cardiovascular, hepatic, and skin (149). 3D-bioprinted MSCs preserved proangiogenic properties and secreted more EVs containing a greater variety of proteins (150). However, not much research focuses on adult stromal cells as seeded cells in skin wound regeneration (Table 1).
In 2010, Koch and colleagues utilized skin cell lines (fibroblasts/keratinocytes) and MSCs as bioink for skin regeneration based on laser printing. MSCs maintained the ability to proliferate and did not show an increase in apoptosis after 3D bioprinting (29). 3D-bioprinted MSCs increased angiogenesis and wound closure rates due to secretion of biological factors rather than direct cell–cell interactions, such as basic fibroblast growth factor (bFGF), and fibroblast growth factor (FGF) and growth differentiation factor (GDF) (30). Moreover, Roshangar et al. evaluated a 3D bioprinting scaffold loaded with MSCs on rat skin burn defects. Data showed that the scaffold promoted wound healing by creating a continuous epidermal layer without scar formation (151).
3D-bioprinted MSCs could improve wound healing in vivo by generating collagen and enhancing cell proliferation. Besides, 3D-bioprinted MSCs would maintain a preferable cell proliferation in the nutritionally deficient environment (11). In addition to utilizing hydrogel and MSCs for 3D bioprinting, various bioactive substances have been applied to enhance the abilities of MSCs, such as angiogenesis. A 3D-bioprinted scaffold loaded with MSCs and SNAP, which could release NO, can improve the migration and angiogenesis of HUVECs. On the other hand, the hydrogel scaffolds accelerated the serve burn wound healing by promoting epithelialization and collagen deposition (152). It has been reported that 3D-bioprinted MSCs could accelerate diabetic wound healing by combining bioink with curcumin and gelatin methacryloyl (GelMA). 3D-bioprinted MSCs with curcumin could better exert antioxidant and anti-apoptotic activity to promote wound healing (10). As widely used biological material with biocompatibility and multichannel printing technology, Turner et al. established a regenerative, dual cell delivery 3D core/shell (c/s) “living dressing” system using MSCs. It indicated that the construct provided an appropriate microenvironment to improve the proliferation and differentiation of MSCs (153). In addition, Turner et al. discovered the 3D core/shell MSCs dressing would accelerate angiogenesis and anti-inflammatory to promote wound healing in thermal injury by increasing the expression of wound healing factors and neovascularization EGF, PDGF, MMP-9, TGF-α and decreasing pro-inflammatory factor IL-6 (154).
In the last few decades, new materials and technologies have been developed to fabricate skin substitutes (31). Compared to conventional tissue engineering technologies, 3D bioprinting can deposit different cell types and specific biomaterials with a high spatial resolution (155). In 2011, Gruene et al. utilized endothelial colony-forming cells (ECFCs) and ADSCs to build a layer-by-layer scaffold using laser-assisted bioprinting and discovered direct cell–cell contacts, which may promote angiogenesis (156). Skin tissue contains a variety of cell types. Baltazar et al. have created artificial dermis using bioink containing human foreskin dermal FBs, human ECs derived from cord blood human endothelial colony-forming cells (HECFCs), and human placental pericytes (PCs) suspended in rat tail type I collagen and printed epidermis with human foreskin KCs. The result showed the 3D bioprinting of artificial dermis enhanced the formation of microvessels and the epidermal rete in vivo (28). Another research used endothelial progenitor cells (EPCs) and MSCs to 3D bioprint a full-thickness skin model. This model accelerated re-epithelization, wound closure, and neovascularization (157). For skin appendages, 3D-bioprinted MSCs have been confirmed could enhance stemness maintenance by sweat gland (SG) lineage in vitro (158). With 3D bioprinting, alginate-gelatin and epidermal progenitors could enhance sweat gland regeneration (159). Through bioprinting an SG-like matrix, MSCs could differentiate into functional SGs and facilitate SGs recovery in mice (160). Despite MSCs and 3D bioprinting technology showing great potential for preparing artificial skin, future research should concentrate on skin tissue bioprinting and make it more adaptable to clinical needs.
Discussion
The objective of skin tissue regeneration is to realize structural and functional reconstruction, at the same time promoting wound healing and reducing scar formation (161). MSCs have been considered to have a promising potential in skin tissue regeneration with their differentiation abilities and paracrine effect (15). Many studies have shown that MSCs could regulate inflammation, promote cell proliferation and migration, and improve angiogenesis during skin wound healing (15). However, MSCs therapy still has obstacles, such as its low frequency in tissues and the limited proliferative potential (162). Recently, studies have focused on the therapeutic potential of EVs derived from MSCs in skin wound regeneration. MSC-EVs and exosomes are considered to affect skin wound healing significantly. Unlike MSC-based therapy, MSC-EVs therapy has advantages in delivery and storage, as well as a lack of endogenous tumor-formation potential (163). One of the most significant advantages of MSC-EVs therapy is the possibility to inject EVs locally, thus minimizing the side effects of cell administration (102). Nevertheless, clinical applications of MSC-EVs require a long-term study on safety to prevent the development of uncontrolled immunosuppression in MSC-EVs recipients (164). Although evidence suggests the therapeutic potential of MSCs and MSC-EVs, there are still further studies to be done before MSCs and MSC-EVs could be offered as a common clinical therapy for skin wound healing.
On the other hand, with the application of 3D bioprinting, MSCs have more ability to proliferate and secrete biological factors to enhance cell–cell interaction. As 3D bioprinting technology developed, in situ bioprinting or “in vivo” bioprinting has been applied to tissue regeneration, including skin, cartilage, and bone (165). It's reported that autologous dermal fibroblasts and epidermal keratinocytes, along with the fibrinogen-collagen hydrogel, were directly printed into the wound of a porcine model, with better re-epithelialization and reducing the healing time (147). Besides, through cellular self-assembly bioprinting, upregulated expression of tissue-specific functional genes indicated increased tissue functionality to realize multitissue organs-on-a-chip with different cell types (166). There is substantial evidence supporting various methods of MSCs culture influence the release of EVs, while little research concerned EVs derived from 3D-bioprinted MSCs (167). According to a recent study, Chen et al. combined 3D core–shell bioprinting and MSCs to increase MSC-derived EVs’ production (150). In his platform, the 3D-bioprinted MSCs enriched particles by ∼1,009-fold compared to traditional 2D culture, expressing higher stemness markers and preserving proangiogenic properties. Moreover, 3D-EVs contained hundreds of unique protein profiles compared to 2D-EVs. Supported by 3D bioprinting technology, the bioprinted 3D structures loaded with EVs recapitulated the blood-perfused microvessels with a new functional vasculature in situ (168). Besides, Bari had found that 3D-bioprinted MSC-EVs could release the secretome from the scaffold with a fast speed for tissue regeneration (169). Moreover, the release was governed by the scaffold shape and the application of different biomaterials, for example, increasing alginate concentration or cross-linking with protamine. Technological developments may present new opportunities and challenges for MSCs in skin tissue regeneration.
Although MSCs and 3D bioprinting technology bring a new therapeutic strategy for skin wound healing, some obstacles still need to be overcome. The skin is one of the most vital organs as a protective barrier against various external agents (170). At present, the mechanical strength of 3D bioprinting hydrogels is insufficient, which could not be satisfied with the unique physical and mechanical characteristics of human skin. In addition, the skin contains various appendages, such as sweat glands, adipose glands, hair follicles, and blood vessels, along with nerve endings (171). Despite the sweat gland regeneration function of 3D bioprinting MSCs, 3D bioprinting skin tissue could not simulate every structure or functional reconstruction, especially nerve regeneration.
After all, 3D-bioprinted MSCs have played a positive role in skin wound healing. With the progress of related technologies and the application of new biomaterials, 3D bioprinting will hopefully overcome the difficulties mentioned above and make a big difference in skin tissue regeneration (Figure 3).
Conclusion
Wound healing and skin tissue regeneration have been critical clinical issues for decades. Various methods have been used to promote skin wound healing by better regulating every phase of wound healing. As depicted in the published studies, 3D-bioprinted MSCs are a promising therapeutic strategy for skin tissue regeneration because of their preferable differentiation and paracrine effect of biological factors. However, interdisciplinary collaboration is still needed to overcome the difficulties, such as the mechanical strength and skin appendages of bioprinted MSCs. In conclusion, 3D-bioprinted MSCs have been proven to have a positive role in skin tissue regeneration. Further studies are needed to assess the long-term outcomes and well-designed clinical studies to apply this strategy in clinical medicine.
Author contributions
YL and XX wrote the original draft. ZY and QX reviewed and edited the manuscript. JL and NL supervised the data. YD and YL critically reviewed and revised the manuscript. All authors contributed to the article and approved the submitted version.
Funding
This review was funded by the Basic and Applied Basic Research Foundation of Guangdong Province, China (No. 2021A1515220120).
Acknowledgments
The Figure was created in the web application BioRender.com (Available online: Biorender.com, accessed on 26 June 2022).
Conflict of interest
The authors declare that the research was conducted in the absence of any commercial or financial relationships that could be construed as a potential conflict of interest.
Publisher's note
All claims expressed in this article are solely those of the authors and do not necessarily represent those of their affiliated organizations, or those of the publisher, the editors and the reviewers. Any product that may be evaluated in this article, or claim that may be made by its manufacturer, is not guaranteed or endorsed by the publisher.
Abbreviations
MSCs, mesenchymal stromal cells; 3D, three-dimensional; ECM, extracellular matrix; ESCs, embryonic stromal cells; iPSCs, induced pluripotent stromal cells; dECM, decellularized extracellular matrix; FBs, fibroblasts; ECs, endothelial cells; HDFs, human dermal fibroblasts; BMSCs, bone marrow stromal cells; ADSCs, adipose-derived stromal cells; HUC-MSCs, human umbilical cord-derived mesenchymal stromal cells; DFU, diabetic foot ulcers; HUVECs, human umbilical vein endothelial cells; LIFT, laser-induced forward transfer; NSCs, neural stromal cells; PCL, polycaprolactone; PLA, polylactic acid; PLGA, poly lactic-co-glycolic acid; Alg-Gel, alginate-gelatin; DLP, digital light processing; SLA, stereolithography; EVs, extracellular vehicles; GelMA, gelatin methacryloyl; ECFCs, endothelial colony-forming cells; KCs, keratinocytes; EPCs, endothelial progenitor cells; SG, sweat gland.
References
1. Karimkhani C, Dellavalle RP, Coffeng LE, Flohr C, Hay RJ, Langan SM, et al. Global skin disease morbidity and mortality: an update from the global burden of disease study 2013. JAMA Dermatol. (2017) 153(5):406–12. doi: 10.1001/jamadermatol.2016.5538
2. Werner S, Krieg T, Smola H. Keratinocyte-fibroblast interactions in wound healing. J Invest Dermatol. (2007) 127(5):998–1008. doi: 10.1038/sj.jid.5700786
3. Richardson R, Slanchev K, Kraus C, Knyphausen P, Eming S, Hammerschmidt M. Adult zebrafish as a model system for cutaneous wound-healing research. J Invest Dermatol. (2013) 133(6):1655–65. doi: 10.1038/jid.2013.16
4. Velnar T, Bailey T, Smrkolj V. The wound healing process: an overview of the cellular and molecular mechanisms. J Int Med Res. (2009) 37(5):1528–42. doi: 10.1177/147323000903700531
5. Lawrence WT. Physiology of the acute wound. Clin Plast Surg. (1998) 25(3):321–40. doi: 10.1016/S0094-1298(20)32467-6
6. Eming SA, Krieg T, Davidson JM. Inflammation in wound repair: molecular and cellular mechanisms. J Invest Dermatol. (2007) 127(3):514–25. doi: 10.1038/sj.jid.5700701
7. Demidova-Rice TN, Hamblin MR, Herman IM. Acute and impaired wound healing: pathophysiology and current methods for drug delivery, part 2: role of growth factors in normal and pathological wound healing: therapeutic potential and methods of delivery. Adv Skin Wound Care. (2012) 25(8):349–70. doi: 10.1097/01.ASW.0000418541.31366.a3
8. Otero-Viñas M, Falanga V. Mesenchymal stem cells in chronic wounds: the spectrum from basic to advanced therapy. Adv Wound Care (New Rochelle). (2016) 5(4):149–63. doi: 10.1089/wound.2015.0627
9. Kim WS, Park BS, Sung JH, Yang JM, Park SB, Kwak SJ, et al. Wound healing effect of adipose-derived stem cells: a critical role of secretory factors on human dermal fibroblasts. J Dermatol Sci. (2007) 48(1):15–24. doi: 10.1016/j.jdermsci.2007.05.018
10. Xia S, Weng T, Jin R, Yang M, Yu M, Zhang W, et al. Curcumin-incorporated 3D bioprinting gelatin methacryloyl hydrogel reduces reactive oxygen species-induced adipose-derived stem cell apoptosis and improves implanting survival in diabetic wounds. Burns Trauma. (2022) 10:tkac001. doi: 10.1093/burnst/tkac001
11. Zhao H, Xu J, Zhang E, Qi R, Huang Y, Lv F, et al. 3D Bioprinting of polythiophene materials for promoting stem cell proliferation in a nutritionally deficient environment. ACS Appl Mater Interfaces. (2021) 13(22):25759–70. doi: 10.1021/acsami.1c04967
12. Steens J, Klein D. Current strategies to generate human mesenchymal stem cells in vitro. Stem Cells Int. (2018) 2018:6726185. doi: 10.1155/2018/6726185
13. Beeravolu N, McKee C, Alamri A, Mikhael S, Brown C, Perez-Cruet M, et al. Isolation and characterization of mesenchymal stromal cells from human umbilical cord and fetal placenta. J. Visualized Exp. (2017) 122:1–13. doi: 10.3791/55224
14. Isakson M, de Blacam C, Whelan D, McArdle A, Clover AJP. Mesenchymal stem cells and cutaneous wound healing: current evidence and future potential. Stem Cells Int. (2015) 2015:1–12. doi: 10.1155/2015/831095
15. Jo H, Brito S, Kwak BM, Park S, Lee MG, Bin BH. Applications of mesenchymal stem cells in skin regeneration and rejuvenation. Int J Mol Sci. (2021) 22(5):1–19. doi: 10.3390/ijms22052410
16. Kern S, Eichler H, Stoeve J, Kluter H, Bieback K. Comparative analysis of mesenchymal stem cells from bone marrow, umbilical cord blood, or adipose tissue. Stem Cells. (2006) 24(5):1294–301. doi: 10.1634/stemcells.2005-0342
17. Teng M, Huang Y, Zhang H. Application of stems cells in wound healing–an update. Wound Repair Regen. (2014) 22(2):151–60. doi: 10.1111/wrr.12152
18. Wu P, Zhang B, Shi H, Qian H, Xu W. MSC-exosome: a novel cell-free therapy for cutaneous regeneration. Cytotherapy. (2018) 20(3):291–301. doi: 10.1016/j.jcyt.2017.11.002
19. Liang X, Zhang L, Wang S, Han Q, Zhao RC. Exosomes secreted by mesenchymal stem cells promote endothelial cell angiogenesis by transferring miR-125a. J Cell Sci. (2016) 129(11):2182–9. doi: 10.1242/jcs.170373
20. Kang T, Jones TM, Naddell C, Bacanamwo M, Calvert JW, Thompson WE, et al. Adipose-derived stem cells induce angiogenesis via microvesicle transport of miRNA-31. Stem Cells Transl Med. (2016) 5(4):440–50. doi: 10.5966/sctm.2015-0177
21. Choi EW, Seo MK, Woo EY, Kim SH, Park EJ, Kim S. Exosomes from human adipose-derived stem cells promote proliferation and migration of skin fibroblasts. Exp Dermatol. (2017) 27(10):1170–2. doi: 10.5966/sctm.2015-0177
22. Hu L, Wang J, Zhou X, Xiong Z, Zhao J, Yu R, et al. Exosomes derived from human adipose mensenchymal stem cells accelerates cutaneous wound healing via optimizing the characteristics of fibroblasts. Sci Rep. (2016) 6(1):1–11. doi: 10.1038/srep32993
23. Liu J, Sun L, Xu W, Wang Q, Yu S, Sun J. Current advances and future perspectives of 3D printing natural-derived biopolymers. Carbohydr Polym. (2019) 207:297–316. doi: 10.1016/j.carbpol.2018.11.077
24. Liu N, Ye X, Yao B, Zhao M, Wu P, Liu G, et al. Advances in 3D bioprinting technology for cardiac tissue engineering and regeneration. Bioact Mater. (2021) 6(5):1388–401. doi: 10.1016/j.bioactmat.2020.10.021
25. Koçak E, Yıldız A, Acartürk F. Three dimensional bioprinting technology: applications in pharmaceutical and biomedical area. Colloids Surf B Biointerfaces. (2021) 197:111396. doi: 10.1016/j.colsurfb.2020.111396
26. Osidak EO, Karalkin PA, Osidak MS, Parfenov VA, Sivogrivov DE, Pereira F, et al. Viscoll collagen solution as a novel bioink for direct 3D bioprinting. J Mater Sci Mater Med. (2019) 30(3):31. doi: 10.1007/s10856-019-6233-y
27. Won JY, Lee MH, Kim MJ, Min KH, Ahn G, Han JS, et al. A potential dermal substitute using decellularized dermis extracellular matrix derived bio-ink. Artif Cells Nanomed Biotechnol. (2019) 47(1):644–9. doi: 10.1080/21691401.2019.1575842
28. Baltazar T, Merola J, Catarino C, Xie CB, Kirkiles-Smith NC, Lee V, et al. Three dimensional bioprinting of a vascularized and perfusable skin graft using human keratinocytes, fibroblasts, pericytes, and endothelial cells. Tissue Eng Part A. (2020) 26(5-6):227–38. doi: 10.1089/ten.tea.2019.0201
29. Koch L, Kuhn S, Sorg H, Gruene M, Schlie S, Gaebel R, et al. Laser printing of skin cells and human stem cells. Tissue Eng Part C. (2010) 16(5):847–54. doi: 10.1089/ten.tec.2009.0397
30. Skardal A, Mack D, Kapetanovic E, Atala A, Jackson JD, Yoo J, et al. Bioprinted amniotic fluid-derived stem cells accelerate healing of large skin wounds. Stem Cells Transl Med. (2012) 1(11):792–802. doi: 10.5966/sctm.2012-0088
31. Tottoli EM, Dorati R, Genta I, Chiesa E, Pisani S, Conti B. Skin wound healing process and new emerging technologies for skin wound care and regeneration. Pharmaceutics. (2020) 12(8):1–30. doi: 10.3390/pharmaceutics12080735
32. Gurtner GC, Werner S, Barrandon Y, Longaker MT. Wound repair and regeneration. Nature. (2008) 453(7193):314–21. doi: 10.1038/nature07039
33. Grose R, Werner S. Wound-healing studies in transgenic and knockout mice. Mol Biotechnol. (2004) 28(2):147–66. doi: 10.1385/MB:28:2:147
34. Opalenik SR, Davidson JM. Fibroblast differentiation of bone marrow-derived cells during wound repair. FASEB J. (2005) 19(11):1561–3. doi: 10.1096/fj.04-2978fje
35. Szabowski A, Maas-Szabowski N, Andrecht S, Kolbus A, Schorpp-Kistner M, Fusenig NE, et al. c-Jun and JunB antagonistically control cytokine-regulated mesenchymal-epidermal interaction in skin. Cell. (2000) 103(5):745–55. doi: 10.1016/S0092-8674(00)00178-1
36. Baum CL, Arpey CJ. Normal cutaneous wound healing: clinical correlation with cellular and molecular events. Dermatol Surg. (2005) 31(6):674–86, discussion 686. doi: 10.1097/00042728-200506000-00011
37. Martin P, Leibovich SJ. Inflammatory cells during wound repair: the good, the bad and the ugly. Trends Cell Biol. (2005) 15(11):599–607. doi: 10.1016/j.tcb.2005.09.002
38. Markova A, Mostow EN. US skin disease assessment: ulcer and wound care. Dermatol Clin. (2012) 30(1):107–11, ix. doi: 10.1016/j.det.2011.08.005
39. Medina A, Scott PG, Ghahary A, Tredget EE. Pathophysiology of chronic nonhealing wounds. J Burn Care Rehabil. (2005) 26(4):306–19. doi: 10.1097/01.BCR.0000169887.04973.3A
40. Van De Water L, Varney S, Tomasek JJ. Mechanoregulation of the myofibroblast in wound contraction, scarring, and fibrosis: opportunities for new therapeutic intervention. Adv Wound Care (New Rochelle). (2013) 2(4):122–41. doi: 10.1089/wound.2012.0393
41. Levenson SM, Geever EF, Crowley LV, Oates JF, Berard CW, Rosen H. The healing of rat skin wounds. Ann Surg. (1965) 161(2):293–308. doi: 10.1097/00000658-196502000-00019
42. Aarabi S, Longaker MT, Gurtner GC. Hypertrophic scar formation following burns and trauma: new approaches to treatment. PLoS Med. (2007) 4(9):e234. doi: 10.1371/journal.pmed.0040234
43. Behr B, Ko SH, Wong VW, Gurtner GC, Longaker MT. Stem cells. Plast Reconstr Surg. (2010) 126(4):1163–71. doi: 10.1097/PRS.0b013e3181ea42bb
44. Biancone L, Bruno S, Deregibus MC, Tetta C, Camussi G. Therapeutic potential of mesenchymal stem cell-derived microvesicles. Nephrol Dial Transplant. (2012) 27(8):3037–42. doi: 10.1093/ndt/gfs168
45. Chen M, Przyborowski M, Berthiaume F. Stem cells for skin tissue engineering and wound healing. Crit Rev Biomed Eng. (2009) 37(4-5):399–421. doi: 10.1615/CritRevBiomedEng.v37.i4-5.50
46. An Y, Lin S, Tan X, Zhu S, Nie F, Zhen Y, et al. Exosomes from adipose-derived stem cells and application to skin wound healing. Cell Prolif. (2021) 54(3):e12993. doi: 10.1111/cpr.12993
47. Friedenstein AJ, Petrakova KV, Kurolesova AI, Frolova GP. Heterotopic of bone marrow. Analysis of precursor cells for osteogenic and hematopoietic tissues. Transplantation. (1968) 6(2):230–47. doi: 10.1097/00007890-196803000-00009
48. Sinno H, Prakash S. Complements and the wound healing cascade: an updated review. Plast Surg Int. (2013) 2013:146764. doi: 10.1155/2013/146764
49. Vestweber D. How leukocytes cross the vascular endothelium. Nat Rev Immunol. (2015) 15(11):692–704. doi: 10.1038/nri3908
50. Takeo M, Lee W, Ito M. Wound healing and skin regeneration. Cold Spring Harbor Perspect Med. (2015) 5(1):a023267. doi: 10.1101/cshperspect.a023267
51. Wang Y, Chen X, Cao W, Shi Y. Plasticity of mesenchymal stem cells in immunomodulation: pathological and therapeutic implications. Nat Immunol. (2014) 15(11):1009–16. doi: 10.1038/ni.3002
52. Aboalola D, Han VKM. Different effects of insulin-like growth factor-1 and insulin-like growth factor-2 on myogenic differentiation of human mesenchymal stem cells. Stem Cells Int. (2017) 2017:8286248. doi: 10.1155/2017/8286248
53. Chiossone L, Conte R, Spaggiari GM, Serra M, Romei C, Bellora F, et al. Mesenchymal stromal cells induce peculiar alternatively activated macrophages capable of dampening both innate and adaptive immune responses. Stem Cells. (2016) 34(7):1909–21. doi: 10.1002/stem.2369
54. Liu F, Qiu H, Xue M, Zhang S, Zhang X, Xu J, et al. MSC-secreted TGF-β regulates lipopolysaccharide-stimulated macrophage M2-like polarization via the Akt/FoxO1 pathway. Stem Cell Res Ther. (2019) 10(1):345. doi: 10.1186/s13287-019-1447-y
55. Babazadeh S, Nassiri SM, Siavashi V, Sahlabadi M, Hajinasrollah M, Zamani-Ahmadmahmudi M. Macrophage polarization by MSC-derived CXCL12 determines tumor growth. Cell Mol Biol Lett. (2021) 26(1):30. doi: 10.1186/s11658-021-00273-w
56. Zhao Y, Zhu XY, Song T, Zhang L, Eirin A, Conley S, et al. Mesenchymal stem cells protect renal tubular cells via TSG-6 regulating macrophage function and phenotype switching. Am J Physiol Renal Physiol. (2021) 320(3):F454–63. doi: 10.1152/ajprenal.00426.2020
57. Song JY, Kang HJ, Hong JS, Kim CJ, Shim JY, Lee CW, et al. Umbilical cord-derived mesenchymal stem cell extracts reduce colitis in mice by re-polarizing intestinal macrophages. Sci Rep. (2017) 7(1):9412. doi: 10.1038/s41598-017-09827-5
58. He X, Dong Z, Cao Y, Wang H, Liu S, Liao L, et al. MSC-derived exosome promotes M2 polarization and enhances cutaneous wound healing. Stem Cells Int. (2019) 2019:7132708. doi: 10.1155/2019
59. Regmi S, Pathak S, Kim JO, Yong CS, Jeong JH. Mesenchymal stem cell therapy for the treatment of inflammatory diseases: challenges, opportunities, and future perspectives. Eur J Cell Biol. (2019) 98(5-8):151041. doi: 10.1016/j.ejcb.2019.04.002
60. Mo Y, Kang H, Bang JY, Shin JW, Kim HY, Cho SH, et al. Intratracheal administration of mesenchymal stem cells modulates lung macrophage polarization and exerts anti-asthmatic effects. Sci Rep. (2022) 12(1):11728. doi: 10.1038/s41598-022-14846-y
61. Goodwin M, Sueblinvong V, Eisenhauer P, Ziats NP, LeClair L, Poynter ME, et al. Bone marrow-derived mesenchymal stromal cells inhibit Th2-mediated allergic airways inflammation in mice. Stem Cells. (2011) 29(7):1137–48. doi: 10.1002/stem.656
62. Song JY, Kang HJ, Ju HM, Park A, Park H, Hong JS, et al. Umbilical cord-derived mesenchymal stem cell extracts ameliorate atopic dermatitis in mice by reducing the T cell responses. Sci Rep. (2019) 9(1):6623. doi: 10.1038/s41598-019-42964-7
63. Zhou H, Guo M, Bian C, Sun Z, Yang Z, Zeng Y, et al. Efficacy of bone marrow-derived mesenchymal stem cells in the treatment of sclerodermatous chronic graft-versus-host disease: clinical report. Biol Blood Marrow Transplant. (2010) 16(3):403–12. doi: 10.1016/j.bbmt.2009.11.006
64. Aggarwal S, Pittenger MF. Human mesenchymal stem cells modulate allogeneic immune cell responses. Blood. (2005) 105(4):1815–22. doi: 10.1182/blood-2004-04-1559
65. Okano T, Yamada N, Okuhara M, Sakai H, Sakurai Y. Mechanism of cell detachment from temperature-modulated, hydrophilic-hydrophobic polymer surfaces. Biomaterials. (1995) 16(4):297–303. doi: 10.1016/0142-9612(95)93257-E
66. Kobayashi J, Kikuchi A, Aoyagi T, Okano T. Cell sheet tissue engineering: cell sheet preparation, harvesting/manipulation, and transplantation. J Biomed Mater Res A. (2019) 107(5):955–67. doi: 10.1002/jbm.a.36627
67. Yu J, Wang MY, Tai HC, Cheng NC. Cell sheet composed of adipose-derived stem cells demonstrates enhanced skin wound healing with reduced scar formation. Acta Biomater. (2018) 77:191–200. doi: 10.1016/j.actbio.2018.07.022
68. Sun L, Huang Y, Bian Z, Petrosino J, Fan Z, Wang Y, et al. Sundew-Inspired adhesive hydrogels combined with adipose-derived stem cells for wound healing. ACS Appl Mater Interfaces. (2016) 8(3):2423–34. doi: 10.1021/acsami.5b11811
69. Li X, Xie X, Lian W, Shi R, Han S, Zhang H, et al. Exosomes from adipose-derived stem cells overexpressing Nrf2 accelerate cutaneous wound healing by promoting vascularization in a diabetic foot ulcer rat model. Exp Mol Med. (2018) 50(4):1–14. doi: 10.1038/s12276-018-0058-5
70. Wang W, Du Z, Yan J, Ma D, Shi M, Zhang M, et al. Mesenchymal stem cells promote liver regeneration and prolong survival in small-for-size liver grafts: involvement of C-jun N-terminal kinase, cyclin D1, and NF-κB. PloS One. (2014) 9(12):e112532. doi: 10.1371/journal.pone.0112532
71. Martin P. Wound healing—aiming for perfect skin regeneration. Science. (1997) 276(5309):75–81. doi: 10.1126/science.276.5309.75
72. Smith AN, Willis E, Chan VT, Muffley LA, Isik FF, Gibran NS, et al. Mesenchymal stem cells induce dermal fibroblast responses to injury. Exp Cell Res. (2010) 316(1):48–54. doi: 10.1016/j.yexcr.2009.08.001
73. Yates CC, Rodrigues M, Nuschke A, Johnson ZI, Whaley D, Stolz D, et al. Multipotent stromal cells/mesenchymal stem cells and fibroblasts combine to minimize skin hypertrophic scarring. Stem Cell Res Ther. (2017) 8(1):193. doi: 10.1186/s13287-017-0644-9
74. McBride JD, Rodriguez-Menocal L, Guzman W, Candanedo A, Garcia-Contreras M, Badiavas EV. Bone marrow mesenchymal stem cell-derived CD63(+) exosomes transport Wnt3a exteriorly and enhance dermal fibroblast proliferation, migration, and angiogenesis in vitro. Stem Cells Dev. (2017) 26(19):1384–98. doi: 10.1089/scd.2017.0087
75. Zhang B, Wang M, Gong A, Zhang X, Wu X, Zhu Y, et al. HucMSC-exosome mediated-Wnt4 signaling is required for cutaneous wound healing. Stem Cells. (2015) 33(7):2158–68. doi: 10.1002/stem.1771
76. Kaita Y, Tarui T, Yoshino H, Matsuda T, Yamaguchi Y, Nakagawa T, et al. Sufficient therapeutic effect of cryopreserved frozen adipose-derived regenerative cells on burn wounds. Regener Ther. (2019) 10:92–103. doi: 10.1016/j.reth.2019.01.001
77. Strzalka W, Ziemienowicz A. Proliferating cell nuclear antigen (PCNA): a key factor in DNA replication and cell cycle regulation. Ann Bot. (2011) 107(7):1127–40. doi: 10.1093/aob/mcq243
78. Tsai WC, Cheng JW, Chen JL, Chen CY, Chang HN, Liao YH, et al. Low-level laser irradiation stimulates tenocyte proliferation in association with increased NO synthesis and upregulation of PCNA and cyclins. Lasers Med Sci. (2014) 29(4):1377–84. doi: 10.1007/s10103-014-1528-1
79. Zhou Y, Zhao B, Zhang XL, Lu YJ, Lu ST, Cheng J, et al. Combined topical and systemic administration with human adipose-derived mesenchymal stem cells (hADSC) and hADSC-derived exosomes markedly promoted cutaneous wound healing and regeneration. Stem Cell Res Ther. (2021) 12(1):257. doi: 10.1186/s13287-021-02287-9
80. Pan H, Lam PK, Tong SW, Leung KK, Teoh AY, Ng EK. Mesenchymal stem cells combined with tissue fusion technology promoted wound healing in porcine bowel anastomosis. Stem Cells Int. (2020) 2020:5142797. doi: 10.1155/2020/5142797
81. Pelizzo G, Avanzini MA, Icaro Cornaglia A, Osti M, Romano P, Avolio L, et al. Mesenchymal stromal cells for cutaneous wound healing in a rabbit model: pre-clinical study applicable in the pediatric surgical setting. J Transl Med. (2015) 13:219. doi: 10.1186/s12967-015-0580-3
82. Cases-Perera O, Blanco-Elices C, Chato-Astrain J, Miranda-Fernández C, Campos F, Crespo PV, et al. Development of secretome-based strategies to improve cell culture protocols in tissue engineering. Sci Rep. (2022) 12(1):10003. doi: 10.1038/s41598-022-14115-y
83. Hassanzadeh H, Matin MM, Naderi-Meshkin H, Bidkhori HR, Mirahmadi M, Raeesolmohaddeseen M, et al. Using paracrine effects of Ad-MSCs on keratinocyte cultivation and fabrication of epidermal sheets for improving clinical applications. Cell Tissue Bank. (2018) 19(4):531–47. doi: 10.1007/s10561-018-9702-5
84. Bellei B, Migliano E, Tedesco M, Caputo S, Papaccio F, Lopez G, et al. Adipose tissue-derived extracellular fraction characterization: biological and clinical considerations in regenerative medicine. Stem Cell Res Ther. (2018) 9(1):207. doi: 10.1186/s13287-018-0956-4
85. Guerra A, Belinha J, Jorge RN. Modelling skin wound healing angiogenesis: a review. J Theor Biol. (2018) 459:1–17. doi: 10.1016/j.jtbi.2018.09.020
86. Huang M, Huang X, Jiang B, Zhang P, Guo L, Cui X, et al. linc00174-EZH2-ZNF24/Runx1-VEGFA regulatory mechanism modulates post-burn wound healing. Mol Ther Nucleic Acids. (2020) 21:824–36. doi: 10.1016/j.omtn.2020.07.010
87. Rehman J, Traktuev D, Li J, Merfeld-Clauss S, Temm-Grove CJ, Bovenkerk JE, et al. Secretion of angiogenic and antiapoptotic factors by human adipose stromal cells. Circulation. (2004) 109(10):1292–8. doi: 10.1161/01.CIR.0000121425.42966.F1
88. Goodarzi P, Alavi-Moghadam S, Sarvari M, Tayanloo Beik A, Falahzadeh K, Aghayan H, et al. Adipose tissue-derived stromal cells for wound healing. Adv Exp Med Biol. (2018) 1119:133–49. doi: 10.1007/5584
89. Liu Y, Chen J, Liang H, Cai Y, Li X, Yan L, et al. Human umbilical cord-derived mesenchymal stem cells not only ameliorate blood glucose but also protect vascular endothelium from diabetic damage through a paracrine mechanism mediated by MAPK/ERK signaling. Stem Cell Res Ther. (2022) 13(1):258. doi: 10.1186/s13287-022-02927-8
90. Cheng NC, Lin WJ, Ling TY, Young TH. Sustained release of adipose-derived stem cells by thermosensitive chitosan/gelatin hydrogel for therapeutic angiogenesis. Acta Biomater. (2017) 51:258–67. doi: 10.1016/j.actbio.2017.01.060
91. Xue J, Sun N, Liu Y. Self-Assembled nano-peptide hydrogels with human umbilical cord mesenchymal stem cell spheroids accelerate diabetic skin wound healing by inhibiting inflammation and promoting angiogenesis. Int J Nanomed. (2022) 17:2459–74. doi: 10.2147/IJN.S363777
92. Luo Y, Yi X, Liang T, Jiang S, He R, Hu Y, et al. Autograft microskin combined with adipose-derived stem cell enhances wound healing in a full-thickness skin defect mouse model. Stem Cell Res Ther. (2019) 10(1):279. doi: 10.1186/s13287-019-1389-4
93. Schweizer R, Kamat P, Schweizer D, Dennler C, Zhang S, Schnider JT, et al. Bone marrow-derived mesenchymal stromal cells improve vascular regeneration and reduce leukocyte-endothelium activation in critical ischemic murine skin in a dose-dependent manner. Cytotherapy. (2014) 16(10):1345–60. doi: 10.1016/j.jcyt.2014.05.008
94. Pugliese E, Coentro JQ, Raghunath M, Zeugolis DI. Wound healing and scar wars. Adv Drug Delivery Rev. (2018) 129:1–3. doi: 10.1016/j.addr.2018.05.010
95. Li L, Zhang S, Zhang Y, Yu B, Xu Y, Guan Z. Paracrine action mediate the antifibrotic effect of transplanted mesenchymal stem cells in a rat model of global heart failure. Mol Biol Rep. (2009) 36(4):725–31. doi: 10.1007/s11033-008-9235-2
96. Li L, Zhang Y, Li Y, Yu B, Xu Y, Zhao S, et al. Mesenchymal stem cell transplantation attenuates cardiac fibrosis associated with isoproterenol-induced global heart failure. Transplant Int. (2008) 21(12):1181–9. doi: 10.1111/j.1432-2277.2008.00742.x
97. Wang L, Hu L, Zhou X, Xiong Z, Zhang C, Shehada HMA, et al. Exosomes secreted by human adipose mesenchymal stem cells promote scarless cutaneous repair by regulating extracellular matrix remodelling. Sci Rep. (2017) 7(1):13321. doi: 10.1038/s41598-017-12919-x
98. Yuan B, Broadbent JA, Huan J, Yang H. The effects of adipose stem cell-conditioned media on fibrogenesis of dermal fibroblasts stimulated by transforming growth factor-beta1. J Burn Care Res. (2018) 39(1):129–40. doi: 10.1097/BCR.0000000000000558
99. Hu CH, Tseng YW, Chiou CY, Lan KC, Chou CH, Tai CS, et al. Bone marrow concentrate-induced mesenchymal stem cell conditioned medium facilitates wound healing and prevents hypertrophic scar formation in a rabbit ear model. Stem Cell Res Ther. (2019) 10(1):275. doi: 10.1186/s13287-019-1383-x
100. Fang S, Xu C, Zhang Y, Xue C, Yang C, Bi H, et al. Umbilical cord-derived mesenchymal stem cell-derived exosomal microRNAs suppress myofibroblast differentiation by inhibiting the transforming growth factor-β/SMAD2 pathway during wound healing. Stem Cells Transl Med. (2016) 5(10):1425–39. doi: 10.5966/sctm.2015-0367
101. Trounson A, McDonald C. Stem cell therapies in clinical trials: progress and challenges. Cell Stem Cell. (2015) 17(1):11–22. doi: 10.1016/j.stem.2015.06.007
102. Taverna S, Pucci M, Alessandro R. Extracellular vesicles: small bricks for tissue repair/regeneration. Ann Transl Med. (2017) 5(4):83. doi: 10.21037/atm.2017.01.53
103. Harrell CR, Jovicic N, Djonov V, Arsenijevic N, Volarevic V. Mesenchymal stem cell-derived exosomes and other extracellular vesicles as new remedies in the therapy of inflammatory diseases. Cells. (2019) 8(12):1–22. doi: 10.3390/cells8121605
104. Mathivanan S, Ji H, Simpson RJ. Exosomes: extracellular organelles important in intercellular communication. J Proteomics. (2010) 73(10):1907–20. doi: 10.1016/j.jprot.2010.06.006
105. Casado-Díaz A, Quesada-Gómez JM, Dorado G. Extracellular vesicles derived from mesenchymal stem cells (MSC) in regenerative medicine: applications in skin wound healing. Front Bioeng Biotechnol. (2020) 8:146. doi: 10.3389/fbioe.2020.00146
106. Kranendonk MEG, Visseren FLJ, van Balkom BWM, Nolte't Hoen ENM, van Herwaarden JA, de Jager W, et al. Human adipocyte extracellular vesicles in reciprocal signaling between adipocytes and macrophages. Obesity. (2014) 22(5):1296–308. doi: 10.1002/oby.20679
107. Chen B, Cai J, Wei Y, Jiang Z, Desjardins HE, Adams AE, et al. Exosomes are comparable to source adipose stem cells in fat graft retention with up-regulating early inflammation and angiogenesis. Plast Reconstr Surg. (2019) 144(5):816e–27e. doi: 10.1097/PRS.0000000000006175
108. Hoang DH, Nguyen TD, Nguyen HP, Nguyen XH, Do PTX, Dang VD, et al. Differential wound healing capacity of mesenchymal stem cell-derived exosomes originated from bone marrow, adipose tissue and umbilical cord under serum- and xeno-free condition. Front Mol Biosci. (2020) 7:119. doi: 10.3389/fmolb.2020.00119
109. Choi EW, Seo MK, Woo EY, Kim SH, Park EJ, Kim S. Exosomes from human adipose-derived stem cells promote proliferation and migration of skin fibroblasts. Exp Dermatol. (2018) 27(10):1170–2. doi: 10.1111/exd.13451
110. Xu T, Jin J, Gregory C, Hickman JJ, Boland T. Inkjet printing of viable mammalian cells. Biomaterials. (2005) 26(1):93–9. doi: 10.1016/j.biomaterials.2004.04.011
111. Gao C, Lu C, Jian Z, Zhang T, Chen Z, Zhu Q, et al. 3D bioprinting for fabricating artificial skin tissue. Colloids Surf B Biointerfaces. (2021) 208:112041. doi: 10.1016/j.colsurfb.2021.112041
112. Faulkner-Jones A, Fyfe C, Cornelissen DJ, Gardner J, King J, Courtney A, et al.. Bioprinting of human pluripotent stem cells and their directed differentiation into hepatocyte-like cells for the generation of mini-livers in 3D. Biofabrication. (2015) 7(4):044102. doi: 10.1088/1758-5090/7/4/044102
113. Germain N, Dhayer M, Dekiouk S, Marchetti P. Current advances in 3D bioprinting for cancer modeling and personalized medicine. Int J Mol Sci. (2022) 23(7):1–31. doi: 10.3390/ijms23073432
114. Zhu W, Ma X, Gou M, Mei D, Zhang K, Chen S. 3D Printing of functional biomaterials for tissue engineering. Curr Opin Biotechnol. (2016) 40:103–12. doi: 10.1016/j.copbio.2016.03.014
115. Schiele NR, Corr DT, Huang Y, Raof NA, Xie Y, Chrisey DB. Laser-based direct-write techniques for cell printing. Biofabrication. (2010) 2(3):032001. doi: 10.1088/1758-5082/2/3/032001
116. Guillemot F, Souquet A, Catros S, Guillotin B. Laser-assisted cell printing: principle, physical parameters versus cell fate and perspectives in tissue engineering. Nanomedicine. (2010) 5(3):507–15. doi: 10.2217/nnm.10.14
117. Ringeisen BR, Othon CM, Barron JA, Young D, Spargo BJ. Jet-based methods to print living cells. Biotechnol J. (2006) 1(9):930–48. doi: 10.1002/biot.200600058
118. Ilkhanizadeh S, Teixeira AI, Hermanson O. Inkjet printing of macromolecules on hydrogels to steer neural stem cell differentiation. Biomaterials. (2007) 28(27):3936–43. doi: 10.1016/j.biomaterials.2007.05.018
119. Sorkio A, Koch L, Koivusalo L, Deiwick A, Miettinen S, Chichkov B, et al. Human stem cell based corneal tissue mimicking structures using laser-assisted 3D bioprinting and functional bioinks. Biomaterials. (2018) 171:57–71. doi: 10.1016/j.biomaterials.2018.04.034
120. Gruene M, Deiwick A, Koch L, Schlie S, Unger C, Hofmann N, et al. Laser printing of stem cells for biofabrication of scaffold-free autologous grafts. Tissue Eng Part C Methods. (2011) 17(1):79–87. doi: 10.1089/ten.tec.2010.0359
121. Gao Q, He Y, Fu JZ, Liu A, Ma L. Coaxial nozzle-assisted 3D bioprinting with built-in microchannels for nutrients delivery. Biomaterials. (2015) 61:203–15. doi: 10.1016/j.biomaterials.2015.05.031
122. Dinca V, Kasotakis E, Catherine J, Mourka A, Ranella A, Ovsianikov A, et al. Directed three-dimensional patterning of self-assembled peptide fibrils. Nano Lett. (2008) 8(2):538–43. doi: 10.1021/nl072798r
123. Gao G, Kim BS, Jang J, Cho DW Recent strategies in extrusion-based three-dimensional cell printing toward organ biofabrication. ACS Biomater Sci Eng. (2019) 5(3):1150–69. doi: 10.1021/acsbiomaterials.8b00691
124. Buyuksungur S, Endogan Tanir T, Buyuksungur A, Bektas EI, Torun Kose G, Yucel D, et al. 3D Printed poly(ε-caprolactone) scaffolds modified with hydroxyapatite and poly(propylene fumarate) and their effects on the healing of rabbit femur defects. Biomater Sci. (2017) 5(10):2144–58. doi: 10.1039/C7BM00514H
125. Sherwood JK, Riley SL, Palazzolo R, Brown SC, Monkhouse DC, Coates M, et al. A three-dimensional osteochondral composite scaffold for articular cartilage repair. Biomaterials. (2002) 23(24):4739–51. doi: 10.1016/S0142-9612(02)00223-5
126. Das P, DiVito MD, Wertheim JA, Tan LP. Collagen-I and fibronectin modified three-dimensional electrospun PLGA scaffolds for long-term in vitro maintenance of functional hepatocytes. Mater Sci Eng C Mater Biol Appl. (2020) 111:110723. doi: 10.1016/j.msec.2020.110723
127. Knowlton S, Anand S, Shah T, Tasoglu S. Bioprinting for neural tissue engineering. Trends Neurosci. (2018) 41(1):31–46. doi: 10.1016/j.tins.2017.11.001
128. Lee H, Ahn S, Bonassar LJ, Kim G. Cell(MC3T3-E1)-printed poly(ɛ-caprolactone)/alginate hybrid scaffolds for tissue regeneration. Macromol Rapid Commun. (2013) 34(2):142–9. doi: 10.1002/marc.201200524
129. Kolan KCR, Semon JA, Bromet B, Day DE, Leu MC. Bioprinting with human stem cell-laden alginate-gelatin bioink and bioactive glass for tissue engineering. Int J Bioprint. (2019) 5(2.2):204. doi: 10.18063/ijb.v5i2.2.204
130. Lawlor KT, Vanslambrouck JM, Higgins JW, Chambon A, Bishard K, Arndt D, et al. Cellular extrusion bioprinting improves kidney organoid reproducibility and conformation. Nat Mater. (2021) 20(2):260–71. doi: 10.1038/s41563-020-00853-9
131. Chang R, Nam J, Sun W. Effects of dispensing pressure and nozzle diameter on cell survival from solid freeform fabrication-based direct cell writing. Tissue Eng Part A. (2008) 14(1):41–8. doi: 10.1089/ten.a.2007.0004
132. Ji S, Guvendiren M. Recent advances in bioink design for 3D bioprinting of tissues and organs. Front Bioeng Biotechnol. (2017) 5:23. doi: 10.3389/fbioe.2017.00023
133. Lu Y, Mapili G, Suhali G, Chen S, Roy K. A digital micro-mirror device-based system for the microfabrication of complex, spatially patterned tissue engineering scaffolds. J Biomed Mater Res A. (2006) 77(2):396–405. doi: 10.1002/jbm.a.30601
134. Xu C, Chai W, Huang Y, Markwald RR. Scaffold-free inkjet printing of three-dimensional zigzag cellular tubes. Biotechnol Bioeng. (2012) 109(12):3152–60. doi: 10.1002/bit.24591
135. Leberfinger AN, Ravnic DJ, Dhawan A, Ozbolat IT. Concise review: bioprinting of stem cells for transplantable tissue fabrication. Stem Cells Transl Med. (2017) 6(10):1940–8. doi: 10.1002/sctm.17-0148
136. Ma X, Qu X, Zhu W, Li YS, Yuan S, Zhang H, et al. Deterministically patterned biomimetic human iPSC-derived hepatic model via rapid 3D bioprinting. Proc Natl Acad Sci U S A. (2016) 113(8):2206–11. doi: 10.1073/pnas.1524510113
137. Hossain Rakin R, Kumar H, Rajeev A, Natale G, Menard F, Li ITS, et al. Tunable metacrylated hyaluronic acid-based hybrid bioinks for stereolithography 3D bioprinting. Biofabrication. (2021) 13(4). doi: 10.1088/1758-5090/ac25cb
138. Ngo TD, Kashani A, Imbalzano G, Nguyen KTQ, Hui D. Additive manufacturing (3D printing): a review of materials, methods, applications and challenges. Composites Part B. (2018) 143:172–96. doi: 10.1016/j.compositesb.2018.02.012
139. Wang X, Dai X, Zhang X, Li X, Xu T, Lan Q. Enrichment of glioma stem cell-like cells on 3D porous scaffolds composed of different extracellular matrix. Biochem Biophys Res Commun. (2018) 498(4):1052–7. doi: 10.1016/j.bbrc.2018.03.114
140. Wang X, Li X, Dai X, Zhang X, Zhang J, Xu T, et al. Coaxial extrusion bioprinted shell-core hydrogel microfibers mimic glioma microenvironment and enhance the drug resistance of cancer cells. Colloids Surf B Biointerfaces. (2018) 171:291–9. doi: 10.1016/j.colsurfb.2018.07.042
141. Li X, Wang X, Chen H, Jin Z, Dai X, Zhang X, et al. A comparative study of the behavior of neural progenitor cells in extrusion-based in vitro hydrogel models. Biomed Mater. (2019) 14(6):065001. doi: 10.1088/1748-605X/ab3b4b
142. Matai I, Kaur G, Seyedsalehi A, McClinton A, Laurencin CT. Progress in 3D bioprinting technology for tissue/organ regenerative engineering. Biomaterials. (2020) 226:119536. doi: 10.1016/j.biomaterials.2019.119536
143. Derr K, Zou J, Luo K, Song MJ, Sittampalam GS, Zhou C, et al. Fully three-dimensional bioprinted skin equivalent constructs with validated morphology and barrier function. Tissue Eng Part C Methods. (2019) 25(6):334–43. doi: 10.1089/ten.tec.2018.0318
144. Cubo N, Garcia M, Del Cañizo JF, Velasco D, Jorcano JL. 3D bioprinting of functional human skin: production and in vivo analysis. Biofabrication. (2016) 9(1):015006. doi: 10.1088/1758-5090/9/1/015006
145. Do AV, Khorsand B, Geary SM, Salem AK. 3D printing of scaffolds for tissue regeneration applications. Adv Healthcare Mater. (2015) 4(12):1742–62. doi: 10.1002/adhm.201500168
146. Guvendiren M, Molde J, Soares RM, Kohn J. Designing biomaterials for 3D printing. ACS Biomater Sci Eng. (2016) 2(10):1679–93. doi: 10.1021/acsbiomaterials.6b00121
147. Albanna M, Binder KW, Murphy SV, Kim J, Qasem SA, Zhao W, et al. In situ bioprinting of autologous skin cells accelerates wound healing of extensive excisional full-thickness wounds. Sci Rep. (2019) 9(1):1856. doi: 10.1038/s41598-018-38366-w
148. Jin R, Cui Y, Chen H, Zhang Z, Weng T, Xia S, et al. Three-dimensional bioprinting of a full-thickness functional skin model using acellular dermal matrix and gelatin methacrylamide bioink. Acta Biomater. (2021) 131:248–61. doi: 10.1016/j.actbio.2021.07.012
149. Ong CS, Yesantharao P, Huang CY, Mattson G, Boktor J, Fukunishi T, et al. 3D Bioprinting using stem cells. Pediatr Res. (2018) 83(1-2):223–31. doi: 10.1038/pr.2017.252
150. Chen J, Zhou D, Nie Z, Lu L, Lin Z, Zhou D, et al. A scalable coaxial bioprinting technology for mesenchymal stem cell microfiber fabrication and high extracellular vesicle yield. Biofabrication. (2021) 14(1):1–17. doi: 10.1088/1758-5090/ac3b90
151. Roshangar L, Rad JS, Kheirjou R, Khosroshahi AF. Using 3D-bioprinting scaffold loaded with adipose-derived stem cells to burns wound healing. J Tissue Eng Regen Med. (2021) 15(6):546–55. doi: 10.1002/term.3194
152. Wu Y, Liang T, Hu Y, Jiang S, Luo Y, Liu C, et al. 3D Bioprinting of integral ADSCs-NO hydrogel scaffolds to promote severe burn wound healing. Regen Biomater. (2021) 8(3):rbab014.
153. Turner PR, Murray E, McAdam CJ, McConnell MA, Cabral JD. Peptide chitosan/dextran core/shell vascularized 3D constructs for wound healing. ACS Appl Mater Interfaces. (2020) 12(29):32328–39. doi: 10.1021/acsami.0c07212
154. Turner PR, McConnell M, Young SL, Cabral JD. 3D living dressing improves healing and modulates immune response in a thermal injury model. Tissue Eng Part C Methods. (2022) 28(8):431–9. doi: 10.1093/rb/rbab014
155. Shapira A, Dvir T. 3D tissue and organ printing-hope and reality. Adv Sci. (2021) 8(10):2003751. doi: 10.1002/advs.202003751
156. Gruene M, Pflaum M, Hess C, Diamantouros S, Schlie S, Deiwick A, et al. Laser printing of three-dimensional multicellular arrays for studies of cell-cell and cell-environment interactions. Tissue Eng Part C Methods. (2011) 17(10):973–82. doi: 10.1089/ten.tec.2011.0185
157. Kim BS, Kwon YW, Kong JS, Park GT, Gao G, Han W, et al. 3D Cell printing of in vitro stabilized skin model and in vivo pre-vascularized skin patch using tissue-specific extracellular matrix bioink: a step towards advanced skin tissue engineering. Biomaterials. (2018) 168:38–53. doi: 10.1016/j.biomaterials.2018.03.040
158. Li J, Zhang Y, Enhe J, Yao B, Wang Y, Zhu D, et al. Bioactive nanoparticle reinforced alginate/gelatin bioink for the maintenance of stem cell stemness. Mater Sci Eng C Mater Biol Appl. (2021) 126:112193. doi: 10.1016/j.msec.2021.112193
159. Cheng L, Yao B, Hu T, Cui X, Shu X, Tang S, et al. Properties of an alginate-gelatin-based bioink and its potential impact on cell migration, proliferation, and differentiation. Int J Biol Macromol. (2019) 135:1107–13. doi: 10.1016/j.ijbiomac.2019.06.017
160. Yao B, Wang R, Wang Y, Zhang Y, Hu T, Song W, et al. Biochemical and structural cues of 3D-printed matrix synergistically direct MSC differentiation for functional sweat gland regeneration. Sci Adv. (2020) 6(10):eaaz1094. doi: 10.1126/sciadv.aaz1094
161. Nourian Dehkordi A, Mirahmadi Babaheydari F, Chehelgerdi M, Raeisi Dehkordi S. Skin tissue engineering: wound healing based on stem-cell-based therapeutic strategies. Stem Cell Res Ther. (2019) 10(1):111. doi: 10.1186/s13287-019-1212-2
162. Bojanic C, To K, Hatoum A, Shea J, Seah KTM, Khan W, et al. Mesenchymal stem cell therapy in hypertrophic and keloid scars. Cell Tissue Res. (2021) 383(3):915–30. doi: 10.1007/s00441-020-03361-z
163. Giebel B, Kordelas L, Börger V. Clinical potential of mesenchymal stem/stromal cell-derived extracellular vesicles. Stem Cell Invest. (2017) 4:84. doi: 10.21037/sci.2017.09.06
164. Kusuma GD, Barabadi M, Tan JL, Morton DAV, Frith JE, Lim R. To protect and to preserve: novel preservation strategies for extracellular vesicles. Front Pharmacol. (2018) 9:1199. doi: 10.3389/fphar.2018.01199
165. Singh S, Choudhury D, Yu F, Mironov V, Naing MW. In situ bioprinting—bioprinting from benchside to bedside? Acta Biomater. (2020) 101:14–25. doi: 10.1016/j.actbio.2019.08.045
166. Rothbauer M, Eilenberger C, Spitz S, Bachmann BEM, Kratz SRA, Reihs EI, et al. Recent advances in additive manufacturing and 3D bioprinting for organs-on-A-chip and microphysiological systems. Front Bioeng Biotechnol. (2022) 10:837087. doi: 10.3389/fbioe.2022.837087
167. Tsiapalis D, O'Driscoll L. Mesenchymal stem cell derived extracellular vesicles for tissue engineering and regenerative medicine applications. Cells. (2020) 9(4):1–27. doi: 10.3390/cells9040991
168. Maiullari F, Chirivì M, Costantini M, Ferretti AM, Recchia S, Maiullari S, et al. In vivo organized neovascularization induced by 3D bioprinted endothelial-derived extracellular vesicles. Biofabrication. (2021) 13(3). doi: 10.1088/1758-5090/abdacf
169. Bari E, Scocozza F, Perteghella S, Sorlini M, Auricchio F, Torre ML, et al. 3D bioprinted scaffolds containing mesenchymal stem/stromal lyosecretome: next generation controlled release device for bone regenerative medicine. Pharmaceutics. (2021) 13(4):1–23. doi: 10.3390/pharmaceutics13040515
170. Kanitakis J. Anatomy, histology and immunohistochemistry of normal human skin. Eur J Dermatol. (2002) 12(4):390–9, quiz 400–1. PMID: 12095893
Keywords: skin tissue regeneration, biological engineering, 3D bioprinting, mesenchymal stromal cells, paracrine
Citation: Luo Y, Xu X, Ye Z, Xu Q, Li J, Liu N and Du Y (2022) 3D bioprinted mesenchymal stromal cells in skin wound repair. Front. Surg. 9:988843. doi: 10.3389/fsurg.2022.988843
Received: 7 July 2022; Accepted: 20 September 2022;
Published: 14 October 2022.
Edited by:
Kun Xiong, Independent researcher, ChinaReviewed by:
Khondoker Mehedi Akram, The University of Sheffield, United KingdomBob Liu, Tsinghua University, China
© 2022 Luo, Xu, Ye, Xu, Li, Liu and Du. This is an open-access article distributed under the terms of the Creative Commons Attribution License (CC BY). The use, distribution or reproduction in other forums is permitted, provided the original author(s) and the copyright owner(s) are credited and that the original publication in this journal is cited, in accordance with accepted academic practice. No use, distribution or reproduction is permitted which does not comply with these terms.
*Correspondence: Yuansen Luo ZHJqYXNvbmxhd0BvdXRsb29rLmNvbQ== Yongjun Du ZnNkeWp1bkAxNjMuY29t
†These authors have contributed equally to this work
Specialty Section: This article was submitted to Reconstructive and Plastic Surgery, a section of the journal Frontiers in Surgery