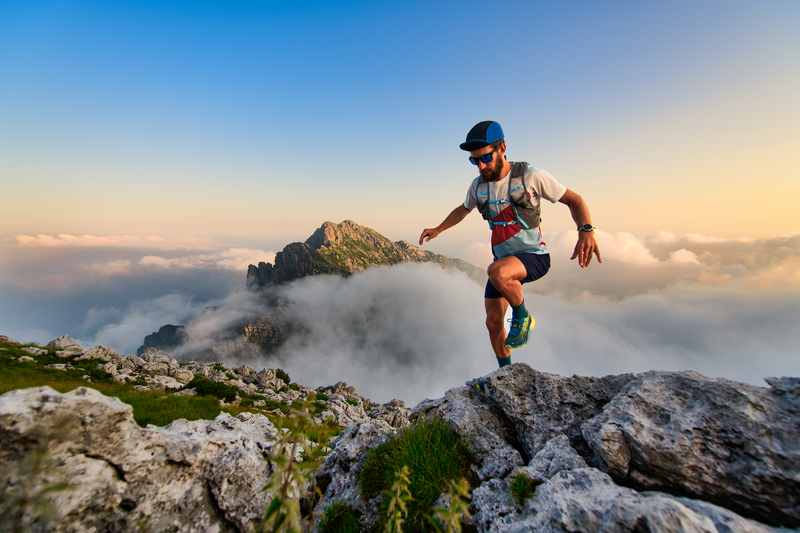
95% of researchers rate our articles as excellent or good
Learn more about the work of our research integrity team to safeguard the quality of each article we publish.
Find out more
REVIEW article
Front. Surg. , 23 September 2022
Sec. Neurosurgery
Volume 9 - 2022 | https://doi.org/10.3389/fsurg.2022.863921
This article is part of the Research Topic Insights in Neurosurgery: 2021 View all 3 articles
Advanced Parkinson's disease (PD) is characterized by increasingly debilitating impaired movements that include motor fluctuations and dyskinesias. At this stage of the disease, pharmacological management can result in unsatisfactory clinical benefits and increase the occurrence of adverse effects, leading to the consideration of advanced therapies. The scope of this review is to provide an overview of currently available therapies for advanced PD, specifically levodopa–carbidopa intestinal gel, continuous subcutaneous apomorphine infusion, radiofrequency ablation, stereotactic radiosurgery, MRI-guided focused ultrasound, and deep brain stimulation. Therapies in clinical trials are also discussed, including novel formulations of subcutaneous carbidopa/levodopa, gene-implantation therapies, and cell-based therapies. This review focuses on the clinical outcomes and adverse effects of the various therapies and also considers patient-specific characteristics that may influence treatment choice. This review can equip providers with updated information on advanced therapies in PD to better counsel patients on the available options.
Parkinson's disease (PD) is a progressive degenerative disorder of the nervous system, characterized by motor and nonmotor symptoms (1). Early in the disease course, medical management with oral medications, including dopaminergic and nondopaminergic options, is the first-line treatment. As the disease progresses, management with oral medications alone becomes increasingly more challenging and may be limited by medication-induced side effects. Advanced PD is characterized by increasingly debilitating impaired movements that include motor fluctuations and dyskinesias. At this stage of the disease, pharmacological management can be associated with unsatisfactory clinical benefits and/or produce adverse effects. Consequently, therapies including device-aided pharmacotherapies, ablative or lesioning procedures, and deep brain stimulation (DBS) are often considered. Device-aided pharmacotherapies improve symptoms through continuous dopaminergic medication delivery, while ablative procedures and DBS provide targeted ablation/stimulation of the motor circuit. This review provides an overview of currently available therapies for advanced PD that are FDA-approved, specifically levodopa–carbidopa intestinal gel (LCIG), radiofrequency (RF) ablation, stereotactic radiosurgery (SRS), MRI-guided focused ultrasound (MRgFUS), and DBS. Therapies in clinical trials or that are not FDA-approved will also be discussed including continuous subcutaneous apomorphine infusion (CSAI), novel subcutaneous carbidopa/levodopa formulations, gene-implantation therapies, and cell-based therapies. The landscape of treatment options for advanced PD is rapidly evolving, and head-to-head studies comparing the efficacy and safety of advanced therapies are lacking. In the following paragraphs, the clinical outcomes and adverse effects of the various advanced therapies will be discussed. Patient-specific characteristics can also impact treatment choice, and these factors will also be considered. This review can equip providers with updated information on advanced therapies to better counsel patients on the available treatment options.
Infusion therapies for PD are applied on the rationale that continuous increased availability of striatal dopamine (DA) can significantly reduce motor fluctuations and the variability in the treatment response (2). Numerous clinical studies demonstrate that continuous dopaminergic stimulation downregulates motor fluctuation and dyskinesia compared to intermittent dopaminergic stimulation. LCIG and CSAI are the only available infusion therapies on the market at this time (3). CSAI is currently available in Europe and is under review for use in the United States. The safety and efficacy of continuous subcutaneous levodopa are also being explored (4).
LCIG infusion, also known as carbidopa/levodopa enteral suspension and by the trademark names of Duopa™ and Duodopa™, is a suspension gel containing carbidopa–levodopa in a standard 1:4 ratio that aims to deliver the drug directly to the proximal small intestine (5). The drug is delivered via a portable pump that is connected to a percutaneous endoscopic transgastric jejunostomy (PEG-J) (6). Pharmacokinetic studies have demonstrated that LCIG results in lower variability and fluctuations in levodopa plasma concentrations compared to oral levodopa (7). Several studies have evaluated the safety and efficacy of LCIG and are described below.
In the first double-blind, placebo-controlled trial of LCIG, Olanow et al. assessed the efficacy and safety of LCIG (8). Over 12 weeks, the off time was reduced by 1.91 h and the on time without troublesome dyskinesia (TSD) was increased by 1.86 h from a baseline of 6.3 and 8.7 h, respectively. In a multicenter, prospective, open-label study by Buongiorno et al., 72 patients with PD were treated with LCIG and followed for a mean observation time of 22 months. There was a significant reduction of 3.8 h from a baseline of 6.8 h in the mean off time per day (9). In an international, prospective, open-label LCIG study, PD patients received LCIG monotherapy and experienced a 4.4 h reduction in off time from a baseline of 6.8 h, a 65.6% improvement. On time without TSD was significantly increased by 4.8 h from a baseline of 7.67 h, a 62.9% improvement (10). Patients who completed this study were eligible for enrollment in a phase III multinational study. A total of 262 patients contributed to the safety dataset, and 86 patients were included in the efficacy subset. The mean total exposure to LCIG was 4.1 years. Patients in the efficacy cohort experienced a significant decrease of nearly 4 h in off time per day from prior to initial LCIG infusion to the study endpoint. Mean daily on-time hours without TSD were increased by approximately 4 h. Unified Parkinson's Disease Rating Scale (UPDRS) II and III scores were significantly improved by 3.03 and 4.77 points, respectively, from prior to initial LCIG infusion to study baseline (11). The GLORIA registry evaluated the 24-month safety and efficacy of LCIG treatment, and 258 patients enrolled by 75 movement disorder centers were used for this registry. At the last visit, treated patients showed a 3.9 h reduction in off time from a baseline of 6.0 and 1.1 h reduction in on time with dyskinesia from a baseline of 4.3 h (12). The GREENFIELD observational study assessed the effect of LCIG therapy on motor and nonmotor symptoms. There was an improvement in mean UPDRS-IV Item 39 (daily off time) of 55% and 50% at second and third follow-up visits, respectively (13).
Despite being an efficacious alternative to oral therapies, the high rate of adverse events (AEs) associated with LCIG must be considered. In total, 55%–95% of patients experience AEs, the majority being mild to moderate (8, 10–13). The most common AEs were device- or procedure-related, including procedural pain, abdominal pain, and postoperative wound infection (14). Serious AEs (SAEs) range from 27% to 53%, and the most common SAEs include peritonitis, pneumonia, and abdominal pain (10–15). The annual rates of percutaneous endoscopic gastrostomy and jejunal tube replacements are around 16% and 30%, respectively, as reported by long-term studies (11).
LCIG is a suitable choice for patients with levodopa-responsive PD with motor fluctuations that are not adequately controlled with oral therapy. There are no age limitations associated with LCIG therapy, although this treatment is cautioned in patients with severe cognitive decline and/or severe dopaminergic psychosis. Nevertheless, this therapy has been successfully applied to some patients with dementia who are unable to receive other advanced therapies (16). Because the initiation of LCIG therapy requires a PEG-J implantation procedure, the presence, or history of, gastrectomy/previous gastroenteroanastomosis must be carefully assessed. If the patient is unable to manage the pump adequately, LCIG also requires the availability of a responsible caregiver who can be taught to operate the pump (17).
Apomorphine is a dopamine agonist with a mixed affinity for D1 and D2 receptors and an affinity for serotonergic and alpha-adrenergic receptors (2). While the motor efficacy of apomorphine is similar to that of levodopa, apomorphine cannot be administered orally due to its low oral bioavailability (18, 19). Subcutaneous infusions have a similar pharmacokinetic profile to the intravenous route. Compared with intermittent subcutaneous injections, CSAI has a longer apparent plasma half-life and simulates the physiological stimulation of striatal neurons (20–22).
In the OPTIPUMP cohort study, the efficacy and safety of CSAI were assessed. The total UPDRS score showed significant improvement in all patients at 6 months. UPDRS-III decreased in the on-medication state by 16.3% (23). In the first prospective, randomized, placebo-controlled trial to investigate the efficacy and safety of apomorphine subcutaneous infusion in PD patients, a daily off time reduction of 1.89 h from a baseline of 6.69 h was seen in treated patients, while there was an increase of 1.97 h in on time without TSD from a baseline of 8.52 h (24). In a retrospective study, 230 patients were treated with CSAI over 10 years. In this cohort, the daily off hours were reduced from 5.4 to 1.2 h (25).
Almost all patients on CSAI experienced infusion site reactions, with subcutaneous nodules being the most common form. The majority of reactions are considered mild to moderate, although there have been reports of severe cases of necrosis and abscess formation (2, 26). Emesis and hypotension can also occur, although these side effects are more common with intermittent injection than CSAI (27, 28). Rare cases of hemolytic anemia have also been reported, the mechanism of which remains unclear (29). Additionally, CSAI may fail to resolve impulse control disorders (ICDs) for PD patients with existing ICDs, or potentially induce new ICDs (30).
Patient suitability for CSAI is similar to that of LCIG in that it is an appropriate option for patients with levodopa-responsive PD with motor fluctuations not adequately controlled with oral therapy. CSAI is less invasive than LCIG in that initiation of treatment does not require a surgical implantation procedure. CSAI also requires the availability of a caregiver to aid with the infusion pump should the patient be unable to manage it on their own adequately. Given the potential risk of worsening neuropsychiatric symptoms, specifically ICDs, patients with these pre-existing conditions should be carefully monitored when placed on CSAI (30).
The efficacy and safety of novel formulations of subcutaneous carbidopa/levodopa are also explored. ND01612 (NeuroDerm) is a novel formulation of levodopa/carbidopa that is administered subcutaneously via a pump. In a 28-day open-label study, 33 PD patients were randomized to treatment with either a 24-h infusion or a 14-h waking day infusion. The overall off time was reduced by 2 h from a baseline 5.3 h, with a larger reduction in the 24-h group (2.8 compared to 1.3). Eight patients in the 24-h group experienced complete resolution of the off time. The on time without TSD was increased by 3.3 h from a baseline of 8.9 h, and the on time with TSD was reduced by 1.2 h overall from 1.9 h at baseline. The 24-h infusion group experienced a −19.1 mean change in the UPDRS-III score from baseline. The most common AEs were infusion site reactions (4).
Foslevodopa/foscarbidopa (ABBV-951) is a subcutaneous levodopa/carbidopa prodrug formulation that is in development for treating the motor complications of advanced PD. A phase 1, single-blind study demonstrated that foslevodopa/foscarbidopa infusion provides stable levodopa and carbidopa exposures compared to oral dosing. The safety and tolerability of the infusion were also assessed, and the safety profile was determined to be favorable. Five of the 28 subjects had treatment-related AEs, including infusion site pain, dizziness, dyskinesia, paresthesia, and euphoric mood. Only one adverse event of anxiety was considered severe, while all other AEs were mild or moderate in severity (31).
Ablative therapies for neurological disorders aim to destroy a targeted volume of brain tissue (32). Here, we discuss three ablative therapies used in the treatment of PD: RF ablation, SRS, and MRgFUS. Radiofrequency ablation has been largely supplanted by DBS and MRgFUS but is still performed in resource-limited areas, as the latter-mentioned therapies are technologically demanding and expensive.
Interstitial RF consists of creating a lesion through frictional heating of ionic oscillations of an intracranially placed electrode coupled to an RF generator. During the procedure, patients are awake, and the target is confirmed with a test stimulation. A lesion is created near the tip of the active electrode, which is where the greatest heating occurs. RF lesioning allows for the creation of distinct lesion borders with immediate results and intraoperative confirmation of symptom improvement (33, 34).
Ablation of the ventral intermediate (VIM) nucleus of the thalamus has been shown to significantly improve tremors in patients with tremor-dominant Parkinson's disease (TDPD). A retrospective study by Jankovic et al. demonstrated that 86% of patients with PD experienced moderate to complete tremor improvement at a mean follow-up of 53 months (35). Most AEs with RF thalamotomy are transient and due to the perilesional edema that resolves with time. However, persistent adverse effects may occur, including ataxia, gait disturbances, dysarthria, and motor/sensory deficits (36). While RF thalamotomy can be performed bilaterally, the risk of dysarthria is significantly higher with bilateral lesions, so the procedure is generally only performed unilaterally (33).
Globus pallidus internus (GPi) ablation has also been shown to improve motor symptoms in PD patients (37). A randomized controlled trial demonstrated that patients receiving unilateral pallidotomy had a 32% improvement in the UPDRS-III score at 6-month follow-up compared to those randomized to medical therapy. Patients’ experienced improvements in tremor, rigidity, bradykinesia, gait, and balance after the procedure (38). Pallidotomy and thalamotomy carry similar cognitive and motor risks, although pallidotomy also carries the risk of visual disturbance (33). Although bilateral pallidotomy can be effective, the increased incidence of adverse effects must be considered against the marginal gain of performing the second side. A bilateral procedure is generally not recommended, especially considering the availability of other techniques/procedures (39).
Ablation of the subthalamic nucleus (STN) has been explored for treating the motor symptoms of PD. A 43%–52% improvement in off-medication UPDRS-III scores has been reported in more recent trials of RF subthalamotomy. Adverse effects include contralateral dyskinesias and transient hemiballism (33).
RF ablation allows for the creation of distinct lesion borders and intraoperative physiological confirmation of lesion creation. RF ablation provides immediate treatment effects, but the effects are not adjustable or reversible. Drawbacks include less predictability in the size and shape of the lesion. While adverse effects are generally mild and transient, the surgical risk of intracerebral hemorrhage must be considered (33). While used less commonly in high-income countries, ablative therapies requiring fewer resources such as RF remain a reasonable treatment strategy in emerging countries with limited resources (40).
SRS lesioning is a noninvasive procedure that uses computerized dosimetry planning and image-guided stereotaxy to deliver a single dose of ionizing radiation to an intracranial target volume. Different devices are used to deliver radiation, including GammaKnife® and linear accelerators (34).
Gamma knife (GK) thalamotomy has been shown to improve motor function in patients with PD. The radiation dose used in GK thalamotomy ranges from 120 to 180 Gy, and it appears that doses less than 120 Gy do not provide clinical benefits (41). In a prospective study of unilateral gamma knife thalamotomy for patients with PD and essential tremor (ET), Ohye et al. reported an improvement in tremor scores (UPDRS-II and III), with 81.1% of patients evaluated as having good or excellent results at 24-month follow-up (42). A more recent prospective, single-blind study of unilateral GK thalamotomy for patients PD and ET reported a 54.2% improvement in the upper limb tremor score (43). GK pallidotomy has also been performed to treat PD, although only a few reports have described the procedure (44). The GPi is considered a high-risk target for GK radiosurgery due to its proximity to the optic tract and the risk of optic neuropathy (45). Likewise, the STN is not an advised target due to the risk of neurologic complications including hemiparesis, dysarthria, and gait disturbances (46).
A major benefit of GK radiosurgery is that it allows for precise intracranial treatment without cranial opening, eliminating the surgical risk of hemorrhage as it does not require the creation of burr holes or puncturing of brain tissue. A major drawback to GK radiosurgery for treating movement disorders is that there are often significant delays before any symptom improvement is appreciated. In previous reports, a delay of 3–6 months was noted prior to the onset of tremor suppression (42, 43). Additionally, the radiation response after GK radiosurgery can be variable. Approximately 10% of patients exhibited excess reactions, demonstrated by a high signal zone in the thalamus and surrounding area on MR images, even when the administered radiation dose was uniform (42). The delayed effect, exposure to ionizing radiation, and lack of intraoperative feedback are all disadvantages that must be considered when utilizing GK radiosurgery as a treatment for advanced PD (33). GK radiosurgery remains a good option for patients with significant medical comorbidities who cannot undergo more invasive procedures like DBS or RF (36).
Early experiments investigating the neurosurgical applications of focused ultrasound demonstrated ultrasound as an effective tool for intracranial lesioning. However, craniotomies were required to avoid trajectory damage and wave distortion by the skull, and effective monitoring techniques were lacking. The subsequent development and hemispheric distribution of phased arrays have allowed for a completely transcranial procedure (47). In this procedure, an intracranial region is targeted with high-intensity focused ultrasound beams. Ablation of the target structure is achieved via ultrasonic mechanical energy that is absorbed within the focal target volume and converted into heat. Real-time monitoring of energy deposition and accurate targeting is made possible by MRI guidance and MR thermography (33). MRgFUS allows for accurate ablation of deep brain structures while eliminating the need for a craniotomy. An additional advancement of MRgFUS over that of other ablative procedures is that the patient can be examined after each sonication. During MRgFUS, a series of verification and low treatment sonications at low temperatures are performed, resulting in transient benefits and transient or minimal adverse effects. After clinically verifying the target with lower-temperature sonications, the temperature is then increased, resulting in a permanent lesion. MRgFUS of the VIM is currently approved for the treatment of tremors. Other targets are currently under investigation (48).
In a study by Bond et al., 20 patients were randomized to focus ultrasound unilateral VIM thalamotomy versus 7 patients to the sham procedure for patients with medically refractory tremor-dominant PD. Hand tremor, as measured with the Clinical Rating Scale for Tremor (CRST) A + B subscores in the on-medication state, improved by 62% at 3 months. Total CRST scores improved by 44% at 3 months. The most common procedure-related AEs for all patients were finger and orofacial paresthesia. Two patients had persistent hemiparesis, and one patient had persistent mild ataxia (49).
A multicenter open-label trial investigated the efficacy and safety of MRgFUS ablation of the GPi for PD patients exhibiting levodopa responsiveness, motor fluctuations, and asymmetrical motor signs. There was a 44.5% improvement in the off-medication UPDRS-III score from baseline value at 3 months and a 45.2% improvement at 12 months. The UPDRS-IV score was used to assess the motor complications of PD. The baseline pretreatment value was reduced by 42% at 3 months, and this improvement was maintained at 12 months. Twenty persistent AEs were reported, including fine motor difficulties, dysarthria, and balance difficulties (50).
In a pilot study conducted by Martinez-Fernandez et al., 10 patients received MRgFUS subthalamotomy for the management of asymmetric PD. In the treated hemibody, the mean UPDRS-III score improved by 53% and 47% in the off-medication and on-medication states, respectively. Persistent AE included upper limb dyskinesia in two patients (51). A randomized controlled trial was then conducted by the same group in 2020. Twenty-seven patients underwent MRgFUS subthalamotomy for asymmetric PD. The mean UPDRS-III score decreased by 52.6% in the off-medication state and by 46.5% in the on-medication state at 4 months in the active-treatment group. Several AEs in the active-treatment group were reported, including dyskinesia in the on-medication state in 6 patients, dyskinesia in the off-medication in 6 patients, weakness in 5 patients, facial weakness in 3 patients, speech disturbance in 15 patients, and gait disturbance in 13 patients. Some of these deficits persisted at 12 months in six patients (52). Consequently, the high rate of AE raises concerns for MRgFUS subthalamotomy despite the clinical benefits.
MRgFUS thermal ablation may be a reasonable treatment option for patients with advanced PD who are not good candidates for more invasive techniques like DBS. As with all ablative therapies, the permanent and nonadjustable nature of MRgFUS ablation must be considered. However, during treatment, patients are clinically assessed for symptomatic improvement and adverse effects, and treatment can be adjusted as necessary. The acoustic properties of the patient's skull impact the efficiency of focusing acoustic energy on a target. These properties vary from patient to patient, and it is critical to understand the relevant ultrasound physics and limits of the technique to avoid any potential adverse effects. In general, it is recommended that patients have a skull density ratio of >0.4 to be considered candidates for this treatment. Patients with artifacts from previous cranial surgical interventions or skull shape deformations may not be suitable candidates for MRgFUS ablation (53). Other considerations are that the patient's head must be shaved, and the MRI environment and increased operative time may be uncomfortable or intolerable for claustrophobic patients. Similarly, the presence of MRI-incompatible implants must be considered (33).
Laser-induced thermal therapy (LITT) is an FDA-approved therapy for ablating intracranial soft tissue and brain structures including brain tumors, radiation necrosis, and epileptic foci. A laser diffusing fiber within a cooling catheter is stereotactically placed into the tissue of interest, and the laser fiber provides high-energy laser light circumferentially. The use of LITT with MRI provides real-time thermal imaging, allowing control of the ablation. MRI thermal imaging and LITT software provide real-time temperature feedback and controls, resulting in precise tissue ablation. A real-time necrotic zone and transitional zone are provided in real time. The surgeon can contour the ablation via either advancing or pulling back the fiber or by using multiple catheters/fibers. This therapy has been used extensively in epilepsy and brain tumors. So far, only a few case reports have been reported describing its unilateral use for pallidotomy or thalamotomy for PD (54).
DBS is an FDA-approved neurosurgical procedure that delivers electrical stimulation through an electrode placed into deeper brain structures that is connected to an implanted battery source located subclavicular. Over the past two decades, DBS has become a standard of care for patients with pharmacological resistant tremors, motor fluctuations, and bothersome dyskinesias (55). The primary surgical targets are the STN and GPi. The VIM is less commonly targeted as VIM DBS is only effective for tremors and does not improve other symptoms of PD. The STN and GPi are more easily targeted with DBS than ablative procedures, which provides an added advantage to DBS.
DBS can be performed under imaging guidance alone, with microelectrode recordings, or in a combination. In addition, the patient may be alert in the setting of performing microelectrode recordings or sedated, as typically performed with imaging-alone-based surgeries or a combination. Lower hemorrhage and infection rates may be evident with general anesthesia, but higher rates of treatment-induced side effects may occur than microelectrode recording procedures. However, there may be no difference in clinical motor outcomes (56). Overall, the surgical methodology performed depends upon the experience of the center with their preferred technique.
Randomized control studies of DBS to best medical treatment have demonstrated superior improvements with DBS. At 6-month postoperatively, motor symptoms in the on-medication/on-stimulation state and the quality of life improved by 20% compared with baseline, while the best medication group showed no clinical improvement (57). Furthermore, long-term reports of bilateral STN-DBS indicated that patients remain considerably improved compared with baseline (58). In a 13-year follow-up study, bilateral STN-DBS significantly improved motor symptoms in patients with early-onset PD. In the off-medication/on-stimulation state, patients had a 54% reduction in the total UPDRS-III score compared to that in the off-medication/off-stimulation state. Similarly, patients had a 48% reduction in the total UPDRS-III score in the on-medication/on-stimulation state compared to that in the on-medication/off-stimulation state. No hardware or surgical-related AEs were reported in the group of patients. Stimulation-induced dyskinesia occurred in four patients during the first 3 months of stimulation (59).
Another study retrospectively evaluated the clinical outcomes of bilateral GPi-DBS in 65 patients with PD. A 29% reduction of the total UPDRS-III score was appreciated in the off-medication state at 1-year follow-up, while no significant change was appreciated in the on-medication state. The most common AEs included gait and postural disorders, lead fractures, and dysarthria (60).
In a randomized controlled trial comparing DBS of the GPi and STN in patients with advanced PD, it was found that STN-DBS provides greater improvement in off-drug phase motor symptoms compared to GPi-DBS. Intention-to-treat analysis showed a greater reduction in the off-drug phase UPDRS-III score after 3 years in the STN group (32% reduction) compared to that in the GPi group (23% reduction) (61).
Adverse events in DBS can be separated into three categories: related to surgery, hardware, and stimulation. Adverse events related to surgery include but are not limited to infection and hemorrhage. The rate of DBS infections is generally less <7% and may necessitate complete removal of the DBS system. The use of intraoperative vancomycin powder has not been shown to decrease infection risk (62–66). The overall risk of intracranial hemorrhage is estimated to be <5%, and 1%–2% of intraventricular or intraparenchymal hemorrhage is symptomatic. Risk factors for intracranial hemorrhage include trans-sulcal trajectories and hypertension. Hardware-related adverse events occur in <2.2% of cases and include lead fracture, lead migration, and electrode malfunction (67). Stimulation side effects occur due to the electrical spread to unwanted regions that may be mediated with parameter adjustments, including focusing the volume of tissue activated with directional contacts (67). Directional leads also allow for increasing the therapeutic window, the onset of clinical benefits to that of stimulation-induced adverse effects, while resulting in less battery consumption (68).
The nonlesional and adjustable nature of DBS is among the advantages offered over other methods for neuromodulation (55). While many advantages exist, several patient-specific and ethical considerations must also be examined. DBS is a minimally invasive surgery with low incidences of infection and symptomatic hemorrhage. Additionally, DBS incurs large capital costs and requires a multidisciplinary expert team to provide optimal programming and troubleshooting for the patient. DBS can also be problematic for some in that it commits patients to a lifelong implant requiring subsequent battery (IPG) changes (55). However, due to advancing technology, battery life continues to increase and patients have the option of a rechargeable battery, thus decreasing the number of battery replacement surgeries. Patients who travel far to receive DBS surgery also now have the option to have DBS programmed remotely (69, 70). Ideal PD candidates for DBS are those with motor fluctuations and/or TSD without having other “red flags” for an alternative diagnosis. Patients with neuropsychiatric issues and multiple comorbidities may be poor candidates. Many centers exclude patients older than 70 years from DBS surgeries, although an age limit for DBS has not been defined (34).
Most clinicians favor DBS over lesioning procedures wherever the former is widely available. However, lesioning procedures are still performed in less developed countries due to financial constraints, lack of infrastructure and training, and limited follow-up care for DBS (40). Lesioning procedures are relatively cheaper and reduce postoperative care and hardware-related complications compared to DBS (71). However, lesioning procedures are not reversible, and postprocedure optimization is impossible without revision surgery. The surgical reversibility of DBS has recently been contested in the literature (72). Postmortem studies evaluating patients who have undergone DBS demonstrate pathological changes including glial scarring. It is unclear as to whether the contribution to these findings is solely due to lead insertion, chronic stimulation, or a combination (73, 74). For our review, we will consider DBS as a reversible procedure, given that the aforementioned irreversible features of DBS are not comparable to the significant irreversible changes that occur with brain lesioning procedures. Moreover, there is a lack of long-term data to support whether or not these irreversible features of DBS are truly permanent (72). The increased rate of side effects with bilateral lesions is a major limitation of lesioning procedures. These side effects include aphasia, dysarthria, dysphagia, and cognitive deficits (34). The question of morbidity with bilateral lesions has been revisited in the focused ultrasound era but has historically been problematic when treating PD patients (33, 75).
Gene supplementation therapy for PD consists of using a viral vector to deliver complementary DNA (cDNA) sequences that code for genes involved in the pathogenesis of PD. Gene therapies aim to either increase the bioavailability of DA in the nigrostriatal pathway via direct enhancement of enzymes involved in dopamine production or promote the health of dopaminergic neurons through maintenance and restoration of neurotrophic factors (76). The putamen is the brain region most affected by dopaminergic denervation in PD. Gene therapies aim to restore DA in the putamen via targeted delivery. Lack of success in earlier gene therapy clinical trials was commonly due to conservative volumes, resulting in suboptimal coverage of the putamen (77–81). Since then, the use of a pressurized infusion method in the latest clinical trials has improved the distribution of the infusate within the target (82–84).
Many gene therapy studies have focused on increasing DA synthesis enzyme expression, namely, tyrosine hydroxylase (TH), L-amino acid decarboxylase (AADC), and GTP cyclohydroxylase (GCH1).
One study assessed the safety and efficacy of adeno-associated serotype 2 viral vector encoding human AADC (AAV-hAADC-2) intraputaminal infusion in advanced PD patients. At 6 months, patients demonstrated a significant improvement in both UPDRS total and UPDRS-III scores (85). In another study, advanced PD patients received low- or high-dose intraputaminal infusion of adeno-associated viral vector (AAV-hAADC). At 6 months, patients exhibited mean improvements of 36% and 28% in the off- and on-state UPDRS-III scores, respectively (86).
AADC gene therapy for PD has a favorable safety and tolerability profile. Five out of the 31 patients who received the drug across all studies reported procedure-related SAEs including four intracranial hemorrhages and one deep vein thrombosis (78, 85–88). Eight participants reported transient dyskinesia increase (87, 88).
ProSavin is a novel lentiviral vector that encodes GCH1, TH, and AADC. The phase ½ open-label, dose-escalation study has been completed, and a long-term safety and efficacy study evaluating the same patient cohort is ongoing (81, 89). OXB-102 (AXO-Lenti-PD) is an optimized version of ProSavin that expresses the same genes. A phase ½ safety and dose-escalation study is currently ongoing (90).
Patients treated with ProSavin experienced a significant reduction in the off-state UPDRS-III score, which was maintained at a 2-year follow-up (81, 89). A 21-point reduction in the UPDRS-III off-state score and a 2.2-h increase in the on time from baseline were reported in the first four patients treated with high-dose OXB-102 (90).
In total, 91% of drug-related AEs in patients treated with ProSavin were mild and/or occurred within 1-year follow-up. Acute psychosis, dyskinesia, and an unspecified nervous system disorder were among the three therapy-associated SAEs (89). No therapy-related SAEs were reported after OXB-102 treatment at 6-month follow-up (90).
Loss of dopaminergic neurons in the substantia nigra results in decreased GABA input to the STN, resulting in the loss of inhibition. Infusion of muscimol (GABA) agonist into the STN has been found to improve parkinsonian symptoms temporarily. Glutamic acid decarboxylase (GAD) is the rate-limiting enzyme for GABA production, and gene therapy consisting of insertion of the GAD gene into an adeno-associated viral vector (AAV2) has been used in animal and human studies with success. The hypothesis is that delivering GAD to the STN restores GABA transmission within the STN and normalizes output from the STN. A phase I trial of 12 patients receiving a unilateral infusion of AAV2-GAD into the STN demonstrated significant improvements in motor UPDRS scores at 3 and 12 months and confirmed the safety of the therapy (91). A phase II double-blinded trial, randomizing 23 patients to sham surgery and 22 patients to bilateral STN infusion of AAV2-GAD, showed a significant improvement in motor UPDRS scores at 6 and 12 months with an associated decrease in daily dyskinesias (92, 93).
PD patients demonstrate loss of endogenous neuronal growth factors in addition to dopaminergic neuron loss (76). Extensive research has been done on restoring growth factors to protect and improve the health of dopaminergic neurons. Glial cell-line derived neurotrophic factor (GDNF) and neurturin (NRTN) are two growth factors that have gained significant attention (94). Currently, only gene therapy with AAV2-GDNF is under active investigation in two clinical trials (76).
Results from the phase 1 study reported 36% and 54% increases in 18F-DOPA putaminal signal at 6- and 18-month follow-up, respectively. UPDRS scores remained stable over the course of the study, although motor symptoms in the patient cohort did not significantly worsen, as would be expected (76). AAV2-GDNF was shown to be safe and well tolerated. Scalp wound dehiscence in one patient was the only procedure-related SAE (79).
With genetic testing becoming more widely available, it is feasible to consider selecting patients for certain therapies based on their genotype. Patients with the advanced parkinsonian phenotype with motor fluctuations are expected to experience the greatest benefit from a gene therapy approach that aims to increase dopamine production. The unmodifiable nature of gene therapy must also be considered, particularly in patients with significantly advanced PD who are more susceptible to complications such as ICD and dopamine dysregulation syndrome. Studies investigating neurotrophic factors have emphasized the importance of targeting patients with earlier stages of PD when protecting and improving the health of dopaminergic neurons would be most beneficial (79, 80, 95).
Researchers are also investigating stem cell replacement as a novel way to treat diseased neuronal tissue (96). It is believed that DA cell transplantation is among the most promising cell-based therapy for PD, as replacing DA neurons into DA-depleted striatum can restore function in patients with PD (97).
Fetal ventral mesencephalon (VM) cell transplantation for the treatment of PD has been studied (97). One study reported the long-term clinical outcomes of two PD patients who received intrastriatal grafts of human fetal VM tissue. Following transplantation, one patient experienced significant motor benefits (38% improvement in the UPDRS-III score) and was able to stop levodopa treatment. This clinical benefit was sustained at 18 years post-transplantation. The other patient showed no improvement in the UPDRS-III score during the first 2 years after transplant but experienced motor function improvement 4 years postprocedure and was able to discontinue all dopaminergic medication. These motor benefits were preserved 15 years following transplantation. Graft-induced dyskinesias occurred in both patients. However, this was outweighed by the achieved clinical benefit on motor function and did not have a significant impact on overall function (98).
Mesenchymal stem cells (MSCs) have the capacity to differentiate toward dopaminergic neurons and release neurotrophic factors and have been shown to provide therapeutic benefits in animal models of PD (99). Clinical trials investigating MSC transplantation for the treatment of PD are currently underway (97).
Pluripotent stem cells (PSCs), including embryonic stem cells (ESCs) and induced pluripotent stem cells (iPSCs), can be differentiated into neurons with DA properties and are a promising source of cells for regenerative medicine. Human-induced PSCs have been shown to survive and function as midbrain dopaminergic neurons in a primate model of PD (100). Clinical trials investigating ESC and iPSC-derived cell products as therapeutic agents for PD have been started in Australia, China, and Japan (97).
Ongoing and future studies of advanced therapies in PD continue to expand. In ablative therapies, the potential to perform these treatments bilaterally and safely target structures with the potential of improving a greater number of parkinsonian symptoms is currently being explored. There is a large amount of ongoing research on DBS. Many research teams, including those in collaboration with DBS manufacturers, are exploring the potential for a “closed-loop” system. Biofeedback from local field potentials (the summation of synchronized cellular electrical activity) recorded from the contacts of the DBS electrode and wearable sensors may provide input to an adaptive DBS program that modifies parameters and the location of stimulation. Such systems may be better able to treat symptoms currently challenging to manage, including freezing of gait. The current location of the IPG is subclavicular connected to the DBS lead by a cable tunneled under the scalp and skin of the neck. Potential complications associated with this current hardware include skin erosion, infection, discomfort with neck and head movements, and bowstringing (migration of the IPG distal creating stretch on the connection cable). An IPG that is skull-mounted or that is the cap of the burr hole anchoring the DBS lead would reduce these potential complications and eliminate the need for a second surgery to place the IPG. Photobiomodulation, the use of infrared light delivered from a laser, may have disease-modifying effects and is also being investigated. If there are clear data demonstrating efficacy in PD human trials, it may be possible to develop technology combining photobiomodulation with DBS. Finally, future studies will hopefully progress gene therapies that either deliver neuroprotective agents or improve the function of cells or halt the neurodegenerative process. This technology will likely be based on an improved understanding of the pathophysiology of PD and potentially could become patient-specific based on the fundamental cause, including genetic for dopaminergic cell loss.
The initiation of advanced therapies for PD is often considered when oral medications fail to control a patient's motor symptoms adequately. The landscape for advanced therapies for PD is rapidly evolving, and head-to-head studies comparing the efficacy and safety of treatment options are lacking. In advanced therapy selection, patient-specific considerations should be weighed along with the safety and efficacy of each treatment modality. LCIG significantly improves daily off time and on time without TSD, ranging from 1.91 to 4.4 h and 1.86 to 4.8 h, respectively (8–11). Results from key studies investigating the efficacy of LCIG are summarized in Table 1. CSAI provides daily off time reduction ranging from 1.89 to 4.2 h and an increase in daily on time without TSD of 1.97 h (24, 25). Infusion therapies are an appropriate choice for patients with levodopa-responsive PD with motor fluctuations. Patients must also be able to independently manage the pump or have a responsible caregiver who can do so (17). Initiation of LCIG or CSAI avoids an intracranial procedure. Abdominal and gastrointestinal ailments need to be considered in evaluating the placement of a PEG tube for LCIG (17). CSAI and potential future subcutaneous treatments are the least invasive, but risks of skin irritation, nodules, and infections are also a consideration (2, 26). Ablative therapies including SRS and MRgFUS allow for precise intracranial treatment without cranial opening and may be reasonable options for patients with significant comorbidities who are not good candidates for other invasive techniques. Studies evaluating the efficacy of GK thalamotomy report a 54.2% improvement in the upper limb tremor score, with up to 81.1% of patients evaluated as having good or excellent results at 24-month follow-up (42, 43). MRgFUS has been shown to provide significant improvements in motor outcomes. The results from key studies evaluating the efficacy of MRgFUS are reported in Table 2. However, drawbacks of SRS and MRgFUS must also be considered. For SRS, patients are exposed to high doses of ionizing radiation, and there are often significant delays before symptomatic improvement (42, 43). For MRgFUS, the patient's tolerability of the MRI environment and the presence of MRI-incompatible implants must be considered (33). Additionally, the acoustic properties of the patient's skull must be understood (53). For all ablative procedures, the nonreversible and nonadjustable nature of the technique must also be considered. RF ablation has largely been supplanted by DBS but remains a reasonable option in lower-resource areas (40). DBS offers the ability to interface with circuit pathology directly and is unique in that it is a nonlesional and adjustable treatment option (55). In the off-medication/on-stimulation state compared to the off-medication/off-stimulation state, bilateral STN-DBS provides reductions in the total UPDRS-III score ranging from 32% to 54% (59, 61). Similarly, bilateral GPi-DBS provides a 23%–29% reduction in the total UPDRS-III score in the off-medication state (60, 61). However, DBS poses the risk of hemorrhage and infection as it is an intracranial procedure. Additionally, DBS incurs large capital costs and commits the patient to a lifelong implant requiring subsequent battery changes (55). The advantages and drawbacks of each advanced therapy are summarized in Table 3. This review intends to help physicians understand retrospective data and patient considerations for each advanced therapy option.
All authors have made a substantial contribution to the conception of the article, and contributed to drafting and revising the article. All authors contributed to the article and approved the submitted version.
The authors declare that the research was conducted in the absence of any commercial or financial relationships that could be construed as a potential conflict of interest.
All claims expressed in this article are solely those of the authors and do not necessarily represent those of their affiliated organizations, or those of the publisher, the editors and the reviewers. Any product that may be evaluated in this article, or claim that may be made by its manufacturer, is not guaranteed or endorsed by the publisher.
1. DeMaagd G, Philip A. Parkinson's disease and its management: part 1: disease entity, risk factors, pathophysiology, clinical presentation, and diagnosis. P T. (2015) 40(8):504–32.26236139
2. Prakash N, Simuni T. Infusion therapies for Parkinson's disease. Curr Neurol Neurosci Rep. (2020) 20(9):44. doi: 10.1007/s11910-020-01062-2
3. Xie CL, Wang WW, Zhang SF, Gan J, Liu ZG. Continuous dopaminergic stimulation (CDS)-based treatment in Parkinson's disease patients with motor complications: a systematic review and meta-analysis. Sci Rep. (2014) 4:6027. doi: 10.1038/srep06027
4. Olanow CW, Espay AJ, Stocchi F, Ellenbogen AL, Leinonen M, Adar L, et al. Continuous subcutaneous levodopa delivery for Parkinson's disease: a randomized study. J Parkinsons Dis. (2021) 11(1):177–86. doi: 10.3233/JPD-202285
5. Camargo SM, Vuille-dit-Bille RN, Mariotta L, Ramadan T, Huggel K, Singer D, et al. The molecular mechanism of intestinal levodopa absorption and its possible implications for the treatment of Parkinson's disease. J Pharmacol Exp Ther. (2014) 351(1):114–23. doi: 10.1124/jpet.114.216317
6. Zhang XR, Jiang ZY, Zhang ZR, Chen HJ, Wu K, He JC, et al. The advantages of levodopa-carbidopa intestinal gel for patients with advanced Parkinson's disease: a systematic review. Drug Des Devel Ther. (2020) 14:845–54. doi: 10.2147/DDDT.S229621
7. Othman AA, Rosebraugh M, Chatamra K, Locke C, Dutta S. Levodopa-carbidopa intestinal gel pharmacokinetics: lower variability than oral levodopa-carbidopa. J Parkinsons Dis. (2017) 7(2):275–8. doi: 10.3233/JPD-161042
8. Olanow CW, Kieburtz K, Odin P, Espay AJ, Standaert DG, Fernandez HH, et al. Continuous intrajejunal infusion of levodopa-carbidopa intestinal gel for patients with advanced Parkinson's disease: a randomised, controlled, double-blind, double-dummy study. Lancet Neurol. (2014) 13(2):141–9. doi: 10.1016/S1474-4422(13)70293-X
9. Buongiorno M, Antonelli F, Camara A, Puente V, de Fabregues-Nebot O, Hernandez-Vara J, et al. Long-term response to continuous duodenal infusion of levodopa/carbidopa gel in patients with advanced Parkinson disease: the Barcelona registry. Parkinsonism Relat Disord. (2015) 21(8):871–6. doi: 10.1016/j.parkreldis.2015.05.014
10. Fernandez HH, Standaert DG, Hauser RA, Lang AE, Fung VS, Klostermann F, et al. Levodopa-carbidopa intestinal gel in advanced Parkinson's disease: final 12-month, open-label results. Mov Disord. (2015) 30(4):500–9. doi: 10.1002/mds.26123
11. Fernandez HH, Boyd JT, Fung VSC, Lew MF, Rodriguez RL, Slevin JT, et al. Long-term safety and efficacy of levodopa-carbidopa intestinal gel in advanced Parkinson's disease. Mov Disord. (2018) 33(6):928–36. doi: 10.1002/mds.27338
12. Antonini A, Poewe W, Chaudhuri KR, Jech R, Pickut B, Pirtosek Z, et al. Levodopa-carbidopa intestinal gel in advanced Parkinson's: final results of the GLORIA registry. Parkinsonism Relat Disord. (2017) 45:13–20. doi: 10.1016/j.parkreldis.2017.09.018
13. Lopiano L, Modugno N, Marano P, Sensi M, Meco G, Solla P, et al. Motor and non-motor outcomes in patients with advanced Parkinson's disease treated with levodopa/carbidopa intestinal gel: final results of the GREENFIELD observational study. J Neurol. (2019) 266(9):2164–76. doi: 10.1007/s00415-019-09337-6
14. Lang AE, Rodriguez RL, Boyd JT, Chouinard S, Zadikoff C, Espay AJ, et al. Integrated safety of levodopa-carbidopa intestinal gel from prospective clinical trials. Mov Disord. (2016) 31(4):538–46. doi: 10.1002/mds.26485
15. Epstein M, Johnson DA, Hawes R, Schmulewitz N, Vanagunas AD, Gossen ER, et al. Long-Term PEG-J tube safety in patients with advanced Parkinson's disease. Clin Transl Gastroenterol. (2016) 7:e159. doi: 10.1038/ctg.2016.19
16. Sanchez-Castaneda C, Campdelacreu J, Miro J, Juncadella M, Jauma S, Calopa M. Cognitive improvement after duodenal levodopa infusion in cognitively impaired Parkinson's disease patients. Prog Neuropsychopharmacol Biol Psychiatry. (2010) 34(1):250–1. doi: 10.1016/j.pnpbp.2009.10.021
17. Abbruzzese G, Barone P, Bonuccelli U, Lopiano L, Antonini A. Continuous intestinal infusion of levodopa/carbidopa in advanced Parkinson's disease: efficacy, safety and patient selection. Funct Neurol. (2012) 27(3):147–54.23402675
18. Kempster PA, Frankel JP, Stern GM, Lees AJ. Comparison of motor response to apomorphine and levodopa in Parkinson's disease. J Neurol Neurosurg Psychiatry. (1990) 53(11):1004–7. doi: 10.1136/jnnp.53.11.1004
19. Merello M, Pikielny R, Cammarota A, Leiguarda R. Comparison of subcutaneous apomorphine versus dispersible madopar latency and effect duration in Parkinson's disease patients: a double-blind single-dose study. Clin Neuropharmacol. (1997) 20(2):165–7. doi: 10.1097/00002826-199704000-00008
20. Gancher ST, Nutt JG, Woodward WR. Absorption of apomorphine by various routes in parkinsonism. Mov Disord. (1991) 6(3):212–6. doi: 10.1002/mds.870060304
21. Manson AJ, Hanagasi H, Turner K, Patsalos PN, Carey P, Ratnaraj N, et al. Intravenous apomorphine therapy in Parkinson's disease: clinical and pharmacokinetic observations. Brain. (2001) 124(Pt 2):331–40. doi: 10.1093/brain/124.2.331
22. Nicolle E, Pollak P, Serre-Debeauvais F, Richard P, Gervason CL, Broussolle E, et al. Pharmacokinetics of apomorphine in parkinsonian patients. Fundam Clin Pharmacol. (1993) 7(5):245–52. doi: 10.1111/j.1472-8206.1993.tb00238.x
23. Drapier S, Eusebio A, Degos B, Verin M, Durif F, Azulay JP, et al. Quality of life in Parkinson's disease improved by apomorphine pump: the OPTIPUMP cohort study. J Neurol. (2016) 263(6):1111–9. doi: 10.1007/s00415-016-8106-3
24. Katzenschlager R, Poewe W, Rascol O, Trenkwalder C, Deuschl G, Chaudhuri KR, et al. Apomorphine subcutaneous infusion in patients with Parkinson's disease with persistent motor fluctuations (TOLEDO): a multicentre, double-blind, randomised, placebo-controlled trial. Lancet Neurol. (2018) 17(9):749–59. doi: 10.1016/S1474-4422(18)30239-4
25. Sesar A, Fernandez-Pajarin G, Ares B, Rivas MT, Castro A. Continuous subcutaneous apomorphine infusion in advanced Parkinson's disease: 10-year experience with 230 patients. J Neurol. (2017) 264(5):946–54. doi: 10.1007/s00415-017-8477-0
26. Skorvanek M, Bhatia KP. The skin and Parkinson's disease: review of clinical, diagnostic, and therapeutic issues. Mov Disord Clin Pract. (2017) 4(1):21–31. doi: 10.1002/mdc3.12425
27. Pietz K, Hagell P, Odin P. Subcutaneous apomorphine in late stage Parkinson's disease: a long term follow up. J Neurol Neurosurg Psychiatry. (1998) 65(5):709–16. doi: 10.1136/jnnp.65.5.709
28. Stocchi F, Vacca L, De Pandis MF, Barbato L, Valente M, Ruggieri S. Subcutaneous continuous apomorphine infusion in fluctuating patients with Parkinson's disease: long-term results. Neurol Sci. (2001) 22(1):93–4. doi: 10.1007/s100720170062
29. Tyne HL, Parsons J, Sinnott A, Fox SH, Fletcher NA, Steiger MJ. A 10 year retrospective audit of long-term apomorphine use in Parkinson's disease. J Neurol. (2004) 251(11):1370–4. doi: 10.1007/s00415-004-0547-4
30. Samuel M, Rodriguez-Oroz M, Antonini A, Brotchie JM, Ray Chaudhuri K, Brown RG, et al. Management of impulse control disorders in Parkinson's disease: controversies and future approaches. Mov Disord. (2015) 30(2):150–9. doi: 10.1002/mds.26099
31. Rosebraugh M, Liu W, Neenan M, Facheris MF. Foslevodopa/foscarbidopa is well tolerated and maintains stable levodopa and carbidopa exposure following subcutaneous infusion. J Parkinsons Dis. (2021) 11(4):1695–702. doi: 10.3233/JPD-212813
32. Haar GT, Coussios C. High intensity focused ultrasound: physical principles and devices. Int J Hyperthermia. (2007) 23(2):89–104. doi: 10.1080/02656730601186138
33. Franzini A, Moosa S, Servello D, Small I, DiMeco F, Xu Z, et al. Ablative brain surgery: an overview. Int J Hyperthermia. (2019) 36(2):64–80. doi: 10.1080/02656736.2019.1616833
34. Sharma VD, Patel M, Miocinovic S. Surgical treatment of Parkinson's disease: devices and lesion approaches. Neurotherapeutics. (2020) 17(4):1525–38. doi: 10.1007/s13311-020-00939-x
35. Jankovic J, Cardoso F, Grossman RG, Hamilton WJ. Outcome after stereotactic thalamotomy for parkinsonian, essential, and other types of tremor. Neurosurgery. (1995) 37(4):680–6, discussion 686–7. doi: 10.1227/00006123-199510000-00011
36. Dallapiazza RF, Lee DJ, De Vloo P, Fomenko A, Hamani C, Hodaie M, et al. Outcomes from stereotactic surgery for essential tremor. J Neurol Neurosurg Psychiatry. (2019) 90(4):474–82. doi: 10.1136/jnnp-2018-318240
37. Alkhani A, Lozano AM. Pallidotomy for Parkinson disease: a review of contemporary literature. J Neurosurg. (2001) 94(1):43–9. doi: 10.3171/jns.2001.94.1.0043
38. Vitek JL, Bakay RA, Freeman A, Evatt M, Green J, McDonald W, et al. Randomized trial of pallidotomy versus medical therapy for Parkinson's disease. Ann Neurol. (2003) 53(5):558–69. doi: 10.1002/ana.10517
39. Gross RE. What happened to posteroventral pallidotomy for Parkinson's disease and dystonia? Neurotherapeutics. (2008) 5(2):281–93. doi: 10.1016/j.nurt.2008.02.001
40. Jourdain VA, Schechtmann G. Health economics and surgical treatment for Parkinson's disease in a world perspective: results from an international survey. Stereotact Funct Neurosurg. (2014) 92(2):71–9. doi: 10.1159/000355215
41. Higuchi Y, Matsuda S, Serizawa T. Gamma knife radiosurgery in movement disorders: indications and limitations. Mov Disord. (2017) 32(1):28–35. doi: 10.1002/mds.26625
42. Ohye C, Higuchi Y, Shibazaki T, Hashimoto T, Koyama T, Hirai T, et al. Gamma knife thalamotomy for Parkinson disease and essential tremor: a prospective multicenter study. Neurosurgery. (2012) 70(3):526–35, discussion 535–6. doi: 10.1227/NEU.0b013e3182350893
43. Witjas T, Carron R, Krack P, Eusebio A, Vaugoyeau M, Hariz M, et al. A prospective single-blind study of gamma knife thalamotomy for tremor. Neurology. (2015) 85(18):1562–8. doi: 10.1212/WNL.0000000000002087
44. Young RF, Vermulen S, Posewitz A. Gamma knife radiosurgery for the treatment of trigeminal neuralgia. Stereotact Funct Neurosurg. (1998) 70(Suppl 1):192–9. doi: 10.1159/000056422
45. Higuchi Y, Iacono RP. Surgical complications in patients with Parkinson's disease after posteroventral pallidotomy. Neurosurgery. (2003) 52(3):558–71, discussion 568–71. doi: 10.1227/01.NEU.0000047817.60776.5C
46. Drummond PS, Pourfar MH, Hill TC, Mogilner AY, Kondziolka DS. Subthalamic gamma knife radiosurgery in Parkinson's disease: a cautionary tale. Stereotact Funct Neurosurg. (2020) 98(2):110–7. doi: 10.1159/000505709
47. Harary M, Segar DJ, Huang KT, Tafel IJ, Valdes PA, Cosgrove GR. Focused ultrasound in neurosurgery: a historical perspective. Neurosurg Focus. (2018) 44(2):E2. doi: 10.3171/2017.11.FOCUS17586
48. Martinez-Fernandez R, Pineda-Pardo JA. Magnetic resonance-guided focused ultrasound for movement disorders: clinical and neuroimaging advances. Curr Opin Neurol. (2020) 33(4):488–97. doi: 10.1097/WCO.0000000000000840
49. Bond AE, Shah BB, Huss DS, Dallapiazza RF, Warren A, Harrison MB, et al. Safety and efficacy of focused ultrasound thalamotomy for patients with medication-refractory, tremor-dominant Parkinson disease: a randomized clinical trial. JAMA Neurol. (2017) 74(12):1412–8. doi: 10.1001/jamaneurol.2017.3098
50. Eisenberg HM, Krishna V, Elias WJ, Cosgrove GR, Gandhi D, Aldrich CE, et al. MR-guided focused ultrasound pallidotomy for Parkinson's disease: safety and feasibility. J Neurosurg. (2020) 135(3):1–7. doi: 10.3171/2020.6.JNS192773
51. Martinez-Fernandez R, Rodriguez-Rojas R, Del Alamo M, Hernandez-Fernandez F, Pineda-Pardo JA, Dileone M, et al. Focused ultrasound subthalamotomy in patients with asymmetric Parkinson's disease: a pilot study. Lancet Neurol. (2018) 17(1):54–63. doi: 10.1016/S1474-4422(17)30403-9
52. Martinez-Fernandez R, Manez-Miro JU, Rodriguez-Rojas R, Del Alamo M, Shah BB, Hernandez-Fernandez F, et al. Randomized trial of focused ultrasound subthalamotomy for Parkinson's disease. N Engl J Med. (2020) 383(26):2501–13. doi: 10.1056/NEJMoa2016311
53. Stieglitz LH, Oertel MF, Accolla EA, Bally J, Bauer R, Baumann CR, et al. Consensus statement on high-intensity focused ultrasound for functional neurosurgery in Switzerland. Front Neurol. (2021) 12:722762. doi: 10.3389/fneur.2021.722762
54. Luciano M S, Katz M, Ostrem J, Martin A, Starr P, Ziman N, et al. Effective interventional magnetic resonance image-guided laser ablations in a Parkinson's disease patient with refractory tremor. Mov Disord Clin Pract. (2016) 3(3):312–4. doi: 10.1002/mdc3.12283
55. Lozano AM, Lipsman N, Bergman H, Brown P, Chabardes S, Chang JW, et al. Deep brain stimulation: current challenges and future directions. Nat Rev Neurol. (2019) 15(3):148–60. doi: 10.1038/s41582-018-0128-2
56. Ho AL, Ali R, Connolly ID, Henderson JM, Dhall R, Stein SC, et al. Awake versus asleep deep brain stimulation for Parkinson's disease: a critical comparison and meta-analysis. J Neurol Neurosurg Psychiatry. (2018) 89(7):687–91. doi: 10.1136/jnnp-2016-314500
57. Deuschl G, Schade-Brittinger C, Krack P, Volkmann J, Schafer H, Botzel K, et al. A randomized trial of deep-brain stimulation for Parkinson's disease. N Engl J Med. (2006) 355(9):896–908. doi: 10.1056/NEJMoa060281
58. Krack P, Batir A, Van Blercom N, Chabardes S, Fraix V, Ardouin C, et al. Five-year follow-up of bilateral stimulation of the subthalamic nucleus in advanced Parkinson's disease. N Engl J Med. (2003) 349(20):1925–34. doi: 10.1056/NEJMoa035275
59. Zhou H, Wang L, Zhang C, Qiu X, Hu W, van der Stelt O, et al. Acute effects of subthalamic deep brain stimulation on motor outcomes in Parkinson's disease; 13 year follow up. Front Neurol. (2019) 10:689. doi: 10.3389/fneur.2019.00689
60. Tsuboi T, Lemos Melo Lobo Jofili Lopes J, Patel B, Legacy J, Moore K, Eisinger RS, et al. Parkinson's disease motor subtypes and bilateral GPi deep brain stimulation: one-year outcomes. Parkinsonism Relat Disord. (2020) 75:7–13. doi: 10.1016/j.parkreldis.2020.05.004
61. Odekerken VJ, Boel JA, Schmand BA, de Haan RJ, Figee M, van den Munckhof P, et al. GPi vs STN deep brain stimulation for Parkinson disease: three-year follow-up. Neurology. (2016) 86(8):755–61. doi: 10.1212/WNL.0000000000002401
62. Abode-Iyamah KO, Chiang HY, Woodroffe RW, Park B, Jareczek FJ, Nagahama Y, et al. Deep brain stimulation hardware-related infections: 10-year experience at a single institution. J Neurosurg. (2018) 130(2):1–10. doi: 10.3171/2017.9.JNS1780
63. Bernstein JE, Kashyap S, Ray K, Ananda A. Infections in deep brain stimulator surgery. Cureus. (2019) 11(8):e5440. doi: 10.7759/cureus.5440
64. Fenoy AJ, Simpson RK Jr. Management of device-related wound complications in deep brain stimulation surgery. J Neurosurg. (2012) 116(6):1324–32. doi: 10.3171/2012.1.JNS111798
65. Kondapavulur S, Burke JF, Volz M, Wang DD, Starr PA. Use of topical vancomycin powder to reduce surgical site infections after deep brain stimulation surgery: UCSF experience and meta-analysis. Stereotact Funct Neurosurg. (2022) 100(2):130–9. doi: 10.1159/000520197
66. Voges J, Waerzeggers Y, Maarouf M, Lehrke R, Koulousakis A, Lenartz D, et al. Deep-brain stimulation: long-term analysis of complications caused by hardware and surgery—experiences from a single centre. J Neurol Neurosurg Psychiatry. (2006) 77(7):868–72. doi: 10.1136/jnnp.2005.081232
67. Kenney C, Simpson R, Hunter C, Ondo W, Almaguer M, Davidson A, et al. Short-term and long-term safety of deep brain stimulation in the treatment of movement disorders. J Neurosurg. (2007) 106(4):621–5. doi: 10.3171/jns.2007.106.4.621
68. Dembek TA, Reker P, Visser-Vandewalle V, Wirths J, Treuer H, Klehr M, et al. Directional DBS increases side-effect thresholds—a prospective, double-blind trial. Mov Disord. (2017) 32(10):1380–8. doi: 10.1002/mds.27093
69. Merola A, Singh J, Reeves K, Changizi B, Goetz S, Rossi L, et al. New frontiers for deep brain stimulation: directionality, sensing technologies, remote programming, robotic stereotactic assistance, asleep procedures, and connectomics. Front Neurol. (2021) 12:694747. doi: 10.3389/fneur.2021.694747
70. Waln O, Jimenez-Shahed J. Rechargeable deep brain stimulation implantable pulse generators in movement disorders: patient satisfaction and conversion parameters. Neuromodulation. (2014) 17(5):425–30, discussion 430. doi: 10.1111/ner.12115
71. Krack P, Martinez-Fernandez R, Del Alamo M, Obeso JA. Current applications and limitations of surgical treatments for movement disorders. Mov Disord. (2017) 32(1):36–52. doi: 10.1002/mds.26890
72. Pugh J. No going back? Reversibility and why it matters for deep brain stimulation. J Med Ethics. (2019) 45(4):225–30. doi: 10.1136/medethics-2018-105139
73. Grill WM. Signal considerations for chronically implanted electrodes for brain interfacing. In: Reichert WM, editor. Indwelling neural implants: Strategies for contending with the in vivo environment. Boca Raton, FL: Royal College of Physicians of London (2008).
74. Ruge D, Cif L, Limousin P, Gonzalez V, Vasques X, Hariz MI, et al. Shaping reversibility? Long-term deep brain stimulation in dystonia: the relationship between effects on electrophysiology and clinical symptoms. Brain. (2011) 134(Pt 7):2106–15. doi: 10.1093/brain/awr122
75. National Collaborating Centre for Chronic Conditions (UK). Parkinson’s disease: national clinical guideline for diagnosis and management in primary and secondary care. London: Royal College of Physicians (2006).
76. Van Laar AD, Van Laar VS, San Sebastian W, Merola A, Elder JB, Lonser RR, et al. An update on gene therapy approaches for Parkinson's disease: restoration of dopaminergic function. J Parkinsons Dis. (2021) 11(s2):S173–S82. doi: 10.3233/JPD-212724
77. Chu Y, Bartus RT, Manfredsson FP, Olanow CW, Kordower JH. Long-term post-mortem studies following neurturin gene therapy in patients with advanced Parkinson's disease. Brain. (2020) 143(3):960–75. doi: 10.1093/brain/awaa020
78. Eberling JL, Jagust WJ, Christine CW, Starr P, Larson P, Bankiewicz KS, et al. Results from a phase I safety trial of hAADC gene therapy for Parkinson disease. Neurology. (2008) 70(21):1980–3. doi: 10.1212/01.wnl.0000312381.29287.ff
79. Heiss JD, Lungu C, Hammoud DA, Herscovitch P, Ehrlich DJ, Argersinger DP, et al. Trial of magnetic resonance-guided putaminal gene therapy for advanced Parkinson's disease. Mov Disord. (2019) 34(7):1073–8. doi: 10.1002/mds.27724
80. Marks WJ Jr., Bartus RT, Siffert J, Davis CS, Lozano A, Boulis N, et al. Gene delivery of AAV2-neurturin for Parkinson's disease: a double-blind, randomised, controlled trial. Lancet Neurol. (2010) 9(12):1164–72. doi: 10.1016/S1474-4422(10)70254-4
81. Palfi S, Gurruchaga JM, Lepetit H, Howard K, Ralph GS, Mason S, et al. Long-term follow-up of a phase I/II study of ProSavin, a lentiviral vector gene therapy for Parkinson's disease. Hum Gene Ther Clin Dev. (2018) 29(3):148–55. doi: 10.1089/humc.2018.081
82. Brady ML, Raghavan R, Alexander A, Kubota K, Sillay K, Emborg ME. Pathways of infusate loss during convection-enhanced delivery into the putamen nucleus. Stereotact Funct Neurosurg. (2013) 91(2):69–78. doi: 10.1159/000342492
83. Richardson RM, Kells AP, Rosenbluth KH, Salegio EA, Fiandaca MS, Larson PS, et al. Interventional MRI-guided putaminal delivery of AAV2-GDNF for a planned clinical trial in Parkinson's disease. Mol Ther. (2011) 19(6):1048–57. doi: 10.1038/mt.2011.11
84. Varenika V, Dickinson P, Bringas J, LeCouteur R, Higgins R, Park J, et al. Detection of infusate leakage in the brain using real-time imaging of convection-enhanced delivery. J Neurosurg. (2008) 109(5):874–80. doi: 10.3171/JNS/2008/109/11/0874
85. Muramatsu S, Fujimoto K, Kato S, Mizukami H, Asari S, Ikeguchi K, et al. A phase I study of aromatic L-amino acid decarboxylase gene therapy for Parkinson's disease. Mol Ther. (2010) 18(9):1731–5. doi: 10.1038/mt.2010.135
86. Christine CW, Starr PA, Larson PS, Eberling JL, Jagust WJ, Hawkins RA, et al. Safety and tolerability of putaminal AADC gene therapy for Parkinson disease. Neurology. (2009) 73(20):1662–9. doi: 10.1212/WNL.0b013e3181c29356
87. Christine CW, Bankiewicz KS, Van Laar AD, Richardson RM, Ravina B, Kells AP, et al. Magnetic resonance imaging-guided phase 1 trial of putaminal AADC gene therapy for Parkinson's disease. Ann Neurol. (2019) 85(5):704–14. doi: 10.1002/ana.25450
88. Mittermeyer G, Christine CW, Rosenbluth KH, Baker SL, Starr P, Larson P, et al. Long-term evaluation of a phase 1 study of AADC gene therapy for Parkinson's disease. Hum Gene Ther. (2012) 23(4):377–81. doi: 10.1089/hum.2011.220
89. Palfi S, Gurruchaga JM, Ralph GS, Lepetit H, Lavisse S, Buttery PC, et al. Long-term safety and tolerability of ProSavin, a lentiviral vector-based gene therapy for Parkinson's disease: a dose escalation, open-label, phase 1/2 trial. Lancet. (2014) 383(9923):1138–46. doi: 10.1016/S0140-6736(13)61939-X
90. Axovant announces positive six-month follow-up data from second cohort of SUNRISE-PD phase 2 trial of AXO-lenti-PD gene therapy [press release]. (2021).
91. Kaplitt MG, Feigin A, Tang C, Fitzsimons HL, Mattis P, Lawlor PA, et al. Safety and tolerability of gene therapy with an adeno-associated virus (AAV) borne GAD gene for Parkinson's disease: an open label, phase I trial. Lancet. (2007) 369(9579):2097–105. doi: 10.1016/S0140-6736(07)60982-9
92. LeWitt PA, Rezai AR, Leehey MA, Ojemann SG, Flaherty AW, Eskandar EN, et al. AAV2-GAD gene therapy for advanced Parkinson's disease: a double-blind, sham-surgery controlled, randomised trial. Lancet Neurol. (2011) 10(4):309–19. doi: 10.1016/S1474-4422(11)70039-4
93. Niethammer M, Tang CC, LeWitt PA, Rezai AR, Leehey MA, Ojemann SG, et al. Long-term follow-up of a randomized AAV2-GAD gene therapy trial for Parkinson's disease. JCI Insight. (2017) 2(7):e90133. doi: 10.1172/jci.insight.90133
94. Jankovic J, Chen S, Le WD. The role of Nurr1 in the development of dopaminergic neurons and Parkinson's disease. Prog Neurobiol. (2005) 77(1-2):128–38. doi: 10.1016/j.pneurobio.2005.09.001
95. Whone A, Luz M, Boca M, Woolley M, Mooney L, Dharia S, et al. Randomized trial of intermittent intraputamenal glial cell line-derived neurotrophic factor in Parkinson's disease. Brain. (2019) 142(3):512–25. doi: 10.1093/brain/awz023
96. Fan Y, Winanto , Ng SY. Replacing what's lost: a new era of stem cell therapy for Parkinson's disease. Transl Neurodegener. 2020;9:2. doi: 10.1186/s40035-019-0180-x
97. Liu Z, Cheung HH. Stem cell-based therapies for Parkinson disease. Int J Mol Sci. (2020) 21(21):1–17. doi: 10.3390/ijms21218060
98. Kefalopoulou Z, Politis M, Piccini P, Mencacci N, Bhatia K, Jahanshahi M, et al. Long-term clinical outcome of fetal cell transplantation for Parkinson disease: two case reports. JAMA Neurol. (2014) 71(1):83–7. doi: 10.1001/jamaneurol.2013.4749
99. Gugliandolo A, Bramanti P, Mazzon E. Mesenchymal stem cell therapy in Parkinson's disease animal models. Curr Res Transl Med. (2017) 65(2):51–60. doi: 10.1016/j.retram.2016.10.007
Keywords: movement disorders, Parkinson's disease, deep brain stimulation, gene therapy, advanced therapies, focused ultrasound
Citation: Serva SN, Bernstein J, Thompson JA, Kern DS and Ojemann SG (2022) An update on advanced therapies for Parkinson's disease: From gene therapy to neuromodulation. Front. Surg. 9:863921. doi: 10.3389/fsurg.2022.863921
Received: 27 January 2022; Accepted: 30 August 2022;
Published: 23 September 2022.
Edited by:
Ricardo Santos De Oliveira, University of São Paulo, BrazilReviewed by:
Luciano Furlanetti, King's College London, United Kingdom© 2022 Serva, Bernstein, Thompson, Kern and Ojemann. This is an open-access article distributed under the terms of the Creative Commons Attribution License (CC BY). The use, distribution or reproduction in other forums is permitted, provided the original author(s) and the copyright owner(s) are credited and that the original publication in this journal is cited, in accordance with accepted academic practice. No use, distribution or reproduction is permitted which does not comply with these terms.
*Correspondence: Steven G. Ojemann c3RldmVuLm9qZW1hbm5AY3VhbnNjaHV0LmVkdQ== Drew S. Kern ZHJldy5rZXJuQGN1YW5zY2h1dHouZWR1
Specialty Section: This article was submitted to Neurosurgery, a section of the journal Frontiers in Surgery
Disclaimer: All claims expressed in this article are solely those of the authors and do not necessarily represent those of their affiliated organizations, or those of the publisher, the editors and the reviewers. Any product that may be evaluated in this article or claim that may be made by its manufacturer is not guaranteed or endorsed by the publisher.
Research integrity at Frontiers
Learn more about the work of our research integrity team to safeguard the quality of each article we publish.