- 1Department of Engineering Technology, College of Science and Engineering Technology, Sam Houston State University, Huntsville, TX, United States
- 2Department of Construction Management, College of Engineering and Computer Science, California State University, Sacramento, CA, United States
This study examines the resilience and sustainability of household-level Food-Energy-Water (FEW) systems in the face of increasing natural disasters exacerbated by climate change. With the backdrop of escalating natural hazards and heightened vulnerabilities, this research explores innovative approaches to enhancing household resilience through the implementation of sustainable FEW systems such as rainwater harvesting, sand filtration, solar energy, geothermal energy, gas-powered and solar generators, hydroponic food production, and small greenhouses. Three primary scenarios, food disruption, energy scarcity, and water shortage are analyzed to evaluate the effectiveness of various household-level FEW systems within a residential context. These systems are compared in terms of cost-effectiveness and performance, highlighting their capacity to supply essential needs during crises. The paper also introduces an integrated nexus approach, considering the interdependencies among food, energy, and water systems, and evaluates the compounded impact of simultaneous disruptions. This comprehensive analysis aims to provide actionable insights into the development of resilient infrastructures at a micro-scale, which can significantly mitigate the adverse effects of global environmental changes on local scales.
1 Introduction
Communities worldwide face recurring disasters stemming from natural hazards such as droughts, earthquakes, fires, floods, hurricanes, and tornadoes. While these events cannot be entirely prevented, their impacts can be mitigated through efforts to reduce vulnerabilities and enhance resilience. In addition to these natural disasters, the COVID-19 pandemic has revealed significant vulnerabilities in global food, energy, and water (FEW) systems, underscoring the importance of household-level resilience. The pandemic exposed weaknesses in supply chains, causing disruptions to food availability, energy consumption patterns, and water demand. The increasing frequency and severity of these disasters, exacerbated by climate change over the past four decades, have made the need for strengthening household resilience and sustainability more urgent than ever. For instance, the devastating flooding in Pakistan in 2022 claimed nearly 1,700 lives (Iqbal, 2022), while over 60,000 fatalities were attributed to heatwaves in Europe during the same year (Ballester et al., 2023).
According to a recent report by the National Oceanic and Atmospheric Administration (NOAA), the United States alone has endured 376 weather and climate disasters with losses exceeding $1 billion each since 1980, with total direct costs surpassing $2.195 trillion (NOAA, 2024). Notably, 2021 marked the seventh consecutive year in which the U.S. experienced 10 or more separate billion-dollar disaster events. As can be seen in Figure 1, the average annual number of such events rose from 8.5 in the period of 1980–2023 to 20.4 in the most recent 5 years (2019–2023), as highlighted in the NOAA report.
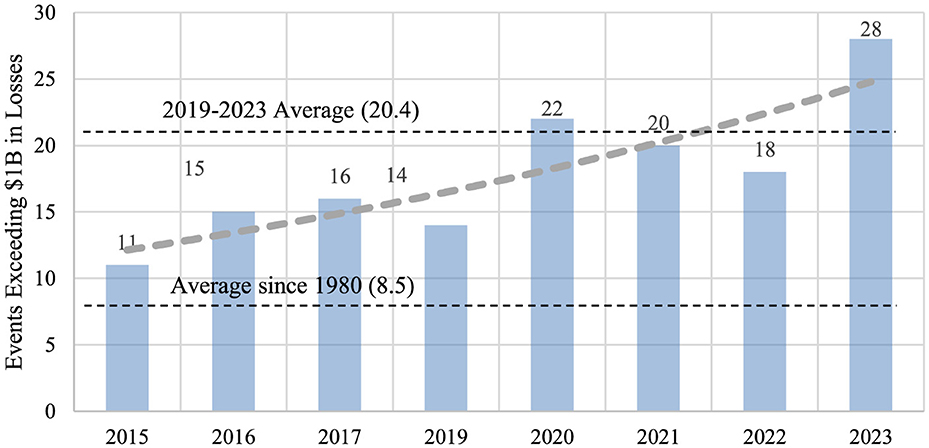
Figure 1. Annual number of weather and climate disasters exceeding $1 billion in losses in the United States (Source: NOAA, 2024).
The necessity for adequate access to FEW is paramount for fulfilling basic human requirements, and resilience stands as a fundamental attribute of robust FEW systems. The COVID-19 pandemic served as a global stress test, highlighting the critical need for households to be more self-sufficient when external systems fail. During the pandemic, many households faced challenges with food shortages, increased energy consumption due to lockdowns, and strained water resources. Resilient FEW systems demonstrate the capacity to endure both short-term disruptions and long-term impacts stemming from natural disasters and extreme events. Given the escalating occurrence of extreme weather phenomena, innovative approaches to bolstering resilience are imperative (Kumar et al., 2024).
While there has been some exploration of FEW nexus resilience over the past decade, research focusing on measuring household-level FEW system resilience remains scarce. Although recent literature has broached the topics of household-level resilience and sustainability, the emphasis often tends to be on individual components like food, energy, or water, rather than considering the interconnections within the nexus (Hertel et al., 2021). Wa'el et al. (2018) stands out as one of the limited studies investigating the influence of seasonal variations on FEW consumption at the household level. Adopting a household-centric approach to assessing sustainability and resilience holds significance, as it provides a foundational framework for more practical development strategies and facilitates the exploration of diverse alternatives for enhancing resilience and sustainability.
The pressures on interconnected Food-Energy-Water (FEW) systems are further heightened by population growth, urbanization, and shifting consumption patterns. Agriculture, which consumes approximately one-third of the world's freshwater resources, is a key stressor on these systems, while energy generation processes such as coal-fired power plants and biofuel crop cultivation also require significant water use (Chen et al., 2018). Additionally, nearly 1.1 billion have limited access to potable water (Ahuja, 2021), 1.5 billion people grapple with inadequate access to modern energy sources and an estimated 1 billion face food insecurity (Scholes, 2020).
Given these challenges, this study examines the resilience and sustainability of household-level FEW systems in the face of increasing natural disasters. Specifically, the research explores sustainable practices such as rainwater harvesting, sand filtration, solar energy, geothermal energy, gas-powered and solar generators, hydroponic food production, and small greenhouses. By focusing on household-level systems, this study seeks to provide practical, scalable solutions for improving sustainability and resilience. Three primary scenarios—food disruption, energy scarcity, and water shortage—are analyzed to evaluate the performance and cost-effectiveness of these systems within a residential context.
An integrated nexus approach is used to consider the interdependencies among food, energy, and water systems, evaluating the compounded impact of simultaneous disruptions. This approach highlights how disruptions in one system can lead to cascading effects on others, making it crucial to explore resilience at a micro-scale. This comprehensive analysis provides actionable insights into the development of resilient infrastructures at the household level, which can significantly mitigate the adverse effects of global environmental changes.
This research adds to the existing body of literature by focusing on the resilience and sustainability of household-level FEW systems, a relatively underexplored area compared to regional and national-scale assessments. The novel approach of quantifying the resilience of decentralized systems, particularly at the household level, provides a framework that can be adapted to different regions and contexts. This study also contributes by incorporating an integrated nexus approach, evaluating the compounded impacts of disruptions across food, energy, and water systems.
2 Exploring household dynamics in the FEW nexus
The choices households make regarding food, energy, and water have profound impacts on each other and the environment. These systems are intricately interconnected, with disruptions in one area leading to direct or indirect consequences in others. Recognizing these interconnections within the FEW nexus is crucial for moving toward a sustainable future. The FEW nexus has been widely studied at regional and global levels, but research on household-level dynamics has been limited. While FEW has been the subject of numerous studies, attention to household-level FEW issues has been relatively limited. This study aims to address this gap by focusing on the practical application of small-scale systems that can enhance household resilience in the face of disruptions.
Studies focusing on household-level FEW have examined various aspects of sustainability. For instance, Dargin et al. (2020) developed a framework for assessing the interaction between physical infrastructure systems in households, considering vulnerability to disruptions as a factor in complex socio-physical system interactions. Their work highlighted the need to develop integrated systems that are adaptable to external shocks, a theme this study explores further through small-scale FEW systems. Itayi et al. (2021) conducted a comprehensive literature review on the FEW nexus, employing qualitative, quantitative, and hybrid methods. This study also scrutinized the assessment scales utilized by existing FEW frameworks, emphasizing the necessity of household-level assessments to comprehensively understand the drivers affecting society at the local level. Building on this, our research applies a more granular approach to assessing household resilience by examining specific small-scale FEW systems, such as solar panels, rainwater harvesting, and hydroponic food production.
Shahbaz et al. (2022) employed an econometric model to explore significant relationships between gender, education, residential area, family size, income, and the sustainability of household consumption patterns. Their findings revealed that females, urban residents, larger families, and wealthier households exhibited higher levels of sustainable consumption practices, underscoring the importance of public policies that prioritize households and promote the adoption of sustainable FEW consumption practices. These socio-demographic factors align with our focus on household-level systems, acknowledging that each household's resources and needs will vary based on its unique circumstances.
Asaki et al. (2024) investigated the determinants of FEW security and their impact on household wellbeing in Ghana, using data from 2,735 households. The findings highlight that while water and energy security have a small influence, food security plays a crucial role in promoting household wellbeing, with various socioeconomic factors affecting each form of resource security.
Additionally, Wa'el et al. (2017) developed a model to capture interactions between FEW at the household level. The developed model offers a means to quantify FEW demands and waste generated by households. Wa'el et al. (2018) conducted a study that assessed the risk of exceeding acceptable levels of shortage in FEW demands and investigated the impact of seasonal variability on WEF consumption. This study builds on Wa'el's work by investigating how small-scale systems can address seasonal variations and resource shortages, providing a practical framework for households to enhance resilience.
While many of these studies have focused on assessing FEW interactions in households, the majority have examined this issue from a top-down perspective, lacking the specific focus on the implementation of practical, small-scale systems that this study aims to address. Another study by Xue et al. (2021) involved the development of a model capable of providing long-term climate change simulations spanning from 2010 to 2050. This model incorporates various factors such as behaviors, appliances, and resource prices. This study aims to complement this work by offering an actionable approach to integrating small-scale FEW systems that can be adapted to changing climate conditions.
Table 1 presents a summary of previous studies elucidating household-level FEW concepts. By offering a practical method for measuring the sustainability and resilience of small-scale FEW systems, this study contributes to the existing literature by focusing on their cost-efficiency and feasibility at the household level.
3 Research questions and objectives
This study focuses on assessing the resilience and sustainability of household-level FEW systems. The impetus for this research stemmed from the challenges faced during the COVID-19 pandemic, which heightened awareness of the vulnerability of centralized FEW systems to disruptions. The pandemic exposed the critical need to evaluate how well households can adapt to shortages and changes in access to food, energy, and water resources. Particularly, the pandemic underscored the importance of evaluating the resilience and sustainability of FEW systems at the household level. This realization became evident as the authors observed the difficulties faced by households accustomed to consistent access to FEW resources.
To delve deeper into this issue, a qualitative study was conducted involving interviews with 11 residents from the City of Huntsville, Texas. These interviews were conducted as part of a class project that aimed to explore the effects of COVID-19 on household-level FEW systems. The relatively small sample size of 11 participants was due to the scope of the class project and resource constraints. Participants were identified by reaching out to a local cultural center near the campus, which facilitated connections with community members interested in sharing their experiences. Selected through purposive sampling based on their willingness to share their experiences with FEW challenges during the pandemic, this sample offers valuable initial insights into how households responded to FEW system disruptions during the pandemic.
The interviews used a structured format, with questions designed to explore key aspects of household-level FEW resilience. Residents were asked about the challenges they encountered with FEW supplies and demands before and during the COVID-19 pandemic, the impact of the pandemic on FEW access and affordability, strategies for building resilient FEW systems, and measures to enhance FEW availability in the community. Through these discussions, the study aimed to understand the specific needs and preferences of households during a crisis, particularly with respect to adapting to changes in FEW access.
The findings from these interviews emphasized the significance of household-level assessment techniques in gauging FEW systems resilience. Unlike large-scale systems, household-level systems are inherently more resilient, less complex, and not as susceptible to disruptions in other systems. While they may lack the efficiency of larger systems due to the absence of economies of scale, household-level systems are more financially feasible.
Consequently, a fundamental research question arises: how to assess the resilience and sustainability of household-level FEW systems? By addressing this question, our objectives are 2-fold: firstly, to develop a framework for analyzing FEW systems in terms of their resilience and sustainability, and secondly, to identify strategies to enhance household resilience through existing or novel FEW systems. Establishing a tangible definition of household FEW resilience and sustainability is imperative for achieving these objectives. The scope and assumptions of the research necessary to address this question are further elucidated in the subsequent section. Having established the importance of household-level FEW systems in enhancing resilience and sustainability, the following section outlines the methodology employed in this study to measure and evaluate these systems.
4 Materials and methods
This study employs a quantitative approach to assess household-level Food, Energy, and Water (FEW) systems by measuring their sustainability and resilience under various disruption scenarios. A scenario-based analysis is used to evaluate the performance of small-scale systems—such as rooftop solar panels and rainwater harvesting—during periods of energy scarcity, water shortages, and food supply disruptions. This approach allows for the practical quantification of system performance and recovery time, providing actionable insights into household-level resilience within the FEW nexus.
The methodology focuses on small-scale systems applicable to individual households, such as rooftop solar panels, but excludes larger-scale installations like nuclear or gas-fired power plants. Additionally, the resilience of each system can vary depending on the type of adverse event. For example, while a food system might demonstrate resilience to earthquakes, it could be vulnerable to windstorms. However, this study does not examine specific types of natural disasters in detail but focuses solely on disruptions that occur after an adverse event. The primary function of FEW systems is to provide food security, energy, and water services to households. Therefore, when measuring sustainability and resilience, it is essential to consider household demand for FEW consumptions. According to the U.S. Census Bureau, an average American household consists of approximately 2.51 individuals (U.S. Census Bureau, 2022). Table 2 presents the daily consumption levels of FEW for an average American household, with estimates indicating a requirement of approximately 5,680 calories, 745 liters of water (Crouch et al., 2021)and 29 kWh of energy per day at home (EIA, 2020).
Assumptions for this study include the scenario in which the household resides in a dwelling with opportunities to install small-scale FEW systems. The analysis will consider an average dwelling in Texas (single-family house) and California (apartment). Furthermore, it is assumed that FEW systems will remain functional as long as the house stands and survives the natural disaster. The National Oceanic and Atmospheric Administration (NOAA) emphasizes the role of climate variability in shaping regional resilience needs (Masao, 2024). In the Midwest and Southern U.S., frequent flooding necessitates robust water management systems, while in Western states prone to drought, water conservation strategies such as rainwater harvesting are essential. Additionally, solar energy adoption may vary across the U.S. based on average sunlight availability, with regions like the Southwest benefitting more from solar installations compared to areas with frequent cloud cover. These regional climate factors should inform household resilience planning, ensuring that FEW systems are adapted to local environmental conditions.
4.1 Measuring sustainability and resilience
In the past decade, methodologies and models for measuring resilience and sustainability of critical infrastructures have undergone significant evolution. However, the practical implementation of quantitative methods in household-scale systems, irrespective of the nature and scale of adverse events, lacks well-developed conceptualization methodologies. This section adopts definitions and metrics primarily developed by built environment and system engineering researchers and presents a practical mechanism for assessing FEW systems regarding their contribution to sustainability and resilience at the household level.
A sustainable system is one that consistently meets its demands from renewable sources (Karan et al., 2018). Therefore, the sustainability of a system can be measured by calculating the percentage of FEW retrieved from renewable sources. The sustainability index (SI) for food (F), energy (E), or water (W) can be determined using the following empirical equation:
where, Srenewables represents the supply for the food, energy, or water component from sustainable sources such as solar, wind, geothermal, biogas, and biofuel for energy, and precipitation, wastewater, and seawater for water. D denotes the expected demand for the food, energy, or water component. Food systems are deemed sustainable unless their water and energy use stem from non-renewable sources. Further details on the key components and sub-components contributing to the sustainability of FEW systems can be found in Karan and Asadi (2018).
Resilience refers to the ability of a system to absorb disturbances, reorganize, and maintain essentially the same function, structure, identity, and feedback despite undergoing change (Walker et al., 2004). In the context of household-level FEW systems, resilience can be defined as the ability of the system to absorb the impacts of system disruption and maintain its essential functions and performance in the case of a disruptive event. Performance monitoring lies at the core of resilience measurement. This study quantifies system performance after the occurrence of a disruptive event based on the system delivery (e.g., kWh of electricity or liter of water not supplied). System performance shall be expressed as a function of time; the faster a system can recover from a disruption and return to its normal performance, the more resilient it becomes. Therefore, an integral equation is proposed to measure resilience of FEW systems:
where P0 represents the original stable performance level (before the occurrence of the disruptive event), P(x) denotes the performance level starting immediately post-disruption and before the final recovery, t0 signifies the start time of the disruptive event, and tr is the time to final recovery.
The necessity and importance of services are considered in defining system performance. The performance of a food system is defined as its ability to meet household food demand, with weights calculated based on recommended daily intake amounts. To quantify these weights, this study utilizes dietary guidelines from the dietary information provided by the Food and Agriculture Organization (FAO) of the United Nations (UN) (Kennedy et al., 2011) and the United States Departments of Agriculture (USDA) and Health and Human Services (HHS) food pattern for an average American household (USDA, 2015). These guidelines specify average daily intake values across various food groups, each assigned a weight that reflects its relative importance in sustaining household dietary needs. Table 3 presents these intake weights, providing a standardized framework for assessing food system performance in terms of its capacity to fulfill daily nutritional requirements under normal and disrupted conditions.
The performance of an energy system is defined as its ability to supply energy to meet household demand. If a household consistently has access to sufficient energy sources—such as electricity or gas—to power essential functions like air conditioning, heating, and lighting, the system is considered to be operating at 100% performance. The performance of a water system is defined as its ability to meet household water demand across essential uses. In this study, performance is measured not only by the quantity of water supplied but also by the priority of each use. For instance, while drinking water constitutes < 3 percent of total water consumption of an average household (see Table 2), it is more essential than landscape irrigation, car washing, or even toilet flushing for the water system. If a household reliably has access to adequate water for high-priority uses like drinking, cooking, and sanitation, the system is considered to be operating at full performance (100%). The amount of time between two uses determines the contributing weight of each consumption category, with shorter time intervals associated with higher weights. Table 3 lists the time intervals to meet the FEW demands and their relative weights. These reported time intervals aim to provide a common quantitative basis for the contribution of each FEW uses regarding system performance.
The U.S. Energy Information Administration (EIA), the Environmental Protection Agency (EPA), and the US Geological Survey (USGS) serve as the primary sources of the data presented in Table 3 (O'Conner et al., 2010; EIA, 2017; Dieter et al., 2018). Regarding energy consumption, the usage for AC/heating varies significantly with outside air temperature. An average of 3 h is employed in this study as it is reported in multiple questionnaires and surveys (Ekasiwi et al., 2013; Meng et al., 2019; Zhang et al., 2020). Food safety considerations during power outages serve as the basis for the time interval assigned to the appliances category.
To assess sustainability and resilience throughout the Food, Energy, and Water (FEW) nexus, a previous study proposed utilizing an unweighted geometric mean to ensure consistent weighting across components, thereby ensuring that any change in one component equally influences the integrated FEW Sustainability Index (SI) (Karan and Asadi, 2018). This study adopts the following equation to measure the sustainability and resilience of FEW systems:
The equations developed for measuring the sustainability and resilience of an energy system are illustrated using an example of a gas-powered portable generator. The generator has a capacity of 3,500 running watts (equivalent to 3.5 kWh), which allows it to power essential appliances during power outages. Table 4 summarizes the generator's performance during different power outage scenarios.
During a 4-h power outage (Scenario 1), the generator is primarily used to power the refrigerator and lighting, consuming 2.5 kWh, which utilizes 71% of the generator's capacity. In a longer, 7-h outage (Scenario 2), additional appliances such as the TV are powered, consuming the full 3.5 kWh capacity.
Two scenarios depicted in Figure 2 are explored: Scenario 1, representing a relatively short 1-h power outage, and Scenario 2, indicating a longer 6-h outage. In Scenario 1, without the portable generator, the system fails to perform. However, with the generator, the household can maintain essential functions such as lighting (2.7 kWh out of 2.7 kWh) and AC/heating (0.8 kWh out of 13.1 kWh) during the outage. In Scenario 2, where the outage persists for 6 h, the portable generator enables the household to sustain essential appliances (3.5 kWh out of 3.7 kWh) until the utility company restores power. In both scenarios, it is assumed that the original stable performance level of the energy system is 100%, while without the generator, the performance of the energy system drops to zero percent during the outage.
The implementation of a generator solution can enhance the resilience of the energy system by 54% in the first scenario and 12% in the second scenario. However, it's worth noting that since the generator is gas-powered, its Sustainability Index (SI) remains at zero. Conversely, employing a fully charged solar-powered generator with an equivalent capacity can bolster the sustainability of the system by 12%, calculated as 3.5 kWh out of an average daily energy consumption of 29 kWh for an American household.
4.2 Estimating output and costs of household-scale FEW systems
The study's scope is confined to available FEW systems suitable for household use. Small-scale systems include solar, geothermal, and portable generators for energy systems, rain barrels for water collection, slow-sand filtration for water purification, and indoor planter hydroponics and outdoor small greenhouses for food production. This section outlines the methodology for estimating the output of these small-scale FEW systems and provides a practical approach to estimating their costs. Table 5 presents the average prices of these small-scale FEW systems.
To contextualize energy needs for typical U.S. households, data from the U.S. Energy Information Administration (EIA) indicate that average annual energy consumption per household is approximately 10,632 kWh (González-Torres et al., 2022). This figure, however, varies significantly by region. Households in the South tend to have higher energy usage primarily due to increased cooling needs, while homes in the Northeast often exhibit high heating demands. Such regional distinctions underscore the varying resilience requirements across U.S. households, suggesting that resilience strategies like solar or geothermal energy systems should be tailored to address region-specific demands. The electricity generation of a solar system can be calculated using the formula:
where Esolar is measured in watts, Ecell is the solar cell efficiency (%) under standard test conditions (temperature of 25 °C, irradiance of 1,000 W/m2, air mass 1.5 spectrum), G is the irradiance of input light (measured in W/m2), Acell is the surface area of the solar panels (measured in m2), TkP is the temperature coefficient of solar panel (%/°C), and AT is the ambient temperature (°C).
In estimating the costs of household-scale solar energy systems, data was collected from 11 major brands available in the U.S. market, including Qcells, Silfab Solar, JA Solar, JinkoSolar, Canadian Solar, Panasonic, and others. A comprehensive database of 46 solar panel models from these brands was compiled to provide an accurate estimate of system costs. The database includes a variety of solar panels differing in terms of efficiency, power output, and price range, ensuring that the cost estimates reflect the diverse options available in the U.S. market. The analysis assumes an average solar cell efficiency of 16%, which is consistent with the performance of standard residential solar panels. The standard surface area for most commercially available panels is 1.7 m2, which was factored into the output calculations. As shown in Table 5, the cost of the solar system is dependent on the number of solar panels installed.
The energy generation of a geothermal system can be calculated using the formula:
where Egeothermal is measured in watts, and the geothermal well depth is measured in meters. In estimating the costs of household-scale geothermal energy systems, a database of 35 geothermal heat pump models from leading manufacturers was compiled to provide accurate cost projections. The data was collected from major U.S. brands, including WaterFurnace, Bosch, ClimateMaster, and Carrier, which are recognized for their high-performance geothermal systems. These models vary in capacity and efficiency, ranging from entry-level to advanced systems designed for larger residential spaces.
The cost estimation considers both the initial installation costs, which include drilling and heat pump installation, and the operational costs over time. The average geothermal system efficiency is based on a Coefficient of Performance (COP) of 3.5, indicating that for every unit of electricity consumed, the system produces 3.5 units of heating or cooling. By utilizing real-world pricing from these leading manufacturers, the financial analysis provides a robust assessment of the investment required for installing geothermal systems at the household level.
There are two popular options for generators aimed at enhancing the resilience of household energy systems: traditional portable generators and inverter generators. Traditional portable generators, due to their constant speed, are generally less efficient compared to inverter generators. Inverter generators incorporate a built-in inverter, which enhances efficiency by adjusting the speed based on the load requirement. While the majority of generators operate on gas, solar generators are also available in smaller capacities. A typical household-level generator can provide power ranging from 10 kW to over 200 kW, which is sufficient for ensuring basic comfort and security. To estimate the cost of a household-level generator, data on pricing, running wattage, and fuel capacity of more than fifteen portable generators available in the market were collected.
The energy generation of a household-level generator can be calculated using the formula:
where E portablegenerator is in kilowatts (kw). The average price of a household-level generator is influenced by the running wattage of the equipment. Fuel and maintenance are also associated costs with household generators.
In estimating the costs of household-scale generators, a database of 15 gas and solar-powered generator models from well-known manufacturers such as Generac, Honda, Westinghouse, and Goal Zero was compiled. These models were selected based on their output capacities, ranging from 3.5 kWh to 10 kWh, to provide a comprehensive understanding of the costs for both small-scale and larger household power backup systems.
where E solar generator is in kilowatts (kw). The price of solar generators varies based on their running wattage.
The cost estimation accounts for the initial purchase price, fuel or charging costs (for solar-powered generators), and maintenance. The gas-powered generators were assessed based on an average fuel efficiency of 0.75 gallons per hour under a typical load, while solar generators were evaluated for their recharge times and solar panel compatibility. The collected data provides a reliable basis for estimating the overall investment required for backup power systems, depending on the household's energy needs during outages.
A rainfall collection system in regions experiencing moderate to high rainfall or snowfall is arguably the most effective and economical option for enhancing both the resilience and sustainability of a household system. The water collection of a rainwater harvesting system can be determined using the formula:
where Wprecipitation is measured in liters, PRC represents the amount of precipitation (rainfall or snowfall) in millimeters, and Asystem denotes the surface area of the precipitation harvesting system (e.g., roof) in square meters.
The costs of household-scale rainwater collection systems and slow sand filtration systems were estimated using data from RS Means, a trusted source for construction cost estimation. The database includes comprehensive cost information for materials, installation, and maintenance, providing an accurate reflection of market conditions for both rainwater harvesting and water filtration systems.
For the rainwater collection system, the cost estimation considered the average cost per square foot for installing a collection surface (roof), gutters, downspouts, and storage tanks. The system capacity was calculated based on typical household water demand and regional rainfall data, following the guidelines from EPA's rainwater harvesting model. Similarly, the slow sand filtration system was assessed based on RS Means data for material and labor costs, which includes the cost of constructing the filtration bed, layering sand and gravel, and routine maintenance.
Slow sand filtration is another water system suitable for household-scale implementation. This filtration system effectively removes suspended solids, turbidity, metals, and microbes through a single physical treatment process. Raw water, such as greywater from faucets or showers, passes over sand and subsequently through a supporting gravel layer. The filtered water, stored at the bottom of the tank, can be utilized for various purposes including toilet flushing, irrigation, and in some cases, dish and cloth washing (Blackwood et al., 2017). The quality of the filtered water is influenced by the depth of the tank (sand and gravel thicknesses), and the output flow rate is determined by the tank's surface area, as illustrated by the following equation:
where Wfilteration is measured in liter per hours and Atank denotes the surface area of the sand filtration system precipitation harvesting system in square meters.
Hydroponics indoor garden grows produce in the smallest space. Hydroponic gardening is the process of growing plants without soil. Regardless of the weather outside, a wide variety of edible plants can be grown indoors all year round (e.g., herbs, veggies, and fruits). Growing plants this way is easy, clean, and efficient, especially in limited spaces. In most indoor settings, hydroponic gardening produces healthier and higher-quality plants.
In estimating the costs of household-scale hydroponic systems, data was collected from a variety of commercially available systems in the market. This includes cost data from 15 hydroponic models offered by leading brands such as AeroGarden, Rise Gardens, and Lettuce Grow. These systems vary in size, plant capacity, and technological sophistication, with models ranging from small countertop setups to larger, more automated systems designed for higher yields.
The cost estimation considers the initial purchase price, electricity consumption for lighting and water circulation, and ongoing operational costs such as nutrient solution and replacement parts. By incorporating real-world pricing from these commercially available hydroponic systems, the study provides a realistic assessment of the investment required for household food production using hydroponics. The selected systems were evaluated for their efficiency in producing vegetables, herbs, and small fruits, making them suitable for households aiming to enhance food security and resilience. The number of plants can be grown in a hydroponics indoor garden based on the cost of the system can be calculated as follows:
where N is the number of different plants can be grown in a hydroponics indoor garden. The pH level of hydroponic systems must be monitored, water and nutrients need to be changed, and the system must be cleaned regularly. To calculate the total cost, these costs must be included.
In areas with cold or unpredictable climates, outdoor greenhouses can provide a controlled environment for growing food plants. By creating a protected environment, small greenhouses extend the growing season for food systems. With a small greenhouse, a large variety of crops can be produced, expanding the food system's variety and nutritional diversity. Protecting plants from pests, diseases, and other environmental adversities can be achieved by building a small greenhouse. Food grown locally supports local agriculture and reduces carbon emissions. The use of greenhouses can contribute to a more resilient and sustainable food system. Depending on the size, location, and complexity of a greenhouse, the cost can vary greatly. To estimate the cost of a greenhouse, the pricing and storage capacity (cu. meters) of 18 greenhouses available on the market have been collected. Costs of greenhouses can vary depending on their size, location, and complexity.
where storage capacity is in cubic meter, refers to how much space plants and other equipment occupy. In addition to the initial cost of the greenhouse, there will be ongoing costs such as heating, cooling, lighting, and maintenance. To estimate the greenhouse's total cost, these costs must be considered.
5 Results
The study employs a scenario-based approach, analyzing three scenarios: food disruption, energy scarcity, and water shortage. The analysis of various scenarios served multiple purposes in the study. Firstly, it allowed the exploration of feasible options aimed at enhancing system resilience. The study of these options provides insights into potential strategies for strengthening the system against unforeseen disruptions. Secondly, the effectiveness of each system when faced with a limited number of disruptions is assessed. It is essential to understand how performance will be affected by such conditions so that robust contingency plans can be developed. In addition, the cost implications of implementing these solutions are examined. This involves identifying the most cost-effective approach and determining the required investment. Addressing questions surrounding cost-effectiveness and investment allocation aims to optimize resource utilization and strategically allocate funds where they are most needed for system enhancement. Each scenario was subjected to detailed analysis and performance of each system was analyzed for 3 days (72 h) disruption. Since the average size of homes in the United States stands at approximately 226 square meters, a single-family residence in Houston, Texas, measuring 223 square meters, was chosen as a case study. The house is the average size of homes in the United States, providing enough space for a comfortable living arrangement for a typical family. The house features a sturdy roof structure and serves as a main factor in various systems, including rainwater collection and solar panels. Its size and orientation determine the potential for harvesting rainwater and capturing solar energy efficiently.
Being located in Houston, Texas, the house receives sunlight throughout the year that can be harnessed for energy generation through solar panels and supports the growth of plants in a greenhouse or hydroponic system. Houston experiences moderate to heavy rainfall, especially during certain seasons. The intensity and frequency of rainfall impact the effectiveness of rainwater collection systems. A higher intensity of rainfall might require larger storage capacity or more efficient drainage systems to prevent overflow and flooding. It is important to note that in different locations, the size and slope of the roof may vary, affecting the efficiency of rainwater collection and solar energy generation. A smaller roof might limit the capacity for rainwater harvesting and reduce the available surface area for solar panels. In regions with less sunlight, such as northern latitudes or areas with frequent cloud cover, solar energy generation would be less efficient compared to a sunny location like Houston. Adjustments to panel orientation or supplementary energy sources might be necessary to compensate for lower sunlight levels. Areas with lower rainfall intensity may require larger roof surfaces or additional water collection infrastructure to meet water demands. Conversely, regions with higher rainfall intensity might need enhanced drainage systems to manage excess water effectively and prevent flooding. The household may rely on a greenhouse or hydroponic system for food production. The effectiveness of these systems during disruptions depends on factors such as sunlight availability and water supply. Contingency plans may involve diversifying food sources or implementing backup systems for indoor farming. Solar energy systems would play a major role in mitigating energy scarcity.
However, variations in sunlight availability and system efficiency due to weather conditions need to be considered. Backup energy sources or energy storage systems may be necessary to maintain essential functions during prolonged disruptions. Rainwater collection systems would be essential for addressing water shortages. The effectiveness of these systems depends on rainfall patterns and storage capacity. During water shortages, conservation measures and alternative water sources such as greywater recycling may need to be implemented to ensure sufficient supply for household needs. However, some systems, such as a chicken garden or hydroponic setup, may exhibit greater resilience to location-specific factors. These systems can be adapted and optimized to suit different environments, mitigating the impact of location variations. Nonetheless, factors like temperature, humidity, and available space still play significant roles in determining their effectiveness and productivity. The characteristics of the selected house in Houston, Texas, influence the feasibility and effectiveness of various resilience systems during disruptions. Adaptations may be required when applying similar strategies to sites in different locations with varying environmental conditions and house sizes. Food disruption, energy scarcity, and water shortage scenarios are discussed in the following sections.
5.1 Scenario 1. Food disruption
The resilience of each food system is evaluated based on its capacity to meet varying levels of household food demand during a 72-h disruption. This includes assessing the ability of hydroponic systems, greenhouses, and chicken gardens to provide essential calories and nutrients, with weights assigned according to dietary needs as outlined in Table 3. The analysis considers scalability, evaluating the extent to which each system can supply 100%, 75%, 50%, and 25% of household food demand under disrupted conditions.
The U.S. Department of Agriculture (USDA) reports that approximately 30%−40% of the national food supply is wasted, equating to around 1,200 calories per person per day (Sun et al., 2022). This level of waste has implications for resource sustainability, as it represents not only lost food but also the energy and water used in food production and distribution. By integrating small-scale, sustainable food systems like hydroponics or home greenhouses, households may reduce their dependency on larger food supply chains, simultaneously lowering food waste. Such systems could play a particularly crucial role during disruptions, as they offer reliable and localized food sources with minimal environmental impact.
In this scenario, food supply with specific small-scale systems, such as hydronic, greenhouse, and chicken garden setups for food disruption were analyzed. The household food intakes in Table 3 are used as the basis for determining the supply of food categories. Subsequently, an analysis is conducted to gauge the scalability of each food system across four levels-fulfilling 100%, 75%, 50%, and 25% of the demand within each food category. The cost for these four scales is computed using the formulas provided in Table 5. Following this, the resilience index is calculated for a duration of 0 < t < 72 h using the specified formula. Figure 3 presents the initial costs of hydronic, greenhouse, and chicken garden setups, along with the cost per 1% improvement in resilience. As depicted in the figure, the initial cost of establishing a greenhouse is a challenge compared to chicken garden and hydroponic systems. The efficiency of hydroponic systems is independent of the system cost, but investing more, it slightly decreases the efficiency (we have to invest more to improve the same percentage of the resilience). The role of the chicken garden is relatively limited due to its sole product being eggs, where increasing egg production does not necessarily enhance resilience. The hydroponic systems can only produce vegetables.
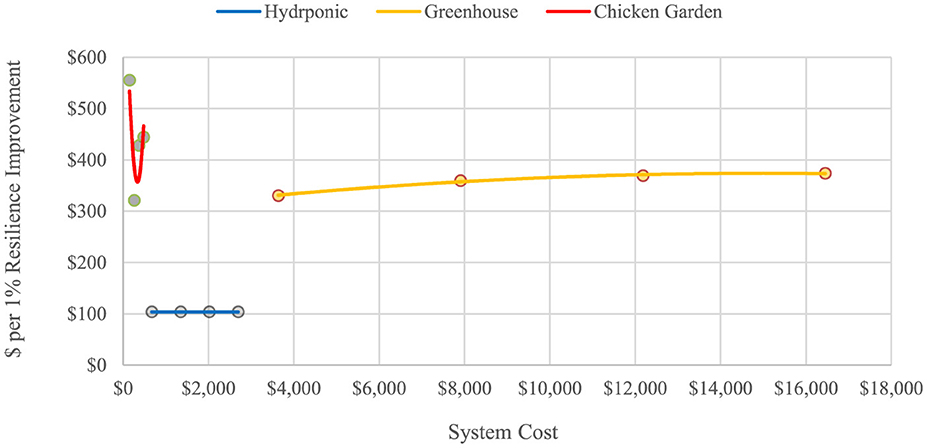
Figure 3. Cost and resilience improvement investments for hydronic, greenhouse, and chicken garden systems.
A comparative analysis of average system cost, average system performance, and the cost per percentage improvement for hydroponic, greenhouse, and chicken garden setups is shown in Figure 4. The hydroponic system demonstrated a moderate average system cost of $1,684, along with an average system performance of 16%. Furthermore, the cost per percentage improvement stands at $104, indicating a reasonable investment requirement for enhancing system performance. In contrast, the greenhouse setup commands a higher average system cost of $10,044, correlating to an average system performance of 28%. However, the cost per percentage improvement is higher at $358, reflecting the considerable financial commitment necessary to achieve incremental performance gains. The chicken garden system exhibits the lowest average system cost at $315, with a modest average system performance of 1%. Moreover, the cost per percentage improvement is high at $437, which indicates a disproportionate investment relative to performance improvement.
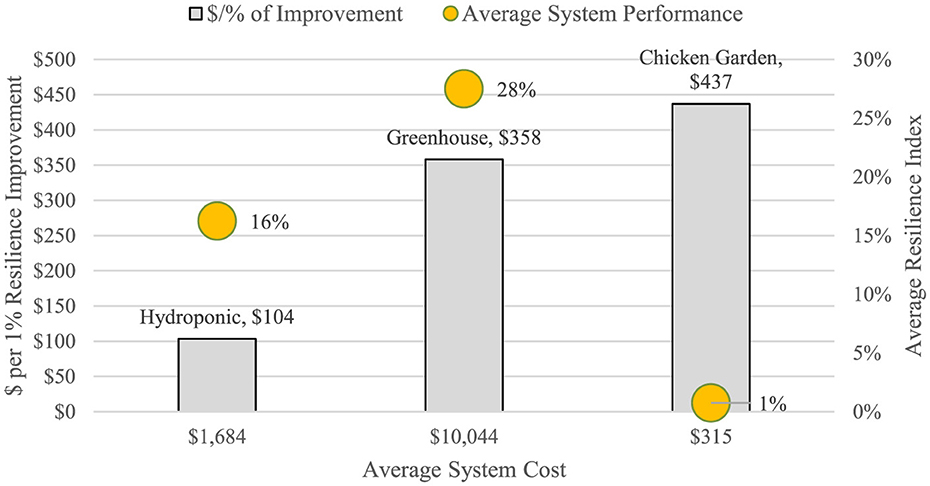
Figure 4. Average system cost, resilience, and average resilience index for hydronic, greenhouse, and chicken garden setup.
5.2 Scenario 2. Energy scarcity
The resilience of each energy system (solar, geothermal, gas-generator, and solar generator) is measured by its ability to consistently power critical household functions (e.g., lighting, HVAC, appliances) during a 3-day outage. Performance metrics consider energy supply continuity, capacity to meet prioritized demand based on time intervals in Table 3, and sustainability of each source under varying conditions. Solar systems are evaluated with assumptions for average daily sunlight, while gas generators are assessed based on fuel supply limitations. In this study, we examined four sources of energy production, solar, geothermal, gas-generator, and solar generator to assess their effectiveness in generating energy during a 3-day disruption in energy supply. The relative weights of energy components in Table 3 serve as metrics for assessing system performance across different scenario analyses. Power supply distribution is then determined based on application priorities and time intervals specified within the table. For instance, initial power allocation prioritizes lighting, with any surplus then directed toward AC/heating, considered secondary in priority. Furthermore, each application is supplied in accordance with its designated time interval, ensuring, for instance, that power demand for appliances is met every 4 h. It is important to highlight that the geothermal system is specifically engineered to deliver heating and cooling services exclusively. As presented in Figure 5, energy to supply 100%, 75%, 50%, and 25% of the demand was considered in each of the sources of energy generator. The assumption used for solar energy involved using the average amount of sunlight per day, but the fluctuations can be more extreme during consecutive cloudy or sunny days throughout the power outage.
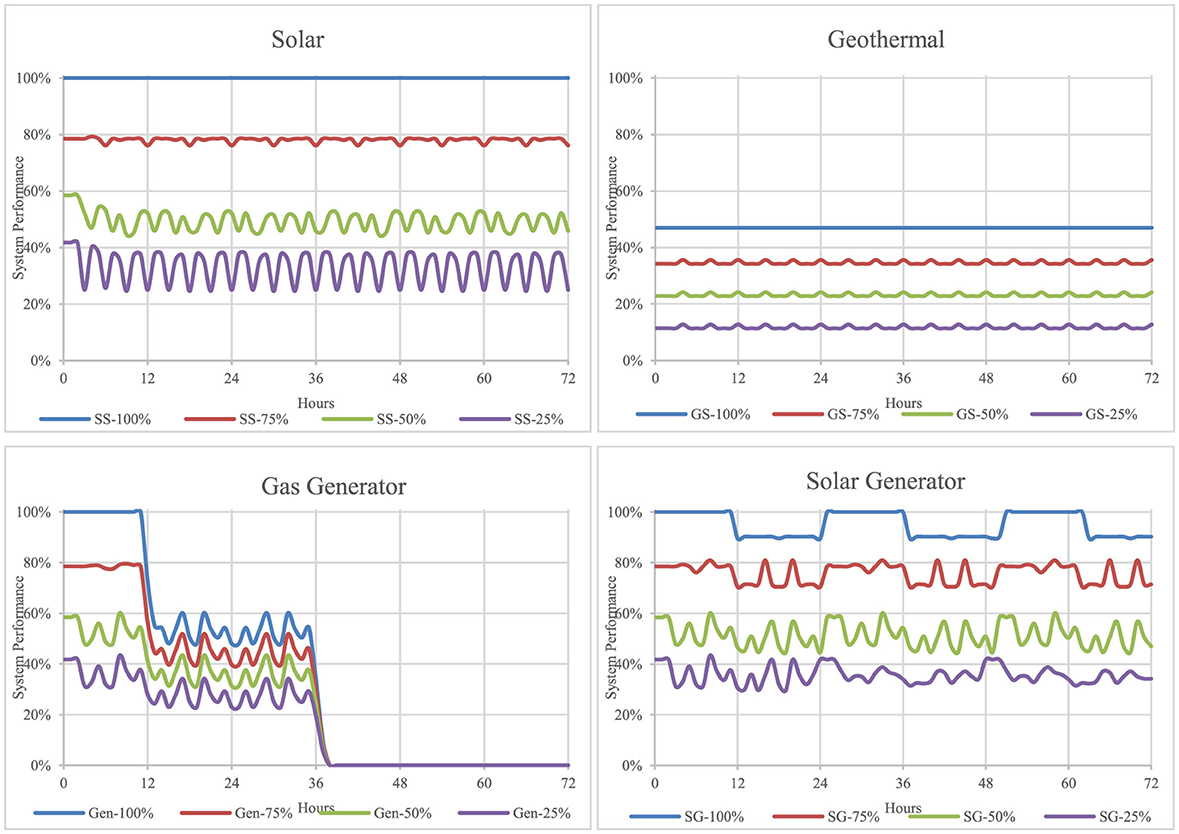
Figure 5. Performance comparison of solar, geothermal, gas-generator, and solar generator systems over 3 days of energy disruption.
Each system is analyzed in order to understand its respective advantages and disadvantages. Solar systems, when combined with a battery to store excess energy generated during the daytime for use at night, function as the most consistent source of energy. This strategy helps mitigate the intermittent nature of solar power, making it a reliable option for continuous energy supply. On the other hand, gas generator systems appear to be the least effective way of producing energy. The analysis indicates gas generators run out of energy after only one and a half days, highlighting their limited capacity and reliance on fuel availability. The battery storage systems for solar energy have the advantage of recharging, but the gas generator is dying after 36 h. Geothermal is the most reliable source of energy, as it is independent of the external factors such as the sunlight.
The increase in the resilience index is rooted in the reduction in the power demand. Although the power supply is decreasing over time, once the peak demand is also reduced, the resilience index can go up. As presented in Figure 6, geothermal systems have the highest average cost of $28,900, followed by solar systems at $22,800, solar generators at $4,621, and gas generators at $2,900. Solar and solar generator systems both have the same performance distribution at 55%, while geothermal systems have a performance distribution of 24%, and gas generators have 21%.
When comparing the cost-effectiveness of resilience enhancement across different systems, solar generators stand out as the most cost-effective option, with the lowest cost per 1% resilience improvement. Despite having a higher average cost, solar systems with battery storage also offer considerable resilience and reliability benefits, making them a valuable investment in the long run. Geothermal systems, while offering significant potential for energy production, exhibit the highest costs for both average resilience enhancement and 1% improvement. This suggests that while geothermal energy may be a promising alternative, it requires substantial investment to enhance its resilience and reliability. Gas generator systems, despite their lower initial cost, prove to be less effective in the long term due to their limited energy supply and reliance on fuel. This highlights the importance of considering not only upfront costs but also the operational effectiveness and sustainability of energy systems when making investment decisions. In summary, while initial cost is an important factor in selecting energy systems, it is equally crucial to consider factors such as performance, resilience, and long-term sustainability. By analyzing both the cost and effectiveness of different energy systems, households can make informed decisions to ensure a reliable and resilient energy infrastructure for their use.
5.3 Scenario 3. Water shortage
Resilience in the water shortage scenario is defined by the ability of rainwater harvesting and sand filtration systems to supply sufficient quantities for prioritized household uses (e.g., drinking, sanitation, and irrigation). Each system is analyzed across demand levels of 100%, 75%, 50%, and 25%, with resilience performance reflecting the percentage of household demand met by stored or treated water. Higher weights are assigned to essential uses like drinking and cooking to reflect their priority in maintaining household resilience.
According to the U.S. Geological Survey (USGS), the average U.S. household consumes about 82 gallons (310 liters) of water per person per day (Marston et al., 2022). However, this rate of consumption can fluctuate widely based on regional climate, with arid states like Nevada and Arizona seeing significantly higher demand. These variations illustrate the critical role that water resilience strategies, such as rainwater harvesting, can play in managing household water needs, especially in drought-prone regions. By capturing and storing rainwater, households in water-scarce areas could reduce their reliance on external sources, thus improving their resilience during periods of water scarcity.
This case scenario explores the effectiveness of two sustainable water systems: rainwater harvesting and sand filtration, implemented in a residential setting facing acute water shortages. The evaluation of these systems is based on their capacity to meet household water demands under varying scenarios of availability and demand. The rainwater harvesting system is designed to capture, store, and utilize rainwater for non-potable needs such as toilet flushing and outdoor water usage. Analysis shows that this system can potentially meet 47% of the total household water demand. Considering the weighted contributions of different water uses, it can achieve up to 29% of the overall system performance. The scenarios analyzed are: 100% demand (fully meets the water requirements for toilet flushing and outdoor uses), 75% demand (three quarters of water needs for the designated uses), 50% demand (fulfills half of the water demand), and 25% demand (covers only a quarter of the required water). The sand filtration system provides a solution for purifying stored rainwater or mildly contaminated municipal water for uses that require cleaner water but not necessarily potable standards, such as car washing and household cleaning. Even when operated at full capacity, the sand filtration system achieves 36% of the system performance. The operational scenarios are similar to those of the rainwater harvesting system, tailored to match varying levels of water purity needs (100%, 75%, 50%, and 25%).
As shown in Figure 7, both systems present cost-effective solutions to the challenge of water scarcity. The rainwater harvesting system costs approximately $70 per 1% improvement in resilience, while the sand filtration system costs about $68 per 1% improvement. This comparative analysis indicates that both systems are financially viable with nearly equivalent costs and benefits, promoting sustainable practices in water-stressed areas.
The results presented above provide critical insights into the performance of household-level FEW systems under various disruption scenarios. The following discussion will analyze these findings, exploring their implications for resilience and sustainability at the household level.
6 Discussion
An integrated FEW nexus approach is considered in this section, where interdependencies and potential disruptions across all three systems simultaneously. This shift in methodology is motivated by the recognition that real-world scenarios often involve disruptions that impact multiple facets of household sustainability. The analysis considers a scenario where the household faces disruptions in food, energy, and water simultaneously. The selected system for this analysis is the house equipped with a solar energy system, a rainwater harvesting system, and a hydroponic food production system. Each of these components was chosen based on consideration of their individual resilience and feasibility characteristics. The solar energy system was selected from the four options introduced in the previous section due to its high resilience index. Specifically, this system demonstrates the capability to improve the overall resilience of the household by 55% in the event of a power outage. The hydroponic food production system was identified as the most feasible option among the three small-scale food systems considered in our previous analysis. Its suitability lies in its capacity to provide a sustainable and reliable source of food even under constrained conditions. Furthermore, the decision to implement a rainwater harvesting system for water supply is rooted in its practicality and effectiveness. By harnessing rooftop rainfall, the household can reduce its reliance on external water sources and increase self-sufficiency in water provision.
In this integrated FEW nexus analysis, it's imperative to note that the priorities for energy and water usage have undergone a strategic shift compared to those outlined in Table 3, which served as the foundation for the assumptions in the previous section. Specifically, in light of the implementation of a hydroponic food production system within the household, the primary focus now centers on meeting the energy and water demands necessary to sustain this system. As such, ensuring an adequate supply of water and energy to support the hydroponic system takes precedence over other household energy and water needs. To provide context, the specific energy and water requirements needed to sustain the hydroponic system in producing and supplying 1.36 kg of vegetables per day are outlined. On average, a hydroponic system requires approximately 0.5 to 1.5 kWh of electricity daily, depending on the specific setup and environmental conditions, and 5.7 to 11.3 liters of water daily, considering the water needs of multiple plants in the system.
Four system configurations within each category are considered: Solar System at 100%, 75%, 50%, and 25% of energy demand (SS-100%, SS-75%, SS-50%, and SS-25%); Hydroponic System at 100%, 75%, 50%, and 25% of vegetable demand; and Harvesting System to Supply 100%, 75%, 50%, and 25% of water demand. The resilience index using the integrated FEW formula and the cost of each system configuration are calculated for all 64 combinations. Based on the findings shown in Figure 8, it is evident that utilizing the 100% water and food systems results in the highest improvements in resilience relative to the cost spent. For instance, opting for a 25% water system (i.e., a harvesting system meeting 25% of water demand for toilet flushing and outdoor usage) instead of a 100% system yields a savings of approximately $1,000 but reduces system resilience by 10%–15%. Similarly, utilizing a 25% food system (i.e., a hydroponic system meeting 25% of vegetable demand) instead of a 100% system saves around $2,000 but decreases system resilience by 12%–15%. However, the solar system notably impacts the resilience of the entire system. Therefore, the chart shown in Figure 8 is designed to focus on four configurations of the solar system. Among the configurations examined, the most cost-effective strategy, with respect to resilience improvement per dollar spent, emerged as the SS-25% system complemented by HS-100% water and HP-100% food systems. This configuration, with an approximate system cost of $16,000, demonstrates expenditure of $507 for achieving a 1% enhancement in system resilience.
Income and location are significant socio-economic factors affecting household-level resilience. Data from the U.S. Census Bureau suggest that higher-income households are more likely to adopt renewable energy solutions, such as rooftop solar panels, due to the initial investment required. In contrast, rural households often have greater access to land, which may facilitate the installation of rainwater harvesting systems or small greenhouses for food production. Recognizing these socio-economic disparities is essential for developing equitable resilience strategies. Policy initiatives targeting financial support for lower-income households could promote the broader adoption of FEW resilience measures across diverse communities.
7 Recommendations for policymakers and future research
Policymakers can use the findings of this study to promote decentralized resource systems at the household level. In particular:
• Incentives for households to adopt renewable energy sources, such as rooftop solar panels and solar-powered generators, could increase resilience to power outages. Policymakers could develop targeted financial programs, such as low-interest loans or grants, for installing renewable energy systems.
• Rainwater harvesting and small-scale hydroponic food production systems should be encouraged through policy measures, particularly in regions vulnerable to climate-related disasters. A good practical example is the City of Austin's Rain Catcher Pilot Program, which promotes Green Stormwater Infrastructure (GSI) by offering discounts, rebates, and educational resources to encourage the use of cisterns and rain gardens. This program aims to enhance stormwater management and water conservation, supporting resilience at the household level through community engagement and incentives.
• Regulatory frameworks should integrate household-level sustainability measures to reduce the dependency on centralized systems, which are often more vulnerable to large-scale disruptions. Local and state regulations could include standards for FEW resilience, such as mandating water storage capacity in drought-prone regions or backup energy requirements in areas with frequent outages. Developing a certification or resilience rating for households that meet these standards could further incentivize compliance.
• Policies could also promote community-level resilience by supporting shared resources, such as community gardens, food storage facilities, and neighborhood-level microgrids that allow households to share surplus resources during disruptions.
Future research should explore the applicability of household-level FEW systems in different socio-economic and climatic contexts. Studies focusing on low-income households, rural areas, and regions with limited infrastructure could provide deeper insights into the scalability and effectiveness of these systems. Additionally, long-term cost-benefit analyses should be conducted to assess the economic viability of these solutions, particularly in regions with frequent natural disasters. While this study focuses on household-level FEW systems in Texas and California, USA, the principles and findings can be adapted to other regions with similar disaster profiles. However, the feasibility and cost-effectiveness of the proposed systems may vary depending on local infrastructure, environmental conditions, and socio-economic factors.
8 Conclusions
This study has critically evaluated the resilience and sustainability of various household-level Food-Energy-Water (FEW) systems in mitigating the impacts of natural disasters, exacerbated by climate change. Through the examination of three primary scenarios—food disruption, energy scarcity, and water shortage—this research underscores the effectiveness of integrated solutions such as rainwater harvesting, sand filtration, solar and geothermal energy, gas and solar generators, hydroponic systems, and small greenhouses. These systems demonstrate significant potential to sustain household functionality during disruptions, offering a diverse range of benefits from increased resilience to enhanced sustainability. The analysis reveals that while no single system offers a complete solution, the strategic combination of these technologies can substantially improve household resilience. Rainwater harvesting and sand filtration systems prove essential for water sustainability, while solar and geothermal systems offer reliable energy solutions. Moreover, hydroponic and greenhouse setups ensure food security under varied conditions. The scenario-based approach utilized in this study has highlighted the crucial role of adaptability and system integration in enhancing the resilience of household FEW systems.
Key findings show that gas-powered generators can provide essential backup power for up to 6 h, supplying 3.5 kWh of energy for critical appliances such as refrigeration and lighting. Portable generators emerged as the most cost-effective small-scale system for improving household resilience, with an investment of approximately $100 yielding a 1% increase in resilience. Although they offer a quick fix, solar generators are preferred for those seeking a more sustainable option. Rooftop solar panels were found to meet 40%−50% of household energy needs under optimal conditions, though their reliance on weather conditions highlights the need for backup systems.
In the food sector, the chicken garden system, despite being the least expensive, offers a minimal resilience improvement of only 1%. In contrast, hydroponic systems present a more robust option, achieving up to 16% resilience with a relative cost of $100 per 1% improvement. This highlights hydroponics as a viable alternative for substantial resilience enhancement at a reasonable cost. Regarding water systems, both rainwater harvesting and sand filtration exhibited comparable performances in bolstering resilience. However, when adopting a comprehensive FEW nexus approach, it becomes clear that maximizing the operational capacity of water and food systems, specifically, fully meeting the water demand for toilet flushing and outdoor uses, along with maximizing food production via hydroponics, results in the most significant improvements in resilience relative to the investment made.
The practical applications of these findings suggest that integrating decentralized FEW systems can significantly enhance household resilience, particularly in disaster-prone areas. Policymakers should consider incentivizing the adoption of these systems through subsidies and building code updates. In conclusion, the development of robust, integrated FEW systems at the household level is imperative for reducing vulnerability to environmental stresses and fostering a resilient infrastructure. Future research should continue to refine these systems, focusing on cost-effectiveness and performance optimization to facilitate widespread adoption and implementation. They should also explore the scalability of these systems and their long-term cost-effectiveness, particularly in regions with limited resources. This study provides a valuable framework for evaluating household-level FEW resilience, offering insights for both researchers and policymakers seeking to improve community sustainability and disaster preparedness. As global environmental challenges intensify, the resilience of local systems will be paramount in safeguarding the wellbeing of communities worldwide.
Data availability statement
The raw data supporting the conclusions of this article will be made available by the authors, without undue reservation.
Author contributions
EK: Writing – original draft, Writing – review & editing. AM: Writing – original draft, Writing – review & editing.
Funding
The author(s) declare that no financial support was received for the research, authorship, and/or publication of this article.
Conflict of interest
The authors declare that the research was conducted in the absence of any commercial or financial relationships that could be construed as a potential conflict of interest.
Publisher's note
All claims expressed in this article are solely those of the authors and do not necessarily represent those of their affiliated organizations, or those of the publisher, the editors and the reviewers. Any product that may be evaluated in this article, or claim that may be made by its manufacturer, is not guaranteed or endorsed by the publisher.
References
Ahuja, S. (2021). “Chapter 2 - Water quality worldwide,” in Handbook of Water Purity and Quality (Second Edition), ed. S. Ahuja (Amsterdam: Academic Press). doi: 10.1016/B978-0-12-821057-4.00003-3
Asaki, F. A., Oteng-Abayie, E. F., and Baajike, F. B. (2024). Effects of water, energy, and food security on household well-being. PLoS ONE 19:e0307017. doi: 10.1371/journal.pone.0307017
Ballester, J., Quijal-Zamorano, M., Méndez Turrubiates, R. F., Pegenaute, F., Herrmann, F. R., Robine, J. M., et al. (2023). Heat-related mortality in Europe during the summer of 2022. Nat. Med. 29, 1857–1866. doi: 10.1038/s41591-023-02419-z
Blackwood, J., Karan, E., Asadi, S., Mohammadpour, A., and Asgari, S. (2017). “Stochastic optimization model for sustainable water treatment with minimal energy use,” in Computing in Civil Engineering, 2017, 215–222. doi: 10.1061/9780784480847.027
Chen, B., Han, M. Y., Peng, K., Zhou, S. L., Shao, L., Wu, X. F., et al. (2018). Global land-water nexus: agricultural land and freshwater use embodied in worldwide supply chains. Sci. Total Environ. 613, 931–943. doi: 10.1016/j.scitotenv.2017.09.138
Crouch, M. L., Jacobs, H. E., and Speight, V. L. (2021). Defining domestic water consumption based on personal water use activities. AQUA—Water Infrastr. Ecosyst. Soc. 70, 1002–1011. doi: 10.2166/aqua.2021.056
Dargin, J., Berk, A., and Mostafavi, A. (2020). Assessment of household-level food-energy-water nexus vulnerability during disasters. Sustain. Cities Soc. 62:102366. doi: 10.1016/j.scs.2020.102366
Dieter, C. A., Maupin, M. A., Caldwell, R. R., Harris, M. A., Ivahnenko, T. I., Lovelace, J. K., et al. (2018). Estimated use of water in the United States in 2015. US Geological Survey. doi: 10.3133/cir1441
EIA (2017). Energy consumption estimates by sector. Monthly Energy Review. U.S. Energy Information Administration (EIA).
EIA (2020). Residential Energy Consumption Survey (RECS) [Online]. Washington, DC: U.S. Energy Information Administration. Available at: https://www.eia.gov/consumption/residential/data/2020/ (accessed November 25, 2022).
Ekasiwi, S. N. N., Majid, N. H. A., Hokoi, S., Oka, D., Takagi, N., and Uno, T. (2013). Field survey of air conditioner temperature settings in hot, humid climates, part 1: Questionnaire results on use of air conditioners in houses during sleep. J. Asian Archit. Build. Eng. 12, 141–148. doi: 10.3130/jaabe.12.141
González-Torres, M., Pérez-Lombard, L., Coronel, J. F., Maestre, I. R., and Yan, D. (2022). A review on buildings energy information: Trends, end-uses, fuels and drivers. Energy Rep. 8, 626–637. doi: 10.1016/j.egyr.2021.11.280
Hertel, T., Elouafi, I., Tanticharoen, M., and Ewert, F. (2021). Diversification for enhanced food systems resilience. Nature Food 2, 832–834. doi: 10.1038/s43016-021-00403-9
Iqbal, A. (2022). Post-Disaster Needs Assessment - Pakistan Floods 2022. Minister for Planning, Development and Special Initiatives.
Itayi, C. L., Mohan, G., and Saito, O. (2021). Understanding the conceptual frameworks and methods of the food–energy–water nexus at the household level for development-oriented policy support: a systematic review. Environ. Res. Lett. 16:033006. doi: 10.1088/1748-9326/abd660
Karan, E., and Asadi, S. (2018). Quantitative modeling of interconnections associated with sustainable food, energy and water (FEW) systems. J. Clean. Prod. 200, 86–99. doi: 10.1016/j.jclepro.2018.07.275
Karan, E., Asadi, S., Mohtar, R., and Baawain, M. (2018). Towards the optimization of sustainable food-energy-water systems: a stochastic approach. J. Clean. Prod. 171, 662–674. doi: 10.1016/j.jclepro.2017.10.051
Kennedy, G., Ballard, T., and Dop, M. C. (2011). Guidelines for measuring household and individual dietary diversity. Food and Agriculture Organization of the United Nations.
Kumar, A., Sood, Y. R., and Maheshwari, A. (2024). “Power systems resilience enhancement through renewable energy integration: Insights and future directions,” in 2024 IEEE 4th International Conference in Power Engineering Applications (ICPEA) (IEEE), 140–145. doi: 10.1109/ICPEA60617.2024.10498721
Marston, L. T., Abdallah, A. M., Bagstad, K. J., Dickson, K., Glynn, P., Larsen, S. G., et al. (2022). Water-use data in the United States: challenges and future directions. JAWRA 58, 485–495. doi: 10.1111/1752-1688.13004
Masao, K. (2024). Oceanic oscillations and their influence on regional climate variability in Japan. Am. J. Clim. Stud. 4, 32–42. doi: 10.47672/ajcs.2037
Meng, X., Gao, Y., Hou, C., and Yuan, F. (2019). Questionnaire survey on the summer air-conditioning use behaviour of occupants in residences and office buildings of China. Indoor Built Environ. 28, 711–724. doi: 10.1177/1420326X18793699
NOAA (2024). U.S. Billion-Dollar Weather and Climate Disasters [Online]. National Oceanic and Atmospheric Administration (NOAA): National Centers for Environmental Information (NCEI). Available at: https://www.ncei.noaa.gov/access/billions/state-summary/US (accessed February 25, 2024).
O'Conner, K., Cantwell, J., Wilson, M., Bolles, S., Schmidt, A., and Burgess, J. (2010). Evaluation of Energy Conservation Measures for Wastewater Treatment Facilities. Washington, DC, USA: EPA.
Scholes, R. J. (2020). The future of semi-arid regions: a weak fabric unravels. Climate 8:43. doi: 10.3390/cli8030043
Shahbaz, P., Ul Haq, S., Abbas, A., Samie, A., Boz, I., Bagadeem, S., et al. (2022). Food, energy, and water nexus at household level: do sustainable household consumption practices promote cleaner environment? Int. J. Environ. Res. Public Health 19:12945. doi: 10.3390/ijerph191912945
Sun, H., Sun, Y., Jin, M., Ripp, S. A., Sayler, G. S., and Zhuang, J. (2022). Domestic plant food loss and waste in the United States: Environmental footprints and mitigation strategies. Waste Manag. 150, 202–207. doi: 10.1016/j.wasman.2022.07.006
U.S. Census Bureau (2022). Current Population Survey (CPS) [Online]. U.S. Bureau of Labor Statistics. Available at: https://www.census.gov/programs-surveys/cps.html (accessed November 25, 2022).
USDA (2015). 2015–2020 Dietary Guidelines for Americans. 8th Edition. US Department of Agriculture (USDA).
Wa'el, A. H., Memon, F. A., and Savic, D. A. (2017). An integrated model to evaluate water-energy-food nexus at a household scale. Environ. Model. Softw. 93, 366–380. doi: 10.1016/j.envsoft.2017.03.034
Wa'el, A. H., Memon, F. A., and Savic, D. A. (2018). A risk-based assessment of the household water-energy-food nexus under the impact of seasonal variability. J. Clean. Prod. 171, 1275–1289. doi: 10.1016/j.jclepro.2017.10.094
Walker, B., Holling, C. S., Carpenter, S. R., and Kinzig, A. (2004). Resilience, adaptability and transformability in social–ecological systems. Ecol. Soc. 9:5. doi: 10.5751/ES-00650-090205
Xue, J., Liu, G., Cristiano, S., Casazza, M., Nawab, A., Ghisellini, P., et al. (2021). Development of an urban household food-energy-water policy nexus dynamic simulator. J. Clean. Prod. 328:129521. doi: 10.1016/j.jclepro.2021.129521
Keywords: household resilience, sustainable FEW systems, climate change adaptation, integrated nexus approach, natural disaster mitigation
Citation: Karan E and Mohammadpour A (2024) Disaster resilience of household-level food-energy-water nexus. Front. Sustain. Resour. Manag. 3:1433447. doi: 10.3389/fsrma.2024.1433447
Received: 15 May 2024; Accepted: 08 November 2024;
Published: 03 December 2024.
Edited by:
Gabriel Faimau, University of Botswana, BotswanaReviewed by:
Hourakhsh Ahmadnia, Alanya University, TürkiyeDimitrios Pappas, Queen's University Belfast, United Kingdom
Copyright © 2024 Karan and Mohammadpour. This is an open-access article distributed under the terms of the Creative Commons Attribution License (CC BY). The use, distribution or reproduction in other forums is permitted, provided the original author(s) and the copyright owner(s) are credited and that the original publication in this journal is cited, in accordance with accepted academic practice. No use, distribution or reproduction is permitted which does not comply with these terms.
*Correspondence: Ebrahim Karan, ZXBrMDA4QHNoc3UuZWR1