- 1Environmental Physiology Laboratory, The University of British Columbia, School of Kinesiology, Vancouver, BC, Canada
- 2Department of Biomedical Physiology and Kinesiology and Sports Analytics Group, Simon Fraser University, Burnaby, BC, Canada
- 3Department of Physical Therapy, The University of British Columbia, Vancouver, BC, Canada
- 4Centre for Heart Lung Innovation, Providence Research, The University of British Columbia and St. Paul’s Hospital, Vancouver, BC, Canada
- 5Vancouver Whitecaps FC, Vancouver, BC, Canada
- 6Division of Sport & Exercise Medicine, The University of British Columbia, Vancouver, BC, Canada
Objective: Minimum muscle oxygen saturation (SmO2min) measured via near-infrared spectroscopy (NIRS) is a common measure during incremental exercise testing (IET). Our objective was to determine the effects of pre-to-post endurance training on SmO2min (ΔSmO2min) during an IET, using a meta-analysis.
Data sources: MEDLINE, EMBASE, and SPORTDiscus.
Study selection: Studies including healthy individuals had to meet the following criteria: (1) endurance training intervention; (2) peripheral muscle NIRS; (3) incremental exercise test pre/post training; (4) SmO2 or analogous saturation parameter measured.
Analysis: A PEDro scale was used for risk of bias analysis. A random effect meta-analysis model was used to synthesize the effect of training on ΔSmO2min in individual studies. Statistical heterogeneity was quantified using I2 statistic. A meta-regression was used to estimate the effect of training on the relationship between peak cycling power output (Wpeak), peak pulmonary oxygen uptake (V˙O2peak), and ΔSmO2min. A mixed-effect model was used to estimate categorical variables.
Results: Five studies met the inclusion criteria. No difference in SmO2min was detected following training pre- and post-intervention IETs. A trend for an effect of training on the relationship between Wpeak and ΔSmO2min was observed (p = 0.06).
Conclusion: This meta-analysis showed no effects of endurance training on SmO2min during an IET. Our results showed a trend for an effect of training on the relationship between Wpeak and ΔSmO2min, with no effect for V˙O2peak and ΔSmO2min. It is possible that SmO2min is not affected by endurance training, and may be used as a physiological marker for improvements in submaximal performance rather than at peak.
Introduction
In healthy individuals, exercising at severe and extreme intensities can cause muscle oxygen demand to surpass convective oxygen delivery, thus causing a mismatch between muscle oxygen demand and supply (1). Following endurance training, physiological adaptations occur both for systemic oxygen delivery and muscle oxygen extraction and utilization, reducing the oxygen mismatch and improving overall athletic performance (2–4). Such improvements from pre- to post-training are often assessed by measuring the intensity-dependent response of various physiological and performance measures during incremental exercise tests (IET).
During an IET, a gold standard measurement of such physiological responses is systemic oxygen consumption (V˙O2), which demonstrates an intensity-dependent increase until peak V˙O2 is attained (V˙O2peak) (5). Conversely, another physiological measure is muscle oxygen saturation (SmO2), measured at the primary locomotor muscle using near-infrared spectroscopy (NIRS), which typically follows a non-linear but generally continuous desaturation (i.e., decreasing oxygen saturation) response until maximal task tolerance where a minimum SmO2 (SmO2min) is observed (6–8). Near-infrared spectroscopy has been used alongside peak cycling power output (Wpeak) and V˙O2peak during IET to describe and investigate local/peripheral metabolic responses alongside systemic physiological responses and performance outcomes (7–10). Maximal task tolerance during an IET is associated with the attainment of V˙O2peak and Wpeak (11, 12). Both measures have been shown to increase with endurance training (13). Muscle oxygen saturation following endurance training has been investigated in relation to these markers, showing a greater desaturation to a lower SmO2min at maximal task tolerance, coincident with a rise in V˙O2peak and performance (14). The integration of NIRS during an IET has increased in popularity within the last 20 years. As such, it will be valuable to systematically review the existing literature and quantify the effects of endurance training interventions on SmO2 during an IET.
The primary signals measured via NIRS are the relative concentration of oxygenated and deoxygenated hemoglobin and myoglobin (O2HbMb and HHbMb, respectively), and their sum (tHbMb) (15). The relative portion of O2HbMb from tHbMb is a measure of muscle oxygen saturation (SmO2). SmO2 may be estimated from different methods depending on the specific NIRS technology (15, 16). The saturation parameter may alternatively be called muscle or tissue oxygen saturation, saturation index, or oxygenation index, which reflect slightly different models of how the NIRS signal interacts with the tissue volume under illumination (15). However, the response profiles during an incremental exercise test (IET) are broadly comparable, so for clarity, the term SmO2 will be used throughout this manuscript.
For athletes, coaches, and sports scientists, the primary benefit of measuring SmO2 response during exercise is the ability to observe muscle metabolic responses within locomotor muscles. This aids in tactical decision-making during exercise, quantifying the relationship between exercise demands and internal muscle perturbation, and providing more information about muscular adaptations to training. Several studies reported breakpoints in the NIRS signal during an IET, relating them to other physiological thresholds such as lactate and ventilatory thresholds (9, 17, 18). Others have looked at SmO2min, V˙O2peak, and Wpeak during IETs to identify the effects of endurance training interventions on muscle metabolic responses over time (19–23). For practitioners, these reports highlight the benefit of NIRS as a non-invasive measure in estimating the effects of training on SmO2, especially with the evolution of commercially available wearable NIRS technology (24, 25). For our purposes, reviewing and quantifying the existing body of knowledge concerning the effects of endurance training on SmO2min during IETs, will address an important question: what change, if any, will SmO2min present following an endurance training intervention during an IET.
As such, in the context of this review, endurance training was defined as an aerobic exercise regimen that lasted for at least 2 weeks, with a minimum of two sessions per week that were composed of continuous modes of exercise (26). Strength exercise regimes were not included unless completed in addition to endurance training as previously defined. Endurance training interventions often include either continuous training sessions or interval training sessions, or a combination of the two (27, 28).
Purpose
From previous studies evaluating changes across different SmO2 signals following endurance training interventions, we hypothesized that endurance training would induce greater desaturation to a lower SmO2min at maximal task tolerance during an IET. As for the effects of training on the change in SmO2min relative to Wpeak and V˙O2peak, we hypothesized that both Wpeak and V˙O2peak would increase with training and that a lower SmO2min would be correlated with higher post-training Wpeak and V˙O2peak. The aim of this meta-analysis was to provide an estimate of the effects of endurance training on SmO2min during an IET in healthy participants.
Methods
The design of the review was based on Preferred Reporting Items for Systematic Reviews and Meta-Analysis (PRISMA), following the 2020 guidelines for new systematic reviews and the Cochrane Handbook for Systematic Reviews of Interventions version 6.3 (29) which included searches of databases, registers, and other sources (30).
Identification of studies search strategy
The online databases MEDLINE (OVID), EMBASE (OVID), and SPORTDiscus (EBSCOHost) were searched for published, full-text articles in English up to and including July 2024. The keywords used to identify relevant studies can be found in Supplementary 1. Further records were identified by manual citation searching through additional online searches. The inclusion criteria were as follows: (1) were in English; (2) endurance training intervention (31); peripheral muscle NIRS (32); IET performed pre/post training (33); SmO2 or analogous saturation parameter measured. Exclusion criteria were as follows: (1) No clinical populations were included in the analysis, but clinical studies that had healthy controls with an endurance training intervention were included, with only the control data included in the extraction; (2). Studies were excluded if they had with no measure of muscle oxygen saturation or provided no data that allowed for calculation of saturation.
If reports included partial data, respective authors were contacted to provide additional data by the lead author (AY). If no response was received, a follow-up email was sent, and studies were excluded if no data were provided after 30 days. In the case where necessary data were only presented in a graphical format, PlotDigitizer (https://plotdigitizer.com, 3rd version, August 2022) was used to extract relevant values.
All records screened independently using Covidence systematic review software, Veritas Health Innovation, Melbourne, Australia, for eligibility by two authors (AY, JA, or HN), any disagreements were discussed and evaluated by the group (AY, JA, HN, and MK). Similarly, reports assessed for eligibility were independently reviewed by two authors (AY, and HN) and reason for exclusion was decided collaboratively (AY, and HN).
Data extraction
Data were initially extracted for a systematic review from reports assessed for eligibility by three authors (AY, HN, and JA) (Figure 1). However, for the five papers included in the quantitative synthesis, further data extraction and analysis was conducted by AY, HN, and MR.
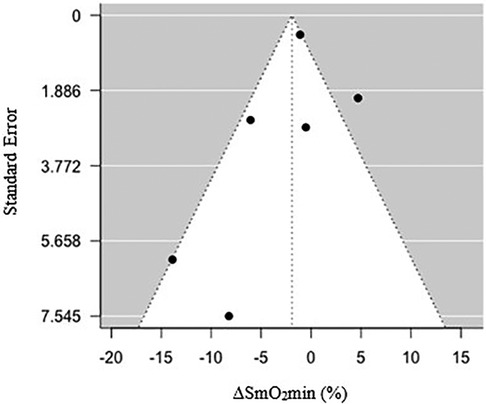
Figure 1. Funnel plot of standard error relative to a percentage change in minimum muscle oxygen saturation (ΔSmO2min) (%) following endurance training.
The extracted data from each included article were: participant characteristics (sample size, sex, age, body mass, and BMI), intervention (exercise type, exercise mode, duration of training, frequency of training, and number of sessions), NIRS (device, baseline IET SmO2 pre-training, IET maximal task tolerance SmO2 pre-training, baseline IET SmO2 post-training, IET maximal task tolerance SmO2 post-training), Wpeak (IET Wpeak pre-training, IET Wpeak post-training), V˙O2peak (IET V˙O2peak pre-training, IET V˙O2peak post-training). Baseline IET SmO2 (%) showed no significant differences pre- to post-training in any of the included studies. Since there were no baseline differences, we normalized baseline SmO2 to 100% and differences from baseline during exercise were reflected as a decrease from 100% to the SmO2min (i.e., the lower the SmO2min value the more oxygen desaturated). To estimate the magnitude and direction of change in the standardized SmO2min between pre-training and post-training, the standardized SmO2min values were subtracted from each other to yield the change (ΔSmO2min). For Wpeak and V˙O2peak, if a p-value was not provided for change following training, it was estimated using the means and standard deviation (SD) from pre-training to post-training. When IET was longer than 12 min, a correction factor was used to standardize Wpeak (34).
Risk of bias analysis
Risk of bias for individual studies was evaluated using the PEDro scale and the completed scale can be found in Supplementary 1. Two reviewers independently assessed studies for bias (AY and HN), and any disagreements were resolved by the reviewers and a third author (MK).
Statistical analysis
Data analysis and statistical analysis were carried out in R (v4.1.2, R Foundation for Statistical Computing, Vienna, Austria). Group data were reported as means and SDs. The effect measure of the continuous primary outcome variable (ΔSmO2min) was presented as the standardized mean difference (SMD) and its 95% confidence intervals for pooled data, following standardization of the data as described above. When SD was not provided, standard error of the mean (SE) was converted using the following formula: . When SE was not reported, the p-value was used to estimate the SD using the following formula: . To provide a conservative estimate of the SD, a p-value expressed as an inequality (i.e., “<”) was changed to an equality (i.e., “=”) (27). A forest plot was produced to visually display the quantitative results; a random effect meta-analysis model (DerSimonian-Laird random effects method) was used to synthesize the individual studies (31). Statistical heterogeneity was quantified using the I2 statistic. I2 values of 25%, 50%, and 75% were considered as low, moderate, and high degrees of statistical heterogeneity (35). To determine the relationship between the effect size and the sample size, a funnel plot was used. An Egger's Test was used to account for any small sample size bias (36).
We conducted 5 subgroup analyses to estimate the effect of endurance training on ΔSmO2min in relation to the following modifiers: continuous variables (Wpeak and V˙O2peak) and categorical variables (sex, training type, and exercise mode). The expected effect of endurance training on the continuous variables is an increase, which was explored using meta-regressions and visually displayed as bubble plots. For these variables, the data were converted to a percentage change from pre-training to post-training. Categorical variables of the retrieved studies were evaluated based on sex (female or male), training type (continuous and interval exercise), and mode (leg cycling, combined arm and leg cycling, and unsupervised training). They were analyzed using a mixed-effects model to estimate an effect of endurance training on the relationship between ΔSmO2min and each of the categorial variables separately.
Results
Descriptive data
A combined total of 869 records were identified in the search prior to the removal of 366 duplicates by an automatic review application (Covidence systematic review software, Veritas Health Innovation, Melbourne, Australia) (Figure 1). After title and abstract screening, 25 reports were retrieved for full-text text review. One could not be retrieved for full text (37). Nineteen papers were excluded at the full text review stage. Eleven were excluded due to exercise protocol used (32, 38–47). Eight were excluded due to ineligible reported outcome measures (33, 48–54). Identification of studies via other methods yielded six studies. Five were ineligible due to exercise protocol used (55–59). Five studies were included in the final quantitative analysis (19–23). Zinner et al. had two eligible groups from the same study, meaning that 6 groups were present in the analysis (23).
A total of 58 participants were included (22 females, age = 26.7 ± 4.0), all were either sedentary or recreationally active, healthy individuals (Table 1) (60). The participants from Rissanen, et al. (21), were a healthy group used as control for a larger study on diabetic patients. Training type included continuous training (n = 17), and interval training (n = 41). Exercise mode included cycling (n = 40), arm and leg cycling exercise (n = 10), and unsupervised training (i.e., no reporting of exercise mode) (n = 8).
The completed PEDro scores for the included studies can be found in Supplementary 1. No form of blinding was used in any of the included studies. All studies included were sufficiently similar at baseline and used measures of point estimates and variability for the primary outcome measure. The included participant data from Rissanen et al., was a control healthy group from a larger clinical study, and five participants were excluded post hoc from the control to match the clinical group in anthropometric and V˙O2peak data (21). The data were excluded both at baseline and post-training and did not affect the comparison. Therefore, there was not sufficient follow-up for those initially allocated to the training intervention.
For individual studies, the effect of endurance training on SmO2min was presented as means and SDs in Table 2, and as effect measures and confidence intervals in Figure 2.
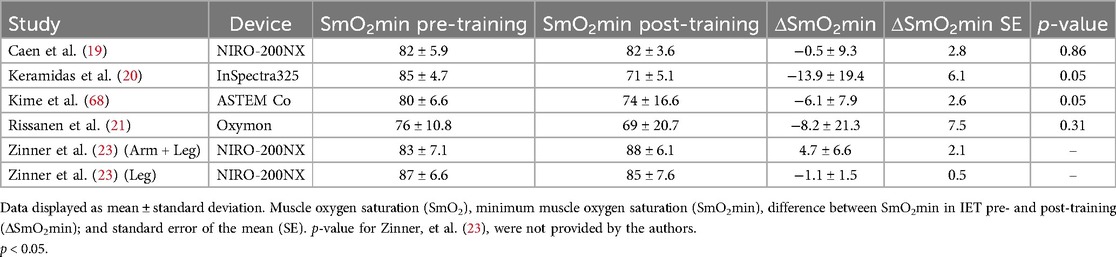
Table 2. The effects of endurance training on SmO2min pre- and post-training at maximal task tolerance during an incremental exercise test when baseline SmO2 is standardized to 100%.
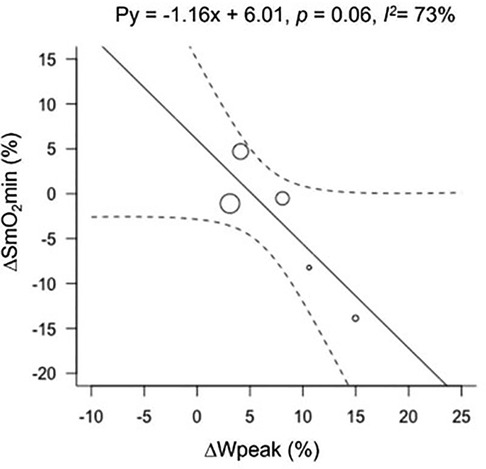
Figure 2. Bubble plot for ΔSmO2min with ΔWpeak as a modifier. Change in muscle oxygen saturation minimum (ΔSmO2min), and peak power output (ΔWpeak).
No significant effect of endurance training on ΔSmO2min was observed. The pooled effect size was −1.92 (95% CI = −5.56 to 1.72, p < 0.3), indicating no effect of training on ΔSmO2min. There was a high degree of heterogeneity in the pooled results (I2 = 70%) for changes in ΔSmO2min. To investigate the source of heterogeneity in ΔSmO2min, we considered all the variables that were extracted. However, a meta-regression was done on Wpeak and V˙O2peak only due to their relevance to our question.
Meta-regression analyses
For analyses relating to Wpeak, only 4 studies and 5 groups were included; Kime et al. 68) did not report any measures of power. The effect of endurance training on the relationship between Wpeak and ΔSmO2min resulted in a tendency for SmO2 to be lower with increased Wpeak (Figure 3). The effect of training on Wpeak showed a significant improvement from 286 ± 45 watts (W) to 308 ± 53 W (p < 0.01). The heterogeneity for ΔSmO2min increased (I2 = 73%) when grouped with Wpeak. Peak power output data can be found in Supplementary 1.
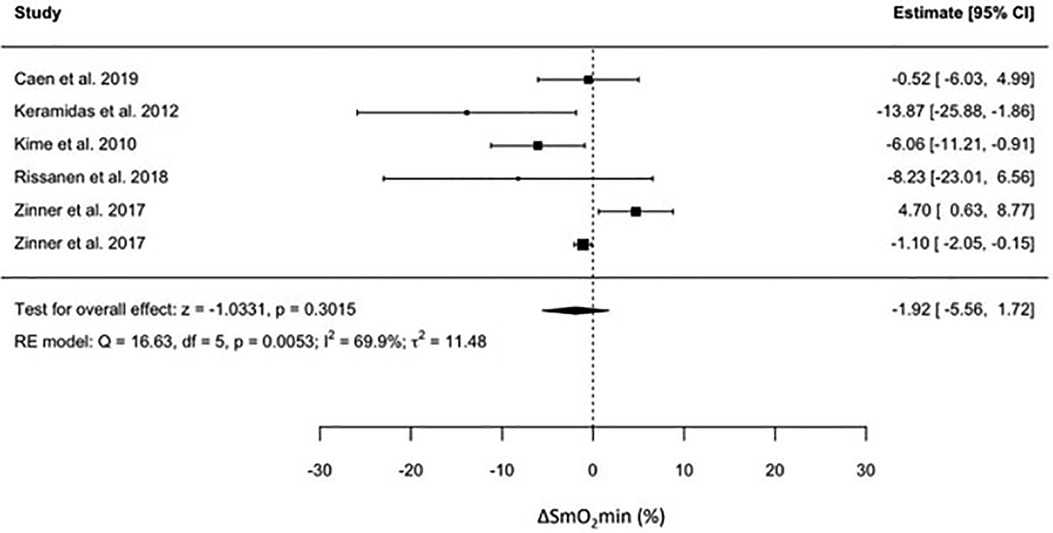
Figure 3. Forest plot of the effect of endurance training on the change in minimum muscle oxygen saturation across the included studies.
The relationship between V˙O2peak and ΔSmO2min showed no effect of training. Keramidas et al. (20) did not state a p-value for V˙O2peak for the difference between pre-training to post-training. As such, we were unable to calculate the SD of the difference in V˙O2peak between the two time points, and it was excluded from the meta-regression. The heterogeneity for ΔSmO2min slightly decreased but remained moderate (I2 = 68%) when grouped with V˙O2peak. V˙O2peak (ml · kg−1 · min−1) values from individual studies can be found in the Supplementary 1.
Stratified analyses
Our analysis detected no effect of training on ΔSmO2min with regards to sex reported for participants (female or male), training type (continuous or interval), and exercise mode (cycling, arm and leg cycling, or unsupervised training). The heterogeneity for all stratified analyses increased from moderate to high, suggesting that these characteristics would likely not influence the change in ΔSmO2min following a training program. For sex, training type, and exercise mode, the I2 increased to 93%, 91%, and 90%, respectively. A summary table of the results, including the p-value for interactions for each of the modifiers, can be found in Supplementary 1.
Between-study bias assessment
Visual inspection of a funnel plot (Figure 4) observing changes in ΔSmO2min yielded no signs of asymmetry. Additionally, an Egger's test was used to confirm the lack of small sample size bias and test for funnel plot asymmetry (z = −1.55, CI = −5.07 to 9.74, and p = 0.12) (36).
Discussion
The aim of our systematic review and meta-analysis was to investigate the effect of endurance training on SmO2min and its relationship with any changes in Wpeak and V˙O2peak. From our results, we rejected our hypothesis that a greater SmO2 desaturation to a lower SmO2min, would occur during an IET following endurance training. Three studies demonstrated either a high degree of variance in their results (20, 21), or a higher absolute SmO2min, and a reduced ΔSmO2min during an IET following training (23). Rissanen et al. (21), included a year-long, unsupervised training intervention, resulting in greater variance in SmO2min relative to studies with a controlled training program. Zinner et al., was the only study that included a group with both arm and leg cycling training and was the only study demonstrating an increase in SmO2min (i.e., less desaturation) measured at the quadricep at the end of an IET following training (23). Omitting this subgroup from the analysis did not change the results for ΔSmO2min. Lastly, Keramidas et al., showed a large CI; however, performance as measured by V˙O2peak did not significantly change from pre-training (38.0 ± 5.5 ml · kg−1 · min−1) to post-training (36.4 ± 4.3 ml · kg−1 · min−1) (20). This finding contrasts with their Wpeak results that significantly increased from 207 ± 61 to 238 ± 77 W. This is the only study in which Wpeak and V˙O2peak did not respond similarly. To further explore the interaction between changes in ΔSmO2min and performance measures such as Wpeak and V˙O2 separately, subgroup analyses were conducted.
Subgroup analyses
Heterogeneity increased across all stratified subgroup analyses, with no effect of training. With regards to the effect of sex, our data included 22 female participants out of 58, in four of the six included groups (20, 23, 61). Previous studies looking at sex-based differences indicated that higher adiposity at the quadricep muscles seen more commonly in female participants, was an important factor affecting muscle oxygenation outcomes (15, 62, 63). None of the included studies reported adiposity for males and females independently, which may explain the increased heterogeneity when stratifying the studies by sex.
The exercise type was divided into continuous or interval training. This analysis showed no effect of training on ΔSmO2min, with a greater heterogeneity relative to the primary analysis. The continuous training intervention was used in two studies (21, 61). Rissanen et al., performed a year-long training intervention that included both endurance and resistance training. The exercise modalities used in the study were not specified. Therefore, we cannot conclude whether interval training was used, or to what degree the intervention differed from other studies that used interval training as their primary mode of training.
For exercise mode, no effect of training was found. The dominant mode of exercise in the included studies was cycling, both in training and during the IET. Zinner, et al., was the only study that included both leg and arm cycling (23). They included two groups that were exposed to a similar training load, one with leg and arm cycling, and the other with leg only. Interestingly, despite a lack of significant change in their SmO2min response after training, their results for the leg and arm group were the only ones showing directionally different findings (increase in SmO2min post-training) in the vastus lateralis muscle oxygenation response during an IET. Their report raises an interesting consideration in light of the findings presented in this study, concerning what NIRS can reveal about local skeletal muscle adaptations across muscle groups, or between upper and lower body muscles, during whole-body exercise. The ability of NIRS to quantify SmO2 across multiple muscle sites is currently underexplored and may shed light on the integrated systemic and local responses to training interventions. The ability to critically evaluate the effect of exercise mode on SmO2 is currently limited due to most studies using cycling as their primary mode of IET, even if the training intervention included a different mode of exercise.
Despite not finding a significant effect of training on ΔSmO2min associated with an increase in Wpeak in an IET after training, a trend was detected showing that with improvements in Wpeak, ΔSmO2min tended to be greater (i.e., greater desaturation to a lower SmO2min post-training). This finding corresponds with previous studies that investigated training interventions and their effects on muscle oxygenation measured by non-IET protocols (43, 56, 64). The most recent study by Paquette et al., investigated the effect of a 3-week training camp on national-level kayakers using muscle oxygen saturation as a primary outcome. Their results showed a greater ability to desaturate to a lower SmO2min after training, implying enhanced muscle oxygen extraction in conjunction with an increase in performance (14). The increase observed in Wpeak in the current meta-analysis shows that IET performance was improved with training, with longer duration to maximum exercise tolerance. Thus, despite only observing a tendency for decreased SmO2min at the end of the IET (p = 0.06), the rate of SmO2 desaturation during the IET was slowed after endurance training, producing the same or lower minimum at a higher Wpeak.
It is important to note that despite showing a trend for a greater SmO2 desaturation at a higher Wpeak, V˙O2peak did not present the same trend. This meta-analysis did not attempt to evaluate how SmO2 changes might be related to either peripheral skeletal muscle or systemic cardiopulmonary adaptations. Our results do raise an important question about implications concerning SmO2 changes in relation to improvements in performance without evidence of an effect of endurance training on the relationship between peripheral muscle measurements and systemic measures of maximal aerobic capacity.
From the meta-regression of ΔSmO2min and V˙O2peak, our results show no relationship between the change in ΔSmO2min to the change in V˙O2peak from pre- to post-training. As mentioned previously, SmO2min is associated with maximal task tolerance during an IET (11, 12). This is due to the progressive disruption of local metabolic milieu with increasing workload (2). Our results suggest that a similar SmO2min value will be achieved at maximal exercise tolerance after a training intervention, regardless of improvements in fitness. This suggests that a higher SmO2 value observed at a similar absolute submaximal workload after a training intervention may be associated with enhanced fitness and predictive of increased performance. For athletes and performance specialists, instead of requiring maximal testing to confirm fitness changes, SmO2 could be evaluated more frequently at submaximal workloads to suggest directional performance changes. This is like the current use of heart rate monitors in-field training relative to objective, external load measures such as running pace or cycling power output. Ideally, NIRS could be used in conjunction with heart rate monitors during regular training bouts instead of dedicated testing sessions, to improve tactical decision-making by practitioners and athletes. In this context, by observing the relationship of SmO2 to cycling power output, performance capacity could be estimated on a session-to-session basis. Before doing so, future studies should investigate the test-retest reliability of NIRS signals, especially when choosing wearable NIRS sensors during training sessions, to determine the minimal worthwhile change that could indicate fitness improvements.
Limitations
Our meta-analysis includes a small number of studies, and limited number of participants. Despite not presenting a risk of bias between studies, a lack of standardization of training intervention may have influenced our results and contributed to the heterogeneity of the analysis (65). In line with this limitation, inherent differences in exercise intensity during the training period may have effected training adaptations. One of the studies defined their intervention as endurance training but did not standardize their training intervention (21). For exercise mode, as indicated previously, cycling is a common exercise mode for IET; however, SmO2 responses may be different for running, rowing, and other exercise modalities related to different muscle recruitment demands. Future studies should use the same mode for IET as for training. Additionally, NIRS signals are sensitive to the absorbance characteristics of biological tissues (15, 62, 66, 67). Therefore, for better quantification of training intervention effects on SmO2, reporting adiposity is critical. Lastly, the focus of the analysis was to quantify the effects of training on SmO2min during an IET; however, future studies should consider investigating the ability of other testing protocols to quantify muscle oxygenation, such as constant workload protocols across intensity domains and intermittent IETs, among other protocols.
Conclusion
From our results, we concluded that endurance training does not affect the SmO2min value attained at the end of an IET. These findings are especially interesting when observing the effect of endurance training on the relationship between ΔSmO2min, Wpeak, and V˙O2peak. A trend found for an effect of training on the relationship between ΔSmO2min to Wpeak may suggest that any changes in SmO2 are best seen during submaximal exercise, rather than at maximal task tolerance. Interestingly, no effect of training on the relationship between ΔSmO2min and V˙O2peak was observed. This finding raises an important question about whether SmO2min is indicative of systemic cardiovascular changes, or peripheral muscle adaptations to endurance training. For practical applications, our findings suggest that NIRS provides a useful tool to quantify muscle adaptations relative to increased power output and, despite not showing any significant changes following endurance training, it may still be used as an additional physiological marker of exercise tolerance during an IET.
Data availability statement
The raw data supporting the conclusions of this article will be made available by the authors, without undue reservation.
Ethics statement
The studies involving humans included in this meta-analysis were conducted in accordance with the principles established in the declaration of Helsinki and approved by the relevant research ethics committees.
Author contributions
AY: Writing – original draft, Writing – review & editing. JA: Writing – review & editing. HN: Writing – review & editing. MR: Data curation, Formal Analysis, Methodology, Writing – review & editing. DC: Supervision, Writing – review & editing. JG: Supervision, Writing – review & editing. BS: Supervision, Writing – review & editing. MK: Supervision, Writing – review & editing.
Funding
The author(s) declare that no financial support was received for the research, authorship, and/or publication of this article.
Conflict of interest
The authors declare that the research was conducted in the absence of any commercial or financial relationships that could be construed as a potential conflict of interest.
Publisher's note
All claims expressed in this article are solely those of the authors and do not necessarily represent those of their affiliated organizations, or those of the publisher, the editors and the reviewers. Any product that may be evaluated in this article, or claim that may be made by its manufacturer, is not guaranteed or endorsed by the publisher.
Supplementary material
The Supplementary Material for this article can be found online at: https://www.frontiersin.org/articles/10.3389/fspor.2024.1406987/full#supplementary-material
References
1. Andersen P, Saltin B. Maximal perfusion of skeletal muscle in man. J Physiol (Lond). (1985) 366(1):233–49. doi: 10.1113/jphysiol.1985.sp015794
2. Kirby BS, Clark DA, Bradley EM, Wilkins BW. The balance of muscle oxygen supply and demand reveals critical metabolic rate and predicts time to exhaustion. J Appl Physiol. (2021) 130(6):1915–27. doi: 10.1152/japplphysiol.00058.2021
3. Lundby C, Montero D, Joyner M. Biology of VO2max: looking under the physiology lamp. Acta Physiol. (2017) 220(2):218–28. doi: 10.1111/apha.12827
4. Skattebo Ø, Calbet JAL, Rud B, Capelli C, Hallén J. Contribution of oxygen extraction fraction to maximal oxygen uptake in healthy young men. Acta Physiol. (2020) 230(2):e13486. doi: 10.1111/apha.13486
5. Poole DC, Richardson RS. Determinants of oxygen uptake. Sports Med. (1997) 24(5):308–20. doi: 10.2165/00007256-199724050-00003
6. Boone J, Vandekerckhove K, Coomans I, Prieur F, Bourgois JG. An integrated view on the oxygenation responses to incremental exercise at the brain, the locomotor and respiratory muscles. Eur J Appl Physiol. (2016) 116(11–12):2085–102. doi: 10.1007/s00421-016-3468-x
7. Spencer MD, Murias JM, Paterson DH. Characterizing the profile of muscle deoxygenation during ramp incremental exercise in young men. Eur J Appl Physiol. (2012) 112(9):3349–60. doi: 10.1007/s00421-012-2323-y
8. Yogev A, Arnold J, Clarke D, Guenette JA, Sporer BC, Koehle MS. Comparing the respiratory compensation point with muscle oxygen saturation in locomotor and non-locomotor muscles using wearable NIRS spectroscopy during whole-body exercise. Front Physiol. (2022) 13:818733. doi: 10.3389/fphys.2022.818733
9. Keir DA, Fontana FY, Robertson TC, Murias JM, Paterson DH, Kowalchuk JM, et al. Exercise intensity thresholds: identifying the boundaries of sustainable performance. Med Sci Sports Exerc. (2015) 47(9):1932–40. doi: 10.1249/MSS.0000000000000613
10. Snyder AC, Parmenter MA. Using near-infrared spectroscopy to determine maximal steady state exercise intensity. J Strength Cond Res. (2009) 23(6):1833–40. doi: 10.1519/JSC.0b013e3181ad3362
11. Boone J, Barstow TJ, Celie B, Prieur F, Bourgois J. The impact of pedal rate on muscle oxygenation, muscle activation and whole-body VO2 during ramp exercise in healthy subjects. Eur J Appl Physiol. (2014) 115(1):57–70. doi: 10.1007/s00421-014-2991-x
12. Rodrigo-Carranza V, González-Mohíno F, Turner AP, Rodriguez-Barbero S, González-Ravé JM. Using a portable near-infrared spectroscopy device to estimate the second ventilatory threshold. Int J Sports Med. (2021) 42(10):905–10. doi: 10.1055/a-1343-2127
13. Astorino TA, Allen RP, Roberson DW, Jurancich M. Effect of high-intensity interval training on cardiovascular function, V̇o2max, and muscular force. J Strength Cond Res. (2012) 26(1):138–45. doi: 10.1519/JSC.0b013e318218dd77
14. Paquette M, Bieuzen F, Billaut F. Effect of a 3-weeks training camp on muscle oxygenation, VO2 and performance in elite sprint kayakers. Front Sports Act Living. (2020) 2:47. doi: 10.3389/fspor.2020.00047
15. Barstow TJ. Understanding near infrared spectroscopy and its application to skeletal muscle research. J Appl Physiol. (2019) 126:1360–76. doi: 10.1152/japplphysiol.00166.2018
16. Hamaoka T, McCully KK. Review of early development of near-infrared spectroscopy and recent advancement of studies on muscle oxygenation and oxidative metabolism. J Physiol Sci. (2019) 69(6):799–811. doi: 10.1007/s12576-019-00697-2
17. Iannetta D, Qahtani A, Maturana FM, Murias JM. The near-infrared spectroscopy-derived deoxygenated haemoglobin breaking-point is a repeatable measure that demarcates exercise intensity domains. J Sci Med Sport. (2017) 20(9):873–7. doi: 10.1016/j.jsams.2017.01.237
18. Jones S, Chiesa ST, Chaturvedi N, Hughes AD. Recent developments in near-infrared spectroscopy (NIRS) for the assessment of local skeletal muscle microvascular function and capacity to utilise oxygen. Artery Res. (2016) 16:25–33. doi: 10.1016/j.artres.2016.09.001
19. Caen K, Vermeire K, Pogliaghi S, Moerman A, Niemeijer V, Bourgois JG, et al. Aerobic interval training impacts muscle and brain oxygenation responses to incremental exercise. Front Physiol. (2019) 10:1195. doi: 10.3389/fphys.2019.01195
20. Keramidas ME, Kounalakis SN, Geladas ND. The effect of interval training combined with thigh cuffs pressure on maximal and submaximal exercise performance. Clin Physiol Funct Imaging. (2012) 32(3):205–13. doi: 10.1111/j.1475-097X.2011.01078.x
21. Rissanen APE, Tikkanen HO, Koponen AS, Aho JM, Peltonen JE. One-year unsupervised individualized exercise training intervention enhances cardiorespiratory fitness but not muscle deoxygenation or glycemic control in adults with type 1 diabetes. Appl Physiol Nutr Metab. (2017) 43(4):387–96. doi: 10.1139/apnm-2017-0222
22. Shiroishi K, Kime R, Osada T, Murase N, Shimomura K, Katsumura T. Decreased muscle oxygenation and increased arterial blood flow in the non-exercising limb during leg exercise. Adv Exp Med Biol. (2010) 662:379–84. doi: 10.1007/978-1-4419-1241-1_55
23. Zinner C, Sperlich B, Born DP, Michels G. Effects of combined high intensity arm and leg training on performance and cardio-respiratory measures. J Sports Med Phys Fitness. (2017) 57(7–8):969–75. doi: 10.23736/S0022-4707.16.06539-7
24. Perrey S. Muscle oxygenation unlocks the secrets of physiological responses to exercise: time to exploit it in the training monitoring. Front Sports Act Living. (2022) 4:864825. doi: 10.3389/fspor.2022.864825
25. Perrey S, Ferrari M. Muscle oximetry in sports science: a systematic review. Sports Med. (2018) 48(3):597–616. doi: 10.1007/s40279-017-0820-1
26. Spiering BA, Mujika I, Sharp MA, Foulis SA. Maintaining physical performance: the minimal dose of exercise needed to preserve endurance and strength over time. J Strength Cond Res. (2021) 35(5):1449–58. doi: 10.1519/JSC.0000000000003964
27. Rosenblat MA, Perrotta AS, Vicenzino B. Polarized vs. threshold training intensity distribution on endurance sport performance: a systematic review and meta-analysis of randomized controlled trials. J Strength Cond Res. (2019) 33:3491–500. doi: 10.1519/JSC.0000000000002618
28. Rosenblat MA, Lin E, da Costa BR, Thomas SG. Programming interval training to optimize time-trial performance: a systematic review and meta-analysis. Sports Med (Auckland). (2021) 51:1687–714. doi: 10.1007/s40279-021-01457-2
29. Higgins JPT, Thomas J, Chandler J, Cumpston M, Li T, Page MJ, et al. Cochrane Handbook for Systematic Reviews of Interventions Version 6.3. 6.3. Welch VA: Cochrane (2022) 2022.
30. Page MJ, McKenzie JE, Bossuyt PM, Boutron I, Hoffmann TC, Mulrow CD, et al. The PRISMA 2020 statement: an updated guideline for reporting systematic reviews. BMJ. (2021) 372:n71. doi: 10.1136/bmj.n71
31. DerSimonian R, Laird N. Meta-analysis in clinical trials. Control Clin Trials. (1986) 7(3):177–88. doi: 10.1016/0197-2456(86)90046-2
32. Burton HM, Wolfe AS, Vardarli E, Satiroglu R, Coyle EF. Background inactivity blunts metabolic adaptations to intense short-term training. Med Sci Sports Exerc. (2021) 53(9):1937–44. doi: 10.1249/MSS.0000000000002646
33. Kime R, Niwayama M, Kaneko Y, Takagi S, Fuse S, Osada T, et al. Muscle deoxygenation and its heterogeneity changes after endurance training. In: Luo Q, Li LZ, Harrison DK, Shi H, Bruley DF, editors. Oxygen Transport to Tissue XXXVIII. Cham: Springer International Publishing (2016). p. 275–81.
34. Granata C, Jamnick NA, Bishop DJ. Principles of exercise prescription, and how they influence exercise-induced changes of transcription factors and other regulators of mitochondrial biogenesis. Sports Med (Auckland, NZ). (2018) 48(7):1541–59. doi: 10.1007/s40279-018-0894-4
35. Higgins JPT, Thompson SG, Deeks JJ, Altman DG. Measuring inconsistency in meta-analyses. Br Med J. (2003) 327:557–60. doi: 10.1136/bmj.327.7414.557
36. Egger M, Smith GD, Schneider M, Minder C. Bias in meta-analysis detected by a simple, graphical test. Br Med J. (1997) 315:629–34. doi: 10.1136/bmj.315.7109.629
37. Wu HJ. Effect of high intensity intermittent training on muscle oxygen content and athletic ability of university wrestlers. Basic Clin Pharmacol Toxicol. (2020) 126:74. doi: 10.1111/bcpt.13405
38. Archiza B, Andaku DK, Caruso FCR, Bonjorno JC, Oliveira CR de, Ricci PA, et al. Effects of inspiratory muscle training in professional women football players: a randomized sham-controlled trial. J Sports Sci. (2018) 36(7):771–80. doi: 10.1080/02640414.2017.1340659
39. Bailey SJ, Wilkerson DP, DiMenna FJ, Jones AM. Influence of repeated sprint training on pulmonary O2 uptake and muscle deoxygenation kinetics in humans. J Appl Physiol. (2009) 106(6):1875–87. doi: 10.1152/japplphysiol.00144.2009
40. Buchheit M, Ufland P. Effect of endurance training on performance and muscle reoxygenation rate during repeated-sprint running. Eur J Appl Physiol. (2011) 111(2):293–301. doi: 10.1007/s00421-010-1654-9
41. Murias JM, Kowalchuk JM, Paterson DH. Speeding of VO2 kinetics with endurance training in old and young men is associated with improved matching of local O2 delivery to muscle O2 utilization. J Appl Physiol. (2010) 108(4):913–22. doi: 10.1152/japplphysiol.01355.2009
42. Barberan-Garcia A, Munoz PA, Gimeno-Santos E, Burgos F, Torralba Y, Gistau C, et al. Training-induced changes on quadriceps muscle oxygenation measured by near-infrared spectroscopy in healthy subjects and in chronic obstructive pulmonary disease patients. Clin Physiol Funct Imaging. (2019) 39(4):284–90. doi: 10.1111/cpf.12572
43. Faiss R, Léger B, Vesin JM, Fournier PE, Eggel Y, Dériaz O, et al. Significant molecular and systemic adaptations after repeated sprint training in hypoxia. PLoS One. (2013) 8(2):1–13. doi: 10.1371/journal.pone.0056522
44. Faiss R, Willis S, Born DP, Sperlich B, Vesin JM, Holmberg HC, et al. Repeated double-poling sprint training in hypoxia by competitive cross-country skiers. Med Sci Sports Exerc. (2015) 47(4):809–17. doi: 10.1249/MSS.0000000000000464
45. Jones B, Hamilton DK, Cooper CE. Muscle oxygen changes following sprint interval cycling training in elite field hockey players. PLoS One. (2015) 10(3):e0120338. doi: 10.1371/journal.pone.0120338
46. Jones S, D’Silva A, Bhuva A, Lloyd G, Manisty C, Moon JC, et al. Improved exercise-related skeletal muscle oxygen consumption following uptake of endurance training measured using near-infrared spectroscopy. Front Physiol. (2017) 8:1018. doi: 10.3389/fphys.2017.01018
47. Hirano M, Shindo M, Mishima S, Morimura K, Higuchi Y, Yamada Y, et al. Effects of 2 weeks of low-intensity cycle training with different pedaling rates on the work rate at lactate threshold. Eur J Appl Physiol. (2015) 115(5):1005–13. doi: 10.1007/s00421-014-3081-9
48. Jacobs RA, Flück D, Bonne TC, Bürgi S, Christensen PM, Toigo M, et al. Improvements in exercise performance with high-intensity interval training coincide with an increase in skeletal muscle mitochondrial content and function. J Appl Physiol. (2013) 115(6):785–93. doi: 10.1152/japplphysiol.00445.2013
49. Prieur F, Mucci P. Effect of high-intensity interval training on the profile of muscle deoxygenation heterogeneity during incremental exercise. Eur J Appl Physiol. (2013) 113(1):249–57. doi: 10.1007/s00421-012-2430-9
50. Neary JP, McKenzie DC, Bhambhani YN. Effects of short-term endurance training on muscle deoxygenation trends using NIRS. Med Sci Sports Exerc. (2002) 34(11):1725–32. doi: 10.1097/00005768-200211000-00006
51. Sako T. The effect of endurance training on resting oxygen stores in muscle evaluated by near infrared continuous wave spectroscopy. In: Takahashi E, Bruley DF, editors. Oxygen Transport to Tissue XXXI. Boston, MA: Springer US (2010). p. 341–6.
52. Bentley RF, Jones JH, Hirai DM, Zelt JT, Giles MD, Raleigh JP, et al. Submaximal exercise cardiac output is increased by 4 weeks of sprint interval training in young healthy males with low initial q˙-v˙O2: importance of cardiac response phenotype. PLoS One. (2019) 14(1):1–17. doi: 10.1371/journal.pone.0195458
53. Williams AM, Paterson DH, Kowalchuk JM. High-intensity interval training speeds the adjustment of pulmonary O2 uptake, but not muscle deoxygenation, during moderate-intensity exercise transitions initiated from low and elevated baseline metabolic rates. J Appl Physiol. (2013) 114(11):1550–62. doi: 10.1152/japplphysiol.00575.2012
54. Caen K, Vermeire K, Bourgois JG, Boone J. Exercise thresholds on trial: are they really equivalent? Med Sci Sports Exerc. (2018) 50(6):1277–84. doi: 10.1249/MSS.0000000000001547
55. Bellinger PM, Sabapathy S, Craven J, Arnold B, Minahan C. Overreaching attenuates training-induced improvements in muscle oxidative capacity. Med Sci Sports Exerc. (2020) 52(1):77–85. doi: 10.1249/MSS.0000000000002095
56. Costes F, Prieur F, Féasson L, Geyssant A, Barthélémy JC, Denis C. Influence of training on NIRS muscle oxygen saturation during submaximal exercise. Med Sci Sports Exerc. (2001) 33(9):1484–9. doi: 10.1097/00005768-200109000-00010
57. Jones S, Kinsella M, Torlasco C, Kaynezhad P, de Roever I, Moon JC, et al. Improvements in skeletal muscle can be detected using broadband NIRS in first-time marathon runners. In: Ryu PD, LaManna JC, Harrison DK, Lee SS, editors. Oxygen Transport to Tissue XLI. Cham: Springer International Publishing (2020). p. 245–51.
58. McKay BR, Paterson DH, Kowalchuk JM. Effect of short-term high-intensity interval training vs. continuous training on O2 uptake kinetics, muscle deoxygenation, and exercise performance. J Appl Physiol. (2009) 107(1):128–38. doi: 10.1152/japplphysiol.90828.2008
59. Murias JM, Edwards JA, Paterson DH. Effects of short-term training and detraining on VO2 kinetics: faster VO2 kinetics response after one training session. Scand J Med Sci Sports. (2016) 26(6):620–9. doi: 10.1111/sms.12487
60. McKay AKA, Stellingwerff T, Smith ES, Martin DT, Mujika I, Goosey-Tolfrey VL, et al. Defining training and performance caliber: a participant classification framework. Int J Sports Physiol Perform. (2022) 17(2):317–31. doi: 10.1123/ijspp.2021-0451
61. Kime R, Im J, Moser D, Nioka S, Katsumura T, Chance B. Noninvasive determination of exercise-induced vasodilation during bicycle exercise using near infrared spectroscopy. Med Sci Monit. (2009) 15(3):CR89–94.19247245
62. McManus CJ, Collison J, Cooper CE. Performance comparison of the MOXY and PortaMon near-infrared spectroscopy muscle oximeters at rest and during exercise. J Biomed Opt. (2018) 23(01):1. doi: 10.1117/1.JBO.23.1.015007
63. Ryan TE, Erickson ML, Brizendine JT, Young HJ, McCully KK. Noninvasive evaluation of skeletal muscle mitochondrial capacity with near-infrared spectroscopy: correcting for blood volume changes. J Appl Physiol. (1985) 113(2):175–83. doi: 10.1152/japplphysiol.00319.2012
64. Paquette M, Bieuzen F, Billaut F. The effect of HIIT vs. SIT on muscle oxygenation in trained sprint kayakers. Eur J Appl Physiol. (2021) 121:2743–59. doi: 10.1007/s00421-021-04743-z
65. Turner RM, Bird SM, Higgins JPT. The impact of study size on meta-analyses: examination of underpowered studies in Cochrane reviews. PLoS One. (2013) 8(3):e59202. doi: 10.1371/journal.pone.0059202
66. Binzoni T, Cooper CE, Wittekind AL, Beneke R, Elwell CE, Van De Ville D, et al. A new method to measure local oxygen consumption in human skeletal muscle during dynamic exercise using near-infrared spectroscopy. Physiol Meas. (2010) 31(9):1257–69. doi: 10.1088/0967-3334/31/9/014
67. Craig JC, Broxterman RM, Wilcox SL, Chen C, Barstow TJ. Effect of adipose tissue thickness, muscle site, and sex on near-infrared spectroscopy derived total-[hemoglobin-myoglobin]. J Appl Physiol. (2017) 123(6):1571–8. doi: 10.1152/japplphysiol.00207.2017
Keywords: NIRS (near infrared reflectance spectroscopy), muscle oxygenation, endurance training, incremental exercise test, systematic review
Citation: Yogev A, Arnold JI, Nelson H, Rosenblat MA, Clarke DC, Guenette JA, Sporer BC and Koehle MS (2024) The effects of endurance training on muscle oxygen desaturation during incremental exercise tests: a systematic review and meta-analysis. Front. Sports Act. Living 6:1406987. doi: 10.3389/fspor.2024.1406987
Received: 26 March 2024; Accepted: 9 August 2024;
Published: 24 October 2024.
Edited by:
Jared R. Fletcher, Mount Royal University, CanadaReviewed by:
Carlos Pascual-Morena, University of Castilla-La Mancha, SpainAldo A. Vasquez-Bonilla, University of Extremadura, Spain
Copyright: © 2024 Yogev, Arnold, Nelson, Rosenblat, Clarke, Guenette, Sporer and Koehle. This is an open-access article distributed under the terms of the Creative Commons Attribution License (CC BY). The use, distribution or reproduction in other forums is permitted, provided the original author(s) and the copyright owner(s) are credited and that the original publication in this journal is cited, in accordance with accepted academic practice. No use, distribution or reproduction is permitted which does not comply with these terms.
*Correspondence: Assaf Yogev, yogevassaf@gmail.com