- Centre for Environmental and Climate Science, Faculty of Science, Lund University, Lund, Sweden
Bioenergy is perceived to play a vital role in climate mitigation, transition to renewable energy consumption, energy security, and local and rural socio-economic development. However, exploiting renewable bioenergy resources may need to be more sustainable in the current predominant paradigm. In this study, we raise two broad research questions: (1) what are the significant challenges to the current global bioenergy production and consumption system, and (2) what are the opportunities for a sustainable and circular bioenergy system? We qualitatively analyzed how the current bioenergy production and consumption system results in unintended negative consequences. Taking the example of biofuels, this research exemplifies some critical systemic flaws in how bioenergy is currently utilized in the transportation sector. We do this by broadening the system boundaries to identify the social, economic, and environmental consequences often distant in time and space. We conducted semi-structured interviews, workshops, and literature studies to gather data on the significant bioenergy production and consumption drivers, socio-economic factors, and ecological impacts. The causal loop diagram technique illustrates this broader system's systemic cause-effect and feedback relationships. In the current system of bioenergy production and consumption, negative socio-economic and ecological consequences limit the potential of exploiting bioenergy for climate mitigation. Firstly, bioenergy is neither carbon neutral nor renewable from a broader systems perspective, given that biomass cultivation, feedstock refining, and processing are closely coupled with natural resource use (e.g., water, energy, chemicals, and fertilizers) and other nutrient cycles (e.g., nitrogen, and phosphorus). Secondly, large-scale bioenergy developments negatively impact food security, land use change, ecosystem services, and biodiversity in certain regions. Thirdly, the current globalized bioenergy economy is fundamentally unsustainable due to the displacement of bioenergy production's negative social and ecological impacts from consumer to producer regions. We identify and discuss the critical system interventions to be placed throughout the system as significant leverages for managing the unintended negative consequences of the present dominant bioenergy production and consumption regimes.
1 Introduction
1.1 Sustainability implications of bioenergy
Increasing energy consumption globally drives carbon emissions and contributes to climate change (Tilman et al., 2009; Péreau et al., 2012; Hammond and Li, 2016; Wang et al., 2017). To address this sustainability challenge, bioenergy is recognized as an essential short- to medium-term solution for addressing climate mitigation by replacing fossil energy and contributing to sustainable development (Blair et al., 2021). The International Energy Agency (2023a) forecasts an expansion of biofuel demand from 22% over 2022–2027 to 35,000 million liters per year, saving significant annual carbon dioxide emissions compared to the continuous utilization of petroleum-based fossil fuels. Further, bioenergy provides added benefits, such as pollution reduction (Wang et al., 2017), energy security, diversification of the energy supply mix, and local economic development through employment and infrastructure development in selected sectors (Humpenöder et al., 2018; Qaim et al., 2020; Sibhatu, 2023).
However, bioenergy production and consumption are only partially carbon-neutral and sustainable from a broader systems perspective, as the production (and consumption) processes of bioenergy require significant resource inputs. For instance, land-based bioenergy cultivation is closely linked to local, regional, and global nutrient cycles, the use of non-renewable fossil resources (e.g., phosphorus), and agricultural inputs impacting ecosystem services and biodiversity (Wang et al., 2017). Indeed, resource inputs and life cycle emissions needed to cultivate biomass, process feedstocks, and transport bioenergy offset (partial or complete) the positive benefits of bioenergy (Staples et al., 2017; Humpenöder et al., 2018). Further, rapid economic growth and urbanization have intensified demand for land, land-use competition, and negative impacts on food security, ecosystem services, and biodiversity (Tilman et al., 2009; Witcover et al., 2013; Essl et al., 2018; Humpenöder et al., 2018). Furthermore, the globalized bioenergy value chains have displaced the bioenergy production's negative socioeconomic and ecological impacts on other regions (Brose et al., 2010; Brinkman et al., 2019). Indeed, the bioenergy policymaking regimes have shaped the global energy sectors and geopolitical landscapes. With the current global socio-economic trends, global energy consumption is expected to increase nearly 50% compared with 2020 levels by 2050 (The U.S. Energy Information Administration, 2023). Without impeding on competing land uses, the relative contribution of utilizing land (agriculture or forest) resources for bioenergy purposes will likely be very limited (Wang et al., 2017). Therefore, the broad sustainability challenges linked to bioenergy production and consumption need to be scrutinized to avoid any unintended negative social, economic, and ecological consequences.
1.2 Systems thinking and policymaking
In today's globalized world, sustainability problems facing policymakers are interconnected, ill-defined, and complex. These problems are acknowledged in policymaking as uncertain, unpredictable, ill-structured, or wicked (Bali, 2021). Despite this, the current global policymaking regimes are dominated by reductionist thinking, a linear thinking approach [also called “open loop thinking” (Sterman, 2000)] that addresses these problems in silos (Head, 2019; Hynes et al., 2020; Mueller, 2020; Bali, 2021; Nel and Taeihagh, 2024). Such an approach to policymaking focuses on immediate cause-and-effect, quick-fix decision-making, and short-term outcomes (Sterman, 2000; Ollhoff and Walcheski, 2002). It is prone to failure because it fails to understand the interconnected, multidimensional, and complex nature of these problems (Hynes et al., 2020). This often results in unintended negative social, economic, and environmental consequences of the intended interventions in the system (Sterman, 2000; Head, 2019; Mueller, 2020; Bali, 2021). On the other hand, systems thinking focuses on a holistic understanding of the problem to develop strategic and longer-lasting solutions.
Systems thinking could assist policymaking by offering tools to disaggregate, understand, and act on connected systemic issues while accounting for their critical linkages (Sterman, 2000, 2012; Meadows, 2008; Hynes et al., 2020). It is part of the complexity science-informed approaches such as systems theory, cybernetics, and complexity theory (Sterman, 2000; Nel and Taeihagh, 2024). It focuses on the system rather than its parts to approach complex problems (Sweeney and Meadows, 2010) to understand their interrelationships and underlying dynamics. Thus, it is concerned with understanding the system's behavior, dynamics, and critical patterns to minimize or eliminate the unintended negative consequences of intended interventions (Sterman, 2000; Sweeney and Meadows, 2010).
Major global economies have introduced several measures to enhance the production, trade, and consumption of bioenergy. For example, the United States (Jeffers et al., 2013; Dumortier et al., 2021; Lark et al., 2022; Newes et al., 2022), the European Union member states (Timilsina, 2014; Debnath and Whistance, 2023), India (Sinha et al., 2019) and China (Zhang and Feng, 2021). Nonetheless, these regimes have an isolated focus on problems related to energy security, climate change, socioeconomic development, and geopolitics rather than a holistic approach to achieving sustainability. This is why, apart from the anticipated positive benefits of bioenergy production and consumption to society, it has also caused unintended negative socioeconomic (Vandergeten et al., 2016) and ecological consequences for certain global regions and communities (Butchart et al., 2010; Tudge et al., 2021; Merfort et al., 2023). This study adopts a systems thinking approach to conceptualize the sustainability challenges to the current bioenergy production and consumption and propose places for policy intervention to address these challenges.
1.3 Transition toward a sustainable and just bioeconomy
The concept of “bioeconomy” has no common definition despite its global adoption in policy strategies (IACGB - International Advisory Council on Global Bioeconomy, 2020). The term “bioeconomy” or “bioeconomics” was first coined by Nicholas Georgescu-Roegen to describe a new economy with a purpose (Georgescu-Roegen, 1971). It was “to conserve resources and to obtain a rational control over the development and use of technologies in order for it to serve the true human wants—and not rising profits, warfare, or national prestige. …a worldwide economy that is predicated on justice and that allows for the wealth of the earth to be shared equally among its inhabitants now and in the future (translation by Vogelpohl and Töller, 2021, p. 143). This description of bioeconomy points to the normative principles of resource conservation, sustainable (de)growth, and inter- and intra-generational justice.
In the past 115 years, the global societal metabolism of materials, energy, and water has increased eight to 12-fold due to rapid population increase, urbanization, and industrialization (Haberl et al., 2019). This has contributed to human-driven climate change (Haberl et al., 2019; Ciobanu and Onofrei, 2021), planetary boundaries' violation (Steffen et al., 2015), and societal injustice (Martinez Alier, 1995; Terwilliger, 2023). Globally, over 3,300 ecological distribution conflicts are recorded on The EJAtlas (Martinez-Alier, 2021) (https://ejatlas.org/). There are no signs of global societal metabolism stabilizing soon, new acceleration is expected due to growth in the developing economies (Haberl et al., 2019; Ciobanu and Onofrei, 2021). The current predominant narratives of bioeconomy are centered around anthropocentric values of economic growth and technological innovation, ignoring the dimensions of social equity and justice (Giuntoli et al., 2023). The transition toward sustainable bioenergy requires acknowledging and operationalizing the underlying normative principles of Georgescu-Roegen's bioeconomy. Such a bioeconomy does not seek sub-optimized solutions focusing on a single product or value chain (e.g., biofuels), and expansion of production or consumption. Based on sufficiency, it rather operates within the ecological limits of production and addresses the socioeconomic externalities throughout the value chain. It avoids shifting socioeconomic externalities of production and consumption to other spatial or temporal scales by establishing regional and global governance mechanisms. There is a research gap in mapping the current system and assessing the challenges that need to be overcome in order to approach this “ideal bioeconomy.”
1.4 Aim and objectives of the study
This study aims to analyze the global sustainability challenges to the current system of bioenergy production and consumption and explore the system leverages to address these challenges. To achieve this aim, the key objectives are to:
1. Identify and highlight the significant challenges in the broader system of bioenergy production and consumption in a global sustainability perspective.
2. Integrate the main systems variables and cause-effect relationships in a causal loop diagram.
3. Explore and integrate key causal relationships and feedback loops to examine the main synergies and conflicts in the current system.
4. Discuss the places for policy interventions to address the sustainability impacts of bioenergy production and consumption.
To achieve this, we adopt a stepwise methodology (Section 2) to present qualitative system models of the major societal drivers behind the global bioenergy economy, its socio-economic and ecological consequences (Section 3), and discuss the need for a sustainable and just bioeconomy (Section 4).
2 Methodology
This research approaches the complex issue of sustainable production and consumption of bioenergy, a post-normal problem with uncertain facts, disputed values across different stakeholders, and high stakes needing urgent decisions (Funtowicz and Ravetz, 1993). To explore the challenges to sustainable bioenergy development and identify policy interventions for leveraging the bioenergy production and consumption system in the context of climate mitigation, we expand the system boundaries of the bioenergy production and consumption system. We utilize a driver-pressure-impact-state-impact-response (DPSIR) framework (Ness et al., 2010) and group model building (Vennix, 1996, 1999) to map the diverse socio-economic interactions between the human activity system and the natural systems (see Figure 1). By human activity system, we mean the socio-technical, political, and economic system associated with the production and consumption of bioenergy. The natural systems consist of ecosystems and environmental systems. The overall drivers of natural resource consumption in society are rapid urbanization, population growth, and socioeconomic development. These drivers lead to pressures in the human systems, such as energy demand and land use (change). These pressures then change the state of the natural systems, e.g., carbon, water and nutrient cycles, atmospheric emissions, and wastes. This altered state of the natural systems has impacts on both the natural (e.g., climate impacts, biodiversity loss, soil depletion, etc.) and human systems (e.g., human development, ecosystem services, etc.). The DPSIR framework was used to conceptualize the cause-and-effect relationships among variables in the system.
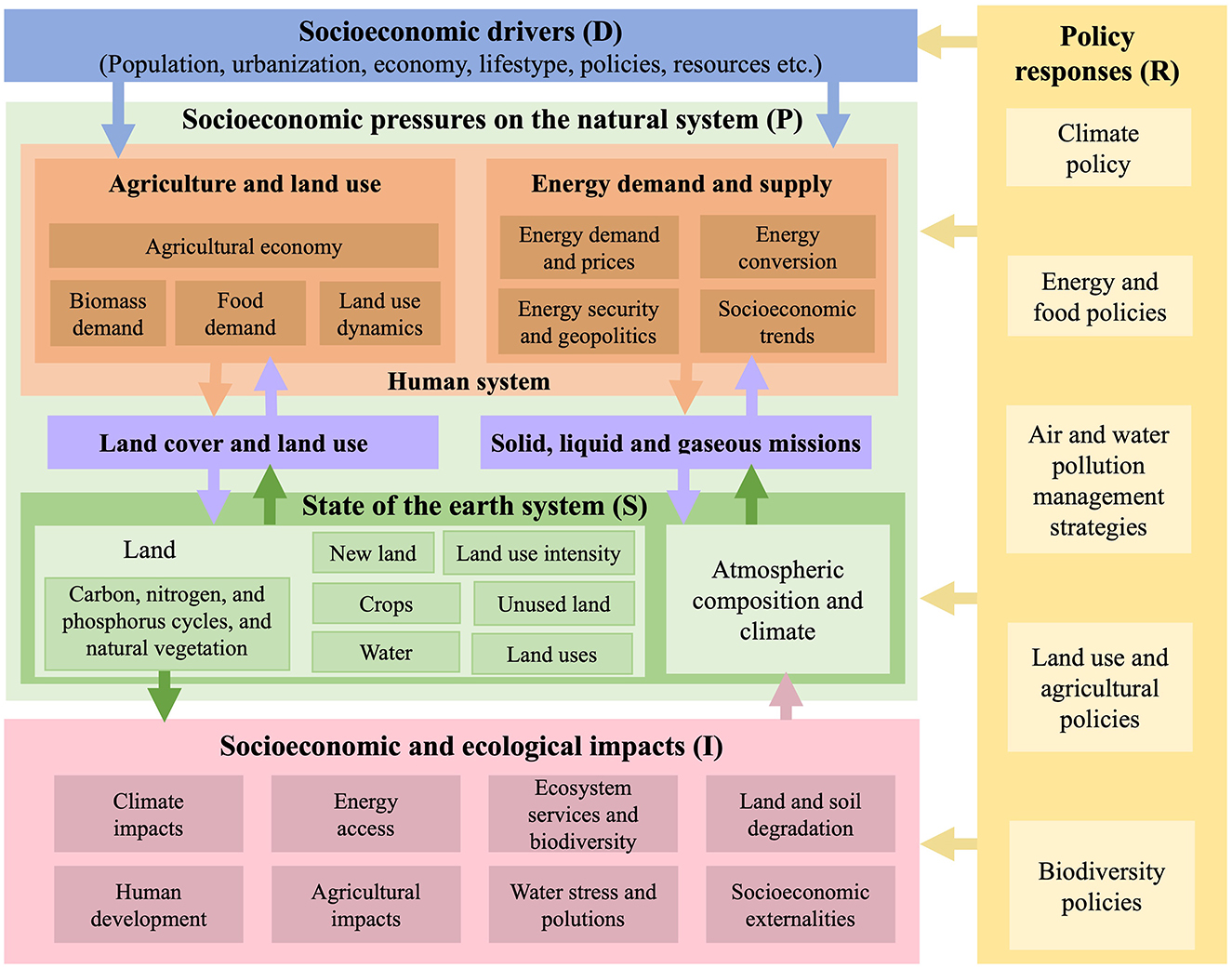
Figure 1. D-P-S-I-R framework to structure the cause-and-effect interrelationships among the key variables in biofuel production and consumption system. Adapted from the IMAGE 3.0 (Integrated Model to Assess the Global Environment) developed by PBL Netherlands Environmental Assessment Agency (https://models.pbl.nl/image/index.php/IMAGE_framework).
2.1 Research methods
The methodology employed includes several steps (Figure 2). In the first step, we used Critical interpretive synthesis (Dixon-Woods et al., 2006) to synthesize multi-disciplinary data from the literature on the social, economic, and ecological impacts of bioenergy production and consumption. In the second step, we conducted semi-structured interviews with experts to explore the more pragmatic stance on the current system of bioenergy production and consumption. In the third step, the quantitative and qualitative inferences from the prior two steps are discussed in (Step 3) group brainstorming sessions. This led to the fourth step, the development of a causal loop diagram (CLD) representing the key causal relationships and feedback loops in the system (Step 4) to examine the main synergies and conflicts for a sustainable bioeconomy. We adopt a life cycle thinking approach to address the broad research questions by including the direct and indirect impacts (i.e., the scope 1, 2, and 3 emissions and other social and economic externalities) of bioenergy production and consumption from a global perspective.
2.1.1 Critical interpretive synthesis (Step 1)
Critical interpretive synthesis (Dixon-Woods et al., 2006) is an approach to synthesizing multidisciplinary and multi-method evidence. In contrast to a traditional literature review, in critical interpretive synthesis, the review questions are not precisely specified at the outset of the review but are iteratively revised during the review and analysis process (Dixon-Woods et al., 2006). It explicitly allows the integration of qualitative and quantitative inferences or evidence. In this research, we utilized critical interpretive synthesis to identify and support key cause-and-effect interactions in the systems of bioenergy production and consumption. Appendix 1 provides an overview of the key research themes synthesized and the cause-and-effect interactions identified during this synthesis.
2.1.2 Semi-structured interviews with experts (Step 2)
Semi-structured interviews are conducted with experts from academia, research institutions, and industry working on bioenergy-related issues in Sweden. It included eight academic and two industrial experts (further details are provided in Appendix 2). The academic experts had backgrounds in social sciences, landscape ecology, energy policy, agricultural science, industrial ecology, environmental science, and biology. The industrial expert worked in managerial roles in organizations with bioenergy refining, storage, and distribution operations in Sweden. These interviews were conducted to get an overview of the socioeconomic drivers and environmental impacts of the bioenergy economy in society (see Appendix 3 for a list of interview questions). The interviews were transcribed and coded using NVivo software (https://lumivero.com/products/nvivo/) to identify the key cause-and-effect variables related to bioenergy production, trade, consumption, and subsequent socioeconomic and ecological impacts. These interviews complemented the cause-and-effect interactions identified in Step 1 and informed the critical interpretive synthesis.
2.1.3 Group brainstorming sessions (Step 3)
The qualitative and quantitative inferences obtained during Steps 1 and 2 were employed to further refine the identified system variables and their causes and consequences (as illustrated in Figure 3, also see Appendix 4). These were represented in individual cause-and-effect tree diagrams to illustrate mental models of socio-economic and environmental interactions in bioenergy production and consumption. A series of group brainstorming sessions were conducted with the researchers at the Center for Environmental and Climate Science, Lund University, to further discuss and validate these cause-and-effect models (not the same experts who were interviewed during Step 2). At this step, these models were complemented with the mental models of the participants of brainstorming sessions. Appendix 4 provides an overview of the process of developing typical cause and effect trees in this research. Nonetheless, in most cases, the model representation and analysis were kept at an abstract level.
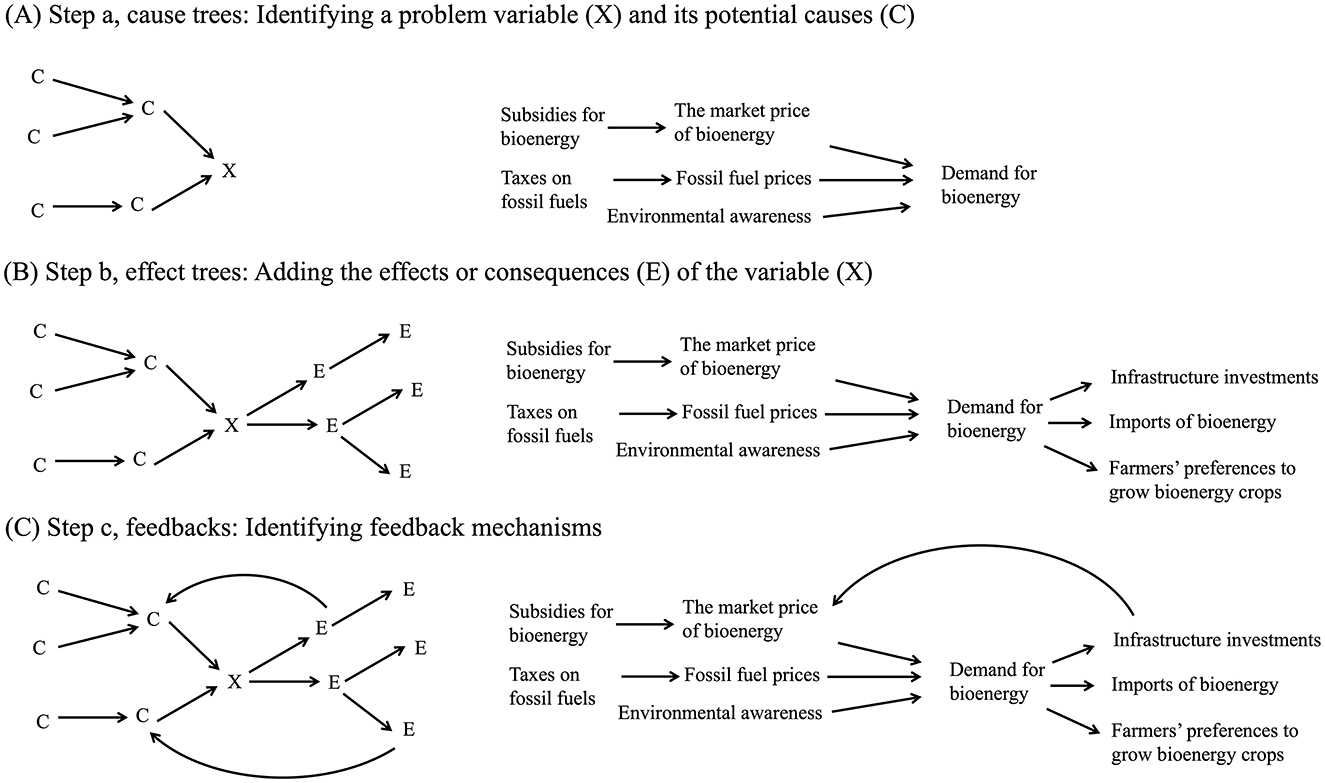
Figure 3. Stepwise process of developing cause and effect trees (Steps a–c). Adapted from Vennix (1996) and Singh et al. (2019). (A) Identifying a problem variable and its causes. (B) Adding effects or consequences. (C) Identifying feedback mechanisms.
At this step, the participants were asked to provide their subjective judgment on the validity of the interrelationships represented in the cause-and-effect trees. The potential observer bias arising from such subjecting judgment was low because the cause-and-effect trees were developed based on theoretical inferences (i.e., step 1) and expert interviews (i.e., step 2). Further, the participants joined the sessions voluntarily and were not part of the research project.
The directionality of cause-and-effect chains was not always straightforward to establish (i.e., similar to the dilemma of “whi chcame first, the eggs or the chicken”). This was especially the case for system variables related to socioeconomic impacts and policy interventions. To solve such conflicting situations, inferences from literature and the DPSIR framework were used.
2.1.4 Causal loop diagrams (Step 4)
The systemic relationships between individual cause-and-effect trees are then represented in a causal loop diagram (CLD; see Appendix 4). This validated system dynamics model represents “a theory about how a system works in some respect” (Barlas, 1996, p. 186). The aim behind developing CLD was to provide insights into the feedback mechanisms behind the socioeconomic and ecological impacts that are often displaced in time and space. This is done to highlight the places where policies are lacking and should focus on to avoid problem shifting and/or impact displacement in time and space. To identify the system structures and/or interactions that need to be disrupted or mitigated and areas of leverage in the system with a large impact, we used a similar process developed by The Omydiar Group (2017).
3 Results
We identified the cause-and-effect interactions in the globalized bioenergy production and consumption system (Table 1). Based on these, we explain the socioeconomic drivers behind the global bioenergy economy (Section 3.1), the global implications of large-scale biomass cultivation for energy purposes (Section 3.2), its ecological challenges (Section 3.3), and socioeconomic negative externalities (Section 3.4).
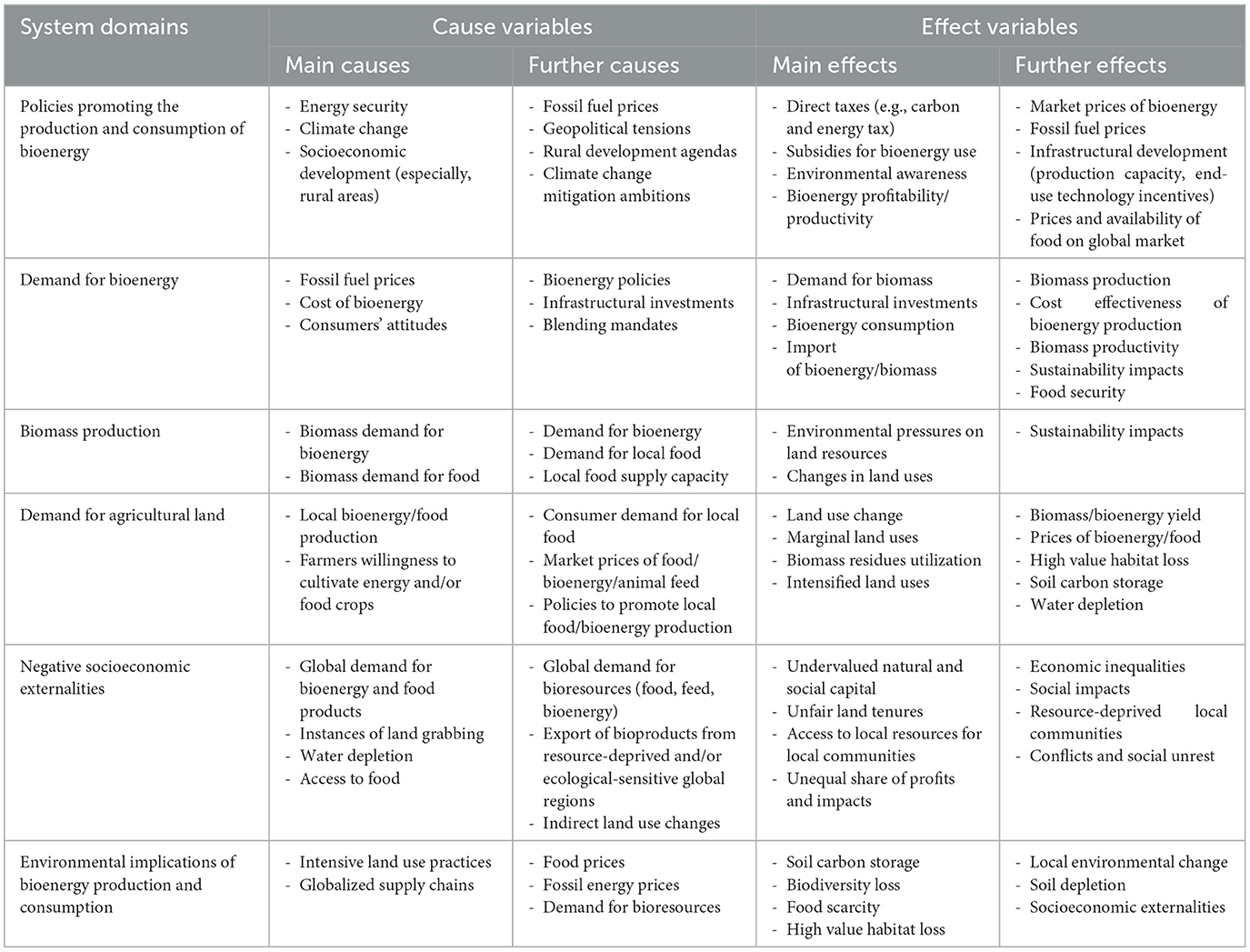
Table 1. Cause-and-effect interactions identified during the critical interpretive synthesis and semi-structured interviews.
3.1 Socioeconomic drivers of the global bioenergy economy
The major drivers of the global bioenergy economy are multifaceted and interconnected, encompassing social, economic, environmental, and geopolitical factors. These are linked to the aims to address climate change, the pursuit of energy security, and socioeconomic development.
3.1.1 Climate change
After the oil crises of the 1970s, biofuels were considered as an alternative fuel and a supplement to fossil fuels for the transportation sector (Timilsina, 2014). In the early 2000s, biofuels were recognized as an essential means of addressing the ongoing climate change and decarbonizing the transportation sector (Ladanai and Vinterbäck, 2009; Fulton et al., 2015). Thus, despite a lack of consensus on whether biofuels could sustainably meet future large-scale energy demand (Fulton et al., 2015), governments worldwide have introduced targets for bioenergy use (Oliveira et al., 2017; Das, 2021). To achieve this, biofuel blending mandates and financial subsidies have been implemented by more than 40 governments worldwide (Timilsina and Shrestha, 2011; Timilsina, 2014; Debnath and Whistance, 2023). These include the European Union (Franco et al., 2010; Schleifer, 2013; Bórawski et al., 2019; Musiał et al., 2021), the United States (Jeffers et al., 2013; Dumortier et al., 2021; Lark et al., 2022; Newes et al., 2022), the United Kingdom (Cross et al., 2021), India (Sinha et al., 2019; Shukla and Mallick, 2023), and China (Weng et al., 2019; Zhang and Feng, 2021).
Fossil fuels supply 96.3% of all transportation fuel needs (International Energy Agency, 2019), accounting for 8 Gt CO2 emissions (International Energy Agency, 2023c). To achieve reductions in greenhouse gas emissions in the transportation sector, biofuels have been proposed as a short- to medium-term solution. In developed countries, climate change issues dominate the energy policy discourses. These countries have implemented financial mechanisms to encourage biofuel use, such as fuel subsidies, tax incentives, and infrastructure-related investments. However, the climate change mitigation potential of biofuels, extensively researched and debated, is contingent upon the type of feedstock, production process, and scale of production (Fargione et al., 2008; Food and Agriculture Organization, 2013).
3.1.2 Energy security
Biofuels can be produced from existing renewable biomass resources. Therefore, they have the potential to enhance energy security by reducing reliance on (fossil)energy imports. Biofuels can help build a sustainable energy system by improving energy flexibility and reliability (Bose and Kumar, 2021; Akhtar, 2023). Biofuels' role in creating a sustainable energy regime and a positive long-term impact on the ecosystem has been recognized globally (Demirbas, 2009; Viju and Kerr, 2013; Gunatilake et al., 2014; Bose and Kumar, 2021). Developing countries have been investing in bioenergy production and consumption infrastructure to reduce (fossil) energy imports, enhance energy security, and lower energy prices. Indeed, biofuel demand is growing in several emerging economies, such as Indonesia, Brazil, and India (International Energy Agency, 2023b). These countries possess significant biomass feedstock, production capacity, and low product costs, e.g., palm oil in Indonesia, soybean in Brazil, and biodiesel in India. They aim to increase bioenergy demand through a mixture of policies focusing on shifting consumers' and producers' preferences for adopting biofuels.
3.1.3 Socio-economic development
Biofuels can help alleviate energy poverty, generate income, and develop local and regional economies (Bose and Kumar, 2021; Sahoo et al., 2022). They can be a low-cost alternative to expensive imported energy, thereby creating more revenues for the governments. Biofuels can help develop agriculture and farming and create more jobs, especially in rural areas (Demirbas, 2009; Sahoo et al., 2022). For instance, the oil palm industry in Indonesia employs more than 6 million people (Purnomo et al., 2020).
These drivers have contributed to developing favorable market conditions for biofuels by increasing the demand for biofuels and reducing the market price of biofuels (represented with reinforcing loop R1 in Figure 4). This was achieved by a range of approaches, including agricultural policies, blending mandates, subsidy support for technologies, import tariffs, tax incentives or penalties, and research and development. The concrete aims to support biofuel development and influencing the financial attractiveness of their production, trade, and use (reinforcing loop R1). For instance, by influencing consumers' preferences toward biofuel use (reinforcing sub-loop R1a), making bioenergy more price-competitive by supporting infrastructure for bioenergy growth (reinforcing sub-loop R1b), motivating farmers to produce energy crops (reinforcing sub-loop R1c). These policies are well-received in society due to their orientation toward addressing climate change and energy security issues and contributing to local or regional socio-economic development. Thus, the global biofuel economy is greatly influenced by geopolitics regimes and international trade dynamics. For instance, an expectation of profitable biofuel exports to the European Union has led certain regions to invest in bioenergy production infrastructure, such as Southern Africa (Henley and Fundira, 2019). The BRICS (Brazil, Russia, India, China, and South Africa) nations have successfully implemented policies to promote biofuel in their respective energy markets (Saravanan et al., 2020). Global biofuel production has rapidly grown in the United States, the European Union, and Brazil. Market factors and policies dictate biofuel trade volumes (Lamers et al., 2012) and influence the prices of other commodities (e.g., fuel, food, and feed) in the global markets. Nonetheless, none of the above-mentioned aims of climate mitigation, energy security, or socioeconomic development have been fully achieved (Debnath and Whistance, 2023).
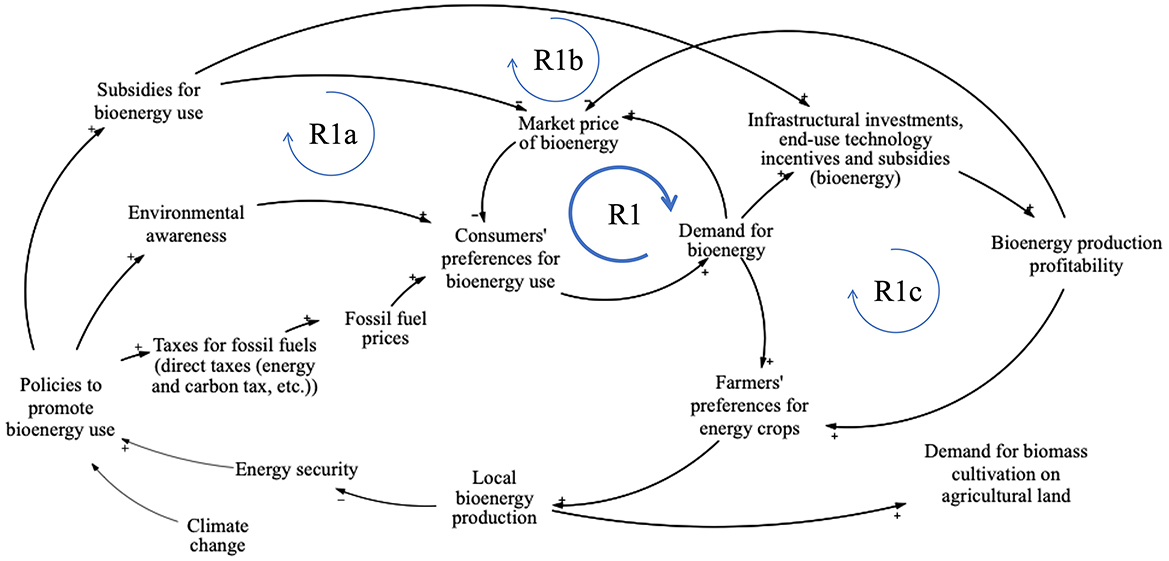
Figure 4. A CLD representing key socioeconomic drivers for the global biofuel demand (reinforcing loop R1). This loop is supported by reinforcing sub-loop R1a (consumers' preferences toward biofuel use), reinforcing sub-loop R1b (bioenergy price-competitiveness), and reinforcing sub-loop R1c (motivating farmers to produce energy crops).
Thus, the global biofuel economy has followed the typical logic of economic growth by addressing the technological, market, financial, and behavioral barriers (creating the reinforcing loop R1). This logic had an implicit assumption that renewable resources are automatically better than fossil fuels. And it has not addressed social and justice issues. This is why the changing (bio)energy policy regimes have created unintended negative socioeconomic and ecological externalities. These are elaborated on in the remainder of this Section.
3.2 Global implications of bioenergy production
In the past decades, significant research has highlighted the socioeconomic and ecological impacts of biofuel production. Biofuel production has been scrutinized for ecological impacts linked to land-use change, increased resource use (e.g., fertilizers), water depletion and soil erosion, and biodiversity loss. The globalization of the biofuel economy has been criticized for its negative socioeconomic externalities, such as food insecurity, land grabbing, unethical contract conditions, social disparity, and gender discrimination.
The CLD in Figure 5 represents some of the major socioeconomic and ecological implications of biofuel production and consumption. This study identified several underlying feedback mechanisms behind these implications (see Table 2). While these implications are spread, spatially and temporally, in the globalized supply chains of biofuels, no such distinction is made in the representation. The following subsystems are represented in this CLD: demand for biomass driving demand for agricultural land (R1 and R2) leading to increased biomass/biofuel yield using marginal lands (or uncultivated farmland, abandoned land, etc.), changes in direct and indirect land use, and intensive farming practices (locally as well as regionally and globally; e.g., R3); and these changes subsequently impacting soil carbon storage, biodiversity, and climate change (R4 and R5). This section outlines the sustainability challenges to biofuel production and consumption from a global perspective.
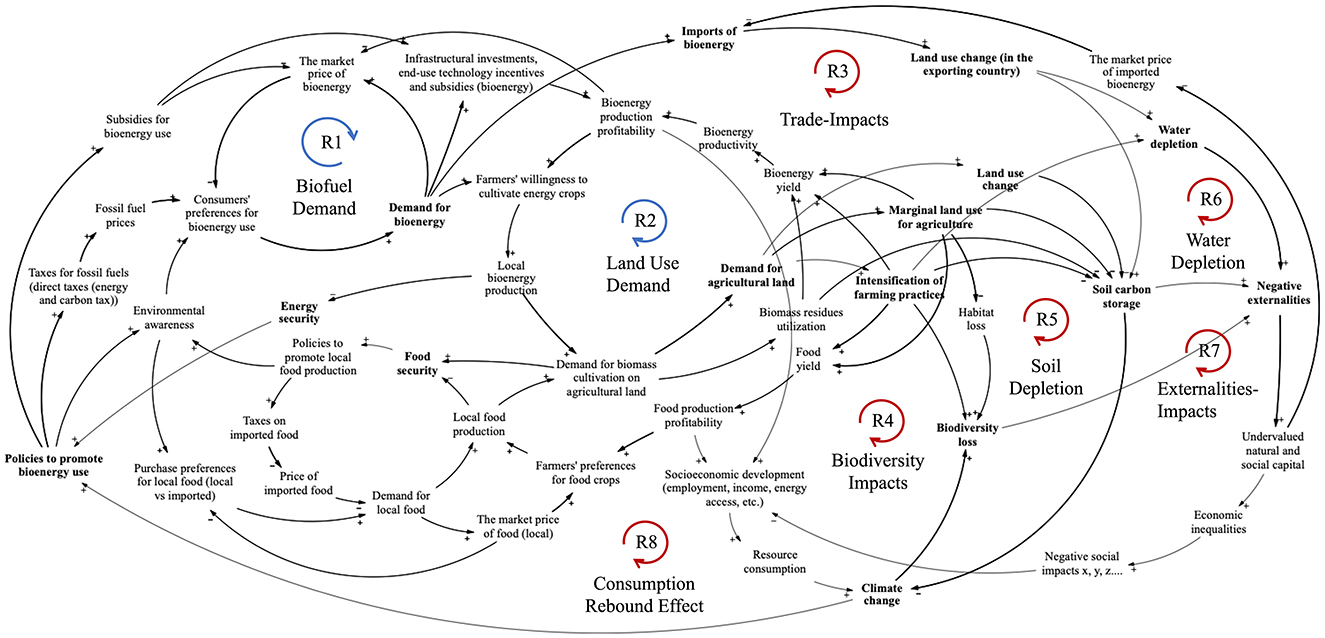
Figure 5. Illustration of the ecological impacts of increased biofuel demand due to environmental policies focusing on climate mitigation by promoting bioenergy production and consumption, leading to reinforcing feedback loops R2: Land Use Demand, R3: Trade-Impacts, R4: Biodiversity Impacts, R5: Soil Depletion, and R6: Water Depletion. The negative social consequences resulting from under-valued natural resources and human capital (R7: Externalities-Impacts) and consumption rebound effects due to overconsumption of (energy or other natural resources) achieved by productivity gains (R8: Consumption Rebound Effects).
3.2.1 Implications of rapid expansion of biofuel production
In the last few decades, global biofuel production has significantly grown due to the above-described drivers. The major biofuel production occurs in the United States, Brazil, the European Union, China, and India due to their agricultural production capacities (Cai et al., 2011). Countries like Argentina, South Africa, Indonesia, Malaysia, Mexico, and Thailand have favorable conditions for biofuel production potential and the requisite technology capabilities (Köhler et al., 2014). Globally, between 2004 and 2010, existing policies and increased oil prices have resulted in a threefold increase in ethanol production and an eightfold increase in biodiesel production (Timilsina and Shrestha, 2011). In 2018, their global production reached a volume of 167.9 billion liters (from 49.9 billion liters in 2005) (Kurowska et al., 2020).
The rapid and large-scale expansion of biomass cultivation for biofuels in some global regions has significant socioeconomic and ecological implications. For instance, in some cases, this expansion has come at the expense of converting forests into cropland and replacing food crops (Achten and Verchot, 2011; Taheripour and Tyner, 2013; Yu and Lu, 2018; Rudke et al., 2022), leading to deforestation, greenhouse gas emissions from land-use change (Timilsina and Shrestha, 2011), water resource depletion (Harto et al., 2010), nitrogen losses, and food prices (Humpenöder et al., 2018). Thus, large-scale bioenergy expansion directly conflicts with the Sustainable Development Goals (SDG) agenda due to negative sustainability impacts and trade-offs (Humpenöder et al., 2018).
3.2.2 Ecological constraints to net primary production of biomass
As compared to stock-limited fossil fuels, biomass-derived biofuels are renewable resources for energy. However, the renewable biomass feedstocks needed to produce biofuels depend on the biosphere's capacity to supply the feedstock flows over time. A useful measure of human intervention in the biosphere is conceptualized by an integrated socioecological indicator—the human appropriation of net primary production (HANPP) (Krausmann et al., 2013). HANPP is the difference between the net primary production (NPP) of the potential vegetation in an ecosystem without humans (NPP0) and the fraction of NPP of actual vegetation left in the ecosystem after human use (Haberl et al., 2007, 2014). HANPP, therefore, quantifies the human-induced effects of changes in productivity and harvest on ecological biomass flows (Haberl et al., 2007, 2014; Krausmann et al., 2013; Mayer et al., 2021). HANPP is the lowest (0%) for a wilderness area and highest (100%) for sealed soils (Haberl et al., 2007; Krausmann et al., 2013). Studies confirm (Chen et al., 2019; Liu et al., 2019) increasing leaf area of vegetation (or NPP) in recent years by human-driven (i.e., human land-use management) and climate-driven factors (e.g., climate change, CO2 fertilization, nitrogen deposition, etc.). However, these increases are partially offset by urbanization (Liu et al., 2019), and at least in some regions and land-use types by warming (Das et al., 2023). Further, globally, NPP has been on the decline due to drivers such as urbanization, human population, and socioeconomic development (Krausmann et al., 2013; Haberl et al., 2014; Liu et al., 2019). To limit global warming to 2°C, an estimated daily biofuel production increase from 9.7 × 106 GJ to 4.6 × 107 GJ—a 20-fold increase - between 2016 and 2040 would be needed (16% of transportation fuels) (International Energy Agency, 2017; Correa et al., 2019). This puts ecological limitations on the use of biomass for human needs, including energy purposes. Therefore, in the future, it will be difficult to raise NPP for longer periods.
3.2.3 Global impacts of rapidly changing trade roles
The production, use, and trade of biofuels are highly globalized. Historically, the major driving factors behind the global biofuel demand and supply depended on (energy) policy regimes in countries that are their major producers as well as consumers, such as the United States, the European Union, and Brazil (Debnath and Whistance, 2023). The other factors that dictate which biofuel's demand grows and where it will be sourced are transport fuel demand, costs, and specific policy design (International Energy Agency, 2022). These factors influence the volume and direction of global biofuel trade (Debnath and Whistance, 2023). For example, in the past, the EU's Renewable Energy Directive (RED) drove the member countries to be the major importers of biodiesel. This (and their national policy agendas) has resulted in unbalanced biofuel production and consumption in these countries. Indeed, Sweden, being one of the biggest consumers of biofuels in the EU (25 TWh of biofuels), produced only 30% of their consumption in 2022 (7.5 TWh) (Lundberg et al., 2023). Sweden has imported low-lifecycle emission biofuels from both within the EU and outside due to their national blending mandates that have created a higher willingness to pay for these fuels (Swedish Energy Agency, 2021; Lundberg et al., 2022, 2023). At the same time, The Netherlands produced twice its domestic consumption due to large investments in the bioenergy production sector and logistical advantages of ports in the country (Lundberg et al., 2023).
In contrast, the USA's Renewable Fuel Standard (RFS) made them the ethanol exporter (Debnath and Whistance, 2023). However, as more and more countries are adopting sustainability criteria, the biofuel trade is declining (Debnath and Whistance, 2023). Indeed, the recent biofuel-related trade restrictions by the EU (after it implemented RED II to reduce the import of fuels with high indirect land use change (iLUC) risk) reduced Indonesia's biodiesel export from 1.1 billion MT in 2019 to 34 thousand MT in 2020. Overall, in 2021, the global trade of biodiesel decreased from 21% of the total production in 2012 to 14% in 2021. Some countries have even changed their trade roles amid these changing energy and sustainability regimes. For example, at the beginning of the biofuel trade, the United States was the largest importer of ethanol, and Brazil was its major exporter. However, as of 2024, the United States has become a major exporter of ethanol, and Brazil is an importer. This globalization of the biofuel economy has caused structural changes in agricultural and food systems across the world that have negative macroeconomic implications, locally or regionally. For instance, Newfarmer and Sztajerowska (2012) described how trade-induced growth may also negatively impact economic growth, employment, income distribution, productivity, and working conditions.
3.2.4 Impact of globalized biofuel economy on energy return on investment
Energy return on investment (EROI) is the ratio of energy returned to society and energy required to get that energy. It is a useful approach for examining the disadvantages and advantages of different fuels (Hall et al., 2009). A low EROI is a limiting factor for the sustainable use of biofuels for mitigating global climate change (Staples et al., 2017). EROI is lower for liquid and gaseous biofuels (German National Academy of Sciences Leopoldina, 2012). The transportation and shipping of biofuels over long distances in the globalized markets negatively impact the overall EROI. Therefore, in principle, a low EROI should promote more local or regional production and consumption of bioenergy. However, this issue may not act as a strong drive because, in the current global bioenergy value chain, the negative (economic) impacts of a low EROI are either disproportionately shared by different actors across the value chain or mitigated by subsidies, or not properly acknowledged.
3.3 Ecological challenges to large-scale bioenergy cultivation
3.3.1 Land-use change
The policies to promote bioenergy have resulted in favorable market conditions for both the producers (farmers, energy suppliers, etc.) and the consumers (households, businesses, and governments; represented by reinforcing loops R1a, R1b, and R1c in Figure 4). This has created a globalized market for high-value bioenergy products, e.g., biofuels (reinforcing loop R3 in Figure 5). Indeed, in recent decades, the bioenergy markets in the European Union, USA, Brazil, the Philippines, and Canada have been one of the major drivers for direct and indirect land use change globally (Merfort et al., 2023). For instance, while the EU's Common Agricultural Policy has reduced the farmed area in their borders, their crops and meat imports have been found to impact deforestation and biodiversity loss in ecologically sensitive areas in Brazil and Indonesia (Merfort et al., 2023). Reducing bioenergy-driven land use change emissions has been recognized as a key factor in deploying bioenergy for climate mitigation (Merfort et al., 2023).
3.3.2 Impacts of intensive farming practices
The increasing demand for agricultural biomass is a major driver for intensive farming practices and the use of marginal lands. Indeed, continuous increases in crop yield over the past 50 years were only possible due to fertilizer application and irrigation. However, this has also contributed to significant climate and ecological risks.
Intensive farming practices have been causing loss of soil carbon, impoverishment of soil structure, biodiversity, and organic matter, including carbon content due to plowing, eutrophication due to excessive fertilizer use (Elobeid et al., 2013; Lark et al., 2022), ecosystem damage, and biodiversity loss (Scheper et al., 2023) due to pesticide use (Fairley, 2022; Sahoo et al., 2022). Indeed, human activities have already exceeded some of the planetary boundaries, for example, biogeochemical flows of nitrogen and phosphorus, freshwater change, functional and genetic biosphere integrity, climate change, and land use change (Rockstrom et al., 2009; Steffen et al., 2015; Richardson et al., 2023). Thus, the option of intensive biomass cultivation to meet future human needs not only becomes a necessity but also a major constraint from an ecological perspective.
Further, intensive harvesting of biomass feedstocks leads to decreased carbon gains or increased carbon losses (from soils), thereby, reducing the potential greenhouse gas benefits (Anderson-Teixeira et al., 2009; Kochsiek and Knops, 2012). Furthermore, the greenhouse gas emissions from biofuel production depend on the type of feedstock and conversion process. A lower density, higher moisture content, and hydrophilic nature of biomass make biofuels more expansive and less efficient than fossil-based energy (Rana et al., 2020). Second-generation biofuels have greater potential for reducing these emissions than first-generation. The emissions from third-generation biofuels are found to be higher than those from conventional fuels (ranging from 10.2 to 1,910 g Co2 eq./MJ) (Patel and Singh, 2023).
Furthermore, biofuel production negatively impacts wild and agricultural biodiversity. A global synthesis by Tudge et al. (2021) shows that local species richness and total abundance were 37% and 49% lower at sites with first-generation, and 19% and 25% lower at sites with second-generation biofuel crops, respectively. However, Winberg et al. (2023) highlight that bioenergy-related effects on ecosystems depend on the original land use, bioenergy crop type, and the scale of production. Nonetheless, they emphasized the need for research and policy to explicitly consider trade-offs between bioenergy production and biodiversity and ecosystem services, and how to avoid them.
3.3.3 The inter-resource dependencies of biofuel production on other inputs
Agricultural biomass cultivation, harvesting, and processing require significant resource inputs such as energy, fertilizers, pesticides, and other chemicals. This puts pressure on other fossil resources, such as phosphorus and water. Further, the water footprints of biofuels are estimated to be 50—240 times higher than those of fossil fuels (Patel and Singh, 2023) and other fossil-free energy technologies, such as solar photovoltaic (Harto et al., 2010). Any trade-offs in such inter-resource interactions will become more critical in the future.
3.4 Socio-economic externalities of the globalized bioenergy economy
CLD in Figure 5 represents these broad negative socioeconomic and ecological externalities of biofuel production and consumption. These negative externalities lead to undervalued natural resources and human capital since the true costs of resource extraction, production, and consumption are not accounted for and reflected in the market prices (loop R7: Externalities Impacts in Figure 5). The undervaluation of natural and human capital has further resulted in rapid and unequal wealth accumulation (and consumption-rebound effects which are not explicitly represented in the CLD).
Firstly, the globalized biofuel value chains produce multiple negative socio-economic and ecological externalities and displace them across geographical boundaries. For instance, as highlighted earlier, biofuel cultivation driven by export demands has resulted in illegal land grabbing [e.g., in some African countries (Robertson and Pinstrup-Andersen, 2010; Palmer, 2014; Vandergeten et al., 2016; Aha and Ayitey, 2017; Conigliani et al., 2018; Cudlínová et al., 2020; Bae, 2023)], corporate lobbying (Pilgrim and Harvey, 2010; Tosun and Schulze, 2015; Deppermann et al., 2016; Cloteau, 2020), large-scale land acquisition by foreign investors in the African continent (Nyantakyi-Frimpong, 2013; Onoja, 2015), soil infertility and erosion (Chatskikh et al., 2013; Khanal et al., 2013; Thomaz et al., 2022), and biodiversity loss (Butchart et al., 2010; Vijay et al., 2016; Essl et al., 2018; Tudge et al., 2021) in producing regions.
Secondly, in the globalized economy, the distributional effects (Martinez Alier, 1995) extend beyond the country implementing the policy (Johnstone and Serret, 2006). Existing research (Ariza-Montobbio et al., 2010; McCarthy, 2010; Obidzinski et al., 2012; Duvenage et al., 2013; Hodbod and Tomei, 2013; Nyantakyi-Frimpong, 2013; Onoja, 2015; Mwale and Mirzabaev, 2016; Schultz and van Riet, 2018) identifies both the positive and negative distributional effects of the biofuel economy. The positive effects include local energy access, labor opportunities, biofuel production-related new economic activity, and improved wages. The marginalization of some local stakeholders, food insecurity, loss of land access for poorer people in rural areas, social disparity, gender discrimination, and adverse labor conditions are some of the significant negative effects (Hodbod and Tomei, 2013).
These socio-economic benefits and/or costs of producing biofuels are not equally shared in society. As per the wide-held perception among policymakers, poor households pay more for the financial costs and receive fewer environmental benefits from environmental policies (Johnstone and Serret, 2006). Globally, biofuel production and consumption in developed countries have been linked to increased global food prices (Fairley, 2022; Lark et al., 2022) due to interactions between agricultural systems and other economic sectors both within and across countries (The Organisation for Economic Co-operation and Development, 2019). Global biofuel production is blamed for the significant consumption of maize, wheat, and vegetable oil due to the increased food prices of these crops, globally (Kurowska et al., 2020; Lark et al., 2022).
OECD countries' agricultural support and protection policies have complex impacts on producers and consumers in other countries. For instance, the EU's Common Agricultural Policy (CAP) setting subsidies based on area rather than on production results in reduced farmland in the region but increased agricultural imports. Indeed, European forests have expanded by 9% [13 million hectares (Mha)], but around 11 Mha was deforested elsewhere to grow crops that were consumed within the EU (Fuchs et al., 2020). Three-quarters of this deforestation was due to oilseed production in Brazil and Indonesia—the regions with global significance for biodiversity conservation, climate mitigation, and carbon storage (Fuchs et al., 2020). Malik et al. (2023) report some of the significant negative externalities of the EU's food trade—5% of the EU's total CO2 consumption-based footprint, 9% of NOX footprint, 16% of the particulate matter footprint, 6% of the total SO2, and 46% of the land-use footprint. The local populations are more likely to be exposed to these negative environmental externalities.
4 Discussion
This study explores and integrates key causal relationships and feedback loops to examine the main synergies and conflicts in the current global bioenergy economy, highlighting the points that our analysis suggests need to be addressed to achieve a globally sustainable and just bioeconomy. We go beyond existing research in eliciting and integrating knowledge from multiple disciplines, such as environmental economics, energy economics, industrial ecology, systems dynamics, environmental justice, and sustainability transitions, utilizing CLDs to visualize the cause-and-effect interactions that are often obscured from different actors in the system, and often distant in time and space.
The study highlights some of the major drivers of the global bioenergy economy are societal concerns related to climate change, energy security, and socio-economic development. These drivers have resulted in a rapid (and unsustainable) global expansion of production, trade, and consumption of bioenergy. This in turn has caused unintended negative ecological and socioeconomic consequences. This research draws from expert interviews, literature synthesis, and discussions with researchers to bring together some of the significant cause-and-effect interactions linking these drivers and consequences, from a systems perspective. For this purpose, we have utilized CLDs to represent and highlight the system structure behind biofuel demand (R1), land use demand (R2), bioenergy trade impacts (R3), biodiversity loss (R4), soil depletion (R5), water depletion (R6) socioeconomic externalities due to undervalued natural and human capital (R7), and consumption rebound effects (R8; see Figure 5). In this complex system, societal policy agendas focusing on the profitability of production, consumption, and trade of bioenergy represent the core of this complex system driving the (unintended) negative ecological and socioeconomic consequences. To address these consequences, systemic transitions are needed in society.
4.1 Need for transition toward a sustainable and just bioeconomy
The CLD model represented a reinforcing loop of climate change and bioenergy development. This implies that the current system of biofuel production and consumption requires balancing modes of system behaviors to fully utilize the climate mitigation potential of bioenergy (or biofuels) and sustainable development. There is a need for a green, just, and sufficient bioeconomy (Giuntoli et al., 2023). Some examples of such balancing modes of system behaviors include sustainable intensification of land use, local or regional production and consumption of bioenergy resources, protection of ecologically sensitive areas from the unintended negative effects of bioenergy-induced demand for land or biomass, and promotion of socio-ecological justice and equity to mitigate the negative social and economic externalities in the globalized bioenergy value chains. In this section, we highlight the need for establishing the following three modes of system behaviors for a sustainable global bioenergy economy:
1. A sustainable bioeconomy to address the ecological crisis.
2. Just transitions for solving the socioeconomic externalities.
3. Coherent governance mechanisms to guide the societal transitions.
CLD in Figure 6 represents the development of a sustainable and just bioeconomy. In this CLD, the system interactions that need balancing effects (the green arrows) through deliberate changes in practices and policies in the bioenergy production and consumption system (in blue text) are shown. Appendix 5 shows these balancing effects. These balancing effects introduce new balancing feedback loops in the system addressing the negative socioeconomic and ecological consequences (see Table 3).
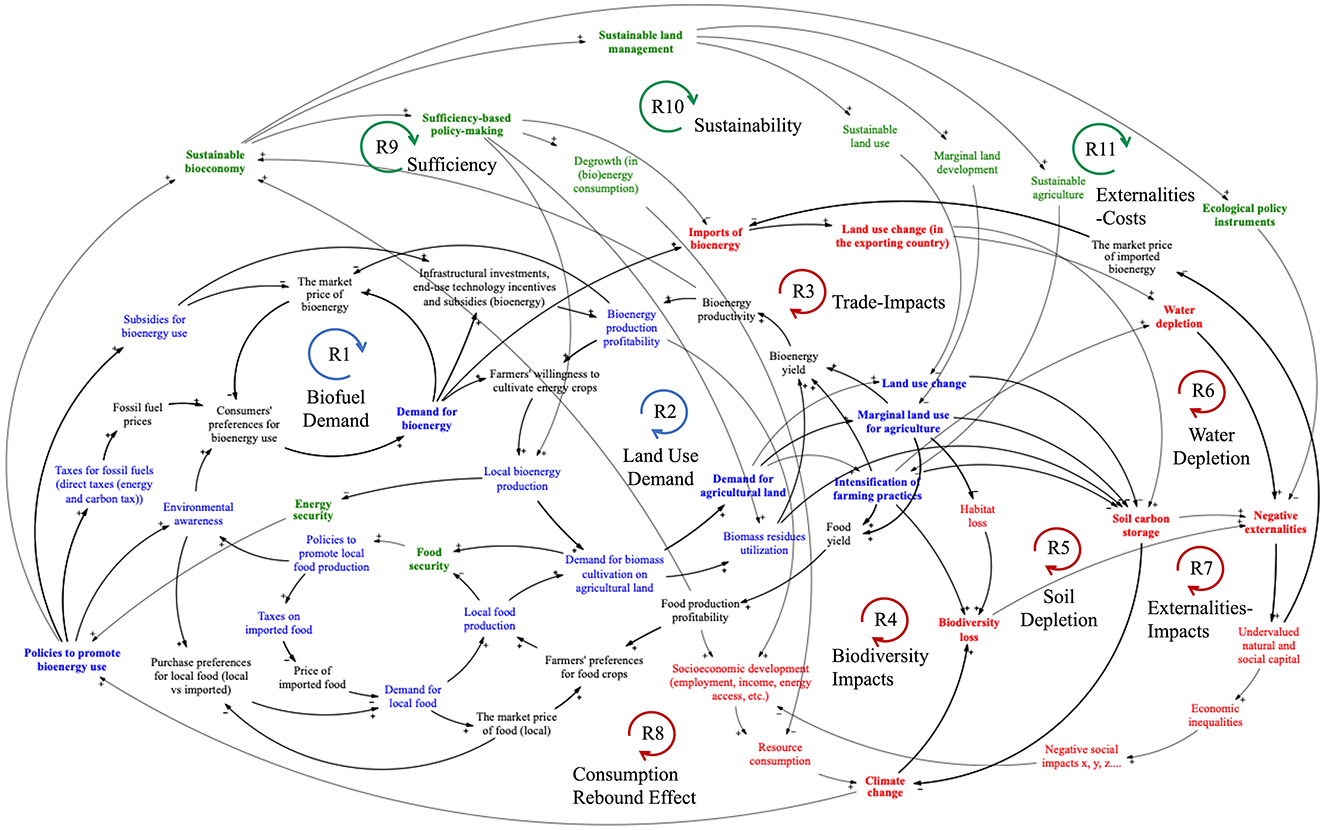
Figure 6. New reinforcing feedback loops R9: Sufficiency, R10: Sustainability, and R11: Externalities-Costs focusing on sustainable and just bioeconomy promoting degrowth and sustainable farming practices and internalizing socioeconomic externalities in biofuel costs (green text). These feedback loops introduce the balancing effects to reduce the negative socioeconomic and ecological impacts of biofuel development (red text) and inform the current production and consumption practices and policies (blue and black text).
Societal transformations toward a sustainable bioeconomy recognize aspects that go beyond anthropocentric or utilitarian views of nature (Giuntoli et al., 2023). This implies the societal demands for biomass (for energy, material, feed, or food purposes) are met within the local or regional ecological limits even at the expense of growth. In this new system, productivity gains (i.e., economic or material—represented by reinforcing sub-loop R1c in Figure 4) are achieved with a sustainable intensification of farming practices, marginal land uses (or under-utilized or abandoned cropland) and utilizing biomass residues but within the ecological limits of the biosphere. These ecological limits serve as the balancing mechanisms to address soil depletion, biodiversity loss, externalities costs, and consumption rebound effects (see Figure 5 representing these loops). Agricultural intensification with improved water resources management and enhanced fertilization efficiency could reduce the negative impacts of bioenergy production (Humpenöder et al., 2018). Utilizing abandoned cropland and biomass residues to enhance bioenergy production could minimize the ecological risks (Gvein et al., 2023).
Until recently, biofuel utilization in society has focused on the substitution of fossil fuels with renewable fuels with added socioeconomic and environmental benefits. This substitution has been led by growth-oriented and market-based approaches rather than sufficiency. Consequently, this resulted in a rapid expansion of biomass resources and the establishment of globalized value chains of renewable fuels. This has further caused significant environmental impacts (as described in Section 3.3) at the cost of reduced biofuel prices. Thus, in this bioeconomy, through suitable policy measures, the reliance on imports of bioresources is discouraged, operationalizing sufficiency and addressing the negative socioeconomic and ecological consequences of expanding the supply sources beyond regions. However, the emphasis is put on approaches to sustainable (bio)energy production and consumption in society. This requires a comprehensive view of bioenergy resources and their potential utilization for meeting societal needs rather than focusing on a single end-use sector or a specific (bio)fuel type. Indeed, a mix of bioenergy end-uses maximizes bioenergy life cycle emission reduction and, thus, its global climate change mitigation potential (Staples et al., 2017).
In the context of the EU Bioeconomy, Giuntoli et al. (2023) highlight how its narratives have predominantly centered around economic growth, technological innovation, and anthropocentric values and have ignored the social and justice dimensions. They have questioned society's roles, relations, and responsibilities since it has failed to produce the desired social and ecological outcomes. They call for a “green, just, and sufficient bioeconomy” (Giuntoli et al., 2023, p. 39) with a focus on societal transformation (i.e., values, norms, and institutions), socio-metabolic limits (i.e., sufficiency and planetary boundaries), and societal responsibility and reciprocity (i.e., justice and equity).
Since the current economic system does not account for the economic costs of socioeconomic and ecological externalities, they are often reflected as disproportionate economic gains. This drives the economic growth engine and causes negative rebound effects (i.e., social, economic, and environmental, as described in Section 3). For instance, low profitability can hamper the adoption of biodiversity-based farming practices (Clough et al., 2016; Scheper et al., 2023; Thomson Ek et al., 2024). This growth engine could be countered if these externalities are fully accounted for through ecological policy instruments. This may result in higher consumer prices and slowed economic growth, but a more just bioeconomy. These ecological and socioeconomic externalities are time- and space-dependent and are often not straightforward (Brose et al., 2010), but need to be accounted for in policy making. This calls for more inclusive environmental policymaking that includes analysis of environmental effectiveness and economic efficiency for different parts of society. The current paradigm of innovation, dominated by private-sector actors and interests, is exclusive and unequal, leading to disruptive technological impacts and uneven spatial development (Schrock and Lowe, 2021). To foster equality and justice, policymakers must enable inclusive innovation (Schrock and Lowe, 2021).
Societal transitions toward a sustainable bioeconomy require coherent governance mechanisms. This must (1) identify the cause-and-effect interactions of all the primary sectors of biomass or bioenergy production (i.e., agricultural, forestry, marine, etc.) with other sectors of the economy and beyond national boundaries (Iriarte et al., 2021); (2) acknowledge and address the synergies and conflicts in interests, roles, and values be enhancing the stakeholders' collaboration and feedback (McLoughlin and Thoms, 2015); and (3) govern the societal transitions by implementing adaptive resource management based on incremental, experiential learning (McLoughlin and Thoms, 2015). The importance of governance of the bioeconomy across sectors and boundaries has increasingly been recognized. However, despite the negative social, economic, and environmental externalities of bioenergy production and consumption, such governance frameworks for the bioeconomy are absent (Lago et al., 2018; Iriarte et al., 2021).
4.2 Limitations of the study and future research opportunities
This paper presents a conceptual systemic model of key drivers of the global biofuel economy and its socioeconomic and environmental impacts. It contains several significant interactions in this system, it is, however, still incomplete like any other model. We do not consider all the beneficial effects of bioenergy use due to the substitution of fossil fuels that are key in promoting the use of biofuels and land-use intensification. Several other causal links and feedback loops could be added. For example, an increased environmental awareness in society can lead to reduced demand for both fossil fuels and biofuels and result in other environmentally conscious behaviors that can have overall positive benefits for the environment. The model also does not fully explore the positive benefits of the globalization of the biofuel economy. For instance, bioenergy could enable energy access to marginalized populations in certain regions and significantly improve the livelihood of farmers and local actors in this economy. The use of bioenergy could also increase fuel costs in some contexts that may lead to reduced energy consumption, such as in Sweden, where regulation of including a higher percentage of biofuels in diesel has increased fuel costs. Certain types of marginal or abandoned land use can have a positive impact on biodiversity and soil quality.
The causality and polarity relationships shown in the CLD are based on the dominant discourse about the issue based on literature. However, some of these causal interactions may have alternative causality and polarity. For example,
• Environmental awareness may not always alter consumers' preferences for bioenergy use or reduced energy demand.
• Reduced prices of bioenergy may cancel out the environmental benefits of using bioenergy due to over-consumption (consumption rebound effects).
• Intensified farming practices may not always lead to increased biomass yield.
• Intensive farming practices may improve the soil quality for some types of lands (e.g., abandoned land).
• An increased biomass demand may not always increase the utilization of agricultural residues
• Bioenergy production profitability may not always result in the farmers' preference for cultivating energy crops, and so on.
The purpose of the model determines its system representation and complexity level (Laurenti et al., 2016). Therefore, we have selected only the relevant variable as per the purpose of this model. This has resulted in an abstract representation of some of the interactions between the natural and social systems (e.g., social externalities). While it was also difficult to represent all of them in a meaningful and reliable way, we intended to represent macroeconomic effects with larger system boundaries. Further, some simplifications are done by representing the behavior of the studied system rather than the detailed interactions between the system variables. Furthermore, there may be “unknown unknowns” that are not captured by the bounded rationality of the authors.
Local, regional, and global interactions are linked not only through the biogeological cycles of carbon, nitrogen, and oxygen but also through imports and exports of resources [see, e.g., the umbrella concept of telecoupling (Liu et al., 2013) that refers to environmental and socioeconomic interaction in today's globalized world]. CLDs have represented these complex and complicated interactions more simply to keep a broad view of the system.
Huge gaps in wealth and wellbeing exist in high-income and medium/low-income countries, as well as rich and poor in these countries. Thus, the benefits of global growth are distributed unevenly, creating significant economic inequalities. Several negative social impacts are associated with economic inequalities, such as adverse effects on social cohesion, physical and mental health, life satisfaction, social trust, wellbeing, and malnutrition. The far-and-wide impacts of these externalities on society are way too complicated and complex to be meaningfully represented in a CLD. Therefore, we have chosen to represent them abstractly (R7 Externalities—Impacts).
To meet the broad objectives of this research, a purposive choice was made to be general, qualitative, and interdisciplinary. This choice led to a few trade-offs, such as a limited analysis of identified issues rather than their in-depth analysis. We may have overlooked specific ecological and socioeconomic consequences regarded as important in an expert field. Further, we have not based our findings on a detailed quantitative or qualitative analysis. CLDs are often developed as a first step to fully understanding a complex problem and exploring potential solution strategies. An in-depth quantitative analysis of the various causal interactions described above can further reveal their relative strength, feedback loops, and dynamics over time.
The developed model of the global bioenergy economy can be used to assess the policy packages at different levels (regional, national, or international). The model can be used to understand critical linkages between the planned policy instruments and their potential negative socioeconomic and ecological consequences. Understanding these linkages can help policymakers identify strategies to proactively address these consequences. The new feedback loops identified in a sustainable and just bioeconomy can guide the development of appropriate economic instruments, institutional structures and governance mechanisms.
Data availability statement
The original contributions presented in the study are included in the article/Supplementary material, further inquiries can be directed to the corresponding author.
Ethics statement
Ethical approval was not required for the studies involving humans because this study does not collect, store, or generate any sensitive data about the interviewed experts for which ethical approval was needed. A verbal consent was obtained from all of the experts' before the start of the interview process. They were also informed about the purpose of the research and the anonymity of their views. No direct quotations are made from the experts' shared information in the manuscript. The studies were conducted in accordance with the local legislation and institutional requirements. The participants provided their written informed consent to participate in this study.
Author contributions
JS: Conceptualization, Data curation, Formal analysis, Investigation, Methodology, Project administration, Resources, Software, Validation, Visualization, Writing – original draft, Writing – review & editing. YC: Formal analysis, Funding acquisition, Methodology, Project administration, Supervision, Validation, Visualization, Writing – review & editing.
Funding
The author(s) declare financial support was received for the research, authorship, and/or publication of this article. This study was funded by FORMAS, The Swedish Research Council for Environment, Agricultural Sciences and Spatial Planning (Grant 2018-01726 to Yann Clough) as part of the National research program on climate and is a contribution to the strategic research area Biodiversity and Ecosystem Services in a Changing Climate (BECC), funded by the Swedish government.
Acknowledgments
We want to thank all the interviewed experts and researchers at the Centre for Environmental and Climate Science, Lund University, for their time to share their expert knowledge with us.
Conflict of interest
The authors declare that the research was conducted in the absence of any commercial or financial relationships that could be construed as a potential conflict of interest.
The author(s) declared that they were an editorial board member of Frontiers, at the time of submission. This had no impact on the peer review process and the final decision.
Publisher's note
All claims expressed in this article are solely those of the authors and do not necessarily represent those of their affiliated organizations, or those of the publisher, the editors and the reviewers. Any product that may be evaluated in this article, or claim that may be made by its manufacturer, is not guaranteed or endorsed by the publisher.
Supplementary material
The Supplementary Material for this article can be found online at: https://www.frontiersin.org/articles/10.3389/fsuep.2024.1460370/full#supplementary-material
References
Achten, W. M. J., and Verchot, L. V. (2011). Implications of biodiesel-induced land-use changes for CO2 emissions: case studies in tropical America, Africa, and Southeast Asia. Ecol. Soc. 16:art14. doi: 10.5751/ES-04403-160414
Aha, B., and Ayitey, J. Z. (2017). Biofuels and the hazards of land grabbing: tenure (in)security and indigenous farmers' investment decisions in Ghana. Land Use Policy 60, 48–59. doi: 10.1016/j.landusepol.2016.10.012
Akhtar, E. T. (2023). Biofuels: a renewable solution for energy security and climate change mitigation. SSRN Electron. J. doi: 10.2139/ssrn.4475528
Anderson-Teixeira, K. J., Davis, S. C., Masters, M. D., and Delucia, E. H. (2009). Changes in soil organic carbon under biofuel crops. GCB Bioenergy 1, 75–96. doi: 10.1111/j.1757-1707.2008.01001.x
Ariza-Montobbio, P., Lele, S., Kallis, G., and Martinez-Alier, J. (2010). The political ecology of Jatropha plantations for biodiesel in Tamil Nadu, India. J. Peasant Stud. 37, 875–897. doi: 10.1080/03066150.2010.512462
Bae, Y. J. (2023). Analyzing the connection between customary land rights and land grabbing: a case study of Zambia. Land 12:200. doi: 10.3390/land12010200
Bali, A. S. (2021). “Navigating complexity in policy implementation,” in The Palgrave Handbook of the Public Servant, eds. H. Sullivan, H. Dickinson, and H. Henderson (Cham: Springer), 669–684. doi: 10.1007/978-3-030-29980-4_28
Barlas, Y. (1996). Formal aspects of model validity and validation in system dynamics. Syst. Dyn. Rev. 12, 183–210. doi: 10.1002/(sici)1099-1727(199623)12:3<183::aid-sdr103>3.0.co;2-4
Blair, J., Gagnon, B., and Klain, A. (2021). Biomass Supply and the Sustainable Development Goals. International Case Studies. Available at: https://www.ieabioenergy.com/wp-content/uploads/2021/10/IEA-Bioenergy-SDG-Case-Study-Report-FINAL-1.pdf (accessed February 23, 2024).
Bórawski, P., Bełdycka-Bórawska, A., Szymańska, E. J., Jankowski, K. J., Dubis, B., and Dunn, J. W. (2019). Development of renewable energy sources market and biofuels in The European Union. J. Clean. Prod. 228, 467–484. doi: 10.1016/j.jclepro.2019.04.242
Bose, S., and Kumar, A. (2021). “Energy security and biofuel,” in Affordable and Clean Energy, eds. W. L. Filho, A. M. Azul, L. Brandli, A. L. Salvia, and T. Wall (Cham: Springer), 530–542. doi: 10.1007/978-3-319-95864-4_154
Brinkman, M. L. J., Wicke, B., Faaij, A. P. C., and van der Hilst, F. (2019). Projecting socio-economic impacts of bioenergy: current status and limitations of ex-ante quantification methods. Renew. Sustain. Energy Rev. 115:109352. doi: 10.1016/j.rser.2019.109352
Brose, I., van Stappen, F., and Castiaux, A. (2010). Articulation of environmental and socio-economic externalities from bioenergy. Manag. Environ. Qual. Int. J. 21, 812–829. doi: 10.1108/14777831011077664
Butchart, S. H. M., Walpole, M., Collen, B., van Strien, A., Scharlemann, J. P. W., Almond, R. E. A., et al. (2010). Global biodiversity: indicators of recent declines. Science 328, 1164–1168. doi: 10.1126/science.1187512
Cai, X., Zhang, X., and Wang, D. (2011). Land availability for biofuel production. Environ. Sci. Technol. 45, 334–339. doi: 10.1021/es103338e
Chatskikh, D., Ovchinnikova, A., Seshadri, B., and Bolan, N. (2013). “Biofuel crops and soil quality and erosion,” in Biofuel Crop Sustainability, ed. B. P. Singh (Hoboken, NJ: WILEY), 261–299. doi: 10.1002/9781118635797.ch8
Chen, C., Park, T., Wang, X., Piao, S., Xu, B., Chaturvedi, R. K., et al. (2019). China and India lead in greening of the world through land-use management. Nat. Sustain. 2, 122–129. doi: 10.1038/s41893-019-0220-7
Ciobanu, N., and Onofrei, C. (2021). “Societal metabolism: a brief introduction,” in Urban Sustainability, eds. J. Papathanasiou, G. Tsaples, and A. Blouchoutzi (Cham: Springer), 1–37. doi: 10.1007/978-3-030-67016-0_1
Cloteau, A. (2020). “How do European lobbyists frame global environmental problems? A case study of the biofuels lobbying campaign through the lens of a major agroindustry,” in Globalizing Issues: How Claims, Frames, and Problems Cross Borders, eds. E. Neveu, and M. Surdez (Cham: Springer), 309–319 doi: 10.1007/978-3-030-52044-1_14
Clough, Y., Krishna, V. V., Corre, M. D., Darras, K., Denmead, L. H., Meijide, A., et al. (2016). Land-use choices follow profitability at the expense of ecological functions in Indonesian smallholder landscapes. Nat. Commun. 7:13137. doi: 10.1038/ncomms13137
Conigliani, C., Cuffaro, N., and D'Agostino, G. (2018). Large-scale land investments and forests in Africa. Land Use Policy 75, 651–660. doi: 10.1016/j.landusepol.2018.02.005
Correa, D. F., Beyer, H. L., Fargione, J. E., Hill, J. D., Possingham, H. P., Thomas-Hall, S. R., et al. (2019). Towards the implementation of sustainable biofuel production systems. Renew. Sustain. Energy Rev. 107, 250–263. doi: 10.1016/j.rser.2019.03.005
Cross, S., Welfle, A. J., Thornley, P., Syri, S., and Mikaelsson, M. (2021). Bioenergy development in the UK and Nordic countries: a comparison of effectiveness of support policies for sustainable development of the bioenergy sector. Biomass Bioenergy 144:105887. doi: 10.1016/j.biombioe.2020.105887
Cudlínová, E., Sobrinho, V. G., Lapka, M., and Salvati, L. (2020). New forms of land grabbing due to the bioeconomy: the case of brazil. Sustainability 12:3395. doi: 10.3390/SU12083395
Das, R., Chaturvedi, R. K., Roy, A., Karmakar, S., and Ghosh, S. (2023). Warming inhibits increases in vegetation net primary productivity despite greening in India. Sci. Rep. 13:21309. doi: 10.1038/s41598-023-48614-3
Das, S. (2021). A perspective of global biofuel policies. Econ. Policy Energy Environ. 31, 45–75. doi: 10.3280/EFE2021-002003
Debnath, D., and Whistance, J. (2023). “The biofuel industry and global trade nexus,” in Biofuels in Circular Economy, eds. S. A. Bandh, and F. A. Malla (Cham: Springer), 33–319. doi: 10.1007/978-981-19-5837-3_16
Demirbas, A. (2009). Political, economic and environmental impacts of biofuels: a review. Appl. Energy 86, S108–S117. doi: 10.1016/j.apenergy.2009.04.036
Deppermann, A., Offermann, F., Puttkammer, J., and Grethe, H. (2016). EU biofuel policies: income effects and lobbying decisions in the German agricultural sector. Renew. Energy 87, 259–265. doi: 10.1016/j.renene.2015.10.005
Dixon-Woods, M., Cavers, D., Agarwal, S., Annandale, E., Arthur, A., Harvey, J., et al. (2006). Conducting a critical interpretive synthesis of the literature on access to healthcare by vulnerable groups. BMC Med. Res. Methodol. 6:35. doi: 10.1186/1471-2288-6-35
Dumortier, J., Carriquiry, M., and Elobeid, A. (2021). Where does all the biofuel go? Fuel efficiency gains and its effects on global agricultural production. Energy Policy 148:111909. doi: 10.1016/j.enpol.2020.111909
Duvenage, I., Langston, C., Stringer, L. C., and Dunstan, K. (2013). Grappling with biofuels in Zimbabwe: depriving or sustaining societal and environmental integrity? J. Clean. Prod. 42, 132–140. doi: 10.1016/j.jclepro.2012.11.011
Elobeid, A., Carriquiry, M., Dumortier, J., Rosas, F., Mulik, K., Fabiosa, J. F., et al. (2013). Biofuel expansion, fertilizer use, and GHG emissions: unintended consequences of mitigation policies. Econ. Res. Int. 2013, 1–12. doi: 10.1155/2013/708604
Essl, F., Erb, K. H., Glatzel, S., and Pauchard, A. (2018). Climate change, carbon market instruments, and biodiversity: focusing on synergies and avoiding pitfalls. Wiley Interdiscip. Rev. Clim Change 9:e486. doi: 10.1002/wcc.486
Fairley, P. (2022). How to rescue biofuels from a sustainable dead end. Nature 611, S15–S17. doi: 10.1038/d41586-022-03649-w
Fargione, J., Hill, J., Tilman, D., Polasky, S., and Hawthorne, P. (2008). Land clearing and the biofuel carbon debt. Science 319, 1235–1238. doi: 10.1126/science.1152747
Food and Agriculture Organization (2013). Biofuels and the sustainability challenge: A global assessment of sustainability issues, trends and policies for biofuels and related feedstocks. Rome: FAO.
Franco, J., Levidow, L., Fig, D., Goldfarb, L., Hönicke, M., and Mendonça, M. L. (2010). Assumptions in the European union biofuels policy: frictions with experiences in Germany, Brazil and Mozambique. J. Peasant Stud. 37, 661–698. doi: 10.1080/03066150.2010.512454
Fuchs, R., Brown, C., and Rounsevell, M. (2020). Europe's Green Deal offshores environmental damage to other nations. Nature 586, 671–673. doi: 10.1038/d41586-020-02991-1
Fulton, L. M., Lynd, L. R., Körner, A., Greene, N., and Tonachel, L. R. (2015). The need for biofuels as part of a low carbon energy future. Biofuels Bioprod. Biorefin. 9, 476–483. doi: 10.1002/bbb.1559
Funtowicz, S. O., and Ravetz, J. R. (1993). Science for the post-normal age. Futures 25, 739–755. doi: 10.1016/0016-3287(93)90022-L
Georgescu-Roegen, N. (1971). The Entropy Law and the Economic Process. Cambridge: Harvard University Press.doi: 10.4159/harvard.9780674281653
German National Academy of Sciences Leopoldina (2012). Bioenergy – Chances and limits. Available at: https://www.leopoldina.org/uploads/tx_leopublication/201207_Stellungnahme_Bioenergie_LAY_en_final_01.pdf (accessed July 4, 2024).
Giuntoli, J., Ramcilovic-Suominen, S., Kallis, G., Monbiot, G., and Oliver, T. (2023). Exploring New Visions for a Sustainable Bioeconomy (eds. J. Giuntoli, S. and Mubareka). Luxembourg: Publications Office of the European Union. doi: 10.2760/79421
Gunatilake, H., Roland-Holst, D., and Sugiyarto, G. (2014). Energy security for India: biofuels, energy efficiency and food productivity. Energy Policy 65, 761–767. doi: 10.1016/j.enpol.2013.10.050
Gvein, M. H., Hu, X., Næss, J. S., Watanabe, M. D. B., Cavalett, O., Malbranque, M., et al. (2023). Potential of land-based climate change mitigation strategies on abandoned cropland. Commun. Earth Environ 4:39. doi: 10.1038/s43247-023-00696-7
Haberl, H., Erb, K. H., and Krausmann, F. (2014). Human appropriation of net primary production: patterns, trends, and planetary boundaries. Annu. Rev. Environ. Resour. 39, 363–391. doi: 10.1146/annurev-environ-121912-094620
Haberl, H., Erb, K. H., Krausmann, F., Gaube, V., Bondeau, A., Plutzar, C., et al. (2007). Quantifying and mapping the human appropriation of net primary production in earth's terrestrial ecosystems. Proc. Natl. Acad. Sci. USA. 104, 12942–12947. doi: 10.1073/pnas.0704243104
Haberl, H., Wiedenhofer, D., Pauliuk, S., Krausmann, F., Müller, D. B., and Fischer-Kowalski, M. (2019). Contributions of sociometabolic research to sustainability science. Nat. Sustain. 2, 173–184. doi: 10.1038/s41893-019-0225-2
Hall, C. A. S., Balogh, S., and Murphy, D. J. R. (2009). What is the minimum EROI that a sustainable society must have? Energies 2:25. doi: 10.3390/en20100025
Hammond, G. P., and Li, B. (2016). Environmental and resource burdens associated with world biofuel production out to 2050: footprint components from carbon emissions and land use to waste arisings and water consumption. Glob. Change Biol. Bioenergy 8, 894–908. doi: 10.1111/gcbb.12300
Harto, C., Meyers, R., and Williams, E. (2010). Life cycle water use of low-carbon transport fuels. Energy Policy 38, 4933–4944. doi: 10.1016/j.enpol.2010.03.074
Head, B. W. (2019). Forty years of wicked problems literature: forging closer links to policy studies. Policy Soc. 38, 180–197. doi: 10.1080/14494035.2018.1488797
Henley, G., and Fundira, T. (2019). Policy and trade issues for a future regional biofuels market in Southern Africa. Dev. South. Afr. 36, 250–264. doi: 10.1080/0376835X.2019.1605882
Hodbod, J., and Tomei, J. (2013). Demystifying the social impacts of biofuels at local levels: Where is the evidence? Geogr Compass 7:12051. doi: 10.1111/gec3.12051
Humpenöder, F., Popp, A., Bodirsky, B. L., Weindl, I., Biewald, A., Lotze-Campen, H., et al. (2018). Large-scale bioenergy production: how to resolve sustainability trade-offs? Environ. Res. Lett. 13:024011. doi: 10.1088/1748-9326/aa9e3b
Hynes, W., Lees, M., and Müller, J. M. (2020). Systemic Thinking for Policy Making: The Potential of Systems Analysis for Addressing Global Policy Challenges in the 21st Century, New Approaches to Economic Challenges. Paris: OECD Publishing. doi: 10.1787/879c4f7a-en
IACGB - International Advisory Council on Global Bioeconomy (2020). Global Bioeconomy Policy Report (IV): A decade of bioeconomy policy development around the world. Available at: https://knowledge4policy.ec.europa.eu/publication/global-bioeconomy-policy-report-iv-decade-bioeconomy-policy-development-around-world_en (accessed September 25, 2024).
International Energy Agency (2022). Renewables 2022, Analysis and forecast to 2027. Available at: https://iea.blob.core.windows.net/assets/5ae32253-7409-4f9a-a91d-1493ffb9777a/Renewables2021-Analysisandforecastto2026.pdf (accessed February 22, 2024).
International Energy Agency (2023a). Renewables 2022: Analysis and forecast to 2027. Available at: https://www.iea.org/reports/renewables-2022 (accessed December 2, 2023).
International Energy Agency (2023b). Renewable Energy Market Update Outlook for 2023 and 2024. Paris: IEA.
Iriarte, L., Fritsche, U., and van Dam, J. (2021). Sustainability governance of bioenergy and the broader bioeconomy. Technical Paper prepared for IEA Bioenergy Task 45 and the Global Bioenergy Partnership (GBEP) Task Force on Sustainability. Available at: http://www.globalbioenergy.org/fileadmin/user_upload/gbep/docs/TFS/Bioeconomy/IINAS__2021__Sustainability_governance_of_bioenergy_and_bioeconomy_-_final.pdf (accessed November 11, 2024).
Jeffers, R. F., Jacobson, J. J., and Searcy, E. M. (2013). Dynamic analysis of policy drivers for bioenergy commodity markets. Energy Policy 52, 249–263. doi: 10.1016/j.enpol.2012.08.072
Johnstone, N., and Serret, Y. (2006). “Distributional effects of environmental policy: introduction,” in The Distributional Effects of Environmental Policy, eds. J. Nick, and S. Yse (Cheltenham: Edward Elgar), 1–2. doi: 10.4337/9781781951132.00006
Khanal, S., Anex, R. P., Anderson, C. J., Herzmann, D. E., and Jha, M. K. (2013). Implications of biofuel policy-driven land cover change for rainfall erosivity and soil erosion in the United States. GCB Bioenergy 5, 713–722. doi: 10.1111/gcbb.12050
Kochsiek, A. E., and Knops, J. M. H. (2012). Maize cellulosic biofuels: Soil carbon loss can be a hidden cost of residue removal. GCB Bioenergy 4, 229–233. doi: 10.1111/j.1757-1707.2011.01123.x
Köhler, J., Walz, R., and Marscheider-Weidemann, F. (2014). Eco-innovation in NICs: conditions for export success with an application to biofuels in transport. J. Environ. Dev. 23, 133–159. doi: 10.1177/1070496513516468
Krausmann, F., Erb, K. H., Gingrich, S., Haberl, H., Bondeau, A., Gaube, V., et al. (2013). Global human appropriation of net primary production doubled in the 20th century. Proc. Natl. Acad. Sci. USA. 110, 10324–1039. doi: 10.1073/pnas.1211349110
Kurowska, K., Marks-Bielska, R., Bielski, S., Kryszk, H., and Jasinskas, A. (2020). Food security in the context of liquid biofuels production. Energies 13:6247. doi: 10.3390/en13236247
Ladanai, S., and Vinterbäck, J. (2009). Global Potential of Sustainable Biomass for Energy. Uppsala: SLU, Institutionen för energi och teknik Swedish University of Agricultural Sciences, Department of Energy and Technology.
Lago, C., Herrera, I., Caldés, N., and Lechón, Y. (2018). “Nexus bioenergy-Bioeconomy,” in The Role of Bioenergy in the Emerging Bioeconomy: Resources, Technologies, Sustainability and Policy, eds. C. Lago, N. Caldés, and Y. Lechón (San Diego, CA: Academic Press), 3–24. doi: 10.1016/B978-0-12-813056-8.00001-7
Lamers, P., Junginger, M., Hamelinck, C., and Faaij, A. (2012). Developments in international solid biofuel trade - an analysis of volumes, policies, and market factors. Renew. Sustain. Energy Rev. 16, 3176–3199. doi: 10.1016/j.rser.2012.02.027
Lark, T. J., Hendricks, N. P., Smith, A., Pates, N., Spawn-Lee, S. A., Bougie, M., et al. (2022). Environmental outcomes of the US Renewable Fuel Standard. Proc. Natl. Acad. Sci. USA. 119:e2101084119. doi: 10.1073/pnas.2101084119
Laurenti, R., Singh, J., Sinha, R., Potting, J., and Frostell, B. (2016). Unintended environmental consequences of improvement actions: a qualitative analysis of systems' structure and behavior. Syst. Res. Behav. Sci. 33, 381–399. doi: 10.1002/sres.2330
Liu, J., Hull, V., and Batistella, M. (2013). Framing sustainability in a telecoupled world. Ecol. Soc. 18:26. doi: 10.5751/ES-05873-180226
Liu, X., Pei, F., Wen, Y., Li, X., Wang, S., Wu, C., et al. (2019). Global urban expansion offsets climate-driven increases in terrestrial net primary productivity. Nat. Commun. 10:5558. doi: 10.1038/s41467-019-13462-1
Lundberg, L., Cintas Sanchez, O., and Zetterholm, J. (2023). The impact of blending mandates on biofuel consumption, production, emission reductions and fuel prices. Energy Policy 183:113835. doi: 10.1016/j.enpol.2023.113835
Lundberg, L., Zetterholm, J., Sanchez, O. C., and Selvakkumaran, S. (2022). Styrmedel Och Biodrivmedel I Eu -Igår, Idag Och Imorgon: Samband mellan konsumtion, produktion och styrmedel för biodrivmedel. FDOS 43:2022. Available at: https://f3centre.se/app/uploads/FDOS-43-2022_50479-1_SR_220524.pdf
Malik, A., Lafortune, G., Dahir, S., Wendling, Z. A., Kroll, C., Carter, S., et al. (2023). Global environmental and social spillover effects of EU's food trade. Glob. Sustain. 6:e6. doi: 10.1017/sus.2023.4
Martinez Alier, J. (1995). Distributional issues in ecological economics. Rev. Soc. Econ. 53, 511–528. doi: 10.1080/00346769500000016
Martinez-Alier, J. (2021). Mapping ecological distribution conflicts: the EJAtlas. Extr. Ind. Soc. 8:100883. doi: 10.1016/j.exis.2021.02.003
Mayer, A., Kaufmann, L., Kalt, G., Matej, S., Theurl, M. C., Morais, T. G., et al. (2021). Applying the Human Appropriation of Net Primary Production framework to map provisioning ecosystem services and their relation to ecosystem functioning across the European Union. Ecosyst. Serv. 51:101344. doi: 10.1016/j.ecoser.2021.101344
McCarthy, J. F. (2010). Processes of inclusion and adverse incorporation: Oil palm and agrarian change in Sumatra, Indonesia. J. Peasant Stud. 37, 821–850. doi: 10.1080/03066150.2010.512460
McLoughlin, C. A., and Thoms, M. C. (2015). Integrative learning for practicing adaptive resource management. Ecol. Soc. 20:art34. doi: 10.5751/ES-07303-200134
Merfort, L., Bauer, N., Humpenöder, F., Klein, D., Strefler, J., Popp, A., et al. (2023). Bioenergy-induced land-use-change emissions with sectorally fragmented policies. Nat. Clim. Change 13, 685–692. doi: 10.1038/s41558-023-01697-2
Mueller, B. (2020). Why public policies fail: policymaking under complexity. Economia 21, 311–323. doi: 10.1016/j.econ.2019.11.002
Musiał, W., Zioło, M., Luty, L., and Musiał, K. (2021). Energy policy of european union member states in the context of renewable energy sources development. Energies 14:2864. doi: 10.3390/en14102864
Mwale, J. T., and Mirzabaev, A. (2016). Agriculture, biofuels and watersheds in the waterenergy- food nexus: governance challenges at local and global scales. Change Adapt. Socio-Ecol. Syst. 2, 91–93. doi: 10.1515/cass-2015-0012
Nel, D., and Taeihagh, A. (2024). The soft underbelly of complexity science adoption in policymaking: towards addressing frequently overlooked non-technical challenges. Policy Sci. 57, 403–436. doi: 10.1007/S11077-024-09531-Y/TABLES/5
Ness, B., Anderberg, S., and Olsson, L. (2010). Structuring problems in sustainability science: the multi-level DPSIR framework. Geoforum 41, 479–488. doi: 10.1016/j.geoforum.2009.12.005
Newes, E., Clark, C. M., Vimmerstedt, L., Peterson, S., Burkholder, D., Korotney, D., et al. (2022). Ethanol production in the United States: the roles of policy, price, and demand. Energy Policy 161:112713. doi: 10.1016/j.enpol.2021.112713
Newfarmer, R., and Sztajerowska, M. (2012). “Trade and employment in a fast-changing world,” in Policy Priorities for International Trade and Jobs (Paris: OECD Publishing). doi: 10.1787/9789264180178-en
Nyantakyi-Frimpong, H. (2013). Biofuels, land grabbing and food security in Africa. Afr. Geogr. Rev. 32, 190–192. doi: 10.1080/19376812.2012.751891
Obidzinski, K., Andriani, R., Komarudin, H., and Andrianto, A. (2012). Environmental and social impacts of oil palm plantations and their implications for biofuel production in Indonesia. Ecol. Soc. 17:art25. doi: 10.5751/ES-04775-170125
Oliveira, G. L. T., McKay, B., and Plank, C. (2017). How biofuel policies backfire: misguided goals, inefficient mechanisms, and political-ecological blind spots. Energy Policy 108, 765–775. doi: 10.1016/j.enpol.2017.03.036
Ollhoff, J., and Walcheski, M. (2002). Stepping in Wholes: Introduction to Complex Systems. Topics in Process Adaptive Systems. 128. Available at: https://openlibrary.org/books/OL9487828M/Stepping_in_Wholes (accessed September 16, 2024).
Onoja, A. O. (2015). Large-scale land acquisitions by foreign investors in West Africa: learning points. Consilience J. Sustain. Dev. 14, 173–188. Available at: https://www.jstor.org/stable/26188748
Palmer, J. R. (2014). Biofuels and the politics of land-use change: tracing the interactions of discourse and place in European policy making. Environ. Plan. A 46, 337–352. doi: 10.1068/a4684
Patel, K., and Singh, S. K. (2023). Environmental sustainability analysis of biofuels: a critical review of LCA studies. Clean. Technol. Environ. Policy 25, 2489–2510. doi: 10.1007/s10098-023-02596-y
Péreau, J. C., Doyen, L., Little, L. R., and Thébaud, O. (2012). The triple bottom line: meeting ecological, economic and social goals with individual transferable quotas. J. Environ. Econ. Manage. 63, 419–434. doi: 10.1016/j.jeem.2012.01.001
Pilgrim, S., and Harvey, M. (2010). Battles over biofuels in Europe: NGOs and the politics of markets. Sociol. Res. Online 15, 45–60. doi: 10.5153/sro.2192
Purnomo, H., Okarda, B., Dermawan, A., Ilham, Q. P., Pacheco, P., Nurfatriani, F., et al. (2020). Reconciling oil palm economic development and environmental conservation in Indonesia: a value chain dynamic approach. For. Policy Econ. 111:102089. doi: 10.1016/j.forpol.2020.102089
Qaim, M., Sibhatu, K. T., Siregar, H., and Grass, I. (2020). Environmental, economic, and social consequences of the oil palm boom. Annu. Rev. Resour. Econ. 12, 321–344. doi: 10.1146/annurev-resource-110119-024922
Rana, R. L., Lombardi, M., Giungato, P., and Tricase, C. (2020). Trends in scientific literature on energy return ratio of renewable energy sources for supporting policymakers. Adm. Sci. 10:21. doi: 10.3390/admsci10020021
Richardson, K., Steffen, W., Lucht, W., Bendtsen, J., Cornell, S. E., Donges, J. F., et al. (2023). Earth beyond six of nine planetary boundaries. Sci. Adv. 9:eadh2458. doi: 10.1126/sciadv.adh2458
Robertson, B., and Pinstrup-Andersen, P. (2010). Global land acquisition: Neo-colonialism or development opportunity? Food Secur 2, 271–283. doi: 10.1007/s12571-010-0068-1
Rockstrom, J., Steffen, W., Noone, K., Persson, A., Chapin, F. S., Lambin, E. F., et al. (2009). A safe operating space for humanity. Nature 461, 472–475. doi: 10.1038/461472a
Rudke, A. P., Xavier, A. C. F., Martins, L. D., Freitas, E. D., Uvo, C. B., Hallak, R., et al. (2022). Landscape changes over 30 years of intense economic activity in the upper Paraná River basin. Ecol. Inform. 72:101882. doi: 10.1016/j.ecoinf.2022.101882
Sahoo, G., Wani, A. M., Swamy, S. L., and Sharma, A. (2022). “Environmental impact and economic benefits of biofuel production,” in Bio-Clean Energy Technologies: Volume 1, eds. P. Chowdhary, N. Khanna, S. Pandit, and R. Kumar (Cham:Springer), 349–378. doi: 10.1007/978-981-16-8090-8_16
Saravanan, A. P., Pugazhendhi, A., and Mathimani, T. (2020). A comprehensive assessment of biofuel policies in the BRICS nations: implementation, blending target and gaps. Fuel 272:117635. doi: 10.1016/j.fuel.2020.117635
Scheper, J., Badenhausser, I., Kantelhardt, J., Kirchweger, S., Bartomeus, I., Bretagnolle, V., et al. (2023). Biodiversity and pollination benefits trade off against profit in an intensive farming system. Proc. Natl. Acad. Sci. USA. 120:e2212124120. doi: 10.1073/pnas.2212124120
Schleifer, P. (2013). Orchestrating sustainability: the case of european union biofuel governance. Regul. Gov. 7, 533–546. doi: 10.1111/rego.12037
Schrock, G., and Lowe, L. (2021). Inclusive innovation editorial: the promise of inclusive innovation. Local Econ. 36, 181–186. doi: 10.1177/02690942211042254
Schultz, C., and van Riet, G. (2018). Biofuel Investments in Africa: lessons for South Africa. S. Afr. Rev. Sociol. 49, 34–52. doi: 10.1080/21528586.2018.1463558
Shukla, R., and Mallick, S. (2023). Conflicting institutional mandates, biofuels, Jatropha in India. J. Asian Afr. Stud. 58, 1173–1188. doi: 10.1177/00219096221081767
Sibhatu, K. T. (2023). Oil palm boom: its socioeconomic use and abuse. Front. Sustain. Food Syst. 7:1083022. doi: 10.3389/fsufs.2023.1083022
Singh, J., Sung, K., Cooper, T., West, K., and Mont, O. (2019). Challenges and opportunities for scaling up upcycling businesses – the case of textile and wood upcycling businesses in the UK. Resour. Conserv. Recycl. 150:104439. doi: 10.1016/J.RESCONREC.2019.104439
Sinha, S. K., Subramanian, K. A., Singh, H. M., Tyagi, V. V., and Mishra, A. (2019). Progressive trends in bio-fuel policies in india: targets and implementation strategy. Biofuels 10, 155–166. doi: 10.1080/17597269.2018.1522483
Staples, M. D., Malina, R., and Barrett, S. R. H. (2017). The limits of bioenergy for mitigating global life-cycle greenhouse gas emissions from fossil fuels. Nat. Energy 2:16202. doi: 10.1038/nenergy.2016.202
Steffen, W., Richardson, K., Rockström, J., Cornell, S. E., Fetzer, I., Bennett, E. M., et al. (2015). Planetary boundaries: guiding human development on a changing planet. Science 347. doi: 10.1126/science.1259855
Sterman, J. D. (2000). Business Dynamics: Systems Thinking and Modeling for a Complex World. New York, NY: McGraw-Hill Education.
Sterman, J. D. (2012). “Sustaining sustainability: creating a systems science in a fragmented academy and polarized world,” in Sustainability Science, eds. M. P. Weinstein and R. E. Turner (New York, NY: Springer), 21–58. doi: 10.1007/978-1-4614-3188-6_2
Swedish Energy Agency (2021). Styrmedel för nya biodrivmedel, Behov och utformning av styrmedel för att främja produktion av biodrivmedel med nya tekniker. ER 2021:22. Available at: https://energimyndigheten.a-w2m.se
Sweeney, L. B., and Meadows, D. (2010). The Systems Thinking Playbook: Exercises to stretch and build learning and Systems Thinking capabilities. White River Junction, Vermont: Chelsea Green Publishing Company.
Taheripour, F., and Tyner, W. E. (2013). Biofuels and land use change: applying recent evidence to model estimates. Appl. Sci. 3, 14–38. doi: 10.3390/app3010014
Terwilliger, J. (2023). The new environmental economics: sustainability and justice. Env. Polit. 32:2172654. doi: 10.1080/09644016.2023.2172654
The Omydiar Group (2017). Systems Practice Worksbook. Available at: https://oecd-opsi.org/toolkits/systems-practice-workbook/ (accessed September 25, 2024).
The Organisation for Economic Co-operation and Development (2019). “The changing landscape of agricultural markets and trade: prospects for future reforms,” in OECD Food, Agriculture and Fisheries Papers, No. 118 (Paris: OECD Publishing).
The U.S. Energy Information Administration (2023). International Energy Outlook 2023 Narrative. Available at: https://www.eia.gov/outlooks/ieo/pdf/IEO2023_Narrative.pdf (accessed December 2, 2023).
Thomaz, E. L., Marcatto, F. S., and Antoneli, V. (2022). Soil erosion on the Brazilian sugarcane cropping system: an overview. Geogr. Sustain. 3, 129–138. doi: 10.1016/j.geosus.2022.05.001
Thomson Ek, H., Singh, J., Winberg, J., Brady, M. V., and Clough, Y. (2024). Farmers' motivations to cultivate biomass for energy and implications. Energy Policy 193:114295. doi: 10.1016/J.ENPOL.2024.114295
Tilman, D., Socolow, R., Foley, J. A., Hill, J., Larson, E., Lynd, L., et al. (2009). Beneficial biofuels - The food, energy, and environment trilemma. Science 325, 270–271. doi: 10.1126/science.1177970
Timilsina, G. R. (2014). Biofuels in the long-run global energy supply mix for transportation. Philos. Trans. R. Soc. A Math. Phys. Eng. Sci. 372:20120323. doi: 10.1098/rsta.2012.0323
Timilsina, G. R., and Shrestha, A. (2011). How much hope should we have for biofuels? Energy 36:23. doi: 10.1016/j.energy.2010.08.023
Tosun, J., and Schulze, K. (2015). Compliance with EU biofuel targets in South-Eastern and Eastern Europe: do interest groups matter? Environ. Plann. C Gov. Policy 33, 950–968. doi: 10.1177/0263774X15605923
Tudge, S. J., Purvis, A., and De Palma, A. (2021). The impacts of biofuel crops on local biodiversity: a global synthesis. Biodivers. Conserv. 30, 2863–2883. doi: 10.1007/s10531-021-02232-5
Vandergeten, E., Azadi, H., Teklemariam, D., Nyssen, J., Witlox, F., and Vanhaute, E. (2016). Agricultural outsourcing or land grabbing: a meta-analysis. Landsc. Ecol. 31, 1395–1417. doi: 10.1007/s10980-016-0365-y
Vennix, J. A. M. (1996). Group Model Building: Facilitating Team Learning Using System Dynamics. New York, NY: John Wiley and Sons, Ltd.
Vennix, J. A. M. (1999). Group model-building: tackling messy problems. Syst. Dyn. Rev. 15, 379–401. doi: 10.1002/(SICI)1099-1727(199924)15:4<379::AID-SDR179>3.0.CO;2-E
Vijay, V., Pimm, S. L., Jenkins, C. N., and Smith, S. J. (2016). The impacts of oil palm on recent deforestation and biodiversity loss. PLoS ONE 11:e0159668. doi: 10.1371/journal.pone.0159668
Viju, C., and Kerr, W. A. (2013). Taking an option on the future: subsidizing biofuels for energy security or reducing global warming. Energy Policy 56, 543–548. doi: 10.1016/j.enpol.2013.01.020
Vogelpohl, T., and Töller, A. E. (2021). Perspectives on the bioeconomy as an emerging policy field. J. Environ. Policy Plann. 23, 143–151. doi: 10.1080/1523908X.2021.1901394
Wang, X., Lim, M. K., and Ouyang, Y. (2017). Food-energy-environment trilemma: policy impacts on farmland use and biofuel industry development. Energy Econ. 67, 35–48. doi: 10.1016/j.eneco.2017.05.021
Weng, Y., Chang, S., Cai, W., and Wang, C. (2019). Exploring the impacts of biofuel expansion on land use change and food security based on a land explicit CGE model: a case study of China. Appl. Energy 236, 514–525. doi: 10.1016/j.apenergy.2018.12.024
Winberg, J., Smith, H. G., and Ekroos, J. (2023). Bioenergy crops, biodiversity and ecosystem services in temperate agricultural landscapes—a review of synergies and trade-offs. GCB Bioenergy 15, 1204–1220. doi: 10.1111/gcbb.13092
Witcover, J., Yeh, S., and Sperling, D. (2013). Policy options to address global land use change from biofuels. Energy Policy 56, 63–74. doi: 10.1016/j.enpol.2012.08.030
Yu, Z., and Lu, C. (2018). Historical cropland expansion and abandonment in the continental U.S. during 1850 to 2016. Glob. Ecol. Biogeogr. 27:12697. doi: 10.1111/geb.12697
Keywords: bioenergy, climate mitigation, challenges, energy policy, impacts, just transitions
Citation: Singh J and Clough Y (2024) Challenges and opportunities to a sustainable bioenergy utilization in climate mitigation: a global perspective. Front. Sustain. Energy Policy 3:1460370. doi: 10.3389/fsuep.2024.1460370
Received: 05 July 2024; Accepted: 25 October 2024;
Published: 27 November 2024.
Edited by:
Alessandro Sciullo, University of Turin, ItalyReviewed by:
Dario Cottafava, University of Turin, ItalySuyash Jolly, Nordland Research Institute, Norway
Copyright © 2024 Singh and Clough. This is an open-access article distributed under the terms of the Creative Commons Attribution License (CC BY). The use, distribution or reproduction in other forums is permitted, provided the original author(s) and the copyright owner(s) are credited and that the original publication in this journal is cited, in accordance with accepted academic practice. No use, distribution or reproduction is permitted which does not comply with these terms.
*Correspondence: Jagdeep Singh, amFnZGVlcC5zaW5naEBjZWMubHUuc2U=