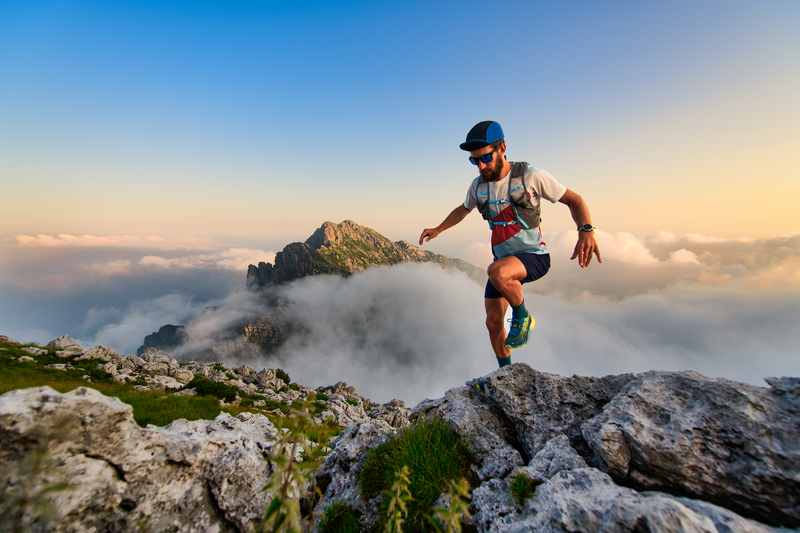
95% of researchers rate our articles as excellent or good
Learn more about the work of our research integrity team to safeguard the quality of each article we publish.
Find out more
REVIEW article
Front. Surg. , 10 March 2023
Sec. Orthopedic Surgery
Volume 9 - 2022 | https://doi.org/10.3389/fsurg.2022.1089244
This article is part of the Research Topic Enhanced Recovery Pathways in Geriatric Orthopaedics View all 14 articles
Low back pain is one of the top disorders that leads to disability and affects disability-adjusted life years (DALY) globally. Intervertebral disc degeneration (IDD) and subsequent discogenic pain composed major causes of low back pain. Recent studies have identified several important risk factors contributing to IDD's development, such as inflammation, mechanical imbalance, and aging. Based on these etiology findings, three categories of animal models for inducing IDD are developed: the damage-induced model, the mechanical model, and the spontaneous model. These models are essential measures in studying the natural history of IDD and finding the possible therapeutic target against IDD. In this review, we will discuss the technical details of these models, the duration between model establishment, the occurrence of observable degeneration, and the potential in different study ranges. In promoting future research for IDD, each animal model should examine its concordance with natural IDD pathogenesis in humans. We hope this review can enhance the understanding and proper use of multiple animal models, which may attract more attention to this disease and contribute to translation research.
Low back pain (LBP) is one of the most common disorders affecting elder and middle-aged persons. It has been estimated that low back pain is the fourth most prevalent disease that causes disability worldwide (1). In the last 30 years, DALYs of low back pain has increased by approximately 33% (2). In the US, the total cost of LBP is 7.4 billion US dollars in 2008 (3). Given the high prevalence and high cost of LBP, it is urgent to search for the pathogenesis of LBP and develop treatments for alleviating LBP (4).
Intervertebral disc degeneration (IDD) is one of the major causes of LBP (5–7). Approximately 40% of LBP presented with the feature of IDD (8). The Intervertebral disc consists of three major histological distinct components: annulus fibrosus (AF), nucleus pulposus (NP), and cartilage endplate (EP). In undegenerated intervertebral disc, NP was surrounded by AF with CEP covering the interface between AF and bony vertebrae. Known risk factors for IDD include excessive mechanical loading, obesity, spine imbalance, diabetes mellitus, and genetic predisposition (9–13). When the process of IDD commences, internal and external stimuli triggers inflammation and oxidative stress. The overproduction of inflammatory mediators, such as tumor tumor necrosis factor-alpha (TNF-α), interleukin 1-beta (IL-1β), and interleukin 6 (IL-6), led to increased expression of extracellular matrix (ECM) degradation enzymes (14, 15). The overproduction of ECM degradation enzymes leads to loss of collagen type II and aggrecan in NP and subsequently compromises the water-retaining ability (16). Loss of water and ECM components leads to biomechanical changes in the intervertebral disc and exacerbates IDD (17, 18).
It is of great significance to develop and utilize IDD animal models to understand the pathogenesis mechanism and test novel treatments. We elaborate on currently available animal models and provide an overview of the utility of these models. In this review, we tried to present the advantages and disadvantages of these models, discuss the duration of constructing these models, and include some necessary technical details of model construction (Figure 1). Finally, we hope this review will contribute to the appropriate selection of IDD models and promote the development of new therapeutic strategies.
Figure 1. Summary of the animal models demonstrated in this review, including the damage-induced, mechanical, and spontaneous models.
The needle puncture model was established through puncture of the intervertebral disc from either the posterior or anterior direction. This model is most commonly used in small animals, including rats, mice, and rabbits. However, needle puncture is also applicable in establishing the IDD model on larger animals like dog (19), sheep (20), bovine (21), and rhesus monkeys (22). The needle puncture model is easy to install by inserting the needle into AF without disrupting NP. The insertion depth can be determined by radiography monitoring or the length of the needle emerged. After insertion, the needles are usually placed in the disc for a period of 30 s to 1 min (23, 24). A proportion of studies rotated the needle for 180°–360° before being placed in the disc (25, 26).
Different diameters of needles are used to induce the IDD animal model. Chen et al. inserted a 21G needle into the AF of rats and IDD was observed in the corresponding level 4 weeks post-operation (27). Our experiments also confirmed the successful induction of IDD histologically and radiographically four weeks after 21G needle insertion (28). Smaller and larger needles are also demonstrated to induce IDD in rats post-surgery. Issy et al. showed that 30G needles insertion can cause IDD 6 weeks post-operative (29). Matta et al. performed the puncture model with 32G needles, and the animals were euthanized ten weeks after modeling (30). In rats inserted with larger needles (20G), the occurrence of IDD is more rapid than minor needle insertion as the histological IDD was observed one week after injury (31, 32). Masuda et al. compared the histological damage of needle puncture using 16G, 18G, and 21G needles in the rabbit model of IDD. Generally, these studies suggested that the radiographic and histologic damage is more severe in mice punctured with a larger needle (33). In large animals, a larger size of needles is needed to induce the IDD model. Tellegen et al. inserted 18G needles on the AF of dogs under the monitoring of intra-operative fluoroscopy. These studies suggested that the development of IDD is closed related to the diameter of needles and choosing the appropriate needle and sampling time are crucial to the conclusion.
Punctures in both lumbar (L) discs and coccygeal (Co) discs can induce IDD. In the lumbar disc puncture model, skin incisions are needed to expose the lumbar disc. Kim et al. performed a right hemilaminectomy to expose the L5/6 disc and inserted a needle. Von Frey test, Basso-Beattie-Bresnahan scale, and the horizontal ladder test found that the rats emerged behavior in response to pain as early as 1-week post-surgery (34). Coccygeal disc puncture can be performed with or without fluoroscopy (35, 36). Co5/6, Co6/7, Co7/8, Co8/9, and Co9/10 levels are usually selected for the IDD modeling (37, 38). Isa et al. established the IDD model by puncturing Co4/5 and Co5/6 discs and then investigated the pain response in the ventral base of the tail by Hargreaves test, von Frey test, and tail-flick test (39). Interestingly, lumbar discs and coccygeal discs puncture may represent the different modeling scenarios. In evaluating the behavioral parameters of IDD, lumbar disc puncture seems more resemble with IDD in patients since it may induce both leg and back pain. The coccygeal discs puncture models are easier to perform and may benefit the research for alleviating the disc degeneration process.
Needle puncture combined with intradiscal injection of reagents also represents a method for constructing the IDD model. Since pro-inflammatory factors contribute to the onset of IDD (5), injection of pro-inflammatory factors such as TNF-α and IL-1β into the intervertebral disc can induce IDD in different animal models (34, 40). Notably, the pain response to needle puncture is associated with the expression level of pro-inflammatory cytokines in the dorsal root ganglion (41). Norcross et al. compared injection of chondroitinase ABS or phosphate-buffered saline into the intervertebral disc of rats. Disc height and histological examination showed that chondroitinase injection leads to the observable degeneration on day 14 of experiment (42). Complete Freund's adjuvant (CFA), a tissue destruction reagent, was injected into to intervertebral disc to induce IDD (43). The rats were subjected to the behavioral test and found CFA injection successfully induced back pain and inflammatory factors accumulation (44). Wei et al. injected pingyangmycin or bleomycin into the subchondral bone adjacent to the lumbar intervertebral disc of rhesus monkeys, and degeneration was observed by MRI 3 months after injection (45, 46).
Discectomy is the standard surgical procedure for treating intervertebral disc herniation caused by IDD (47). Discectomy can relieve the nerve root compression by disc herniation, but the loss of NP tissue may cause the subsequent collapse of the intervertebral space (48). Therefore, discectomy model is suitable for studying disc-healing therapy, especially implants or biomaterials. The discectomy was performed on multiple animals, including rats (49), rabbits (50), sheep (51), pigs (52), and bovine (53). Since many studies suggested that goats and sheep possess similar biomechanical properties that are similar and comparable to the human spine, both animals are considered to be suitable for investigating spine mechanical properties (54, 55). Sloan et al. performed the discectomy in 3-4-year-old Finn sheep by performing a 3 × 10 mm annulotomy and then removing 200 mg of NP tissue (56). The intervertebral discs were subjected to histological examination six weeks after surgery. NP heterogeneity, AF lesions, and increased proteoglycan staining were observed in AF (56). Oehme et al. investigated a mini-invasive approach in sheep by making a 3 × 5 mm rectangular annular incision on AF using an 11-blade scalpel (57). A mixture of NF and AF tissue weighing 200 mg was removed.
Nucleuotomy refers to the partial excision of NP tissue with little disturbance of AF structure (58). Schwan et al. introduced a novel surgical approach to performing nucleotomy (59). A skin incision was made, and the corresponding disc level was determined with x-ray fluoroscopy. The discs were punctured with a straight awl through the whole layer of AF, and surgical channels were created. A 12 cm long rongeur was inserted through the tunnel, and approximately 0.15 cm3 of NP tissue was removed (59, 60). Partial nucleotomy resulted in the loss of disc height six weeks after surgery. Takeoka et al. performed percutaneous nucleotomy in rats according to the method by Nishimura et al., and loss of disc height was observed as early as seven days post-surgery (61).
In 1991, Miyamoto et al. proposed a model of constructing cervical spondylosis by surgically induced spine instability (62). The posterior paravertebral muscles were separated, and the cervical, thoracic and lumbar spine was exposed. Then the spinous processes and attached supraspinous and interspinous ligaments were resected. The model was commonly used in constructing the IDD model in the lumbar spine and is therefore referred to lumbar spine instability (LSI) model. Zheng et al. applied the L3–L4 LSI to investigate the contributory role of parathyroid hormone in maintaining intervertebral disc homeostasis (63). Xue et al. further exploited the model to examine the role of skeletal interoception in causing EP degeneration and spinal-associated pain (64). Recently, Liu et al. demonstrated that the LSI model leads to spinal hypersensitivity in DRG, which explains the pain caused by IDD (65).
As for constructing the caudal spine instability model, Bian et al. stapped through the full depth of the Co7/8 AF, and then removed the NP (66). The adjacent Co8/9 intervertebral disc was subjected to histological analysis four weeks post-surgery and confirmed the successful establishment of the IDD model. Another study by the same group also made an incision into the whole layer of AF and performed NP removal to induce IDD. The bony EP was then analyzed and found that CSI leads to bony EP porosity of the same level (67).
Clinical observations suggested spinal deformities such as adolescent idiopathic scoliosis (AIS) and Scheuermann's disease are associated with IDD (68, 69). The spine deformity alters the normal pattern of force distribution and undermines the mechanical property. Tail-looping model is a novel method to construct the IDD model by creating force imbalance within the intervertebral disc. Saikai et al. looped the tail of mice and made fixation between Co5 and Co13 vertebrae with 0.8-mm stainless steel wire (70). The extra vertebrae were excised. The NP of Co7/8, and Co8/9 discs were aspirated to generate more severe degeneration. In this model, the Co2/3 and Co3/4 discs were selected as control discs, while Co10/11 and Co11/12 discs were chosen as mildly degeneration discs. The researchers demonstrated that histological severity correlates with previous treatment, and degeneration occurred as early as eight weeks after looping (70). Nakamichi et al. established the tail-looping induced IDD model by joining Co2 and Co9 vertebrae together (71). In this study, outer AF was removed, and the role of Mohawk-induced AF regeneration was explored. Further, Huang et al. modified the looping method by tying the tail with thin wire instead of stitching the vertebrae. The model was successfully constructed after two months of fixation and continued with the adenovirus treatment for one month before sample collection (72).
Under the condition of compression and angulation, the intervertebral discs may become narrowed and stiffer (73). MacLean et al. annexed external rings to adjacent levels of intervertebral discs by inserting 0.5 mm pins transfixing the vertebral bodies percutaneously (74). Stokes et al. modified the method by installing rings either parallel to each other or with an angle of 15-degree (75). Their results showed that 15-degree angulation plus compression yielded greater disc space loss. Hirata et al. exerted temporary static compression using an Ilizarov-type apparatus with springs between Co8 and Co10 (76). An axial force of 1.3 MPa was exerted and subsequent analysis found that the compression reproduced different stages of degeneration. The same compression pressure was adopted by other studies (77, 78), suggesting this pressure may be the appropriate pressure for constructing model. In a recent study, Ji et al. developed a novel device by inserting Kirschner wire into Co8 and Co10 vertebral bodies (79). Then the tail was bent for 40° and springs are used to exert 1.8N and 4.5N of force on Co8/9 and Co9/10 intervertebral disc. Pfirrmann grades and histological examinations revealed the occurrence of IDD two weeks after surgery. The severity of degeneration correlates positively with the force exerted (79).
In addition to constructing the IDD model on a histological and radiographic level, the axial-compressing external fixation devices can simulate pain caused by IDD. Miyagi et al. used both the compression model and needle puncture model to establish IDD in rats and found that the pro-inflammatory factors were elevated (80). Moreover, the positive labeling of calcitonin gene-related peptide (CGRP) neurons increased, suggesting a potential mechanism IDD leads to low back pain (80). Since many studies have shown neurogenic factors like brain-derived neurotrophic factor (BDNF), nerve growth factor (NGF), and CGRP and closely related to discogenic pain of IDD (81, 82), the compression model is applicable in pain-related phenomenon.
High-frequency, low-amplitude whole-body vibration (WBV) is a common physical therapy in some disorders. Notably, WBV has been used as an adjuvant treatment for osteoporosis, muscle weakness, and low back pain (83, 84). However, it remains controversial whether WBV retarded the progression of IDD. Clinical observations found that workers exposed to occupational vibration are susceptible to IDD (85). Studies have linked vibration exposure with increased matrix metalloproteinase and decreased ECM (86), suggesting vibration is a potential risk for IDD progression. In a study by McCann et al., they applied clinically used vibration frequency (45 Hz with peak acceleration at 0.3 g for 30 min per day and 5 days per week) on mice for four weeks (87). The morphologic grade was analyzed and confirmed the IDD occurrence, especially characterized by AF degeneration. Furthermore, they found that 4-week WBV followed by 4-week cessation did not reverse the IDD in mice, suggesting the damage is permanent (88). Zeeman et al. demonstrated that 8 Hz and 15 Hz WBV is associated with long-lasting cervical and lumbar pain in rats (89), indicating the WBV model may also be useful in pain-related research. Although it is now known that WBV contributes to the development of IDD and IDD-related pain, the ideal vibration mode (time, frequency, orientation) to induce IDD still needs more investigation (90).
The bipedal animal model was established by forelimb amputation in rodents. After forelimb amputation, a forced bipedal stance mimics the bipedal gait of human(91). Liang et al. performed amputation surgery on 1-month-old male rats and the rats were kept in custom-made cages to force them to stand in an upright position (92). The rat was kept for 5 months and 7 months before histological analysis. Loss of cervical disc height was observed in the amputation surgery group five months after surgery, and the height loss was more severe seven months after surgery. The down-regulation of Col2a1 and aggrecan was also observed and may decrease anti-compression capacity (92). Using the bipedal rat model, Liu et al. discovered ligustrazine attenuate cartilage EP hypertrophy, a characteristic of IDD, within an observation period of 9 months (93). Kong et al. Found the myocardin-related transcription factor A (MRTF-A) inhibitor CCG-1423 attenuated IDD progression over six months (94). Although the bipedal animal model mimics the upright posture similar to human beings, this model may take as long as six months to gain a histologically observable degeneration. Another concern that hampers the application of this model is animal welfare since amputation surgery causes trauma and alters the feeding habits of animals (91).
In addition to forelimb amputation, bipedal models are modified to yield better potency. Liang et al. performed both brachial plexus rhizotomy and tail amputation on 4-weeks-old female rats (95). The rats were euthanized six weeks post-surgery and lumbar discs were dissected (L1–S1) and subjected to qPCR analysis. Data showed the loss of ECM matrix in six weeks post-surgery, suggesting the efficacy of this model (95). Recently, Ao et al. developed a novel bipedal model utilizing the water-escape nature of rodents without amputation surgery (96). The mice were kept in a chamber with a 5 mm depth of water on the bottom of the chamber. The mice were kept in the chamber for 6 h each day and were allowed to access water and food freely for 2 h. Because of the water-escape nature, the mice are more likely to keep an upright position in the chamber. Degeneration of the facet joint and the intervertebral disc was observed 6-week after treatment (96). Lao et al. developed a hot plate cage to exert accumulated spinal axial force on mice's spine (97). The mice were placed on the 50 °C hot plate for 15 min per day and were forced to jump before returning to the regular cage. IDD was observed one-month post-modeling and progressed more severely in 3-months of observation (97).
Certain gene deficiency impairs intervertebral disc metabolic homeostasis and structural integrity. Secreted protein acidic and rich in cysteine (SPARC) is a matricellular protein involved in the pathogenesis of IDD (98). The expression level of SPARC decreased with aging and intervertebral disc degeneration. Moreover, it has been demonstrated that SPARC deletion accelerates IDD in mice (98, 99). Histological analysis revealed that herniations of lower lumbar discs in SPARC-null mice occur as early as 14-month-old. Millecamps et al. discovered that in addition to typical IDD pathologic features, the SPARC-null mice also developed the feature of chronic back pain (100). The chronic back pain was characterized by hind paw sensitivity to mechanical and cold stimuli, intolerance to axial stretching, and motor impairment, which all implied nerve root impairment. More studies confirmed the association between low back pain and IDD in the SPARC-null mice (101). In a study by Lee et al., the SPARC-null mice developed IDD and low back pain at 14 months (98). Krock et al. found that the SPARC-null mice presented with low back pain at a relatively young age of 7–9 months (102).
SM/J mice is a strain characterized by lacking cartilage regeneration ability (103). It is reasonable to propose the hypothesis that intervertebral disc homeostasis in SM/J mice may be disrupted. Choi et al. found that the cellularity and matrix components of SM/J mice are altered at a young age (104). Severe IDD was observed in 17-week-old SM/J mice, marked by increased apoptosis and collagen degradation. Moreover, the intervertebral disc of SM/J mice showed increased stiffness and the vertebral bone showed decreased bone quality (104). Zhang et al. compared LG/J mice, a mice strain characterized by a remarkable ability to heal after cartilage injury, with SM/J mice in spontaneous IDD (105). Their result suggested the potential use of combining LG/J mice and SM/J mice in the genetic and biological study of IDD. Study by Novais et al., demonstrated that SM/J mice have increased susceptibility to IDD. However, the same study found that the LG/J mice showed increased disc calcification and degeneration compared with the BL6 strain, which is inconsistent with research mentioned above (106).
Studies have identified many genes associated with IDD, and knockout of these genes may also replicate the phenotype of spontaneous IDD. For example, collagen type II, encoded by the Col2a1 gene, has been identified as the critical regulator in intervertebral discs embryonic development (107). Col2a1 knockout showed the feature of AF glycosaminoglycans loss and EP degeneration in 9-month-old mice (108). Deletion in other collagen encoding genes, including Col9a2 (109), and Col9a1 (110), also exhibited the feature of spontaneous IDD. Besides the extracellular matrix components, loss in other genes (e.g,. Smad3 (111), IL-1rn (112), Hif-1a (113), Apoe 114) may also contribute to IDD's pathogenesis (Table 1). However, because IDD has long been considered a heterogeneous disease with different etiology, the use of the gene-specific knockout mice model may be limited.
In 1988, Silberberg demonstrated that the sand rat (Psammomys obesus), a small desert rodent, is susceptible to age-related IDD (127). The severity of IDD was correlated with greater age. Helen et al. examined the age-related IDD of sand rats in a more detailed manner. The intervertebral discs of younger (2–11.9 months) and older (12–25 months) animals were collected and subjected to histological analysis. Their results suggested that the cervical spine of both younger and older sand rats is more likely to develop osteophytes than the lumbar spine. Moreover, the occurrence of osteophytes correlates with the extrusion of the intervertebral disc (128).
Some previous studies have demonstrated that mice are less susceptible to age-related IDD, which may limit the application of this model to some extent. For example, Marfia et al. showed that half of the mice did not exhibit IDD via MRI analysis in 19 months (129). Ohnishi et al.explored the availability of age-related IDD model in mice by MRI analysis followed by Pfirrmann classification and histological analysis followed by classification proposed in this research (130). They analyzed the mice aged 6 months, 14 months, and 22 months with both Pfirrmann classification and histological classification and found the feature of IDD progressed with increased age. The 14-months-old mice exhibited mild IDD while the 22-months-old mice developed moderate to severe IDD, which suggested that at least a 14-month follow-up is required for age-related IDD in C57BL/6 mice (130). Aging alters IDD's metabolism in many aspects, such as elevated chondrocyte hypertrophy and loss of notochordal markers (131).
The age-related IDD mice model is also widely used in investigating factors associated with senescence and longevity. Lin et al. found that tenomodulin (Tnmd), an anti-angiogenic transmembrane glycoprotein, maintained the structural integrity and matrix gene expression in outer AF and NP (132). Loss of Tnmd gene leads to early-onset IDD in 6-month-old mice and the IDD progressed more severely in 18-month-old mice compared with wild-type mice. Novais et al. investigated the role of senolytic drugs in ameliorating age-related IDD and defined different age groups, namely healthy adult (6-month-old), middle-aged (14-month-old), aged (18-month-old), and old-aged (23-month-old) (133). The mice started senolysis treatment at 6,14 and 18 months and IDD was harvested at 23 months.
Intervertebral disc degeneration is a disease with complex etiology and clinical heterogeneity. Therefore, it is hard to find an ideal animal model that mimics all the inherent pathophysiology of IDD. Among these pathophysiology changes, some features are considered extra important, including loss of extracellular matrix and proteoglycans, biomechanical property alternations, and increased cell death. Discogenic pain is not necessarily associated with the severity of IDD (134), but the pain is the most disturbing symptom and chief complaint in IDD cases. Lack of early signs impairs the ability of early identification of IDD. Thus the disease is commonly irreversible at a later stage. These remind us that more in-detail studies into the common pattern of human IDD development are needed. Encouragingly, some recent studies combined new technology, including single-cell RNA-sequencing, with human specimens to discover the disease's very nature. Recent studies by Gan et al. (135), Gao et al. (136), Han et al. (137) and Zhang et al. (138) made delightful exploration into the possible reason for IDD initiation. Subsequent studies are needed to determine the similarities and differences between patients with different natural disease histories.
In developing appropriate animal models, some important considerations need extensive attention. Firstly, the upright position determined the unique mechanical property of the human spine and intervertebral disc. Secondly, the notochordal cells undergo apoptosis and differentiation after birth and are absent in the adult human spine. But notochordal cells may remain in the intervertebral discs in certain specimens, which may promote the regeneration ability of damaged discs. Thirdly, the duration between modeling and detectable degeneration should be taken into consideration. If the degeneration occurs too soon, it is unlikely to replicate the actual circumstances in IDD. Severe structural destruction will conceal the effectiveness of certain therapy. Lastly, the ethical and cost issue should also be taken into consideration.
In this review, we elaborated on the commonly used method to construct IDD models, which mainly fall into three categories: damage-induced, mechanical, and spontaneous. Damage-induced models make punctures or incisions into the intervertebral discs and impair the integrity of the disc structure, while mechanical models exert external force into the disc and accelerate the degeneration process. The spontaneous models focus on common IDD causes, such as aging and collagen loss, which spontaneously lead to IDD development. Each category replicates a certain stage of IDD to some extent. Therefore, in searching for possible treatments for IDD, we should emphasize the importance of selecting correct animal models. For example, SPARC-null mice develop significant chronic back pain, making it suitable for researching IDD-related pain. The discectomy model mimiked the clinical situation of disc resection and seemed ideal for developing disc regeneration therapy. Integrating more than one IDD animal model into one study is becoming more common (139). Combining these models is a helpful approach to gaining solid evidence for the efficacy of specific interventions.
In conclusion, animal models are indispensable for understanding, characterizing, and treating disc degeneration. However, despite the methods listed in this review, there is still no consensus on which model best mimics IDD. More importantly, there is still some gap between model-induced IDD and actual clinical features. Further studies are needed to determine the fidelity of these models and eventually contribute to developing new IDD therapeutic strategies.
TL, BG, and JZ: performed literature search and summarized related literature. XQ, TC, YL, and JQ: prepared the manuscript draft. TL, XQ, YL, JZ and WG: revised and finalized the manuscript. XQ and YL provided the idea for this study. All authors contributed to the article and approved the submitted version.
This work was supported by the grants from the Guangdong Basic and Applied Basic Research Foundation (No. 2019A1515110122 & No. 2021A1515012619); Sun Yat-sen Scholarship for Young Scientist for Wenjie Gao.
The authors declare that the research was conducted in the absence of any commercial or financial relationships that could be construed as a potential conflict of interest.
All claims expressed in this article are solely those of the authors and do not necessarily represent those of their affiliated organizations, or those of the publisher, the editors and the reviewers. Any product that may be evaluated in this article, or claim that may be made by its manufacturer, is not guaranteed or endorsed by the publisher.
1. GBD 2019 Diseases and Injuries Collaborators. Global burden of 369 diseases and injuries in 204 countries and territories, 1990–2019: a systematic analysis for the global burden of disease study 2019. Lancet. (2020) 396:1204–22. doi: 10.1016/S0140-6736(20)30925-9
2. Tucker HR, Scaff K, McCloud T, Carlomagno K, Daly K, Garcia A, et al. Harms and benefits of opioids for management of non-surgical acute and chronic low back pain: a systematic review. Br J Sports Med. (2020) 54:664. doi: 10.1136/bjsports-2018-099805
3. Dagenais S, Caro J, Haldeman S. A systematic review of low back pain cost of illness studies in the United States and internationally. Spine J. (2008) 8:8–20. doi: 10.1016/j.spinee.2007.10.005
4. Miyamoto GC, Lin CC, Cabral C, van Dongen JM, van Tulder MW. Cost-effectiveness of exercise therapy in the treatment of non-specific neck pain and low back pain: a systematic review with meta-analysis. Br J Sports Med. (2019) 53:172–81. doi: 10.1136/bjsports-2017-098765
5. Risbud MV, Shapiro IM. Role of cytokines in intervertebral disc degeneration: pain and disc content. Nat Rev Rheumatol. (2014) 10:44–56. doi: 10.1038/nrrheum.2013.160
6. Simon J, McAuliffe M, Shamim F, Vuong N, Tahaei A. Discogenic low back pain. Phys Med Rehabil Clin N Am. (2014) 25:305–17. doi: 10.1016/j.pmr.2014.01.006
7. Bonnheim NB, Wang L, Lazar AA, Zhou J, Chachad R, Sollmann N, et al. The contributions of cartilage endplate composition and vertebral bone marrow fat to intervertebral disc degeneration in patients with chronic low back pain. Eur Spine J. (2022) 31:1866–72. doi: 10.1007/s00586-022-07206-x
8. Cheung KM, Karppinen J, Chan D, Ho DW, Song YQ, Sham P, et al. Prevalence and pattern of lumbar magnetic resonance imaging changes in a population study of one thousand forty-three individuals. Spine. (2009) 34:934–40. doi: 10.1097/BRS.0b013e3181a01b3f
9. Fu F, Bao R, Yao S, Zhou C, Luo H, Zhang Z, et al. Aberrant spinal mechanical loading stress triggers intervertebral disc degeneration by inducing pyroptosis and nerve ingrowth. Sci Rep. (2021) 11:772. doi: 10.1038/s41598-020-80756-6
10. Samartzis D, Karppinen J, Chan D, Luk KD, Cheung KM. The association of lumbar intervertebral disc degeneration on magnetic resonance imaging with body mass index in overweight and obese adults: a population-based study. Arthritis Rheum. (2012) 64:1488–96. doi: 10.1002/art.33462
11. Alpantaki K, Kampouroglou A, Koutserimpas C, Effraimidis G, Hadjipavlou A. Diabetes mellitus as a risk factor for intervertebral disc degeneration: a critical review. Eur Spine J. (2019) 28:2129–44. doi: 10.1007/s00586-019-06029-7
12. Wong E, Altaf F, Oh LJ, Gray RJ. Adult degenerative lumbar scoliosis. Orthopedics. (2017) 40:e930–9. doi: 10.3928/01477447-20170606-02
13. Takeda K, Kou I, Hosogane N, Otomo N, Yagi M, Kaneko S, et al. Association of susceptibility genes for adolescent idiopathic scoliosis and intervertebral disc degeneration with adult spinal deformity. Spine. (2019) 44:1623–9. doi: 10.1097/BRS.0000000000003179
14. Li Y, Zhang T, Tian W, Hu H, Xin Z, Ma X, et al. Loss of TIMP3 expression induces inflammation, matrix degradation, and vascular ingrowth in nucleus pulposus: a new mechanism of intervertebral disc degeneration. Faseb J. (2020) 34:5483–98. doi: 10.1096/fj.201902364RR
15. Francisco V, Pino J, Gonzalez-Gay MA, Lago F, Karppinen J, Tervonen O, et al. A new immunometabolic perspective of intervertebral disc degeneration. Nat Rev Rheumatol. (2022) 18:47–60. doi: 10.1038/s41584-021-00713-z
16. Adams MA, Roughley PJ. What is intervertebral disc degeneration, and what causes it? Spine. (2006) 31:2151–61. doi: 10.1097/01.brs.0000231761.73859.2c
17. Vergroesen PA, Emanuel KS, Peeters M, Kingma I, Smit TH. Are axial intervertebral disc biomechanics determined by osmosis? J. Biomech. (2018) 70:4–9. doi: 10.1016/j.jbiomech.2017.04.027
18. Fields AJ, Berg-Johansen B, Metz LN, Miller S, La B, Liebenberg EC, et al. Alterations in intervertebral disc composition, matrix homeostasis and biomechanical behavior in the UCD-T2DM rat model of type 2 diabetes. J Orthop Res. (2015) 33:738–46. doi: 10.1002/jor.22807
19. Tellegen AR, Rudnik-Jansen I, Beukers M, Miranda-Bedate A, Bach FC, de Jong W, et al. Intradiscal delivery of celecoxib-loaded microspheres restores intervertebral disc integrity in a preclinical canine model. J Control Release. (2018) 286:439–50. doi: 10.1016/j.jconrel.2018.08.019
20. Vadala G, Russo F, Pattappa G, Schiuma D, Peroglio M, Benneker LM, et al. The transpedicular approach as an alternative route for intervertebral disc regeneration. Spine. (2013) 38:E319–24. doi: 10.1097/BRS.0b013e318285bc4a
21. Yang JJ, Li F, Hung KC, Hsu SH, Wang JL. Intervertebral disc needle puncture injury can be repaired using a gelatin-poly (gamma-glutamic acid) hydrogel: an in vitro bovine biomechanical validation. Eur Spine J. (2018) 27:2631–8. doi: 10.1007/s00586-018-5727-5
22. Xi Y, Kong J, Liu Y, Wang Z, Ren S, Diao Z, et al. Minimally invasive induction of an early lumbar disc degeneration model in rhesus monkeys. Spine. (2013) 38:E579–86. doi: 10.1097/BRS.0b013e31828b695b
23. Guo Q, Zhu D, Wang Y, Miao Z, Chen Z, Lin Z, et al. Targeting STING attenuates ROS induced intervertebral disc degeneration. Osteoarthritis Cartilage. (2021) 29:1213–24. doi: 10.1016/j.joca.2021.04.017
24. Xiao L, Xu SJ, Liu C, Wang J, Hu B, Xu HG. Sod2 and catalase improve pathological conditions of intervertebral disc degeneration by modifying human adipose-derived mesenchymal stem cells. Life Sci. (2021) 267:118929. doi: 10.1016/j.lfs.2020.118929
25. Lei M, Zhao K, Hua W, Wang K, Li S, Wu X, et al. An in vivo study of the effect of c-jun on intervertebral disc degeneration in rats. Bioengineered. (2021) 12:4320–30. doi: 10.1080/21655979.2021.1946459
26. Xu D, Jin H, Wen J, Chen J, Chen D, Cai N, et al. Hydrogen sulfide protects against endoplasmic reticulum stress and mitochondrial injury in nucleus pulposus cells and ameliorates intervertebral disc degeneration. Pharmacol Res. (2017) 117:357–69. doi: 10.1016/j.phrs.2017.01.005
27. Chen F, Jiang G, Liu H, Li Z, Pei Y, Wang H, et al. Melatonin alleviates intervertebral disc degeneration by disrupting the IL-1beta/NF-kappaB-NLRP3 inflammasome positive feedback loop. Bone Res. (2020) 8:10. doi: 10.1038/s41413-020-0087-2
28. Qiu X, Liang T, Wu Z, Zhu Y, Gao W, Gao B, et al. Melatonin reverses tumor necrosis factor-alpha-induced metabolic disturbance of human nucleus pulposus cells via MTNR1B/Galphai2/YAP signaling. Int J Biol Sci. (2022) 18:2202–19. doi: 10.7150/ijbs.65973
29. Issy AC, Castania V, Silveira JW, Nogueira-Barbosa MH, Salmon CE, Del-Bel E, et al. Does a small size needle puncture cause intervertebral disc changes? Acta Cir Bras. (2015) 30:574–9. doi: 10.1590/S0102-865020150080000009
30. Matta A, Karim MZ, Gerami H, Jun P, Funabashi M, Kawchuk G, et al. NTG-101: a novel molecular therapy that halts the progression of degenerative disc disease. Sci Rep. (2018) 8:16809. doi: 10.1038/s41598-018-35011-4
31. Issy AC, Castania V, Castania M, Salmon CE, Nogueira-Barbosa MH, Bel ED, et al. Experimental model of intervertebral disc degeneration by needle puncture in wistar rats. Braz J Med Biol Res. (2013) 46:235–44. doi: 10.1590/1414-431X20122429
32. Han B, Zhu K, Li FC, Xiao YX, Feng J, Shi ZL, et al. A simple disc degeneration model induced by percutaneous needle puncture in the rat tail. Spine. (2008) 33:1925–34. doi: 10.1097/BRS.0b013e31817c64a9
33. Masuda K, Aota Y, Muehleman C, Imai Y, Okuma M, Thonar EJ, et al. A novel rabbit model of mild, reproducible disc degeneration by an anulus needle puncture: correlation between the degree of disc injury and radiological and histological appearances of disc degeneration. Spine. (2005) 30:5–14. doi: 10.1097/01.brs.0000148152.04401.20
34. Kim H, Hong JY, Lee J, Jeon WJ, Ha IH. IL-1beta promotes disc degeneration and inflammation through direct injection of intervertebral disc in a rat lumbar disc herniation model. Spine J. (2021) 21:1031–41. doi: 10.1016/j.spinee.2021.01.014
35. Chen D, Xia D, Pan Z, Xu D, Zhou Y, Wu Y, et al. Metformin protects against apoptosis and senescence in nucleus pulposus cells and ameliorates disc degeneration in vivo. Cell Death Dis. (2016) 7:e2441. doi: 10.1038/cddis.2016.334
36. Tang Z, Hu B, Zang F, Wang J, Zhang X, Chen H. Nrf2 drives oxidative stress-induced autophagy in nucleus pulposus cells via a Keap1/Nrf2/p62 feedback loop to protect intervertebral disc from degeneration. Cell Death Dis. (2019) 10:510. doi: 10.1038/s41419-019-1701-3
37. Kobori S, Miyagi M, Orita S, Gemba T, Ishikawa T, Kamoda H, et al. Inhibiting IkappaB kinase-beta downregulates inflammatory cytokines in injured discs and neuropeptides in dorsal root ganglia innervating injured discs in rats. Spine. (2014) 39:1171–7. doi: 10.1097/BRS.0000000000000374
38. Liu Y, Lin J, Wu X, Guo X, Sun H, Yu B, et al. Aspirin-mediated attenuation of intervertebral disc degeneration by ameliorating reactive oxygen Species in vivo and in vitro. Oxid Med Cell Longev. (2019) 2019:7189854. doi: 10.1155/2019/7189854
39. Mohd II, Abbah SA, Kilcoyne M, Sakai D, Dockery P, Finn DP, et al. Implantation of hyaluronic acid hydrogel prevents the pain phenotype in a rat model of intervertebral disc injury. Sci Adv. (2018) 4:q597. doi: 10.1126/sciadv.aav0295
40. Kang R, Li H, Rickers K, Ringgaard S, Xie L, Bunger C. Intervertebral disc degenerative changes after intradiscal injection of TNF-alpha in a porcine model. Eur Spine J. (2015) 24:2010–6. doi: 10.1007/s00586-015-3926-x
41. Li Z, Liu H, Yang H, Wang J, Wang H, Zhang K, et al. Both expression of cytokines and posterior annulus fibrosus rupture are essential for pain behavior changes induced by degenerative intervertebral disc: an experimental study in rats. J Orthop Res. (2014) 32:262–72. doi: 10.1002/jor.22494
42. Norcross JP, Lester GE, Weinhold P, Dahners LE. An in vivo model of degenerative disc disease. J Orthop Res. (2003) 21:183–8. doi: 10.1016/S0736-0266(02)00098-0
43. Jiang H, Wang J, Xu B, Yang Q, Liu Y. Study on the expression of nerve growth associated protein-43 in rat model of intervertebral disc degeneration. J Musculoskelet Neuronal Interact. (2017) 17:104–7. PMCID: PMC5492325
44. Jung WW, Kim HS, Shon JR, Lee M, Lee SH, Sul D, et al. Intervertebral disc degeneration-induced expression of pain-related molecules: glial cell-derived neurotropic factor as a key factor. J Neurosurg Anesthesiol. (2011) 23:329–34. doi: 10.1097/ANA.0b013e318220f033
45. Wei F, Zhong R, Wang L, Zhou Z, Pan X, Cui S, et al. Pingyangmycin-induced in vivo lumbar disc degeneration model of rhesus monkeys. Spine. (2015) 40:E199–210. doi: 10.1097/BRS.0000000000000736
46. Wei F, Zhong R, Zhou Z, Wang L, Pan X, Cui S, et al. In vivo experimental intervertebral disc degeneration induced by bleomycin in the rhesus monkey. BMC Musculoskelet Disord. (2014) 15:340. doi: 10.1186/1471-2474-15-340
47. Alvi MA, Kerezoudis P, Wahood W, Goyal A, Bydon M. Operative approaches for lumbar disc herniation: a systematic review and multiple treatment meta-analysis of conventional and minimally invasive surgeries. World Neurosurg. (2018) 114:391–407. doi: 10.1016/j.wneu.2018.02.156
48. Hudson KD, Alimi M, Grunert P, Hartl R, Bonassar LJ. Recent advances in biological therapies for disc degeneration: tissue engineering of the annulus fibrosus, nucleus pulposus and whole intervertebral discs. Curr Opin Biotechnol. (2013) 24:872–9. doi: 10.1016/j.copbio.2013.04.012
49. Liu Y, Du J, Peng P, Cheng R, Lin J, Xu C, et al. Regulation of the inflammatory cycle by a controllable release hydrogel for eliminating postoperative inflammation after discectomy. Bioact Mater. (2021) 6:146–57. doi: 10.1016/j.bioactmat.2020.07.008
50. Ukeba D, Yamada K, Tsujimoto T, Ura K, Nonoyama T, Iwasaki N, et al. Bone marrow aspirate concentrate combined with in situ forming bioresorbable gel enhances intervertebral disc regeneration in rabbits. J Bone Joint Surg Am. (2021) 103:e31. doi: 10.2106/JBJS.20.00606
51. Sloan SJ, Wipplinger C, Kirnaz S, Delgado R, Huang S, Shvets G, et al. Imaging the local biochemical content of native and injured intervertebral disc using Fourier transform infrared microscopy. JOR Spine. (2020) 3:e1121. doi: 10.1002/jsp2.1121
52. Chiang CJ, Cheng CK, Sun JS, Liao CJ, Wang YH, Tsuang YH. The effect of a new anular repair after discectomy in intervertebral disc degeneration: an experimental study using a porcine spine model. Spine. (2011) 36:761–9. doi: 10.1097/BRS.0b013e3181e08f01
53. Varma DM, Lin HA, Long RG, Gold GT, Hecht AC, Iatridis JC, et al. Thermoresponsive, redox-polymerized cellulosic hydrogels undergo in situ gelation and restore intervertebral disc biomechanics post discectomy. Eur Cell Mater. (2018) 35:300–17. doi: 10.22203/eCM.v035a21
54. Alini M, Eisenstein SM, Ito K, Little C, Kettler AA, Masuda K, et al. Are animal models useful for studying human disc disorders/degeneration? Eur Spine J. (2008) 17:2–19. doi: 10.1007/s00586-007-0414-y
55. Sheng SR, Wang XY, Xu HZ, Zhu GQ, Zhou YF. Anatomy of large animal spines and its comparison to the human spine: a systematic review. Eur Spine J. (2010) 19:46–56. doi: 10.1007/s00586-009-1192-5
56. Sloan SJ, Wipplinger C, Kirnaz S, Navarro-Ramirez R, Schmidt F, McCloskey D, et al. Combined nucleus pulposus augmentation and annulus fibrosus repair prevents acute intervertebral disc degeneration after discectomy. Sci Transl Med. (2020) 58:12(534):eaay2380. doi: 10.1126/scitranslmed.aay2380
57. Oehme D, Ghosh P, Shimmon S, Wu J, McDonald C, Troupis JM, et al. Mesenchymal progenitor cells combined with pentosan polysulfate mediating disc regeneration at the time of microdiscectomy: a preliminary study in an ovine model. J Neurosurg Spine. (2014) 20:657–69. doi: 10.3171/2014.2.SPINE13760
58. Yang B, Klineberg E, O'Connell GD. Intervertebral disc mechanics with nucleotomy: differences between simple and dual loading. J Biomech Eng. (2021) 60:143(8):081002. doi: 10.1115/1.4050538
59. Schwan S, Ludtka C, Wiesner I, Baerthel A, Friedmann A, Gohre F. Percutaneous posterolateral approach for the simulation of a far-lateral disc herniation in an ovine model. Eur Spine J. (2018) 27:222–30. doi: 10.1007/s00586-017-5362-6
60. Schwan S, Ludtka C, Friedmann A, Heilmann A, Baerthel A, Brehm W, et al. Long-term pathology of ovine lumbar spine degeneration following injury via percutaneous minimally invasive partial nucleotomy. J Orthop Res. (2019) 37:2376–88. doi: 10.1002/jor.24402
61. Nishimura K, Mochida J. Percutaneous reinsertion of the nucleus pulposus. An experimental study. Spine. (1998) 23:1531–1538; 1539. doi: 10.1097/00007632-199807150-00006
62. Miyamoto S, Yonenobu K, Ono K. Experimental cervical spondylosis in the mouse. Spine. (1991) 16:S495–500. doi: 10.1097/00007632-199110001-00008
63. Zheng L, Cao Y, Ni S, Qi H, Ling Z, Xu X, et al. Ciliary parathyroid hormone signaling activates transforming growth factor-beta to maintain intervertebral disc homeostasis during aging. Bone Res. (2018) 6:21. doi: 10.1038/s41413-018-0022-y
64. Xue P, Wang S, Lyu X, Wan M, Li X, Ma L, et al. PGE2/EP4 skeleton interoception activity reduces vertebral endplate porosity and spinal pain with low-dose celecoxib. Bone Res. (2021) 9:36. doi: 10.1038/s41413-021-00155-z
65. Liu S, Wang Q, Li Z, Ma L, Li T, Li Y, et al. TRPV1 Channel activated by the PGE2/EP4 pathway mediates spinal hypersensitivity in a mouse model of vertebral endplate degeneration. Oxid Med Cell Longev. (2021) 2021:9965737. doi: 10.1155/2021/9965737
66. Bian Q, Ma L, Jain A, Crane JL, Kebaish K, Wan M, et al. Mechanosignaling activation of TGFbeta maintains intervertebral disc homeostasis. Bone Res. (2017) 5:17008. doi: 10.1038/boneres.2017.8
67. Bian Q, Jain A, Xu X, Kebaish K, Crane JL, Zhang Z, et al. Excessive activation of TGFbeta by spinal instability causes vertebral endplate sclerosis. Sci Rep. (2016) 6:27093. doi: 10.1038/srep27093
68. Lonner BS, Toombs CS, Mechlin M, Ciavarra G, Shah SA, Samdani AF, et al. MRI Screening in operative scheuermann kyphosis: is it necessary? Spine Deform. (2017) 5:124–33. doi: 10.1016/j.jspd.2016.10.008
69. Lonner BS, Ren Y, Upasani VV, Marks MM, Newton PO, Samdani AF, et al. Disc degeneration in unfused caudal motion segments ten years following surgery for adolescent idiopathic scoliosis. Spine Deform. (2018) 6:684–90. doi: 10.1016/j.jspd.2018.03.013
70. Sakai D, Nishimura K, Tanaka M, Nakajima D, Grad S, Alini M, et al. Migration of bone marrow-derived cells for endogenous repair in a new tail-looping disc degeneration model in the mouse: a pilot study. Spine J. (2015) 15:1356–65. doi: 10.1016/j.spinee.2013.07.491
71. Nakamichi R, Ito Y, Inui M, Onizuka N, Kayama T, Kataoka K, et al. Mohawk promotes the maintenance and regeneration of the outer annulus fibrosus of intervertebral discs. Nat Commun. (2016) 7:12503. doi: 10.1038/ncomms12503
72. Huang Y, Zhang Z, Wang J, Shen S, Yao T, Xu Y, et al. circSPG21 protects against intervertebral disc disease by targeting miR-1197/ATP1B3. Exp Mol Med. (2021) 53:1547–58. doi: 10.1038/s12276-021-00674-z
73. Stokes IA, McBride C, Aronsson DD, Roughley PJ. Intervertebral disc changes with angulation, compression and reduced mobility simulating altered mechanical environment in scoliosis. Eur Spine J. (2011) 20:1735–44. doi: 10.1007/s00586-011-1868-5
74. MacLean JJ, Lee CR, Grad S, Ito K, Alini M, Iatridis JC. Effects of immobilization and dynamic compression on intervertebral disc cell gene expression in vivo. Spine. (2003) 28:973–81. doi: 10.1097/01.BRS.0000061985.15849.A9
75. Stokes IA, McBride CA, Aronsson DD, Roughley PJ. Metabolic effects of angulation, compression, and reduced mobility on Annulus fibrosis in a model of altered mechanical environment in scoliosis. Spine Deform. (2013) 1:161–70. doi: 10.1016/j.jspd.2013.02.001
76. Hirata H, Yurube T, Kakutani K, Maeno K, Takada T, Yamamoto J, et al. A rat tail temporary static compression model reproduces different stages of intervertebral disc degeneration with decreased notochordal cell phenotype. J Orthop Res. (2014) 32:455–63. doi: 10.1002/jor.22533
77. He R, Wang Z, Cui M, Liu S, Wu W, Chen M, et al. HIF1A Alleviates compression-induced apoptosis of nucleus pulposus derived stem cells via upregulating autophagy. Autophagy. (2021) 17:3338–60. doi: 10.1080/15548627.2021.1872227
78. Chen S, Wu X, Lai Y, Chen D, Bai X, Liu S, et al. Kindlin-2 inhibits Nlrp3 inflammasome activation in nucleus pulposus to maintain homeostasis of the intervertebral disc. Bone Res. (2022) 10:5. doi: 10.1038/s41413-021-00179-5
79. Ji Y, Zhu P, Zhang L, Yang H. A novel rat tail disc degeneration model induced by static bending and compression. Animal Model Exp Med. (2021) 4:261–7. doi: 10.1002/ame2.12178
80. Miyagi M, Ishikawa T, Kamoda H, Suzuki M, Murakami K, Shibayama M, et al. ISSLS Prize winner: disc dynamic compression in rats produces long-lasting increases in inflammatory mediators in discs and induces long-lasting nerve injury and regeneration of the afferent fibers innervating discs: a pathomechanism for chronic discogenic low back pain. Spine. (2012) 37:1810–8. doi: 10.1097/BRS.0b013e31824ffac6
81. Krock E, Rosenzweig DH, Chabot-Dore AJ, Jarzem P, Weber MH, Ouellet JA, et al. Painful, degenerating intervertebral discs up-regulate neurite sprouting and CGRP through nociceptive factors. J Cell Mol Med. (2014) 18:1213–25. doi: 10.1111/jcmm.12268
82. Ohtori S, Miyagi M, Inoue G. Sensory nerve ingrowth, cytokines, and instability of discogenic low back pain: a review. Spine Surg Relat Res. (2018) 2:11–7. doi: 10.22603/ssrr.2016-0018
83. Verschueren SM, Roelants M, Delecluse C, Swinnen S, Vanderschueren D, Boonen S. Effect of 6-month whole body vibration training on hip density, muscle strength, and postural control in postmenopausal women: a randomized controlled pilot study. J Bone Miner Res. (2004) 19:352–9. doi: 10.1359/JBMR.0301245
84. Wang XQ, Gu W, Chen BL, Wang X, Hu HY, Zheng YL, et al. Effects of whole-body vibration exercise for non-specific chronic low back pain: an assessor-blind, randomized controlled trial. Clin Rehabil. (2019) 33:1445–57. doi: 10.1177/0269215519848076
85. Kuisma M, Karppinen J, Haapea M, Niinimaki J, Ojala R, Heliovaara M, et al. Are the determinants of vertebral endplate changes and severe disc degeneration in the lumbar spine the same? A magnetic resonance imaging study in middle-aged male workers. BMC Musculoskelet Disord. (2008) 9:51. doi: 10.1186/1471-2474-9-51
86. Yamazaki S, Banes AJ, Weinhold PS, Tsuzaki M, Kawakami M, Minchew JT. Vibratory loading decreases extracellular matrix and matrix metalloproteinase gene expression in rabbit annulus cells. Spine J. (2002) 2:415–20. doi: 10.1016/S1529-9430(02)00427-8
87. McCann MR, Patel P, Pest MA, Ratneswaran A, Lalli G, Beaucage KL, et al. Repeated exposure to high-frequency low-amplitude vibration induces degeneration of murine intervertebral discs and knee joints. Arthritis Rheumatol. (2015) 67:2164–75. doi: 10.1002/art.39154
88. McCann MR, Veras MA, Yeung C, Lalli G, Patel P, Leitch KM, et al. Whole-body vibration of mice induces progressive degeneration of intervertebral discs associated with increased expression of il-1beta and multiple matrix degrading enzymes. Osteoarthritis Cartilage. (2017) 25:779–89. doi: 10.1016/j.joca.2017.01.004
89. Zeeman ME, Kartha S, Jaumard NV, Baig HA, Stablow AM, Lee J, et al. Whole-body vibration at thoracic resonance induces sustained pain and widespread cervical neuroinflammation in the rat. Clin Orthop Relat Res. (2015) 473:2936–47. doi: 10.1007/s11999-015-4315-9
90. Patterson F, Miralami R, Tansey KE, Prabhu RK, Priddy LB. Deleterious effects of whole-body vibration on the spine: a review of in vivo, ex vivo, and in vitro models. Animal Model Exp Med. (2021) 4:77–86. doi: 10.1002/ame2.12163
91. Yao W, Jee WS, Chen J, Liu H, Tam CS, Cui L, et al. Making rats rise to erect bipedal stance for feeding partially prevented orchidectomy-induced bone loss and added bone to intact rats. J Bone Miner Res. (2000) 15:1158–68. doi: 10.1359/jbmr.2000.15.6.1158
92. Liang QQ, Cui XJ, Xi ZJ, Bian Q, Hou W, Zhao YJ, et al. Prolonged upright posture induces degenerative changes in intervertebral discs of rat cervical spine. Spine. (2011) 36:E14–9. doi: 10.1097/BRS.0b013e3181d2dec2
93. Liu S, Zhao B, Shi H, Liang Q, Fu Y, Yang Z, et al. Ligustrazine inhibits cartilage endplate hypertrophy via suppression of TGF-beta1. Evid Based Complement Alternat Med. (2016) 2016:1042489. doi: 10.1155/2016/1042489
94. Kong M, Zhang Y, Song M, Cong W, Gao C, Zhang J, et al. Myocardinrelated transcription factor A nuclear translocation contributes to mechanical overloadinduced nucleus pulposus fibrosis in rats with intervertebral disc degeneration. Int J Mol Med. (2021) 96:48(1):123. doi: 10.3892/ijmm.2021.4956
95. Liang X, Shen H, Shi WD, Ren S, Jiang W, Liu H, et al. Effect of axial vertical vibration on degeneration of lumbar intervertebral discs in modified bipedal rats: an in-vivo study. Asian Pac J Trop Med. (2017) 10:714–7. doi: 10.1016/j.apjtm.2017.07.014
96. Ao X, Wang L, Shao Y, Chen X, Zhang J, Chu J, et al. Development and characterization of a novel bipedal standing mouse model of intervertebral disc and facet joint degeneration. Clin Orthop Relat Res. (2019) 477:1492–504. doi: 10.1097/CORR.0000000000000712
97. Lao YJ, Xu TT, Jin HT, Ruan HF, Wang JT, Zhou L, et al. Accumulated spinal axial biomechanical loading induces degeneration in intervertebral disc of mice lumbar spine. Orthop Surg. (2018) 10:56–63. doi: 10.1111/os.12365
98. Lee S, Jang SH, Suzuki-Narita M, Gregoire S, Millecamps M, Stone LS. Voluntary running attenuates behavioural signs of low back pain: dimorphic regulation of intervertebral disc inflammation in male and female SPARC-null mice. Osteoarthritis Cartilage. (2022) 30:110–23. doi: 10.1016/j.joca.2021.06.014
99. Gruber HE, Sage EH, Norton HJ, Funk S, Ingram J, Hanley EJ. Targeted deletion of the SPARC gene accelerates disc degeneration in the aging mouse. J Histochem Cytochem. (2005) 53:1131–8. doi: 10.1369/jhc.5A6687.2005
100. Millecamps M, Czerminski JT, Mathieu AP, Stone LS. Behavioral signs of axial low back pain and motor impairment correlate with the severity of intervertebral disc degeneration in a mouse model. Spine J. (2015) 15:2524–37. doi: 10.1016/j.spinee.2015.08.055
101. Millecamps M, Tajerian M, Naso L, Sage HE, Stone LS. Lumbar intervertebral disc degeneration associated with axial and radiating low back pain in ageing SPARC-null mice. Pain. (2012) 153:1167–79. doi: 10.1016/j.pain.2012.01.027
102. Krock E, Millecamps M, Anderson KM, Srivastava A, Reihsen TE, Hari P, et al. Interleukin-8 as a therapeutic target for chronic low back pain: upregulation in human cerebrospinal fluid and pre-clinical validation with chronic reparixin in the SPARC-null mouse model. Ebiomedicine. (2019) 43:487–500. doi: 10.1016/j.ebiom.2019.04.032
103. Rai MF, Hashimoto S, Johnson EE, Janiszak KL, Fitzgerald J, Heber-Katz E, et al. Heritability of articular cartilage regeneration and its association with ear wound healing in mice. Arthritis Rheum. (2012) 64:2300–10. doi: 10.1002/art.34396
104. Choi H, Tessier S, Silagi ES, Kyada R, Yousefi F, Pleshko N, et al. A novel mouse model of intervertebral disc degeneration shows altered cell fate and matrix homeostasis. Matrix Biol. (2018) 70:102–22. doi: 10.1016/j.matbio.2018.03.019
105. Zhang Y, Xiong C, Kudelko M, Li Y, Wang C, Wong YL, et al. Early onset of disc degeneration in SM/J mice is associated with changes in ion transport systems and fibrotic events. Matrix Biol. (2018) 70:123–39. doi: 10.1016/j.matbio.2018.03.024
106. Novais EJ, Tran VA, Miao J, Slaver K, Sinensky A, Dyment NA, et al. Comparison of inbred mouse strains shows diverse phenotypic outcomes of intervertebral disc aging. Aging Cell. (2020) 19:e13148. doi: 10.1111/acel.13148
107. Aszodi A, Chan D, Hunziker E, Bateman JF, Fassler R. Collagen II is essential for the removal of the notochord and the formation of intervertebral discs. J Cell Biol. (1998) 143:1399–412. doi: 10.1083/jcb.143.5.1399
108. Sahlman J, Inkinen R, Hirvonen T, Lammi MJ, Lammi PE, Nieminen J, et al. Premature vertebral endplate ossification and mild disc degeneration in mice after inactivation of one allele belonging to the Col2a1 gene for type II collagen. Spine. (2001) 26:2558–65. doi: 10.1097/00007632-200112010-00008
109. Xu H, Dong R, Zeng Q, Fang L, Ge Q, Xia C, et al. Col9a2 gene deletion accelerates the degeneration of intervertebral discs. Exp Ther Med. (2022) 23:207. doi: 10.3892/etm.2022.11130
110. Boyd LM, Richardson WJ, Allen KD, Flahiff C, Jing L, Li Y, et al. Early-onset degeneration of the intervertebral disc and vertebral end plate in mice deficient in type IX collagen. Arthritis Rheum. (2008) 58:164–71. doi: 10.1002/art.23231
111. Li CG, Liang QQ, Zhou Q, Menga E, Cui XJ, Shu B, et al. A continuous observation of the degenerative process in the intervertebral disc of Smad3 gene knock-out mice. Spine. (2009) 34:1363–9. doi: 10.1097/BRS.0b013e3181a3c7c7
112. Phillips KL, Jordan-Mahy N, Nicklin MJ, Le Maitre CL. Interleukin-1 receptor antagonist deficient mice provide insights into pathogenesis of human intervertebral disc degeneration. Ann Rheum Dis. (2013) 72:1860–7. doi: 10.1136/annrheumdis-2012-202266
113. Wu WJ, Zhang XK, Zheng XF, Yang YH, Jiang SD, Jiang LS. SHH-dependent knockout of HIF-1 alpha accelerates the degenerative process in mouse intervertebral disc. Int J Immunopathol Pharmacol. (2013) 26:601–9. doi: 10.1177/039463201302600304
114. Beierfuss A, Dietrich H, Kremser C, Hunjadi M, Ritsch A, Rulicke T, et al. Knockout of apolipoprotein E in rabbit promotes premature intervertebral disc degeneration: a new in vivo model for therapeutic approaches of spinal disc disorders. Plos One. (2017) 12:e187564. doi: 10.1371/journal.pone.0187564
115. Whittal MC, Molladavoodi S, Zwambag DP, Millecamps M, Stone LS, Gregory DE. Mechanical consequence of induced intervertebral disc degeneration in the SPARC-null mouse. J Biomech Eng. (2021) 143(2):024501. doi: 10.1115/1.4047995
116. Dudek M, Yang N, Ruckshanthi JP, Williams J, Borysiewicz E, Wang P, et al. The intervertebral disc contains intrinsic circadian clocks that are regulated by age and cytokines and linked to degeneration. Ann Rheum Dis. (2017) 76:576–84. doi: 10.1136/annrheumdis-2016-209428
117. Semba K, Araki K, Li Z, Matsumoto K, Suzuki M, Nakagata N, et al. A novel murine gene, sickle tail, linked to the Danforth's Short tail locus, is required for normal development of the intervertebral disc. Genetics. (2006) 172:445–56. doi: 10.1534/genetics.105.048934
118. Kamper M, Hamann N, Prein C, Clausen-Schaumann H, Farkas Z, Aszodi A, et al. Early changes in morphology, bone mineral density and matrix composition of vertebrae lead to disc degeneration in aged collagen IX -/- mice. Matrix Biol. (2016) 49:132–43. doi: 10.1016/j.matbio.2015.09.005
119. Tessier S, Tran VA, Ottone OK, Novais EJ, Doolittle A, DiMuzio MJ, et al. TonEBP-deficiency accelerates intervertebral disc degeneration underscored by matrix remodeling, cytoskeletal rearrangements, and changes in proinflammatory gene expression. Matrix Biol. (2020) 87:94–111. doi: 10.1016/j.matbio.2019.10.007
120. Vo N, Seo HY, Robinson A, Sowa G, Bentley D, Taylor L, et al. Accelerated aging of intervertebral discs in a mouse model of progeria. J Orthop Res. (2010) 28:1600–7. doi: 10.1002/jor.21153
121. Gorth DJ, Shapiro IM, Risbud MV. A new understanding of the role of IL-1 in age-related intervertebral disc degeneration in a murine model. J Bone Miner Res. (2019) 34:1531–42. doi: 10.1002/jbmr.3714
122. Silagi ES, Novais EJ, Bisetto S, Telonis AG, Snuggs J, Le Maitre CL, et al. Lactate efflux from intervertebral disc cells is required for maintenance of spine health. J Bone Miner Res. (2020) 35:550–70. doi: 10.1002/jbmr.3908
123. Tsingas M, Ottone OK, Haseeb A, Barve RA, Shapiro IM, Lefebvre V, et al. Sox9 deletion causes severe intervertebral disc degeneration characterized by apoptosis, matrix remodeling, and compartment-specific transcriptomic changes. Matrix Biol. (2020) 94:110–33. doi: 10.1016/j.matbio.2020.09.003
124. Alkhatib B, Liu C, Serra R. Tgfbr2 is required in acan-expressing cells for maintenance of the intervertebral and sternocostal joints. JOR Spine. (2018) 1(2):e1025. doi: 10.1002/jsp2.1025
125. Bedore J, Sha W, McCann MR, Liu S, Leask A, Seguin CA. Impaired intervertebral disc development and premature disc degeneration in mice with notochord-specific deletion of CCN2. Arthritis Rheum. (2013) 65:2634–44. doi: 10.1002/art.38075
126. Alvarez-Garcia O, Matsuzaki T, Olmer M, Miyata K, Mokuda S, Sakai D, et al. FOXO Are required for intervertebral disk homeostasis during aging and their deficiency promotes disk degeneration. Aging Cell. (2018) 17:e12800. doi: 10.1111/acel.12800
127. Silberberg R. Histologic and morphometric observations on vertebral bone of aging sand rats. Spine. (1988) 13:202–8. doi: 10.1097/00007632-198802000-00013
128. Gruber HE, Phillips R, Ingram JA, Norton HJ, Hanley EJ. Spontaneous age-related cervical disc degeneration in the sand rat. Clin Orthop Relat Res. (2014) 472:1936–42. doi: 10.1007/s11999-014-3497-x
129. Marfia G, Campanella R, Navone SE, Zucca I, Scotti A, Figini M, et al. Potential use of human adipose mesenchymal stromal cells for intervertebral disc regeneration: a preliminary study on biglycan-deficient murine model of chronic disc degeneration. Arthritis Res Ther. (2014) 16:457. doi: 10.1186/s13075-014-0457-5
130. Ohnishi T, Sudo H, Tsujimoto T, Iwasaki N. Age-related spontaneous lumbar intervertebral disc degeneration in a mouse model. J Orthop Res. (2018) 36:224–32. doi: 10.1002/jor.23634
131. Silva MJ, Holguin N. Aging aggravates intervertebral disc degeneration by regulating transcription factors toward chondrogenesis. Faseb J. (2020) 34:1970–82. doi: 10.1096/fj.201902109R
132. Lin D, Alberton P, Delgado CM, Prein C, Clausen-Schaumann H, Dong J, et al. Loss of tenomodulin expression is a risk factor for age-related intervertebral disc degeneration. Aging Cell. (2020) 19:e13091. doi: 10.1111/acel.13091
133. Novais EJ, Tran VA, Johnston SN, Darris KR, Roupas AJ, Sessions GA, et al. Long-term treatment with senolytic drugs dasatinib and quercetin ameliorates age-dependent intervertebral disc degeneration in mice. Nat Commun. (2021) 12:5213. doi: 10.1038/s41467-021-25453-2
134. Brinjikji W, Diehn FE, Jarvik JG, Carr CM, Kallmes DF, Murad MH, et al. MRI Findings of disc degeneration are more prevalent in adults with low back pain than in asymptomatic controls: a systematic review and meta-analysis. AJNR Am J Neuroradiol. (2015) 36:2394–9. doi: 10.3174/ajnr.A4498
135. Gan Y, He J, Zhu J, Xu Z, Wang Z, Yan J, et al. Spatially defined single-cell transcriptional profiling characterizes diverse chondrocyte subtypes and nucleus pulposus progenitors in human intervertebral discs. Bone Res. (2021) 9:37. doi: 10.1038/s41413-021-00163-z
136. Gao B, Jiang B, Xing W, Xie Z, Luo Z, Zou W. Discovery and application of postnatal nucleus Pulposus progenitors essential for intervertebral disc homeostasis and degeneration. Adv Sci (Weinh). (2022) 9(13):e2104888. doi: 10.1002/advs.202104888
137. Han S, Zhang Y, Zhang X, Zhang H, Meng S, Kong M, et al. Single-cell RNA sequencing of the nucleus pulposus reveals chondrocyte differentiation and regulation in intervertebral disc degeneration. Front Cell Dev Biol. (2022) 10:824771. doi: 10.3389/fcell.2022.824771
138. Zhang Y, Han S, Kong M, Tu Q, Zhang L, Ma X. Single-cell RNA-seq analysis identifies unique chondrocyte subsets and reveals involvement of ferroptosis in human intervertebral disc degeneration. Osteoarthritis Cartilage. (2021) 29:1324–34. doi: 10.1016/j.joca.2021.06.010
Keywords: intervertebral disc degeneration, nucleus pulposus, intervertebral disc, animal model, surgery technique, orthopedics surgery
Citation: Liang T, Gao B, Zhou J, Qiu X, Qiu J, Chen T, Liang Y, Gao W, Qiu X and Lin Y (2023) Constructing intervertebral disc degeneration animal model: A review of current models. Front. Surg. 9:1089244. doi: 10.3389/fsurg.2022.1089244
Received: 4 November 2022; Accepted: 8 December 2022;
Published: 10 March 2023.
Edited by:
Xiaolong Chen, University of New South Wales, AustraliaReviewed by:
Jinqian Liang, Peking Union Medical College Hospital (CAMS), China© 2023 Liang, Gao, Zhou, Qiu, Qiu, Chen, Liang, Gao, Qiu and Lin. This is an open-access article distributed under the terms of the Creative Commons Attribution License (CC BY). The use, distribution or reproduction in other forums is permitted, provided the original author(s) and the copyright owner(s) are credited and that the original publication in this journal is cited, in accordance with accepted academic practice. No use, distribution or reproduction is permitted which does not comply with these terms.
*Correspondence: Xuemei Qiu cWlxaTAyMTRAMTI2LmNvbQ== Youxi Lin bGlueXg5OUBtYWlsLnN5c3UuZWR1LmNu
†These authors have contributed equally to this work
Specialty Section: This article was submitted to Orthopedic Surgery, a section of the journal Frontiers in Surgery
Disclaimer: All claims expressed in this article are solely those of the authors and do not necessarily represent those of their affiliated organizations, or those of the publisher, the editors and the reviewers. Any product that may be evaluated in this article or claim that may be made by its manufacturer is not guaranteed or endorsed by the publisher.
Research integrity at Frontiers
Learn more about the work of our research integrity team to safeguard the quality of each article we publish.