- 1Department of Stomatology, Sichuan Provincial People’s Hospital, University of Electronic Science and Technology of China, Chengdu, China
- 2Department of Stomatology, Hospital of Chengdu Office of People's Government of Tibetan Autonomous Region (West China Hospital Sichuan University Tibet Chengdu Branch Hospital), Chengdu, China
- 3School of Materials Science and Engineering, Southwest Jiaotong University, Chengdu, China
Copper has been used as an antimicrobial agent long time ago. Nowadays, copper-containing nanoparticles (NPs) with antimicrobial properties have been widely used in all aspects of our daily life. Copper-containing NPs may also be incorporated or coated on the surface of dental materials to inhibit oral pathogenic microorganisms. This review aims to detail copper-containing NPs’ antimicrobial mechanism, cytotoxic effect and their application in dentistry.
Introduction
Copper is a common metal with unique physical and chemical properties. Copper is the 26th most abundant element in the Earth's crust (1). Copper has been used in coins, jewelry, and utensils since ancient times, and the potential of copper to promote health effects was recognized as early as 3,000 BC (2, 3). A large variety of dental restorative materials contain copper, such as dental amalgam, porcelain-fused-to-metal crowns, implants, and partial denture attachments and frameworks (4–7). Copper is an essential trace element for humans and can promote angiogenesis, bone formation, wound healing, and the activities of various enzymes (8–10). Additionally, it also catalyzes the formation of crosslinks in collagen and elastin precursors (11–13). Moreover, copper is essential for maintaining the normal physiological functions of microorganisms. But high concentrations of copper can be used as microbicides (14–16). Prior to the development of antibiotics, inorganic antibacterial agents, such as silver and copper, were used to treat microbial infections (17). The paper reported copper as an antimicrobial coating as early as 1962 (18). Also, current research has shown that copper has a much less toxic effect on mammalian cells than silver (19).
With the progress of nanotechnology, copper has been increasingly used in the medical field, such as the latest copper-containing Nanoparticles (NPs), which have been proven to inhibit a variety of oral microorganisms, such as Streptococcus mutans (S. mutans) (20–22), Porphyromonas gingivalis (P. gingivalis) (23), and Candida albicans (C. albicans) (20, 24–26). In 2008, the International Organization for Standardization (ISO) defined NPs as discrete nano-objects with all three external dimensions less than 100 nm. In 2011, the European Commission set a more technical but wider ranging definition: a natural, incidental, or manufactured material containing particles in an unbound state, as an aggregate, or as an agglomerate and where, for 50% or more of the particles in the number size distribution, one or more external dimensions is within the size range 1–100 nm. Under this definition, nanomaterials can be classified as NPs only if one of their characteristic dimensions is within the range of 1–100 nm. NPs have many unique physical and chemical properties, such as tunable size, biocompatibility, and singlet oxygen generation, which allows them to be widely used (27, 28).
In recent years, the application of nanomaterials in dentistry has gradually increased, and copper NPs can be used as a new type of antimicrobial material (28). This paper reviews the antimicrobial mechanisms of copper-containing NPs and their application in dentistry.
Different types of copper-containing NPs
Various types of copper-containing NPs are successfully synthesized, such as copper NPs (Cu NPs), copper oxide NPs (CuxO NPs), and copper-containing bimetallic NPs. CuxO NPs are widely used in the fields of biomedicine, environmental restoration, and industry (29–31). In biomedicine, cuprous oxide (Cu2O) and cupric oxide (CuO) are often used as antimicrobial agents (32–34). Compared with organic antimicrobial agents, copper oxide has the advantages of stable physical and chemical properties, solidity, and a relatively long shelf life. Moreover, copper oxide has physical properties that allow it to be easily mixed with polymers, which enables CuxO NPs to be prepared into a variety of composite materials. Compared with CuxO NPs, Cu NPs are relatively unstable and easily oxidized. Copper (Cu) is easily oxidized to form Cu2O and CuO when exposed to the air, making it difficult to synthesize Cu NPs in an ambient environment. Therefore, it is usually necessary to synthesize Cu NPs in the presence of polymers and surfactants and form coatings on the surface of Cu NPs (25). Copper-containing bimetal NPs are NPs containing copper and another metal element. The combination of two metal elements will have a synergistic effect and may have better antimicrobial performance than single metal. For example, Perdikaki et al. (35) have shown that synthesized Ag/Cu bimetallic NPs have stronger antimicrobial properties than Ag and Cu monometallic NPs.
These different types of copper-containing NPs can be incorporated into supporting materials (chitosan, cellulose polymers, hydrogels, etc.), which are biocompatible and retain antimicrobial activity (36–40). Tran CD et al. (41) synthesized composites containing cellulose, chitosan, and CuO NPs. This composite can prevent the aggregation, coagulation, and changes in size and morphology of CuO NPs without changing the unique properties of the NPs. Moreover, they can exert superior antibacterial activity against a variety of bacteria and fungi, and the antibacterial activity is related to the content of CuO NPs. As chitosan is a biocompatible, biodegradable, and non-toxic polymer, copper-containing NPs can be incorporated into chitosan and used in dental materials. Chitosan can interact with hydroxyapatite and the bacterial cell walls of teeth to improve the adhesion of copper on the tooth surface and the anti-biofilm action of copper (42). Chitosan not only has a good inhibitory effect on Gram-negative bacteria, Gram-positive bacteria, and fungi (43), but also interferes with oral microbial adhesion, inhibit biofilm formation and maturation, and promote wound and oral ulcer healing (43–46). Mishra et al. (47) synthesized biocompatible thiol-functionalized cellulose-grafted copper oxide nanoparticles, which alleviated colitis conditions and recovered damaged colon structure. Cellulose enhances the biocompatibility of copper oxide NPs and avoids the adverse effects of CuO NPs on the biological systems. The Cu-NP-embedded hydrogels also possessed remarkable antibacterial ability, and reduced the inflammatory response and promoted angiogenesis in vivo to accelerate the wound healing process (48). By preparing copper-containing NPs and other materials into composites, the original physical and chemical properties of copper-containing NPs can be retained while giving composites new characteristics, making them more suitable for clinical application.
Antimicrobial mechanism of copper-containing NPs
Copper can cause damage to various cell functions and exert cytotoxicity, making it an effective microbial inhibitor. In general, copper damages microbial cells by generating reactive oxygen species (ROS) and replacing or binding the native cofactors in metalloproteins (49). Besides, copper is also involved in innate immunity and can catalyze the formation of ROS in the blasting reaction taking place within phagocytes, enhancing the bactericidal activity during bacterial phagocytosis (14, 50).
Copper-containing NPs can inhibiting microorganisms through the same mechanism as other types of copper materials mentioned above (51–53). Many studies have shown that NPs can exert stronger antimicrobial properties than ordinary size materials, but the reason for this is not completely clear at present. Compared with other copper molecular materials, Copper-containing NPs has higher surface area and different crystal structure, and can affect different cellular components of microbial cells through some unique mechanisms to exert better antibacterial activity (54–59). Copper-containing NPs can dissolve faster in solutions, release more metal ions, and exert a stronger antimicrobial effect (60). In addition, Copper-containing NPs can bring multiple antibacterial mechanisms simultaneously, but it is difficult for the same microorganism to have multiple gene mutations to cope with various antimicrobial mechanisms of NPs, so the probability of antimicrobial resistance is low.
In general, Copper-containing NPs added to many dental materials inhibits microorganisms mainly through the release of the NPs and copper ions. The antimicrobial process of copper-containing NPs is to produce ROS, destroy cell walls and cell membranes, and react with proteins and DNA (61). In this process, copper-containing NPs can damage different microbial cell components through a variety of mechanisms (Figure 1).
Generation of ROS
Oxidative stress caused by ROS is crucial in the antibacterial effect of copper. ROS are oxygen-containing derivatives composed of highly unstable oxygen radicals, such as superoxide (O2•−), hydroxyl (OH•), hydrogen peroxide (H2O2), and singlet oxygen (O2) (62). The atomic or molecular orbitals of ROS contain one or more unpaired electrons, which makes them highly reactive (63). Transition metals, such as copper, iron, and silicon, can generate ROS through Fenton type and Haber-Weiss reactions:
During these reactions, copper accepts and donates an electron during cycling between the Cu+ and Cu2+ oxidation states, producing O2•− and hydroxyl OH•, which are highly reactive and have strong damaging potential, leading to lipid peroxidation, protein oxidation, and DNA damage (64–66).
In the presence of water and oxygen molecules, copper can only dissolve a small amount of copper ions (67). In fact, the metal ions released by dissolution outside the cell are not the main antibacterial mechanism of copper-containing NPs. A recent study showed that dissolved copper ions contributed less than half of the total cytotoxicity induced by CuO NPs (68). A possible reason for this is that metal-based NPs enter the acidic lysosomal environment (pH 5.5) in cells, which promotes the formation of free radicals and the degradation/corrosion of NPs, thereby converting the core metal into an ionic form and resulting in the intracellular release of metal ions. This process of the internalization of NPs is often referred to as “the Trojan horse mechanism”, which promotes the formation of intracellular ROS (69, 70). The formation of ROS is an important part of the antimicrobial mechanism of copper-containing NPs. Copper-containing NPs can not only generate ROS by directly leaching ions but may also generate ROS through multiple mechanisms, such as the disruption of mitochondrial membrane potential, and produce singlet oxygen to mediate the degradation of DNA (71, 72). However, these mechanisms have not been fully clarified, and relevant microbial cytotoxicity studies are lacking.
Disruption of microbial cell walls and cell membranes
The early stage of copper-containing NPs damage to microbials is their direct contact with the microbial surface, which leads to the alteration of the microbial cell wall and cell membrane (73). Metal-based NPs and their leached metal ions are positively charged, and the surface of both Gram-positive and Gram-negative bacteria are negatively charged. Therefore, through electrostatic interaction, metal-based NPs will be adsorbed onto the surface of bacteria and build strong bonding leads for the destruction of the cell wall. This process increases cell permeability and allows metal-based NPs to enter the cell easily (74). Besides, copper ions can be combined with negatively charged areas on the cell membrane to reduce the potential difference and cause depolarization. When the potential difference drops to zero, it will cause membrane leakage or even rupture, exposure of the cellular components, and, eventually, bacterial death (75). Many studies have shown that the cell membrane is a direct target of copper exposure (76, 77). Hong et al. (78) found that E. coli died after 45 min of copper alloy contact, but no degradation of genomic DNA was observed. Copper ions can cause oxidative damage to the unsaturated fatty acids of bacterial cell membrane phospholipids through the production of extracellular ROS, while OH· can drive the non-enzymatic peroxidation of the unsaturated double bonds of fatty acids, thereby triggering a series of reactions and leading to extensive changes in the structure of the phospholipid bilayer and destroying the biophysical properties of the membrane, which ultimately leads to a loss of membrane integrity, exposure of the cell components, and cell death.
However, the bactericidal effect of copper-containing NPs on Gram-positive bacteria is stronger than that of Gram-negative bacteria, which may be due to the difference in the cell wall structure of these two classes. Compared with lipids, copper has a higher affinity for proteins, so Gram-positive bacteria with higher levels of peptidoglycan and protein content in the cell wall is more easily destroyed by copper-containing NPs (44).
Replace or bind the native cofactors in metalloproteins
Previous studies believed that copper toxicity was mainly related to the production of ROS, but later studies found that, under anaerobic conditions, copper accumulation can also increase cytotoxicity to bacteria (79, 80). Moreover, recent studies have shown that copper's cytotoxicity to microorganisms is also closely related to its ability to replace or bind to the native cofactors in metalloproteins. Intracellular copper accumulation promotes mismetallation, which is mainly related to the iron-sulfur cluster protein and its assembly process (81). Specifically, the copper accumulated in bacterial cells mainly exists in the form of highly toxic Cu+, which coordinates with the thiolate or inorganic sulfur ligands of the solvent-exposed dehydratase and replaces the iron atom, rapidly inactivating Fe/S cluster dehydratases to cause cell dysfunction (82, 83). In addition, copper and iron in Escherichia coli (E. coli) cells seem to share the same binding site in the Iron-sulfur cluster assembly protein (IscA), and excessive copper can also compete with iron for metal binding sites in IscAs and effectively inhibit the IscA-mediated assembly of [4Fe-4S] clusters (81, 84).
Damage of intracellular components
As described above, the uniqueness of the toxicity of copper-containing NPs to microorganisms is due to that they can directly enter the cells, and are internalized into complete intracellular particles in microbial cells through the Trojan horse mechanism. Studies by Kaweeteerawat et al. (85) showed that, at low concentrations, copper ions mainly inhibit microorganisms by damaging cell membranes rather than by causing oxidative stress in cells. However, they found that, even at lower concentrations, copper-containing NPs are also sufficient to promote the production of large amounts of intracellular ROS. In general, copper-containing NPs entering cells can directly damage oxidative organelles, such as mitochondria, and lead to increased intracellular ROS, protein oxidation, and DNA degradation (86, 87). Studies by Chatterjee et al. (87) showed that the oxidation of proteins in cells is mediated by ROS, but the degradation of DNA is a ROS-independent phenomenon caused by the intracellular release of copper ions. Studies by Giannousi et al. (33) have also found that copper-containing NPs induce DNA degradation in a dose-dependent manner and extensively degrade double-stranded calf thymus DNA (dsCT-DNA) at low concentrations. In general, the exact mechanism of the antimicrobial effect of copper-containing NPs is unclear and needs to be elucidated.
Factors affecting antimicrobial effect
The main physical factors affecting the antimicrobial activity of copper-containing NPs are include the size, morphology and environmental conditions (temperature) of the NPs., Chemical factors include environmental conditions (PH value, dry or wet, and composition of the surrounding medium), the doping modification of other elements, and the oxidation state of copper.
Size and morphology
It has been suggested that due to the small size and high surface-to-volume ratio, metallic NPs can exert better antimicrobial activity than ordinary metals (88–91). At similar surface area doses, copper NPs and copper microparticles have similar effects on cell membrane damage, reflecting the fact that the damage of the cell membrane is related to the surface area of NPs (60, 89). Different sizes of copper-containing NPs have different inhibitory effects on Gram-positive and Gram-negative bacteria (92). Azam et al. (93) found that the small size CuO NPs is more stable than the large size CuO NPs and has significantly stronger antibacterial properties. Some studies have also proved that the antibacterial activity of CuO NPs and Cu2O NPs are size-dependent: the reduction in the size of the NPs leads to an increase in antibacterial properties (93–95). Applerot et al. (94) believed that the reason for the stronger antibacterial effect of small-size CuO NPs is due to their stronger ability to penetrate cells. The high surface-to-volume ratio and small size of copper-containing NPs enhance their interaction with microbial membranes, enabling them to exert stronger antimicrobial activity than copper ions.
The antimicrobial activity of NPs is also related to morphology, and different morphologies of NPs can cause different degrees of bacterial cell damage through interactions with periplasmic enzymes (96). Copper-containing NPs with different crystal planes have different surface energies, and this difference may also be responsible for the morphologically dependent antimicrobial activity of copper-containing NPs. The higher surface energy of the exposed facets of copper-containing NPs may generate copper ions more effectively and lead to stronger antimicrobial activity (97, 98). Xiong et al. (99) synthesized polyhedral, flower-like, and thumbtack-like Cu/CuxO NPs. And they proved that, among the three kinds of Cu / CuxO NPs, the main exposed facets {111} of the flower-like Cu / CuxO NPs had the highest surface energy, dissolved the most copper ions in the culture medium, and had the best antibacterial ability. Studies by Feng et al. (100) have shown that {100} facets of the Cu2O nanocrystals can release more copper ions and produce more ROS in a shorter amount of time than {111} facets of the Cu2O nanocrystals, resulting in stronger toxicity in the short term. Besides, some studies believe that {110} facets of the Cu2O microcrystals have better antibacterial activity against E. coli than that of {111} facets (101, 102). However, on the contrary, some studies also believe that {111} facets of the Cu2O microcrystals have stronger antibacterial properties (103). In addition, studies by Pang et al. (97) have shown that the antibacterial activity of cubic Cu2O has a broad spectrum, while the antibacterial activity of octahedral Cu2O has high selectivity (Figure 2).
Ambient conditions
The ambient conditions of copper-containing nanoparticles are one of the factors that affect the antibacterial effect (Figure 3). The temperature and pH of the solvent affect the rate at which copper inhibits microorganisms. Studies by Sharan R et al. (17) showed that copper can cause the rapid inactivation of E. coli at higher temperatures and exhibit a faster inactivation at pH 6.0 and 9.0 than at pH 7.0 and 8.0. However, the effect of pH on bacterial inactivation is not as significant as that of temperature. The dissolution of copper ions is also an important part of the antimicrobial activity of copper-containing NPs. Usually, copper-containing NPs release more copper ions in an acid lysosomal environment than in a neutral environment (104, 105). Dry conditions bring about faster microbicidal effects to copper, as the contact killing caused by dry copper surfaces can kill microorganisms in a short amount of time. Tian et al. (106) demonstrated that the Enterobacter cell structure was severely degraded after exposure to the dry copper surface for 30 s. Moreover, compared to wet conditions, copper kills Enterococcus 80% to 90% faster under dry conditions (77). In the case of contact killing, the antimicrobial effect of copper is not related to the dissolution of copper but the copper content on the contact surface (107).
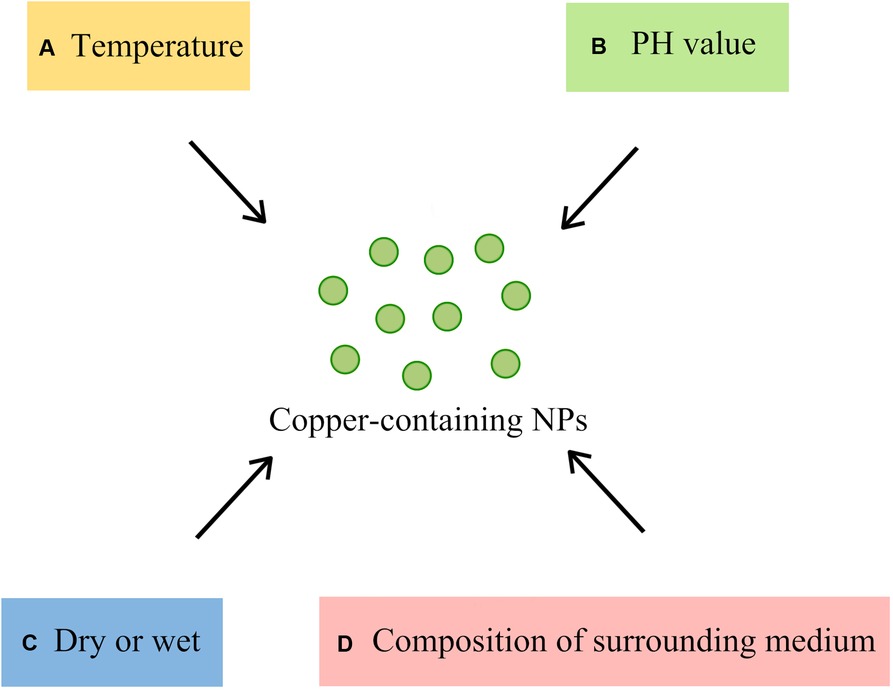
Figure 3. Ambient conditions affecting the antibacterial effect of Copper-containing NPs (A) temperature: the higher the temperature, the faster the sterilization; (B) PH value: the sterilization of acidic and alkaline conditions is faster than that of neutral conditions; (C) Dry or Wet: dry conditions kill bacteria faster than wet conditions; (D) composition of the surrounding medium: interaction of NPs with other molecules in the medium).
Besides, the interaction of copper-containing NPs with other molecules present in biological and environmental media may greatly affect the solubility, aggregation state, and surface properties of the NPs, resulting in changes of the toxicity (108). Studies by Badetti et al. (109) found that CuO NPs can react with some natural amino acids to affect their antimicrobial properties. L-Glutamic can be bonded to the surface of CuO NPs to enhance their antibacterial activity, while L-Asparaginase, L-Leucine, l-Phenylalanine, and L-Tyrosine can weaken the antibacterial activity of CuO NPs by forming complexes with copper ions. Ruparelia et al. (110) found that the chloride-containing nutrient media can promote the dissolution of Cu NPs, so as to release copper ions, which may be due to the interaction between the chloride ions and the oxide layer of the NPs.
Doping modification
Doping modifications can modulate the interaction between NPs and microorganisms. Some studies have shown that doping other materials into Cu NPs and CuxO NPs can improve their antimicrobial ability. For example, studies by Lv et al. (111) have shown that the doping of Mg, Zn, and Ce ions can promote the release of Cu2+ in the doped CuO NPs and promote their antibacterial activity. Studies by Malka et al. (112) have shown that Zn-doped CuO NPs can generate more ROS than pure CuO NPs or ZnO NPs and thus exert stronger antibacterial activity. After being exposed to E. coli and Staphylococcus aureus (S. aureus) for 10 min, the antibacterial activity of Zn-doped CuO NPs was 10,000 times greater than that of pure CuO NPs or ZnO NPs. Besides, other metal component of copper-containing bimetal NPs, such as iron, can promote the conversion of Cu2+ to more toxic Cu+ and Cu3+, which makes bimetallic iron-copper NPs exhibit a stronger antimicrobial activity than Cu NPs and iron NPs (Fe NPs) (113).
Oxidation state
Copper has different antimicrobial properties under different oxidation states. The surface of pure copper is susceptible to oxidation and forms both CuO and Cu2O. Oxidative conditions (e.g., clean water in the air, upper hatched areas) contribute to the formation of CuO, while reducing conditions (e.g., the presence of organic matter and bacteria) contribute to the formation of Cu2O. These changes in oxidation state may affect the antibacterial properties of copper-containing NPs (105). Studies by O Akhavan et al. (114) have shown that Cu NPs exhibit greater antibacterial activity than CuO NPs, which may be attributed to the stronger electron-accepting ability and better electron transfer with bacteria of Cu NPs. The electron transfer between the negatively charged bacteria and the metal NPs is one of the effective mechanisms that cause the bacterial membrane rupturing and exerting antibacterial activity. Studies by Hans M et al. (115) have shown that Cu NPs and cuprous oxide NPs (Cu2O NPs) have strong contact killing activity against bacteria, while CuO NPs significantly inhibit contact killing. This difference is roughly related to the release of copper ions: pure copper releases the most copper ions, followed by Cu2O and CuO. Studies by Giannousi et al. (33) have also shown that the antibacterial activity of Cu2O NPs against a variety of Gram-negative and Gram-positive bacteria strains is stronger than CuO NPs. However, CuO NPs can induce higher ROS than Cu2O NPs, which is probably because CuO NPs can generate ROS through Haber-Weiss and Fenton type reactions, while Cu2O NPs can only generate ROS through Fenton type reactions. Moreover, CuO NPs have a higher degree of internalization and better antifungal activity at lower concentrations (24).
Host tissue interaction of copper-containing NPs
Although copper-containing NPs are highly anticipated new materials, it is necessary to ensure their biosafety to human bodies (116–120). Copper is an essential trace element for the human body, participating in various kinds of physiological activities. Copper containing enzymes and transcription factors are essential for cellular integrity, energy production, signalling, proliferation, oxidation and radiation defence (121). The liver, brain, heart and kidneys have the highest copper concentration in the body, followed by the lungs, intestines and spleen.
Research concerning acute or chronic toxicity of copper due to its deficiency or excess is growing rapidly and interest in the subject is pervasive (122–129). The four major routes of human exposure to engineered NPs include inhalation, dermal penetration, ocular exposure, and ingestion. Studies have shown that oral exposure of copper containing NPs in rats mainly accumulates in liver, kidney, stomach, intestine, lung, brain and blood, among which liver and kidney are the main organs most affected by Cu NPs (130, 131) (Figure 4). Exposure to NPs induces an inflammatory response and activates the immune system (132). The toxicity mechanism of copper-containing NPs to human cells is similar to that of microbial cells. Copper-containing NPs will dissolve and release copper ions, generate ROS, disrupt normal cellular functions and cause DNA damage. Changing the physicochemical properties of copper-containing NPs can change the induced toxic response/mechanism of action, such as size (aerodynamic, hydrodynamic), surface (surface area:mass ratio), chemical composition (core structure, surface functionalization, coatings), solubility (hydrophobic, hydrophilic) (133).
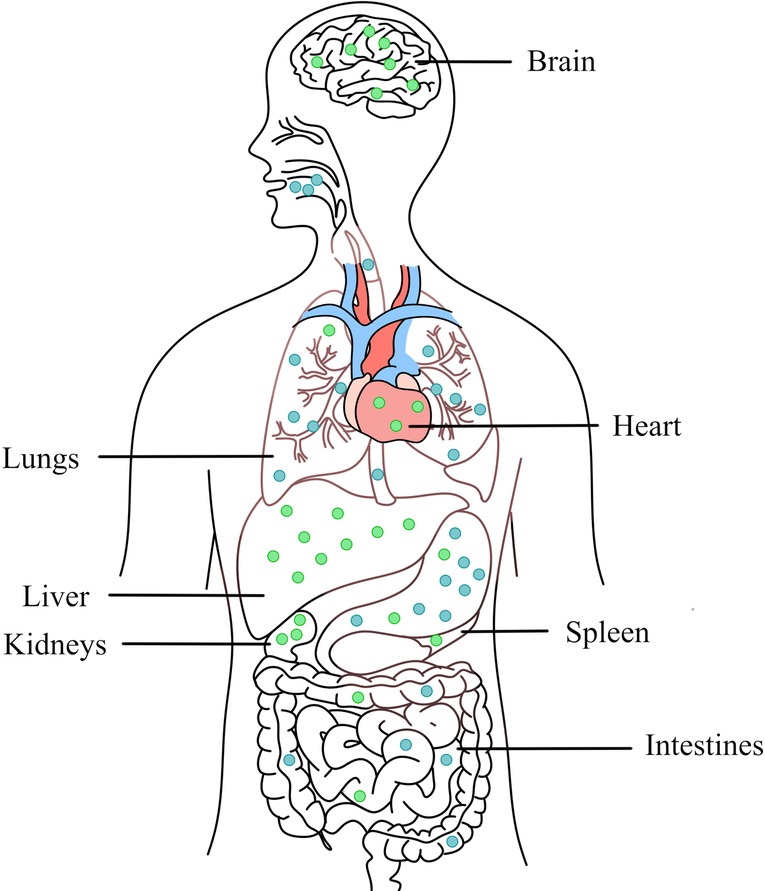
Figure 4. Main routes of transfer (blue) and accumulation (green) in organs of copper-containing NPs in the body through oral intake.
In dentistry, copper-containing NPs are expected to be used in restorative materials, prosthodontic materials, dental implants and orthodontic appliances. Researchers tried to explore safe forms to reduce toxicity of copper-containing NPs (116, 118). In fact, copper-containing NPs applied to dental materials rarely enter the body,and the concentration and morphological characteristics of copper-containing NPs can be controlled so that they will not be cytotoxic to normal cells (134). In addition, since copper-containing NPs can exert better microbial inhibition effect than copper native, addition amount of copper-containing NPs can be lower (44).
Inhibitory effect of copper-containing nps on oral pathogenic microorganisms
Since microorganisms are less susceptible to resistance against metal antibacterial substances, the application of metals as antibacterial substances to control oral plaque has become a research hotspot. Studies have shown that copper-containing NPs can inhibit various oral pathogens, such as S. mutans (20, 21), P. gingivalis (23), and C. albicans (20, 24–26) (Table 1).
S. mutans
It is well-known that S. mutans is the main pathogen of dental caries, which can adhere to the surface of tooth or dental prosthesis to form plaque biofilm, produces acid, and causes dental caries (135–139). Numerous studies have shown that copper can inhibit the growth of S. mutans and caries formation (140–143). In the case of extracellular high concentrations of copper, copper ions enter S. mutans cells and inhibit the transcription of glucosyltransferase (gtf) genes and glucan binding protein (gbp) genes to reduce cell adherence and biofilm biomass (144, 145).
Generally, bacteria have a “copper transport system” to cope with fluctuating copper ion concentrations in complex ecosystems and maintain copper homeostasis (Figure 5). S. mutans can tolerate extracellular high concentrations of copper through a conserved P-type ATPase, a copper-transport operon (146, 147). S. mutans can also oxidize intracellular Cu+ to less toxic Cu2+. Despite a certain degree of resistance to copper, copper-containing NPs can still effectively inhibit S. mutans through various mechanisms. Studies by Amiri et al. (20) have shown that the MIC50 value for CuO NPs with a size of 40 nm is 1–10 µg/ml for S. mutans, and higher concentrations of CuO NPs (100–1,000 µg/ml) can significantly inhibit bacterial growth. Similarly, Khan et al. (21) also demonstrated that CuO NPs at a size of 40 nm can significantly inhibit the growth of human oral pathogens (such as S. mutans), the extracellular polysaccharide production, and the multispecies biofilm formation at a concentration of 50 µg/ml. In another study, Eshed et al. (148) used the sonochemistry method to coat CuO NPs on the teeth surface, and the biofilm formation on the teeth coated with CuO NPs was significantly reduced by 70%. Similarly, Covarrubias et al. (42) synthesized hybrid NPs of chitosan-coated copper NPs (CuCh NPs), which can significantly inhibit the growth of S. mutans and significantly reduce biofilm formation.
P. gingivalis
P. gingivalis is the main periodontal pathogen and is closely related not only to the occurrence of periodontal disease but also to the occurrence of systemic diseases, such as atherosclerosis, diabetes, and rheumatoid arthritis (149–153). Studies have shown that copper and copper alloys can inhibit the growth of P. gingivalis by contact killing, such as the Ti-Cu alloy, which can exert good antibacterial activity by killing the bacteria as well as reducing the activity of any surviving bacteria (154, 155). In addition, copper ions can inhibit the coaggregation of P. gingivalis with other bacteria, thereby reducing the accumulation of P. gingivalis in plaque biofilm and also reducing the pathogenicity and occurrence of periodontal disease (156). P. gingivalis is a Gram-negative bacterium with a lipopolysaccharide on the cell membrane surface that can prevent copper-containing NPs entering the cell. However, copper-containing NPs still have a significant inhibitory effect on P. gingivalis. Vargas-Reus et al. (23) found that the MICs of CuO NPs, Cu2O NPs and Ag + CuO composite with a size ranging between 10 nm and 50 nm were 500 µg/ml, <100 µg/ml and <100 µg/ml for P. gingivalis, respectively, suggesting that they have good antibacterial activity. Additionally, CuO NPs, Cu2O NPs and Ag + CuO composite has better antibacterial ability to P. gingivalis than ZnO NPs, TiO2 NPs and Ag NPs.
C. albicans
C. albicans is the most common fungus in the oral cavity and is a conditional pathogen that often causes fungal infections in the elderly population or in denture patients. (157, 158) C. albicans can form a biofilm on the oral mucosa against external antifungal agents, which makes it pathogenic (159). Copper-containing NPs have also exhibited considerable antimicrobial activity against C. albicans. Padmavathi et al. (24) found that both CuO NPs and Cu2O NPs have fungal inhibitory activity. They can destroy the cell membrane of C. albicans by inhibiting the production of ergosterol, which lead to the loss of virulence. They can also alter the expression of the genes involved in the morphogenesis of C. albicans. CuO NPs can inhibit mycelial growth, while Cu2O NPs can distinctively inhibit morphological switching. Moreover, CuO NPs have a stronger inhibitory effect on C. albicans than Cu2O NPs and exhibit better antifungal activity at a low concentration. Amiri et al. (20) found that CuO NPs with a size of 40 nm couldreduce the growth of C. albicans, Candida krusei, and Candida glabrata, and the MIC50 value of CuO NPs was 1,000 µg/ml for these three species of oral Candida. Pugazhendhi et al. (26) synthesized Fe-doped CuO using a sol-gel method, which has a rectangular shape and agglomerates at an average size of 21 nm. The Fe-doped CuO has excellent antimicrobial and anti-biofilm properties to C. albicans, which can reduce the growth OD of C. albicans to 0.28 at 30°C for 24 h and reduce the biofilm by 76.4% at a concentration of 100 µg/ml. In another study, Lara et al. (159) synthesized chitosan-copper NPs with a size between 2 nm and 350 nm and proved that they had good antimicrobial activity against C. albicans.
Application of copper-containing NPs in dentistry
Copper-containing NPs can be applied to various aspects of dentistry. Applying NPs to the surface of dental materials or incorporating them in dental materials can not only impart different antibacterial activity to the material, but also improve or maintain the mechanical properties of the material (22, 160, 161). When applied to dental materials, they can also play a variety of beneficial roles by inhibiting metalloproteinases (MMPs) (146). Many current studies have synthesized different types of copper-containing NPs that can be used for dental materials, including dental adhesive es and filling materials, implant and bracket coatings, etc. (Figure 6) and (Table 2).
Dental adhesives
Many recent studies have shown that copper-containing NPs in dental adhesives can not only effectively inhibit bacteria but also improve the performance of the adhesive. Copper ions released by copper-containing NPs can be used as an effective dentin metalloproteinase (mainly on the Matrix metalloproteinase's subtypes −2 and −9) inhibitor, which can stimulate the secretion of the tissue inhibitors of MMPs (162). Matrix metalloproteinase 2 (MMP-2) is involved in the destruction of periodontal tissue and the development of oral squamous cell carcinoma, and it also plays an important role in the destruction of dentin during the progression of caries (162–165). MMPs can also mediate the degradation of adhesives, while inhibiting MMP can increase the longevity of the adhesive-hard tissue interface and improve the bonding effect of adhesives (166–169). Besides, MMPs inhibitors can prevent dental caries, reduce dentinal caries progression, and promote remineralization (170–172). Studies by Gutiérrez et al. (173) have shown that the addition of Cu NPs and ZnO NPs to the universal adhesive system can provide the adhesive with antibacterial activity against S. mutans and anti-MMPs properties without affecting its mechanical properties, thereby improving the integrity of the hybrid layer on caries-affected dentin. The addition of copper-containing NPs to the adhesive may also improve its mechanical properties. Vidal Oet al. (174) incorporated copper nanoparticles (CuNp) into a universal adhesive and applied it to dentin surfaces. The addition of copper nanoparticles can significantly enhance the antibacterial activity of the resin-dentin interface, showing higher bond strength and mechanical properties, even under cariogenic challenges. Javed et al. (175) incorporated CuO NPs and CuO-chitosan NPs into dentin adhesives, which can significantly inhibit Lactobacillus acidophilus (L. acidophilu) and S.mutans and effectively treat secondary caries. The addition of NPs also improved the mechanical properties, water absorption and solubility of the adhesive without affecting the shear bond strength.
Many studies have also shown that adhesives with copper-containing NPs can exhibit long-lasting and effective antimicrobial effects. For example, studies by Gutiérrez et al. (176) have shown that the addition of Cu NPs at a concentration of 0.1 wt% in the adhesive system can provide antibacterial properties without reducing the mechanical and optical properties of the adhesive formulations. Moreover, compared with copper-free adhesives, copper-containing adhesives can significantly reduce the dentin degradation of the resin-dentin bonded interfaces dentin after two years of water storage. In addition, a sufficient concentration of copper still exists in the adhesive interface, which can exert anti-MMPs effects. In another study, Jun et al. (177) synthesized novel copper-doped bioactive glass NPs (CuBGn NPs) and added them to the resin-dentin adhesive system. Although there are no antibacterial experiments to prove its antibacterial properties, the adhesive can release up to 0.5 ppm copper ions over a 28-day period, which is sufficient to deactivate MMPs, promote remineralization, and extend the longevity of resin-dentin interfaces dentin regeneration.
Other studies have shown that adding copper-containing NPs to adhesives will not cause additional cytotoxicity to the pulp or oral soft tissues. For example, Sabatini et al. (178) synthesized polyacrylic acid coated copper iodide NPs (PAA-CuI NPs) and incorporated them into adhesives. After ageing for 18 h or one year, the adhesive can exert effective antibacterial effects without affecting the bond strength and cytotoxicity. A study by Matos et al. (179) also confirmed that the addition of 0.1 wt% Cu NPs to the adhesive can improve the clinical performance of universal adhesive systems in non-carious cervical lesions without increasing cytotoxicity.
Moreover, Cu NPs and CuO NPs can also be added to orthodontic adhesives to exert a certain antibacterial effect. Studies have shown that the addition of Cu NPs can significantly improve the material shear bond strength, while the addition of CuO NPs will not adversely affect the shear bond strength (180).
Dental filling materials
Copper-containing NPs can also be used in dental filling materials. Studies by Renné et al. (181) have shown that the incorporation of polyacrylic acid coated copper NPs (PAA-CuI NPs) into glass ionomer-based materials can improve their antibacterial properties and reduce collagen degradation without affecting the mechanical properties, which can help increase the longevity of adhesive restorations. Aguilar-Perez et al. (182) synthesized copper-containing NPs composed of metallic copper and cuprous oxide and added them to commercial glass ionomer cement, confirming that they can inhibit oral anaerobic bacteria strains. In addition, the glass ionomer cement doped with copper-containing NPs has no cytotoxic effect and will not damage the dental pulp.
Antimicrobial coatings
NPs can be used to control the formation of microbial biofilms in the oral cavity, which allows them to be incorporated into coatings and applied to a variety of dental materials (183, 184). Although dental implants have a high success rate, there are still failures. Poor osseointegration and infection are important reasons for implant failure (185). Coatings containing copper-containing NPs are commonly used in dental and orthopedic implants to increase their success rates by improving bone binding capacity and reducing the incidence of post-surgery infections (186, 187). Copper-containing NPs reduce the formation of biofilms on the surface of titanium implants. Moreover copper is involved in enzyme-based processes for bone metabolism and stimulates the formation of new blood vessels, which, in turn, reduces implant-related infections (90). Therefore, coating the titanium surface of the implant with copper-containing NPs can reduce the use of prophylactic antibiotics, which may cause the development of antibiotic resistant strains (188–190). Besides, the incorporation of an appropriate amount of Cu NPs on the implant surface not only has no cytotoxicity to endothelial cells and osteoblasts but also promotes osteoblast proliferation and adhesion as well as extracellular matrix mineralization (191).
Many studies have confirmed that the coatings containing copper-containing NPs can exert antimicrobial activity and be used in dentistry (192–194). For example, Li et al. (195) prepared an antibacterial coating material based on mussel-inspired dendritic polyglycerol embedded with Cu NPs, which not only has a bacteriostatic rate of over 99.99% against S. aureus, E. coli, and kanamycin-resistant E. coli, but also can exert effective long-term and durable antibacterial properties against E. coli. Rosenbaum et al. (196) prepared copper nanocubes with an average size of 20 nm on the surface of TiO nanotubes. This copper derived TiO surfaces could cause the death of E. coli and S. aureus and exert a powerful bactericidal ability. Ghosh et al. (197) used a two-stage electrochemical method to synthesize copper-hydroxyapatite (Cu-HA) composite coatings on titanium surfaces, which can slowly release copper ions while enhancing implant osseointegration to provide a sustained bacteriostatic effect. In addition, CuO NPs can be coated on the surface of orthodontic brackets. Studies have also shown that the CuO NPs and ZnO-CuO NPs coatings on the surface of orthodontic brackets have stronger antibacterial effects on S. mutans than ZnO NPs coatings (198).
Mouthwashes
Copper-containing NPs also have the potential to be added to mouthwashes for antimicrobial action. In one study, CuO NPs were prepared in colloidal solutions as mouthwashes, and it was found that, although not as good as chlorhexidine mouthwash, CuO NPs can also have a certain antibacterial effect on S. mutans (199).
Soft denture liners
The intrinsic porosity of soft denture pads facilitates the adhesion and colonization of microorganisms and promotes the formation of biofilms. A study by et al. (200) showed that the incorporation of CuO NPs at a concentration of 500 µg/ml into soft denture liners exerted an effective prevention of oral microbial infection. The biofilm inhibition rates of soft denture liners containing CuO NPs against C.albicans, Streptococcus sobrinus (S. sobrinus), S. mutans, and Streptococcus salivarius (S. salivariu) were 75%, 66%, 30%, and 60%, respectively.
Conclusion
Many current studies indicate that copper-containing NPs can be used in dentistry due to their antimicrobial and anti-biofilm properties. Copper-containing NPs are a new type of ideal antimicrobial material, which can inhibit or kill a variety of oral pathogenic microorganisms without causing microbial resistance, and can also produce a certain degree of beneficial effects on oral tissues. Various forms of copper-containing NPs are still being explored for use in dental filling materials, prosthetic devices and implant coatings, and oral antimicrobial agents. However, many of these studies have been performed under in vitro conditions, and further in vivo studies are needed to assess their safety and clinical efficacy.
Author contributions
XM contributed to the motivation, the interpretation of the methods, the data analysis and results; SZ provided the draft versions and revised versions, references; XX provided the data and results, the revised versions and references; QD provided the related concepts and minor recommendations, extracted the conclusion and discussion. All authors contributed to the article and approved the submitted version.
Funding
This work was supported by The Applied Foundation in Science and Technology office of Sichuan Province (No. 2021YFG0230)
Publisher's note
All claims expressed in this article are solely those of the authors and do not necessarily represent those of their affiliated organizations, or those of the publisher, the editors and the reviewers. Any product that may be evaluated in this article, or claim that may be made by its manufacturer, is not guaranteed or endorsed by the publisher.
References
1. Mainz VV. The metals of antiquity and their alloys. Chem Technol Antiq. (2015) 1211:139–79. doi: 10.1021/bk-2015-1211.ch005
2. Sudha VP, Ganesan S, Pazhani G, Ramamurthy T, Nair G, Venkatasubramanian P. Storing drinking-water in copper pots kills contaminating diarrhoeagenic bacteria. J Health Popul Nutr. (2012) 30(1):17–21. doi: 10.3329/jhpn.v30i1.11271
3. Sarsan S. Effect of storage of water in different metal vessels on coliforms. Int J Curr Microbiol Appl Sci. (2013) 2(11):24–9. PMID: 617104
4. Edlich RF, Amanda GJ, Cochran AA, Kelley AR, Dean GK, Olson BM, et al. Need for informed consent for dentists who use mercury amalgam restorative material as well as technical considerations in removal of dental amalgam restorations. J Environ Path Toxicol Oncol Off Organ Int Soc Environ Toxicol Cancer. (2007) 26(4):305–22. doi: 10.1615/JEnvironPatholToxicolOncol.v26.i4.70
5. Papazoglou E, Brantley WA, Johnston WM. Evaluation of high-temperature distortion of high-palladium metal-ceramic crowns - sciencedirect. J Prosthet Dent. (2001) 85(2):133–40. doi: 10.1067/mpr.2001.113707
6. Liu H, Tang YL, Zhang SY, Liu H, Wang ZJ, Li Y, et al. Anti-infection mechanism of a novel dental implant made of titanium-copper (ticu) alloy and its mechanism associated with oral microbiology. Bioactive Mater. (2021) 8:381–95. doi: 10.1016/j.bioactmat.2021.05.053
7. Shimizu H, Takahashi Y, Mckinney T. Resin-bonded castings with a cingulum rest seat and a guide plane for a removable partial denture: a case report. Quintessence Int (Berl). (2008) 39(1):e11–4. doi: 10.1111/j.1600-0757.2007.00243.x
8. Cucci LM, Satriano C, Marzo T, La MD. Angiogenin and copper crossing in wound healing. Int J Mol Sci. (2021) 22(19):10704–18. doi: 10.3390/ijms221910704
9. Wang LJ, Ni XH, Zhang F, Peng Z, Yu FX, Zhang LB, et al. Osteoblast response to copper-doped microporous coatings on titanium for improved bone integration. Nanoscale Res Lett. (2021) 16(1):146–61. doi: 10.1186/s11671-021-03602-2
10. Alizadeh S, Seyedalipour B, Shafieyan S, Kheime A, Mohammadi P, Aghdami N. Copper nanoparticles promote rapid wound healing in acute full thickness defect via acceleration of skin cell migration, proliferation, and neovascularization. Biochem Biophys Res Commun. (2019) 517(4):684–90. doi: 10.1016/j.bbrc.2019.07.110
11. Dollwet HHA, Sorenson JRJ. Roles of copper in bone maintenance and healing. Biol Trace Elem Res. (1988) 18(1):39–48. doi: 10.1007/BF02917487
12. Sen CK, Khanna S, Venojarvi M, Trikha P, Ellison EC, Hunt TK, et al. Copper-induced vascular endothelial growth factor expression and wound healing. AJP-Heart Circ Physiol. (2002) 282(5):H1821–7. doi: 10.1152/ajpheart.01015.2001
13. Habibovic P, Barralet JE. Bioinorganics and biomaterials: bone repair. Acta Biomater. (2011) 7(8):3013–26. doi: 10.1016/j.actbio.2011.03.027
14. Dupont CL, Grass G, Rensing C. Copper toxicity and the origin of bacterial resistance–new insights and applications. Metallomics. (2011) 3(11):1109–18. doi: 10.1039/c1mt00107h
15. Chatterjee AK, Sarkar RK, Chattopadhyay AP, Aich P, Chakraborty R, Basu T. A simple robust method for synthesis of metallic copper nanoparticles of high antibacterial potency against E. coli. Nanotechnology. (2012) 23(8):085103–14. doi: 10.1088/0957-4484/23/8/085103
16. Maung MT, Carlson A, Olea-Flores M, Elkhadragy L, Schachtschneider KM, Navarro-Tito N, et al. The molecular and cellular basis of copper dysregulation and its relationship with human pathologies. FASEB J. (2021) 35(9):21810–60. doi: 10.1096/fj.202100273RR
17. Sharan R, Chhibber S, Attri S, Reed RH. Inactivation and injury of escherichia coli in a copper water storage vessel: effects of temperature and ph. Antonie Van Leeuwenhoek. (2010) 97(1):91–7. doi: 10.1007/s10482-009-9395-7
18. Rosenberg M, Ilic K, Juganson K, Ivask A, Ahonen M, Vrček IV, et al. Potential ecotoxicological effects of antimicrobial surface coatings: a literature survey backed up by analysis of market reports. PeerJ. (2019) 7:e6315. doi: 10.7717/peerj.6315
19. Bondarenko O, Juganson K, Ivask A, Kasemets K, Mortimer M, Kahru A. Toxicity of ag, cuo and zno nanoparticles to selected environmentally relevant test organisms and mammalian cells in vitro: a critical review. Arch Toxicol. (2013) 87(7):1181–200. doi: 10.1007/s00204-013-1079-4
20. Amiri M, Etemadifar Z, Daneshkazemi A, Nateghi M. Antimicrobial effect of copper oxide nanoparticles on some oral bacteria and candida species. J Dent Biomater. (2017) 4(1):347–52. PMID: 28959764
21. Khan ST, Ahamed M, Al-Khedhairy A, Musarrat J. Biocidal effect of copper and zinc oxide nanoparticles on human oral microbiome and biofilm formation. Mater Lett. (2013) 97(8):67–70. doi: 10.1016/j.matlet.2013.01.085
22. Torres-Rosas R, Torres-Gómez N, Garcia-Contreras R, Scougall-Vilchis RJ, Argueta-Figueroa L. Copper nanoparticles as nanofillers in an adhesive resin system: an in vitro study. Dent Med Probl. (2020) 57(3):239–46. doi: 10.17219/dmp/121973
23. Vargas-Reus MA, Memarzadeh K, Jie H, Ren GG, Allaker RP. Antimicrobial activity of nanoparticulate metal oxides against peri-implantitis pathogens - sciencedirect. Int J Antimicrob Agents. (2012) 40(2):135–9. doi: 10.1016/j.ijantimicag.2012.04.012
24. Padmavathi AR, Das A, Priya A, Sushmitha TJ, Pandian SK, Toleti SR. Impediment to growth and yeast-to-hyphae transition in Candida albicans by copper oxide nanoparticles. Biofouling. (2020) 36(1):56–72. doi: 10.1080/08927014.2020.1715371
25. El Zowalaty M, Ibrahim NA, Salama M, Shameli K, Usman M, Zainuddin N. Synthesis, characterization, and antimicrobial properties of copper nanoparticles. Int J Nanomed. (2013) 8:4467–79. doi: 10.2147/IJN.S50837
26. Pugazhendhi A, Kumar SS, Manikandan M, Saravanan M. Photocatalytic properties and antimicrobial efficacy of Fe doped CuO nanoparticles against the pathogenic bacteria and fungi. Microb Pathog. (2018) 122:84–9. doi: 10.1016/j.micpath.2018.06.016
27. Krajczewski J, Rucinska K, Townley HE, Kudelski A. Role of various nanoparticles in photodynamic therapy and detection methods of singlet oxygen. Photodiagnosis Photodyn Ther. (2019) 26(JUN):162–78. doi: 10.1016/j.pdpdt.2019.03.016
28. Slavin YN, Asnis J, Hfeli UO, Bach H. Metal nanoparticles: understanding the mechanisms behind antibacterial activity. J Nanobiotechnol. (2017) 15(1):65–85. doi: 10.1186/s12951-017-0308-z
29. Erci F, Çakır Koç R, Yontem M, Torlak E. Synthesis of biologically active copper oxide nanoparticles as promising novel antibacterial-antibiofilm agents. Prep Biochem Biotechnol. (2020) 50(6):538–48. doi: 10.1080/10826068.2019.1711393
30. Sharif M, Rahman AU, Ahmed B, Rao ZA, Hassan FU. Copper nanoparticles as growth promoter, antioxidant and anti-bacterial agents in poultry nutrition: prospects and future implications. Biological Trace Element Research suppl. (2020) 11:1–12. doi: 10.1007/s12011-020-02485-1
31. Arakawa FS, Shimabuku-Biadola QL, Fernandes Silva M, Bergamasco R. Development of a new vacuum impregnation method at room atmosphere to produce silver-copper oxide nanoparticles on activated carbon for antibacterial applications. Environ Technol. (2019) 41(18):2400–11. doi: 10.1080/09593330.2019.1567607
32. Ghadiri AM, Rabiee N, Bagherzadeh M, Kiani M, Fatahi Y, Di Bartolomeo A, et al. Green synthesis of CuO- and CuO-NPs in assistance with high-gravity: The flowering of nanobiotechnology. Nanotechnology. (2020) 31(42):425101. doi: 10.1088/1361-6528/aba142
33. Giannousi K, Sarafidis G, Mourdikoudis S, Pantazaki A, Dendrinou-Sjamara C. Selective synthesis of cu2o and cu/cu2o nps: antifungal activity to yeast saccharomyces cerevisiae and dna interaction. Inorg Chem. (2014) 18:9657–66. doi: 10.1021/ic501143z
34. Singh A, Ahmed A, Prasad KN, Khanduja S, Gajbhiye NS. Antibiofilm and membrane-damaging potential of cuprous oxide nanoparticles against staphylococcus aureus with reduced susceptibility to vancomycin. Antimicrob Agents Chemother. (2015) 59(11):6882–90. doi: 10.1128/AAC.01440-15
35. Perdikaki A, Galeou A, Pilatos G, Karatasios I, Kanellopoulos NK, Prombona A, et al. Ag and Cu monometallic and Ag/Cu bimetallic nanoparticle–graphene composites with enhanced antibacterial performance. ACS Appl Mater Interfaces. (2016) 8(41):27498–510. doi: 10.1021/acsami.6b08403
36. Zhou X, Yin A, Sheng J, Wang J, Chen H, Fang Y, et al. In situ deposition of nano CuO on electrospun chitosan nanofibrous scaffolds and their antimicrobial properties. Int J Biol Macromol. (2021) 09:121–31. doi: 10.1016/j.ijbiomac.2021.09.121
37. Saleh SN, Khaffaga MM, Ali NM, Hassan MS, Rabie A. Antibacterial functionalization of cotton and cotton/polyester fabrics applying hybrid coating of copper/chitosan nanocomposites loaded polymer blends via gamma irradiation. Int J Biol Macromol. (2021) 183:23–34. doi: 10.1016/j.ijbiomac.2021.04.059
38. He W, Huang X, Zheng Y, Sun Y, Xie Y, Wang Y, et al. In situ synthesis of bacterial cellulose/copper nanoparticles composite membranes with long-term antibacterial property. J Biomater Sci, Polym Ed. (2018) 29(17):2137–53. doi: 10.1080/09205063.2018.1528518
39. Kong Y, Hou Z, Zhou L, Zhang P, Luo X. Injectable self-healing hydrogels containing cus nanoparticles with abilities of hemostasis, antibacterial activity, and promoting wound healing. ACS Biomater Sci Eng. (2020) 7(1):335–49. doi: 10.1021/acsbiomaterials.0c01473
40. Jayaramudu T, Varaprasad K, Pyarasani RD, Reddy KK, Amalraj J. Hydroxypropyl methylcellulose-copper nanoparticle and its nanocomposite hydrogel films for antibacterial application. Carbohydr Polym. (2020) 254:117302–32. doi: 10.1016/j.carbpol.2020.117302
41. Tran CD, Makuvaza J, Munson E, Bennett B. Biocompatible copper oxide nanoparticle composites from cellulose and chitosan: facile synthesis, unique structure, and antimicrobial activity. ACS Appl Mater Interfaces. (2017) 9(49):42503–15. doi: 10.1021/acsami.7b11969
42. Covarrubias C, Trepiana D, Corral C. Synthesis of hybrid copper-chitosan nanoparticles with antibacterial activity against cariogenic Streptococcus mutans. Dent Mater J. (2018) 37(3):379–84. doi: 10.4012/dmj.2017-195
43. No HK, Park NY, Lee SH, Meyers SP. Antibacterial activity of chitosans and chitosan oligomers with different molecular weights. Int J Food Microbiol. (2002) 74(1):65–72. doi: 10.1016/s0168-1605(01)00717-6
44. Tiwari M, Thakar MB, Jagani HV, Narayanan K, Venkata Rao J. Biosynthesis and wound healing activity of copper nanoparticles. IET Nanobiotechnol. (2014) 8(4):230–7. doi: 10.1049/iet-nbt.2013.0052
45. Costa EM, Silva S, Madureira AR, Cardelle-Cobas A, Tavaria FK, Pintado MM. A comprehensive study into the impact of a chitosan mouthwash upon oral microorganism's Biofilm formation in vitro. Carbohydr Polym. (2014) 101:1081–6. doi: 10.1016/j.carbpol.2013.09.041
46. Luo Z, Xue K, Zhang X, Lim JYC, Lai X, Young DJ, et al. Thermogelling chitosan-based polymers for the treatment of oral Mucosa ulcers. Biomater Sci. (2020) 8(5):1364–79. doi: 10.1039/C9BM01754B
47. Mishra RK, Selim A, Gowri V, Ahmad A, Nadeem A, Siddiqui N, et al. Thiol-functionalized cellulose-grafted copper oxide nanoparticles for the therapy of experimental colitis in Swiss albino mice. ACS Biomater Sci Eng. (2002) 8:2088–95. doi: 10.1021/acsbiomaterials.2c00124
48. Tao B, Lin C, Deng Y, Yuan Z, Shen X, Chen M, et al. Copper-nanoparticle-embedded hydrogel for killing bacteria and promoting wound healing with photothermal therapy. J Mater Chem B. (2019) 7(15):2534–48. doi: 10.1039/C8TB03272F
49. Fu Y, Chang F, Giedroc DP. Copper transport and trafficking at the host–bacterial pathogen interface. Acc Chem Res. (2014) 47(12):3605–13. doi: 10.1021/ar500300n
50. Djoko KY, Ong CLY, Walker MJ, Mcewan AG. The role of copper and zinc toxicity in innate immune defense against bacterial pathogens. J Biol Chem. (2015) 290(31):18954–61. doi: 10.1074/jbc.R115.647099
51. Ulloa-Ogaz AL, Piñón-Castillo HA, Muñoz-Castellanos LN, Athie-García MS, Orrantia-Borunda E. Oxidative damage to pseudomonas aeruginosa atcc 27833 and staphylococcus aureus atcc 24213 induced by cuo-nps. Environ Sci Pollut Res Int. (2018) 24(27):22048–60. doi: 10.1007/s11356-017-9718-6
52. Pramanik A, Laha D, Bhattacharya D, Pramanik P, Karmakar P. A novel study of antibacterial activity of copper iodide nanoparticle mediated by dna and membrane damage. Colloids Surf B Biointerfaces. (2012) 96:50–5. doi: 10.1016/j.colsurfb.2012.03.021
53. Denluck L, Wu F, Crandon LE, Harper B, Harper S. Reactive oxygen species generation is likely a driver of copper-based nanomaterial toxicity. Environ Sci. (2018) 5(6):1473–81. doi: 10.1039/C8EN00055G
54. Mutalik C, Okoro G, Krisnawati DI, Jazidie A, Rahmawati EQ, Rahayu D, et al. Copper sulfide with morphology-dependent photodynamic and photothermal antibacterial activities. J Colloid Interface Sci. (2021) 607:1825–35. doi: 10.1016/j.jcis.2021.10.019
55. Bao S, Lu Q, Fang T, Dai H, Zhang C. Assessment of the toxicity of cuo nanoparticles by using saccharomyces cerevisiae mutants with multiple genes deleted. Appl Environ Microbiol.. (2015) 81(23):8098–107. doi: 10.1128/AEM.02035-15
56. Yuzer B, Aydın MI, Con AH, Inan H, Can S, Selcuk H, et al. Photocatalytic, self-cleaning and antibacterial properties of Cu(II) doped TiO. J Environ Manage. (2021) 302:114023–33. doi: 10.1016/j.jenvman.2021.114023
57. Tsymbal SA, Moiseeva AA, Agadzhanian NA, Efimova SS, Markova AA, Guk DA, et al. Copper-containing nanoparticles and organic complexes: metal reduction triggers rapid cell death via oxidative burst. Int J Mol Sci. (2021) 22(20):11065–84. doi: 10.3390/ijms222011065
58. Wang X, Wang WX. Cu-based nanoparticle toxicity to zebrafish cells regulated by cellular discharges. Environ Pollut. (2021) 292:118296–316. doi: 10.1016/j.envpol.2021.118296
59. Morsy EA, Hussien AM, Ibrahim MA, Farroh KY, Hassanen EI. Cytotoxicity and genotoxicity of copper oxide nanoparticles in chickens. Biol Trace Elem Res. (2021) 23(01):1–15. doi: 10.1007/s12011-021-02595-4
60. Karlsson HL, Cronholm P, Hedberg Y, Tornberg M, De Battice L, Svedhem S, et al. Cell membrane damage and protein interaction induced by copper containing nanoparticles—importance of the metal release process. Toxicology. (2013) 313(1):59–69. doi: 10.1016/j.tox.2013.07.012
61. Wang L, Hu C, Shao L. The antimicrobial activity of nanoparticles: present situation and prospects for the future. Int J Nanomed. (2017) 12:1227–49. doi: 10.2147/IJN.S121956
62. Moloney JN, Cotter TG. Ros signalling in the biology of cancer. Semin Cell Dev Biol. (2018) 80:50–64. doi: 10.1016/j.semcdb.2017.05.023
63. Mangialasche F, Polidori MC, Monastero R, Ercolani S, Camarda C, Cecchetti R, et al. Biomarkers of oxidative and nitrosative damage in Alzheimer's Disease and mild cognitive impairment. Ageing Res Rev. (2009) 8(4):285–305. doi: 10.1016/j.arr.2009.04.002
64. Gunther MR, Hanna PM, Mason RP, Cohen MS. Hydroxyl radical formation from cuprous ion and hydrogen peroxide: a spin-trapping study. Arch Biochem Biophys. (1995) 316(1):515–22. doi: 10.1006/abbi.1995.1068
65. Ezraty B, Gennaris A, Barras F, Collet J-F. Oxidative stress, protein damage and repair in bacteria. Nat Rev Microbiol. (2017) 15(7):385–96. doi: 10.1038/nrmicro.2017.26
66. Cheloni G, Marti E, Slaveykova VI. Interactive effects of copper oxide nanoparticles and light to green alga Chlamydomonas reinhardtii. Aquat Toxicol. (2016) 170:120–8. doi: 10.1016/j.aquatox.2015.11.018
67. Flemming CA, Trevors JT. Copper toxicity and chemistry in the environment: a review. Water, Air, Soil Pollut. (1989) 44(1-2):143–58. doi: 10.1007/BF00228784
68. Wang Z, Li N, Zhao J, White JC, Qu P, Xing B. Cuo nanoparticle interaction with human epithelial cells: cellular uptake, location, export, and genotoxicity. Chem Res Toxicol. (2012) 25(7):1512–21. doi: 10.1021/tx3002093
69. Sabella S, Carney RP, Brunetti V, Malvindi MA, Al-Juffali N, Vecchio G, et al. A general mechanism for intracellular toxicity of metal-containing nanoparticles. Nanoscale. (2014) 6(12):7052–61. doi: 10.1039/c4nr01234h
70. Hsiao I-L, Hsieh Y-K, Wang C-F, Chen I-C, Huang Y-J. Trojan-horse mechanism in the cellular uptake of silver nanoparticles verified by direct intra- and extracellular silver speciation analysis. Environ Sci Technol. (2015) 49(6):3813–21. doi: 10.1021/es504705p
71. Jose GP, Santra S, Mandal SK, Sengupta TK. Singlet oxygen mediated DNA degradation by copper nanoparticles: potential towards cytotoxic effect on cancer cells. J Nanobiotechnol. (2011) 9(1):9–17. doi: 10.1186/1477-3155-9-9
72. Soenen SJ, Rivera-Gil P, Montenegro J-M, Parak WJ, De Smedt SC, Braeckmans K. Cellular toxicity of inorganic nanoparticles: common aspects and guidelines for improved nanotoxicity evaluation. Nano Today. (2011) 6(5):446–65. doi: 10.1016/j.nantod.2011.08.001
73. Espana-Sanchez BL, Avila-Orta CA, Padilla-Vaca LF, Barriga-Castro ED, Soriano-Corral F, Gonzalez-Morones P, et al. Early stages of antibacterial damage of metallic nanoparticles by TEM and STEM-HAADF. Curr Nanosci. (2017) 14(1):54–61. doi: 10.2174/2468187307666170906150731
74. Sánchez-López E, Gomes D, Esteruelas G, Bonilla L, Lopez-Machado AL, Galindo R, et al. Metal-based nanoparticles as antimicrobial agents: an overview. Nanomaterials (Basel). (2020) 10(2):292–335. doi: 10.3390/nano10020292
75. Mitra D, Kang E-T, Neoh KG. Antimicrobial copper-based materials and coatings: potential multifaceted biomedical applications. ACS Appl Mater Interfaces. (2019) 12(19):21159–82. doi: 10.1021/acsami.9b17815
76. Santo CE, Lam EW, Elowsky CG, Quaranta D, Domaille DW, Chang CJ, et al. Bacterial killing by dry metallic copper surfaces. Appl Environ Microbiol. (2010) 77(3):794–802. doi: 10.1128/AEM.01599-10
77. Warnes SL, Caves V, Keevil CW. Mechanism of copper surface toxicity in Escherichia coli O157:H7 and Salmonella involves immediate membrane depolarization followed by slower rate of DNA destruction which differs from that observed for Gram-positive bacteria. Environ Microbiol. (2011) 14(7):1730–43. doi: 10.1111/j.1462-2920.2011.02677.x
78. Hong R, Kang TY, Michels CA, Gadura N. Membrane lipid peroxidation in copper alloy-mediated contact killing of Escherichia coli. Appl Environ Microbiol. (2012) 78(6):1776–84. doi: 10.1128/AEM.07068-11
79. Aruoma OI, Halliwell B, Gajewski E, Dizdaroglu M. Copper-ion-dependent damage to the bases in DNA in the presence of hydrogen peroxide. Biochem J. (1991) 273(3):601–4. doi: 10.1042/bj2730601
80. Outten FW, Huffman DL, Hale JA, O’Halloran TV. The independent cue and cussystems confer copper tolerance during aerobic and anaerobic growth in Escherichia coli. J Biol Chem. (2001) 276(33):30670–7. doi: 10.1074/jbc.M104122200
81. Tan G, Yang J, Li T, Zhao J, Sun S, Li X, et al. Anaerobic copper toxicity and iron-sulfur cluster biogenesis in escherichia coli. Appl Environ Microbiol. (2017) 83(16):00867–17. doi: 10.1128/AEM.00867-17
82. Macomber L, Rensing C, Imlay JA. Intracellular copper does not catalyze the formation of oxidative DNA damage in Escherichia coli. J Bacteriol. (2006) 189(5):1616–26. doi: 10.1128/JB.01357-06
83. Macomber L, Imlay JA. The iron-sulfur clusters of dehydratases are primary intracellular targets of copper toxicity. Proc Natl Acad Sci USA. (2009) 106(20):8344–9. doi: 10.1073/pnas.0812808106
84. Tan G, Cheng Z, Pang Y, Landry AP, Li J, Lu J, et al. Copper binding in IscA inhibits iron-sulphur cluster assembly in Escherichia coli. Mol Microbiol. (2014) 93(4):629–44. doi: 10.1111/mmi.12676
85. Kaweeteerawat C, Chong HC, Roy KR, Rong L, Godwin HA. Cu nanoparticles have different impacts in escherichia coli and lactobacillus brevis than their microsized and ionic analogues. Acs Nano. (2015) 9(7):7215–25. doi: 10.1021/acsnano.5b02021
86. Adeyemi JA, Machado ART, Ogunjimi AT, Alberici LC, Antunes LMG, Barbosa F. Cytotoxicity, mutagenicity, oxidative stress and mitochondrial impairment in human hepatoma (HepG2) cells exposed to copper oxide, copper-iron oxide and carbon nanoparticles. Ecotoxicol Environ Saf. (2020) 189:109982–91. doi: 10.1016/j.ecoenv.2019.109982
87. Chatterjee AK, Chakraborty R, Basu T. Mechanism of antibacterial activity of copper nanoparticles. Nanotechnology. (2014) 25(13):135101–12. doi: 10.1088/0957-4484/25/13/135101
88. Basavegowda N, Baek KH. Multimetallic nanoparticles as alternative antimicrobial agents: challenges and perspectives. Molecules. (2021) 26(4):912–33. doi: 10.3390/molecules26040912
89. Silva B, Abuafy MP, Manaia EB, Junior J, Chiari-Andréo BG, Pietro R, et al. Relationship between structure and antimicrobial activity of zinc oxide nanoparticles: an overview. Int J Nanomed. (2019) 14:9395–410. doi: 10.2147/IJN.S216204
90. Khezerlou A, Alizadeh-Sani M, Azizi-Lalabadi M, Ehsani A. Nanoparticles and their antimicrobial properties against pathogens including bacteria, fungi, parasites and viruses. Microb Pathog. (2018) 123:505–26. doi: 10.1016/j.micpath.2018.08.008
91. Cheon JY, Kim SJ, Rhee YH, Kwon OH, Park WH. Shape-dependent antimicrobial activities of silver nanoparticles. Int J Nanomed. (2019) 14:2773–80. doi: 10.2147/IJN.S196472
92. Černík M, Thekkae Padil VV. Green synthesis of copper oxide nanoparticles using gum karaya as a biotemplate and their antibacterial application. Int J Nanomed. (2013) 8:889–98. doi: 10.2147/IJN.S40599
93. Azam A, Ahmed AS, Oves M, Khan MS, Memic A. Size-dependent antimicrobial properties of CuO nanoparticles against gram-positive and -negative bacterial strains. Int J Nanomed. (2012) 7:3527–35. doi: 10.2147/IJN.S29020
94. Applerot G, Lellouche J, Lipovsky A, Nitzan Y, Lubart R, Gedanken A, et al. Understanding the antibacterial mechanism of CuO nanoparticles: revealing the route of induced oxidative stress. Small. (2012) 8(21):3326–37. doi: 10.1002/smll.201200772
95. Xiong L, Yu H, Nie C, Xiao Y, Zeng Q, Wang G, et al. Size-controlled synthesis of Cu2O nanoparticles: size effect on antibacterial activity and application as a photocatalyst for highly efficient H2O2 evolution. RSC Adv. (2017) 7(82):51822–30. doi: 10.1039/C7RA10605J
96. Cha S-H, Hong J, McGuffie M, Yeom B, VanEpps JS, Kotov NA. Shape-dependent biomimetic inhibition of enzyme by nanoparticles and their antibacterial activity. ACS Nano. (2015) 9(9):9097–105. doi: 10.1021/acsnano.5b03247
97. Pang H, Gao F, Lu Q. Morphology effect on antibacterial activity of cuprous oxide. Chem Commun. (2009) 9(9):1076–8. doi: 10.1039/b816670f
98. Yan W, Fu X, Gao Y, Shi L, Liu Q, Yang W, et al. Synthesis, antibacterial evaluation, and safety assessment of CuS NPs against Pectobacterium carotovorum subsp. Carotovorum. Pest Manage Sci. (2021) 24(10):6686–96. doi: 10.1002/ps.6686
99. Xiong L, Tong Z-H, Chen J-J, Li L-L, Yu H-Q. Morphology-dependent antimicrobial activity of Cu/CuxO nanoparticles. Ecotoxicology. (2015) 24(10):2067–72. doi: 10.1007/s10646-015-1554-1
100. Feng Y, Chang Y, Sun X, Liu N, Cheng Y, Feng Y, et al. Understanding the property-activity relationships of polyhedral cuprous oxide nanocrystals in terms of reactive crystallographic facets. Toxicol Sci. (2017) 156(2):480–91. doi: 10.1093/toxsci/kfx011
101. Jung H-Y, Seo Y, Park H, Huh Y-D. Morphology-controlled synthesis of octahedral-to-rhombic dodecahedral Cu2O microcrystals and shape-dependent antibacterial activities. Bull Korean Chem Soc. (2015) 36(7):1828–33. doi: 10.1002/bkcs.10351
102. Lee Y-J, Kim S, Park S-H, Park H, Huh Y-D. Morphology-dependent antibacterial activities of Cu2O. Mater Lett. (2011) 65(5):818–20. doi: 10.1016/j.matlet.2010.12.023
103. Ren J, Wang W, Sun S, Zhang L, Wang L, Chang J. Crystallography facet-dependent antibacterial activity: the case of Cu2O. Ind Eng Chem Res. (2011) 50(17):10366–9. doi: 10.1021/ie2005466
104. Studer AM, Limbach LK, Van Duc L, Krumeich F, Athanassiou EK, Gerber LC, et al. Nanoparticle cytotoxicity depends on intracellular solubility: comparison of stabilized copper metal and degradable copper oxide nanoparticles. Toxicol Lett. (2010) 197(3):169–74. doi: 10.1016/j.toxlet.2010.05.012
105. Hans M, Mathews S, Mücklich F, Solioz M. Physicochemical properties of copper important for its antibacterial activity and development of a unified model. Biointerphases. (2016) 11(1):018902–09. doi: 10.1116/1.4935853
106. Tian W-X, Yu S, Ibrahim M, Almonaofy AW, He L, Hui Q, et al. Copper as an antimicrobial agent against opportunistic pathogenic and multidrug resistant Enterobacter bacteria. J Microbiol. (2012) 50(4):586–93. doi: 10.1007/s12275-012-2067-8
107. Rosenberg M, Vija H, Kahru A, Keevil CW, Ivask A. Rapid in situ assessment of Cu-ion mediated effects and antibacterial efficacy of copper surfaces. Sci Rep. (2018) 8(1):8172–83. doi: 10.1038/s41598-018-26391-8
108. Phogat N, Kohl M, Uddin I. Interaction of nanoparticles with biomolecules, protein, enzymes, and its applications. Precis Med. (2018) 2018:253–76. doi: 10.1016/B978-0-12-805364-5.00011-1
109. Badetti E, Calgaro L, Falchi L, Bonetto A, Bettiol C, Leonetti B, et al. Interaction between copper oxide nanoparticles and amino acids: influence on the antibacterial activity. Nanomaterials. (2019) 9(5):792–801. doi: 10.3390/nano9050792
110. Ruparelia JP, Chatterjee AK, Duttagupta SP, Mukherji S. Strain specificity in antimicrobial activity of silver and copper nanoparticles. Acta Biomater. (2008) 4(3):707–16. doi: 10.1016/j.actbio.2007.11.006
111. Lv Y, Li L, Yin P, Lei T. Synthesis and evaluation of the structural and antibacterial properties of doped copper oxide. Dalton Trans. (2020) 49(15):4699–709. doi: 10.1039/D0DT00201A
112. Malka E, Perelshtein I, Lipovsky A, Shalom Y, Gedanken A. Eradication of multi-drug resistant bacteria by a novel zn-doped cuo nanocomposite. Small. (2013) 9(23):4069–76. doi: 10.1002/smll.201301081
113. Kim HE, Lee HJ, Kim MS, Kim T, Lee H, Kim HH, et al. Differential microbicidal effects of bimetallic iron-copper nanoparticles on Escherichia coli and MS2 coliphage. Environ Sci Technol. (2019) 53(5):2679–87. doi: 10.1021/acs.est.8b06077
114. Akhavan O, Ghaderi E. Cu and CuO nanoparticles immobilized by silica thin films as antibacterial materials and photocatalysts. Surf Coat Technol. (2010) 205(1):219–23. doi: 10.1016/j.surfcoat.2010.06.036
115. Hans M, Erbe A, Mathews S, Chen Y, Solioz M, Mücklich F. Role of copper oxides in contact killing of Bacteria. Langmuir. (2013) 29(52):16160–6. doi: 10.1021/la404091z
116. Kubo AL, Vasiliev G, Vija H, Krishtal J, Tugu V, Visnapuu M, et al. Surface carboxylation or pegylation decreases cuo nanoparticles’ cytotoxicity to human cells in vitro without compromising their antibacterial properties. Arch Toxicol. (2020) 94(5):1561–73. doi: 10.1007/s00204-020-02720-7
117. Qi F, Chang Y, Zheng R, Wu X, Wu Y, Li B, et al. Copper phosphide nanoparticles used for combined photothermal and photodynamic tumor therapy. ACS Biomater Sci Eng. (2021) 7(6):2745–54. doi: 10.1021/acsbiomaterials.1c00189
118. Ishida N, Hosokawa Y, Imaeda T, Hatanaka T. Reduction of the cytotoxicity of copper (II) oxide nanoparticles by coating with a surface-binding peptide. Appl Biochem Biotechnol. (2019) 190(2):645–59. doi: 10.1007/s12010-019-03108-9
119. Reddy ARN, Lonkala S. In vitro evaluation of copper oxide nanoparticle-induced cytotoxicity and oxidative stress using human embryonic kidney cells. Toxicol Ind Health. (2019) 35(2):159–64. doi: 10.1177/0748233718819371
120. Piret JP, Jacques D, Audinot JN, Mejia J, Toussaint O. Copper(ii) oxide nanoparticles penetrate into hepg2 cells, exert cytotoxicity via oxidative stress and induce pro-inflammatory response. Nanoscale. (2012) 4(22):7168–84. doi: 10.1039/c2nr31785k
121. Kardos J, Héja L, Simon Á, Jablonkai I, Kovács R, Jemnitz K. Copper signalling: causes and consequences. Cell Commun Signal. (2018) 16(1):71–93. doi: 10.1186/s12964-018-0277-3
122. Alghobashy AA, Alkholy UM, Talat MA, Abdalmonem N, Zaki A, Ahmed IA, et al. Trace elements and oxidative stress in children with type 1 diabetes mellitus. Diab Metab Syndr Obes-Targets and Ther. (2018) 11:85–92. doi: 10.2147/DMSO.S157348
123. Angelé-Martínez C, Nguyen KV, Ameer FS, Anker JN, Brumaghim JL. Reactive oxygen species generation by copper(II) oxide nanoparticles determined by DNA damage assays and EPR spectroscopy. Nanotoxicology. (2017) 11:278–88. doi: 10.1080/17435390.2017.1293750
124. Bulcke F, Dringen R, Scheiber IF. Neurotoxicity of copper. Adv Neurobiol. (2017) 18:313–43. doi: 10.1007/978-3-319-60189-2_16
125. Gaetke LM, Chow-Johnson HS, Chow CK. Copper: toxicological relevance and mechanisms. Arch Toxicol. (2014) 88:1929–38. doi: 10.1007/s00204-014-1355-y
126. Ladomersky E, Petris MJ. Copper tolerance and virulence in bacteria. Metallomics. (2015) 7(6):957–64. doi: 10.1039/C4MT00327F
127. Sadiq S, Ghazala Z, Chowdhury A, Büsselberg D. Metal toxicity at the synapse: presynaptic, postsynaptic, and long-term effects. J Toxicol. (2012) 2012:132671–713. doi: 10.1155/2012/132671
128. Semprine J, Ferrarotti N, Musacco-Sebio R, Saporito-Magriñá C, Fuda J, Torti H, et al. Brain antioxidant responses to acute iron and copper intoxications in rats. Metallomics. (2014) 6(11):2083–92. doi: 10.1039/C4MT00159A
129. Shimberg GD, Ok K, Neu HM, Splan KE, Michel SLJ. Cu(I) disrupts the structure and function of the nonclassical zinc finger protein tristetraprolin (TTP). Inorg Chem. (2017) 56:6838–48. doi: 10.1021/acs.inorgchem.7b00125
130. Stepankov MS, Zemlyanova MA, Zaitseva NV, Ignatova AM, Nikolaeva AE. Features of bioaccumulation and toxic effects of copper (II) oxide nanoparticles under repeated oral exposure in rats. Pharm Nanotechnol. (2021) 9(4):288–97. doi: 10.2174/2211738509666210728163901
131. Tang H, Xu M, Zhou X, Zhang Y, Zhao L, Ye G, et al. Acute toxicity and biodistribution of different sized copper nano-particles in rats after oral administration. Mater Sci Eng C. (2018) 93:649–63. doi: 10.1016/j.msec.2018.08.032
132. Ameh T, Sayes CM. The potential exposure and hazards of copper nanoparticles: a review. Environ Toxicol Pharmacol. (2019) 71:103220–30. doi: 10.1016/j.etap.2019.103220
133. Chen Z, Meng H, Xing G, Chen C, Zhao Y, Jia G, et al. Acute toxicological effects of copper nanoparticles in vivo. Toxicol Lett. (2006) 163(2):109–20. doi: 10.1016/j.toxlet.2005.10.003
134. Di Bucchianico S, Fabbrizi MR, Misra SK, Valsami-Jones E, Berhanu D, Reip P, et al. Multiple cytotoxic and genotoxic effects induced in vitro by differently shaped copper oxide nanomaterials. Mutagenesis. (2013) 3:287–99. doi: 10.1093/mutage/get014
135. Scharnow AM, Solinski AE, Wuest WM. Targeting S. mutans biofilms: a perspective on preventing dental caries. MedChemComm. (2019) 10(7):1057–67. doi: 10.1039/C9MD00015A
136. Islam B, Khan SN, Khan AU. Dental caries: from infection to prevention. Medical Science Monitor: Int Med J Exp Clin Res. (2007) 13(11):196–203. doi: 10.1051/medsci/200723111063
137. Forssten SD, Bjrklund M, Ouwehand AC. Streptococcus mutans, caries and simulation models. Nutrients. (2010) 2(3):290–8. doi: 10.3390/nu2030290
138. Taubman MA, Nash DA. The scientific and public-health imperative for a vaccine against dental caries. Nat Rev Immunol. (2006) 6(7):555–63. doi: 10.1038/nri1857
139. Krzyściak W, Jurczak A, Kościelniak D, Bystrowska B, Skalniak A. The virulence of streptococcus mutans and the ability to form biofilms. Eur J Clin Microbiol Infect Dis. (2014) 33(4):499–515. doi: 10.1007/s10096-013-1993-7
140. Grytten J, Tollefsen T, Afseth J. The effect of a combination of copper and hexetidine on plaque formation and the amount of copper retained by dental plaque bacteria. Acta Odontol Scand. (1987) 45(6):429–33. doi: 10.3109/00016358709096368
141. Drake DR, Grigsby W, Cardenzana A, Dunkerson D. Synergistic, growth-inhibitory effects of chlorhexidine and copper combinations on streptococcus mutans, actinomyces viscosus, and actinomyces naeslundii. J Dent Res. (1993) 72(2):524–8. doi: 10.1177/00220345930720020901
142. Afseth J, Oppermann RV, Rolla G. Thein vivoeffect of glucose solutions containing Cu++ and Zn++ on the acidogenicity of dental plaque. Acta Odontol Scand. (1980) 38(4):229–33. doi: 10.3109/00016358009003494
143. Maltz M, Emilson CG. Effect of copper fluoride and copper sulfate on dental plaque, Streptococcus mutans and caries in hamsters. Eur J Oral Sci. (1988) 96(5):390–2. doi: 10.1111/j.1600-0722.1988.tb01572.x
144. Singh K, Senadheera DB, Lévesque CM, Cvitkovitch DG. The copYAZ operon functions in copper efflux, biofilm formation, genetic transformation, and stress tolerance in streptococcus mutans. J Bacteriol. (2015) 197(15):2545–57. doi: 10.1128/JB.02433-14
145. Devulapalle KS, Mooser G. Glucosyltransferase inactivation reduces dental caries. J Dent Res. (2001) 80(2):1505–6. doi: 10.1177/00220345010800021301
146. Vats N, Song FL. Characterization of a copper-transport operon, copyaz, from streptococcus mutans. Microbiology. (2001) 147(Pt 3):653–62. doi: 10.1099/00221287-147-3-653
147. Garcia SS, Qin D, Hui W. Streptococcus mutans copper chaperone, copz, is critical for biofilm formation and competitiveness. Mol Oral Microbiol. (2016) 31(6):515–25. doi: 10.1111/omi.12150
148. Eshed M, Lellouche J, Matalon S, Gedanken A, Banin E. Sonochemical coatings of ZnO and CuO nanoparticles inhibit Streptococcus mutans biofilm formation on teeth model. Langmuir. (2012) 28(33):12288–95. doi: 10.1021/la301432a
149. Howard KC, Gonzalez OA, Garneau-Tsodikova S. Porphyromonas gingivalis: where do we stand in our battle against this oral pathogen? R Soc Chem Med Chem. (2021) 12(5):666–704. doi: 10.1039/d0md00424c
150. Mulhall H, Huck O, Amar S. Porphyromonas gingivalis, a long-range pathogen: systemic impact and therapeutic implications. Microorganisms. (2020) 8(6):869–81. doi: 10.3390/microorganisms8060869
151. Xie M, Tang Q, Nie J, Zhang C, Zhou X, Yu S, et al. BMAL1-downregulation aggravates porphyromonas gingivalis-induced atherosclerosis by encouraging oxidative stress. Circ Res. (2020) 126:e15–29. doi: 10.1161/CIRCRESAHA.119.315502
152. Bahar B, Kanagasingam S, Tambuwala MM, Aljabali AAA, Dillon SA, Doaei S, et al. Porphyromonas gingivalis (W83) infection induces Alzheimer's disease-like pathophysiology in obese and diabetic mice. J Alzheimer Dis. (2021) 82(3):1259–75. doi: 10.3233/JAD-210465
153. Zhou N, Zou F, Cheng X, Huang Y, Zou H, Niu Q, et al. Porphyromonas gingivalis induces periodontitis, causes immune imbalance, and promotes rheumatoid arthritis. J Leukocyte Biol. (2021) 110(3):461–73. doi: 10.1002/JLB.3MA0121-045R
154. Liu R, Memarzadeh K, Chang B, Zhang Y, Ma Z, Allaker RP, et al. Antibacterial effect of copper-bearing titanium alloy (Ti-Cu) against Streptococcus mutans and Porphyromonas gingivalis. Sci Rep. (2016) 6:29985–94. doi: 10.1038/srep29985
155. Bai B, Zhang E, Liu J, Zhu J. The anti-bacterial activity of titanium-copper sintered alloy against Porphyromonas gingivalis in vitro. Dent Mater J. (2016) 35(4):659–67. doi: 10.4012/dmj.2016-001
156. Tamura M, Ochiai K. Zinc and copper play a role in coaggregation inhibiting action ofPorphyromonas gingivalis. Oral Microbiol Immunol. (2009) 24(1):56–63. doi: 10.1111/j.1399-302X.2008.00476.x
157. Mothibe JV, Patel M. Pathogenic characteristics of Candida albicans isolated from oral cavities of denture wearers and cancer patients wearing oral prostheses. Microb Pathog. (2017) 110:128–34. doi: 10.1016/j.micpath.2017.06.036
158. Buranarom N, Komin O, Matangkasombut O. Hyposalivation, oral health, and candida colonization in independent dentate elders. PLoS ONE. (2020) 15(11):0242832–850. doi: 10.1371/journal.pone.0242832
159. Lara HH, Guisbiers G, Mendoza J, Mimun LC, Vincent B, Lopez-Ribot JL, et al. Synergistic antifungal effect of chitosan-stabilized selenium nanoparticles synthesized by pulsed laser ablation in liquids against Candida albicans biofilms. Int J Nanomed. (2018) 13:2697–708. doi: 10.2147/IJN.S151285
160. Argueta-Figueroa L, Scougall-Vilchis RJ, Morales-Luckie RA, Olea-Mejía OF. An evaluation of the antibacterial properties and shear bond strength of copper nanoparticles as a nanofiller in orthodontic adhesive. Aust Orthod J. (2015) 31(1):42–50. doi: 10.21307/aoj-2020-139
161. Cao J, Yang DL, Wang D, Wang JX. Spray-dryingassisted fabrication of CaF/SiO nanoclusters for dental restorative composites. Dent Mater. (2022) 38(5):835–47. doi: 10.1016/j.dental.2022.04.007
162. De Souza AP, Gerlach RF, Line SRP. Inhibition of human gingival gelatinases (MMP-2 and MMP-9) by metal salts. Dent Mater. (2000) 16(2):103–8. doi: 10.1016/S0109-5641(99)00084-6
163. Hassona Y, Cirillo N, Heesom K, Parkinson EK, Prime SS. Senescent cancer-associated fibroblasts secrete active MMP-2 that promotes keratinocyte dis-cohesion and invasion. Br J Cancer. (2014) 111(6):1230–7. doi: 10.1038/bjc.2014.438
164. Zee E, Everts V, Beertsen W. Cytokine-induced endogenous procollagenase stored in the extracellular matrix of soft connective tissue results in a burst of collagen breakdown following its activation. J Periodontal Res. (1996) 31(7):483–8. doi: 10.1111/j.1600-0765.1996.tb01413.x
165. Tjäderhane L, Larjava H, Sorsa T, Uitto V-J, Larmas M, Salo T. The activation and function of host matrix metalloproteinases in dentin matrix breakdown in caries lesions. J Dent Res. (1998) 77(8):1622–9. doi: 10.1177/00220345980770081001
166. De Munck J, Mine A, Van den Steen PE, Van Landuyt KL, Poitevin A, Opdenakker G, et al. Enzymatic degradation of adhesive-dentin interfaces produced by mild self-etch adhesives. Eur J Oral Sci. (2010) 118(5):494–501. doi: 10.1111/j.1600-0722.2010.00758.x
167. Mazzoni A, Scaffa P, Carrilho M, Tjaderhane L, Lenarda RD, Polimeni A, et al. Effects of etch-and-rinse and self-etch adhesives on dentin mmp-2 and mmp-9. J Dent Res. (2012) 92(1):82–6. doi: 10.1177/0022034512467034
168. Almahdy A, Koller G, Sauro S, Bartsch JW, Sherriff M, Watson TF, et al. Effects of mmp inhibitors incorporated within dental adhesives. J Dent Res. (2012) 91(6):605–11. doi: 10.1177/0022034512446339
169. Serkies KB, Garcha R, Tam LE, De Souza GM, Finer Y. Matrix metalloproteinase inhibitor modulates esterase-catalyzed degradation of resin–dentin interfaces. Dent Mater. (2016) 32(12):1513–23. doi: 10.1016/j.dental.2016.09.007
170. Mazzoni A, Tjäderhane L, Checchi V, Di Lenarda R, Salo T, Tay FR, et al. Role of dentin MMPs in caries progression and bond stability. J Dent Res. (2014) 94(2):241–51. doi: 10.1177/0022034514562833
171. Maciejczyk M, Pietrzykowska A, Zalewska A, Kna M, Daniszewska I. The significance of matrix metalloproteinases in oral diseases. Adv Clin Exp Med. (2016) 25(2):383–90. doi: 10.17219/acem/30428
172. Femiano F, Femiano R, Femiano L, Jamilian A, Rullo R, Perillo L. Dentin caries progression and the role of metalloproteinases: an update. Eur J Paediatr Dent. (2016) 17(3):243–7. PMID: 27759416
173. Gutiérrez MF, Bermudez J, Dávila-Sánchez A, Alegría-Acevedo LF, Méndez-Bauer L, Hernández M, et al. Zinc oxide and copper nanoparticles addition in universal adhesive systems improve interface stability on caries-affected dentin. J Mech Behav Biomed Mater. (2019) 100:103366–75. doi: 10.1016/j.jmbbm.2019.07.024
174. Vidal O, de Paris MT, Núñez A, Méndez-Bauer L, Sutil E, Ñaupari-Villasante R, et al. A universal adhesive containing copper nanoparticles improves the stability of hybrid layer in a cariogenic oral environment: an in situ study. J Mech Behav Biomed Mater. (2022) 126:105017–27. doi: 10.1016/j.jmbbm.2021.105017
175. Javed R, Rais F, Kaleem M, Jamil B, Ahmad MA, Yu T, et al. Chitosan capping of CuO nanoparticles: facile chemical preparation, biological analysis, and applications in dentistry. Int J Biol Macromol. (2021) 167:1452–67. doi: 10.1016/j.ijbiomac.2020.11.099
176. Gutiérrez MF, Malaquias P, Hass V, Matos TP, Lourenço L, Reis A, et al. The role of copper nanoparticles in an etch-and-rinse adhesive on antimicrobial activity, mechanical properties and the durability of resin-dentine interfaces. J Dent. (2017) 61:12–20. doi: 10.1016/j.jdent.2017.04.007
177. Jun SK, Yang SA, Kim YJ, El-Fiqi A, Mandakhbayar N, Kim DS, et al. Multi-functional nano-adhesive releasing therapeutic ions for MMP-deactivation and remineralization. Sci Rep. (2018) 8(1):5663. doi: 10.1038/s41598-018-23939-6
178. Sabatini C, Mennito AS, Wolf BJ, Pashley DH, Renné WG. Incorporation of bactericidal poly-acrylic acid modified copper iodide particles into adhesive resins. J Dent. (2015) 43(5):546–55. doi: 10.1016/j.jdent.2015.02.012
179. Matos TP, Gutiérrez MF, Hanzen TA, Malaquias P, de Paula AM, de Souza JJ, et al. 18-month Clinical evaluation of a copper-containing universal adhesive in non-carious cervical lesions: a double-blind, randomized controlled trial. J Dent. (2019) 90:103219–38. doi: 10.1016/j.jdent.2019.103219
180. Toodehzaeim MH, Zandi H, Meshkani H, Firouzabadi AH. The effect of cuo nanoparticles on antimicrobial effects and shear bond strength of orthodontic adhesives. J Dent. (2018) 19(1):1–5. PMID: 29492409
181. Renné WG, Lindner A, Mennito AS, Agee KA, Pashley DH, Willett D, et al. Antibacterial properties of copper iodide-doped glass ionomer-based materials and effect of copper iodide nanoparticles on collagen degradation. Clin Oral Investig. (2016) 21(1):369–79. doi: 10.1007/s00784-016-1799-y
182. Aguilar-Perez D, Vargas-Coronado R, Cervantes-Uc JM, Rodriguez-Fuentes N, Aparicio C, Covarrubias C, et al. Antibacterial activity of a glass ionomer cement doped with copper nanoparticles. Dent Mater J. (2020) 39(3):389–96. doi: 10.4012/dmj.2019-046
183. Morones JR, Elechiguerra JL, Camacho A, Holt K, Kouri JB, Ramírez JT, et al. The bactericidal effect of silver nanoparticles. Nanotechnology. (2005) 16(10):2346–53. doi: 10.1088/0957-4484/16/10/059
184. Lubojanski A, Dobrzynski M, Nowak N, Rewak-Soroczynska J, Wiglusz RJ. Application of selected nanomaterials and ozone in modern clinical dentistry. Nanomaterials. (2021) 11(2):259–99. doi: 10.3390/nano11020259
185. Sakka S, Baroudi K, Nassani MZ. Factors associated with early and late failure of dental implants. J Investig Clin Dent. (2012) 3(4):258–61. doi: 10.1111/j.2041-1626.2012.00162.x
186. Zzab C, Ywab C, Wstab C, Xzab C, Yy F, Hao Z, et al. An orthobiologics-free strategy for synergistic photocatalytic antibacterial and osseointegration. Biomaterials. (2021) 274:120853–70. doi: 10.1016/j.biomaterials.2021.120853
187. Xia C, Cai D, Tan J, Li K, Qiao Y, Liu X. Synergistic effects of N/Cu dual ions implantation on stimulating antibacterial ability and angiogenic activity of titanium. ACS Biomater Sci Eng. (2018) 4(9):1–40. doi: 10.1021/acsbiomaterials.8b00501
188. Singh Gill A, Morrissey H, Rahman A. A systematic review and meta-analysis evaluating antibiotic prophylaxis in dental implants and extraction procedures. Medicina (B Aires). (2018) 54(6):95–112. doi: 10.3390/medicina54060095
189. Zhuang Y, Ren L, Zhang S, Wei X, Yang K, Dai K. Antibacterial effect of a copper-containing titanium alloy against implant-associated infection induced by methicillin-resistant Staphylococcus aureus. Acta Biomater. (2021) 119:472–84. doi: 10.1016/j.actbio.2020.10.026
190. Liu W, Li J, Cheng M, Wang Q, Qian Y, Yeung KW, et al. A surface-engineered polyetheretherketone biomaterial implant with direct and immunoregulatory antibacterial activity against methicillin-resistant staphylococcus aureus. Biomaterials. (2019) 208:8–20. doi: 10.1016/j.biomaterials.2019.04.008
191. Zhang X, Li J, Wang X, Wang Y, Hang R, Huang X, et al. Effects of copper nanoparticles in porous TiO 2 coatings on bacterial resistance and cytocompatibility of osteoblasts and endothelial cells. Mater Sci Eng C Mater Biol Appl. (2018) 82:110–20. doi: 10.1016/j.msec.2017.08.061
192. El Saeed AM, Abd El-Fattah M, Azzam AM, Dardir MM, Bader MM. Synthesis of cuprous oxide epoxy nanocomposite as an environmentally antimicrobial coating. Int J Biol Macromol. (2016) 89:190–7. doi: 10.1016/j.ijbiomac.2016.04.043
193. Nguyen TN, Hur J, Kim IT, Bui VKH, Lee YC. Development of antimicrobial CuO/(3-aminopropyl)Triethoxysilane activated carbon fiber. J Nanosci Nanotechnol. (2021) 21(8):4519–23. doi: 10.1166/jnn.2021.19428
194. Deokar AR, Perelshtein I, Saibene M, Perkas N, Mantecca P, Nitzan Y, et al. Antibacterial and in vivo studies of a green, one-pot preparation of copper/zinc oxide nanoparticle-coated bandages. Membranes. (2021) 11(7):462–73. doi: 10.3390/membranes11070462
195. Li M, Gao L, Schlaich C, Zhang J, Donskyi IS, Yu G, et al. Construction of functional coatings with durable and broad-spectrum antibacterial potential based on mussel-inspired dendritic polyglycerol and in situ-formed copper nanoparticles. ACS Appl Mater Interfaces. (2017) 9(40):35411–8. doi: 10.1021/acsami.7b10541
196. Rosenbaum J, Versace DL, Abbad-Andallousi S, Pires R, Azevedo C, Cénédese P, et al. Antibacterial properties of nanostructured Cu–TiO2 surfaces for dental implants. Biomater Sci. (2017) 5(3):455–62. doi: 10.1039/C6BM00868B
197. Ghosh R, Swart O, Westgate S, Miller BL, Yates MZ. Antibacterial copper-hydroxyapatite composite coatings via electrochemical synthesis. Langmuir. (2019) 35(17):5957–66. doi: 10.1021/acs.langmuir.9b00919
198. Ramazanza De B, Jahanbin A, Yaghoubi M, Shahtahmassbi N, Shafaee H. Comparison of antibacterial effects of zno and cuo nanoparticles coated brackets against streptococcus mutans. J Dent. (2015) 16(3):200–5. PMID: 26331150
199. Ahrari F, Eslami N, Rajabi O, Ghazvini K, Barati S. The antimicrobial sensitivity of streptococcus mutans and streptococcus sangius to colloidal solutions of different nanoparticles applied as mouthwashes. Dent Res J (Isfahan). (2015) 12(1):44–9. doi: 10.4103/1735-3327.150330
Keywords: copper nanoparticles, antimicrobial effect, oral pathogen, streptococcus mutans, porphyromonas gingivalis, candida albicans
Citation: Ma X, Zhou S, Xu X and Du Q (2022) Copper-containing nanoparticles: Mechanism of antimicrobial effect and application in dentistry-a narrative review. Front. Surg. 9:905892. doi: 10.3389/fsurg.2022.905892
Received: 28 March 2022; Accepted: 19 July 2022;
Published: 5 August 2022.
Edited by:
Chun Hung Chu, The University of Hong Kong, Hong Kong, ChinaReviewed by:
Mohammed Zahedul Islam Nizami, The University of Hong Kong, ChinaVeena Xu, The University of Hong Kong, China
Gabriela Sanchez-Sanhueza, Universidad de Concepción, Chile
© 2022 Ma, Zhou, Xu and Du. This is an open-access article distributed under the terms of the Creative Commons Attribution License (CC BY). The use, distribution or reproduction in other forums is permitted, provided the original author(s) and the copyright owner(s) are credited and that the original publication in this journal is cited, in accordance with accepted academic practice. No use, distribution or reproduction is permitted which does not comply with these terms.
*Correspondence: Qin Du ZHVkdWRpY25AMTYzLmNvbQ== Xiaoling Xu eGlhb2xpbmd4dUBzd2p0dS5lZHUuY24=
Specialty Section: This article was submitted to Otorhinolaryngology - Head and Neck Surgery, a section of the journal Frontiers in Surgery