- 1Faculty of Sport Science, Burapha University, Mueang, Thailand
- 2Centre of Research, Education, Innovation and Intervention in Sport (CIFI2D), Faculty of Sport, University of Porto, Porto, Portugal
- 3Porto Biomechanics Laboratory (LABIOMEP-UP), University of Porto, Porto, Portugal
- 4Faculty of Physical Education and Physiotherapy, Federal University of Amazonas, Manaus, Brazil
- 5Superior Institute of Engineering of Porto, Polytechnic Institute of Porto, Porto, Portugal
- 6Research Center in Physical Activity, Health and Leisure (CIAFEL), Faculty of Sports, University of Porto (FADEUP), Porto, Portugal
- 7Laboratory for Integrative and Translational Research in Population Health (ITR), Porto, Portugal
This study aimed to identify the biomechanical features of backstroke to breaststroke transition techniques (open, somersault, bucket, and crossover) in age-group swimmers. Eighteen preadolescent swimmers (12.2 ± 0.4 years old and 3–4 Tanner stages) underwent 4 weeks of systematic contextual interference training, comprising 16 sessions (40 min·session−1). Soon after, experimental testing was conducted where swimmers randomly performed 12 × 15 m maximal turns (composed of 7.5 m turn-in and 7.5 m turn-out of the wall segments), three in each transition technique. Kinematical, kinetic, and hydrodynamic variables were assessed with a dual-media motion capture system (12 land and 11 underwater cameras), triaxial underwater force plates, and inverse dynamics. Variables were grouped in turn-in (approach and rotation) and turn-out (wall contact, gliding, and pull-out) phases, with factor analysis used to select the variables entering on multiple regressions. For the turn-in phase, 86, 77, 89, and 87% of the variance for open, somersault, bucket, and crossover turning techniques, respectively, was accounted by the 7.5 and 2.5 m times, mean stroke length, and rotation time. For the turn-out phase, first gliding distance and time, second gliding depth, turn-out time, and dominating peak_Z push-off force accounted for 93% in open turn, while wall contact time, first gliding distance, breakout distance and time, turn-out time, dominating peak_Y push-off force, and second gliding drag coefficient accounted for 92% in a somersault turn. The foot plant index, push-off velocity, second gliding distance, and turn-out time accounted for 92% in bucket turn while breakout and turn-out time, non-dominating peak_Y and peak_Z push-off force, first and second gliding drag force and second gliding drag coefficient accounted for 90% in crossover turn, respectively. The findings in this study were novel and provided relevant biomechanical contribution, focusing on the key kinematic–temporal determinant during turn-in, rotation, and push-off efficacy, and the kinetic and hydrodynamic during turn-out, which would lead to improved backstroke to breaststroke transition techniques in 11–13 years-old age-group swimmers.
Introduction
Performing fast and skilled turning actions, and start and swim phases, is fundamental for improving competitive swimming performance (Arellano et al., 1994; McGibbon et al., 2018; Zacca et al., 2020a). However, conclusive information on the 200- and 400-m individual medley events, in which butterfly, backstroke, breaststroke, and freestyle swim in this order, is limited. Therefore, extensive research is required to identify the key biomechanical variables and their respective contributions to each transition technique (Chainok et al., 2021).
Among the medley turns, there are four well-described backstroke to breaststroke transition techniques (the open, the somersault, the bucket, and the crossover), which are very complex movements (i.e., performed in different planes and axes). In addition, swimmers need to comply with the FINA rules, i.e., touch the wall while on their back, maintain the shoulders at or past the vertical direction toward the breast when leaving the wall, and assume a ventral gliding position prior to the first breaststroke upper limbs action. Studies on the backstroke to breaststroke transition techniques are scarce, lacking scientific and practical validation of the specific determinant factors that play a vital role in gaining the advantage in each backstroke to breaststroke transition techniques.
Key biomechanical variables related to swimming turn performance have been studied using temporal, kinematic (Blanksby et al., 2004; Araujo et al., 2010; Pereira et al., 2015), kinetic (Prins and Patz, 2006; Pereira et al., 2015; Chainok et al., 2021), and hydrodynamic data (Benjanuvatra et al., 2001; Vilas-Boas et al., 2010; Chainok et al., 2021), but no study has examined the biomechanical determinants for optimal backstroke to breaststroke transition performance. Knowing that this information is a key factor for coaches when planning their specific training activities, we aimed to identify the key biomechanical variables that affect the performance in the four backstroke to breaststroke transition techniques in age-group swimmers. It was hypothesized that the 15 m turning time performance is described by combining contributions from the turn-in and turn-out phases, and different combinations of feature variables depending on the chosen backstroke to breaststroke transition technique.
Materials and Methods
Subjects
A total of 18 age-group swimmers, nine boys and nine girls, from the 11–13 years old age group of a competitive swimming club, volunteered to participate in the current study. Boys and girls characteristics were (respectively): 12.5 ± 0.5 vs. 11.6 ± 0.5 years old, 48.7 ± 12.4 vs. 46.7 ± 10.8 kg of body mass, 1.59 ± 0.14 vs. 1.52 ± 0.07 m of height, 14.8 ± 5.1 vs. 21.8 ± 7.10% of fat mass, 3–4 Tanner stages (Zacca et al., 2020b) and 59 ± 9 vs. 55 ± 12% of 200 m individual medley best performances of the 2018 short-course World Junior Record. Swimmers parents were informed about the benefits and risks of participating before they were asked to sign an informed consent form (approved by the ethics board of the local university—CEFADE 08.2014) in agreement with the Declaration of Helsinki.
Procedures
Four backstroke-to-breaststroke transition techniques were identified (FINA rules; https://www.fina.org/, see Figure 1). Prior to the experiments, swimmers answered a questionnaire about their backstroke to breaststroke transition techniques preferences, with 18 selecting the open technique and only two the somersault. The experimental protocol took place in a 25-m (1.90 m deep) indoor pool with ~27 and ~26°C of water and air temperatures (respectively) and 59% relative humidity. Age-group swimmers joined 16 practice sessions throughout a 4-week training program (see details in Chainok et al., 2021) performing variants of the same task with structured increases in contextual interference (Porter and Magill, 2010). Contextual interference can be defined as the interference in performance and learning that arises from practicing one task in the context of other tasks (Schmidt and Lee, 2005; Porter and Magill, 2010). The 16 practice sessions were part of the regular training sessions, with the turning practice occurring during the last 40 min of every session. Two experienced coaches conducted all practice sessions and specific coaching feedback based on mechanical factors to ensure consistency in coaching techniques, proper familiarization (Galbraith et al., 2008; de Jesus et al., 2016). All the participants followed a scheduled program from the 1st to the 16th practice session program (see details in Chainok et al., 2021). At the end of the intervention period, swimmers were invited for an evaluation session. Thus, after a 400-m moderate-intensity warm-up including some elements of backstroke to breaststroke transition techniques (Figure 1), swimmers were invited to perform 12 × 15 m maximal turns (composed of 7.5 m turn-in and 7.5 m turn-out of the wall segments). Each swimmer completed three attempts of each backstroke to breaststroke transition technique (randomized order), with a 3 min rest interval between trials (see details in Chainok et al., 2021).
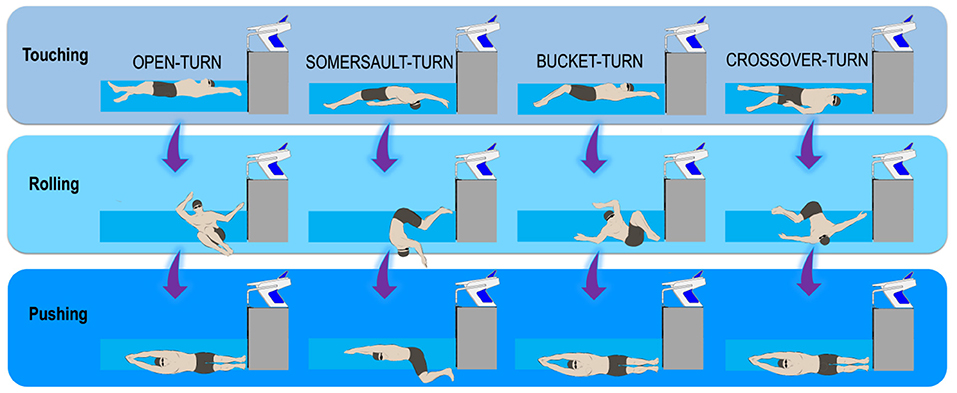
Figure 1. Backstroke to breaststroke turning techniques are distinguished by the different body orientations of the swimmers throughout the touching, rolling and pushing-off phases.
Dual-media motion capture system with 12 land and 11 underwater cameras (Oqus 3 and 4 series, Qualisys, Gothenburg, Sweden) and a full-body marker setup (with 51 spherical retroreflective markers, see Figure 2) were used to track swimmer's actions at 100 Hz (Lauer et al., 2016) (see details of camera placement and configuration and calibration in Chainok et al., 2021). The kinetic assessment was obtained with two triaxial underwater force plates (Mourão et al., 2016) operating at a 2,000 Hz sampling frequency and fixed into the pool's wall on a custom built support (see details in de Jesus et al., 2019). The limits of this structure were identified with four retroreflective markers.
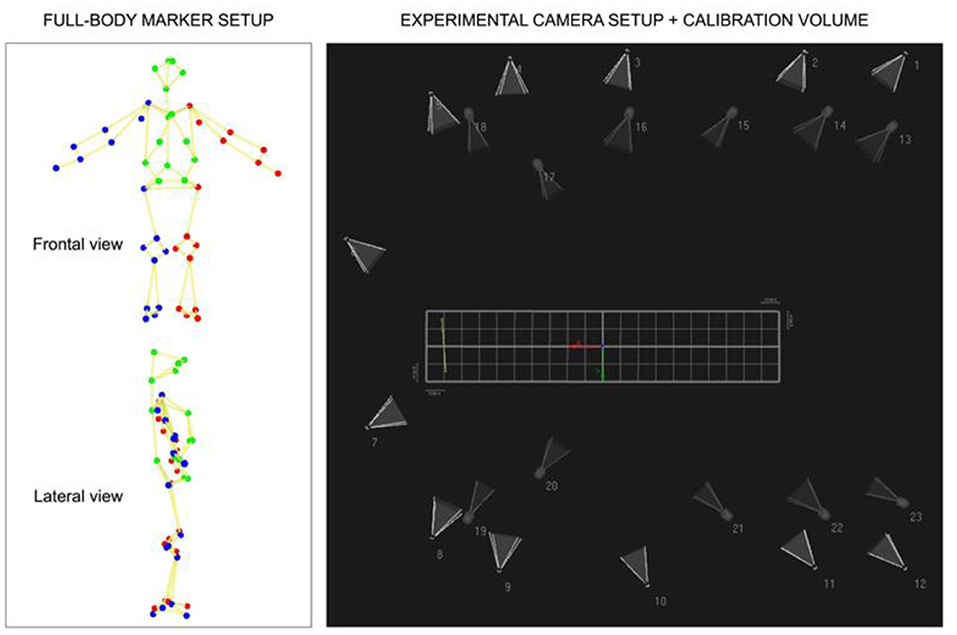
Figure 2. Configuration of kinematic-temporal data: full-body marker setup in Qualisys Track, experimental camera positioning with 12 land and 11 underwater cameras, and calibration volume covered. The orthogonal axes were defined as X, Y, and Z for horizontal, mediolateral, and vertical (Z = 0 defines water surface) movements. The yellow line depicts the reference system and positioning of the triaxial two force platforms.
The 15 m turning time performance (composed of 7.5 m turn-in and 7.5 m turn-out of the wall segments) encompassed approaching, touching (wall contact), rolling, pushing glide, and swimming resumption until the vertex passes the 7.5 m marker (Figure 1). The Qualisys Track Manager (Oqus 3 and 4 series, Qualisys, Gothenburg, Sweden) software was used to acquire the temporal and 3D kinematic data. Built-in spline interpolation was used to fill markers' missing trajectories (representing up to ~50, 120, and 60 frames, i.e., 3.3, 8.0, and 4.0% of the trial duration in the approach, rotation, and turnout phases, respectively). The software Acqknowledge v.3.9.0 (BIOPAC Systems Incorporation, Santa Barbara, California, USA) was used to perform residual analysis to optimize the digital filter cutoff frequency (fast Fourier transform) and kinematic–temporal data were low-pass filtered using a digital filter with a cutoff frequency of 6 Hz (FIR—Window Blackman-61dB) (Acqknowledge, BIOPACiopac Systems Incorporation, Santa Barbara, California, USA). The bow wave effect at the beginning of the feet contact was considered negligible (not edited in the kinetic analysis) since swimmers glided in before touching the wall and rotated to push-off. Despite that, the underwater force platforms were synchronized with the motion capture system and the image-based kinematics allowed a reasonable verification of the force-to-time curve symmetry. Dominant (DPO) and non-dominant (NPO) push-off force terms were used to identify the characteristic peak force contributions in the x, y, and z components. Kinetic data processing was divided in to: (i) acquisition, plotting, and saving the strain readings of each triaxial force and the moment-of-force components from each force plate using a custom LabVIEW™ program (National Instruments, Austin, Texas, USA, http://www.ni.com/en-us/shop/labview.html) (Mourão et al., 2016; de Jesus et al., 2019); (ii) converting the strain readings into force values according to the previous calibration (Matlab R2014a, MathWork Inc., Natick, Massachusetts, USA), and (iii) filtering curves using a fourth-order zero-phase digital Butterworth filter with a 10 Hz cut-off frequency (Mourão et al., 2016; de Jesus et al., 2019) (Figure 3). The hydrodynamic variables (drag, drag coefficient, and body cross-sectional area) were assessed through an inverse dynamics approach (Vilas-Boas et al., 2010). We used planimetry (Clarys, 1979; Vilas-Boas et al., 2010) for cross-sectional area (S) assessment (Figure 4; see details in Chainok et al., 2021). The description of the studied kinematic-temporal, kinetic, and hydrodynamic variables is shown in Tables 1, 2.
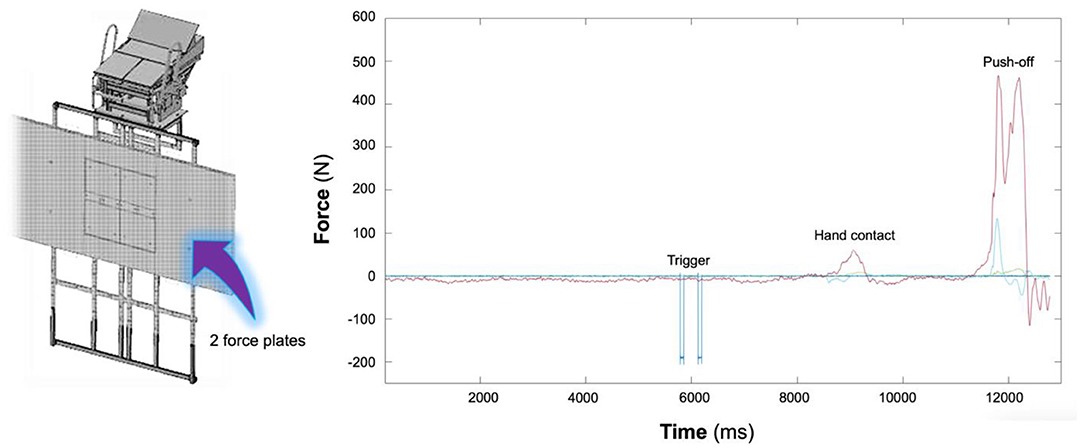
Figure 3. Kinetic data setup and data processing: two triaxial force plates set up and force-time curve of two triaxial force plate profiles (left and right panels). Fx and Fy are the mediolateral (green) and up and down (blue) components, and Fz is the horizontal force component (red).
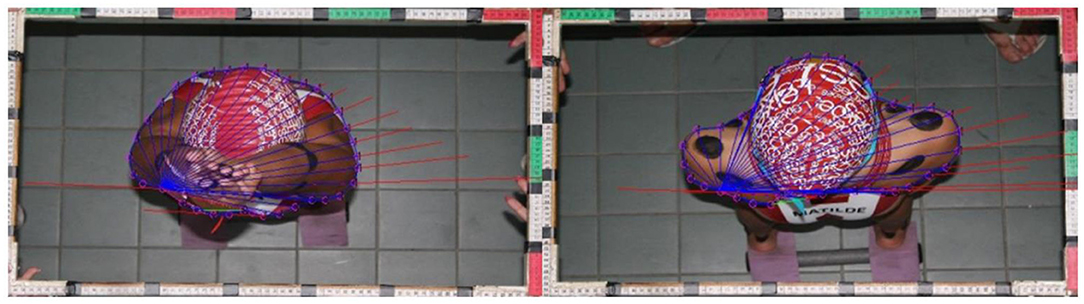
Figure 4. Body surface area determined through planimetry: data processing of the first and second gliding positions.
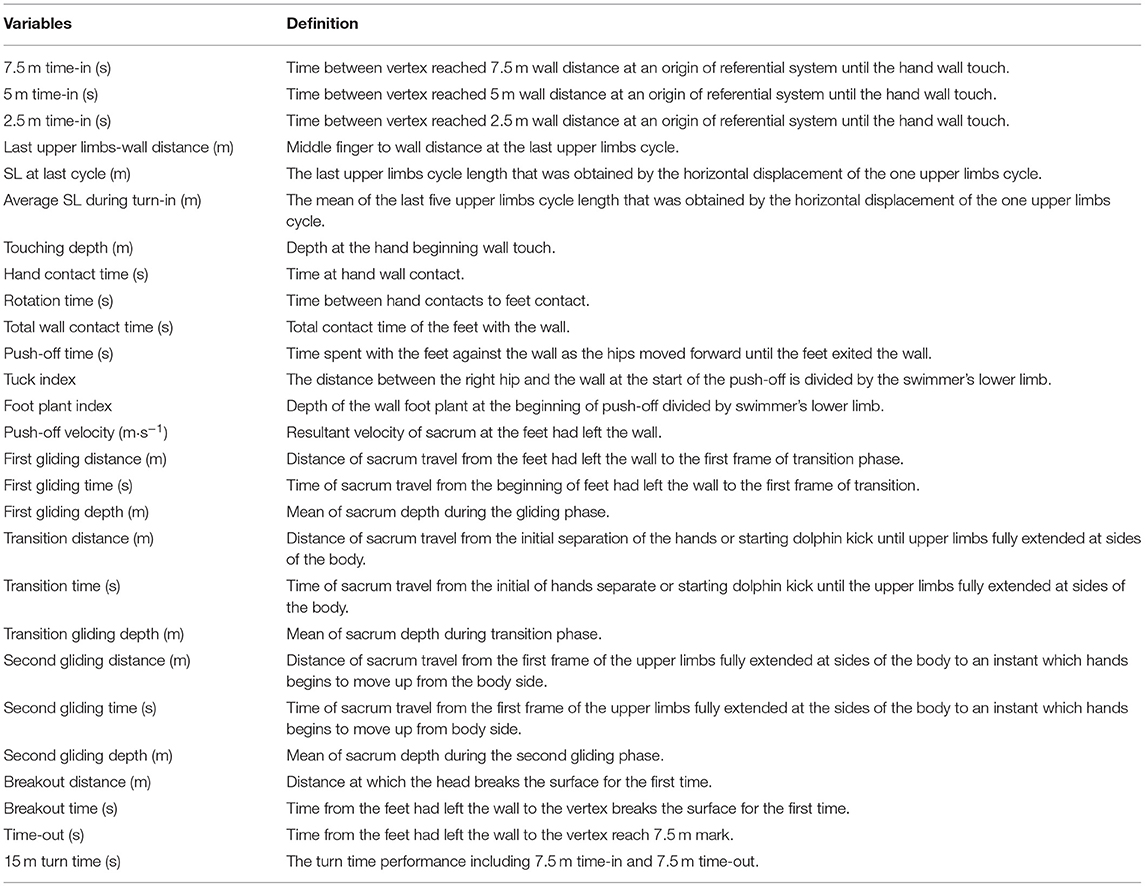
Table 1. Kinematic-temporal variables selected for studying backstroke to breaststroke turning techniques.
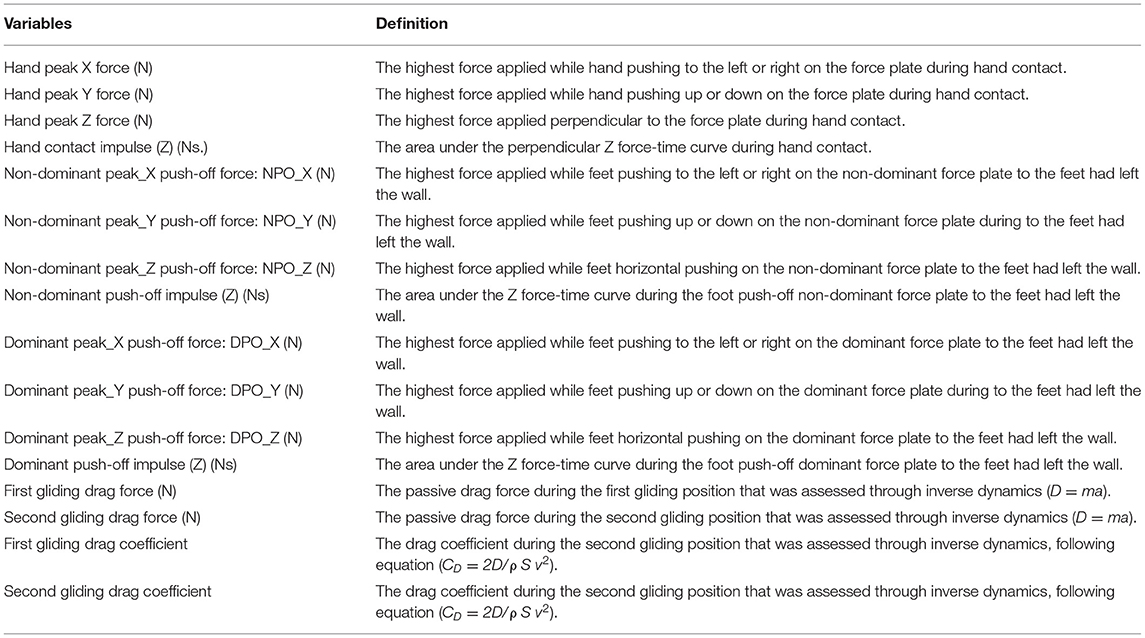
Table 2. Kinetic and hydrodynamic variables selected for analyzing the backstroke to breaststroke turns.
Statistical Analysis
Basic exploratory and descriptive statistics were computed using SPSS Statistics for Windows Version 24.0 (IBM Corporation, Armonk, New York, USA) aiming to detect potential errors in data entry and eventual outliers, and assessing data distribution normality (Shapiro–Wilk test), multicollinearity (variance inflation factors), and homoscedasticity (Levene's test). A one-way ANOVA was used to observe differences in the selected kinematic-temporal, kinetic, and hydrodynamic variables among the four different backstroke to breaststroke turning techniques. If a significant effect was found, post-hoc pairwise comparisons using Tukey's HSD were conducted. Then, factor analysis was conducted to lower the number of variables and to analyze the relationships structures between variables. For this purpose, selected variables were grouped into turn-in and turn-out variables (approach and rotation vs. wall contact, gliding, and pull-out phases), factors were chosen on the basis of a cutoff eigenvalue of 1, principal component extraction with a varimax rotation, and the scree plot proposed (Tor et al., 2015), and best-subsets analysis was conducted to determine the best regression equation for 15 m turn time prediction (using Minitab 19, Minitab Incorporation, State College, Pennsylvania, USA). Finally, a multiple regression analysis (with the enter method) was used to determine and predict the 15 m turn time based on each turning technique selected variables. The full multiple linear regression analysis was completed with SPSS based on the largest R2 value and the smallest error.
Results
Descriptive- and variance-related analysis on selected variables for each backstroke to breaststroke turning technique is given in Table 3. The turning techniques showed no significant effects on the turn-in (F3, 232 = 0.61; p = 0.61), rotation time (F3, 232 = 0.69; p = 0.56), turn-out (F3, 232 = 0.33; p = 0.80), and 15 m turn times (F3, 232 = 0.64; p = 0.59).
The best subsets regression for turn-in and turn-out to predict 15 m turning time in each backstroke to breaststroke turning technique are given in Tables 4, 5. Regarding the open turn, there were three predictors (7.5 m time-in, average SL, and hand contact time) explained 86% (R2 = 0.86; p < 0.01) for turn-in, five predictors (first gliding distance, first gliding time, second gliding depth, turn-out time, and DPO_Z) explained 93% (R2 = 0.93; p < 0.01) for turn-out on the 15 m turning time. For the somersault turn, there were three predictors (7.5 m time-in, 2.5 m time, and rotation time) explained 78% (R2 = 0.78; p < 0.01) for turn-in, seven predictors (wall contact time, first gliding distance, breakout distance, breakout time, turn-out time, DPO_Y, and CD2) explained 92% (R2 = 0.92; p < 0.01) for turn-out on the 15 m turning time, respectively.
For the bucket turn, there were three predictors (7.5 m time-in, 2.5 m time-in, and last upper limbs-wall distance) explained 89% (R2 = 0.89; p < 0.01) for turn-in, five predictors (foot plant index, push-off velocity, second gliding distance, turn-out time, and CD1) explained 92% (R2 = 0.92; p < 0.01) for turn-out on the 15 m turning time. For the crossover turn, there were four predictors (7.5 m time-in, 2.5 m time-in, average SL, and rotation time) explained 87% (R2 = 0.87; p < 0.01) for turn-in, seven predictors (breakout time, turn-out time, NPO_Y, NPO_Z, D1, D2, and CD2) explained 90% (R2 = 0.90; p < 0.01) for turn-out on the 15 m turning time, respectively.
Discussion
The main aim of this study was to identify the biomechanical features of backstroke to breaststroke transition techniques (open, somersault, bucket, and crossover) in age-group swimmers. We believed that 15 m turning time performance is described by combining contributions from the turn-in and turn-out phases, and different combinations of feature variables depending on the chosen backstroke to breaststroke transition technique. As expected, general turn-in performance can be predicted mostly by faster times during the 7.5 m, 2.5 m to the wall. The average SL is a predictor of turn-in performance for both open and crossover turns, with faster rotation time being the most relevant variable for somersault and crossover turns. The last upper limbs-to-wall distance, which refers to kinesthetic awareness and sense of space, affects bucket turn performance. Our results from the turn-out phase highlighted the importance of the interaction between kinematic and kinetic variables at the wall contact and push-off phase, which influenced turn-out performance across all backstroke to breaststroke turns studied. However, the importance of the turn-out variables changes depending on the chosen technique.
Open Turn
The 7.5 m time-in, average stroke length, and hand contact time were the three key variables for the turn-in performance, while the first gliding distance, first gliding time, second gliding depth, turn-out time, and dominant push-off_Z force were identified as key for the turn-out. Our results are consistent with some previous findings in elite swimmers that indicated that their turn-in performance was highly associated with their total turn time in the 200 and 400 m backstroke to breaststroke (Mason and Cossor, 2001). From the perspective of turn-in performance, the simple direction switch from the supine to the prone position during the open turn may require specific skills to maintain the swimming speed that incorporates the fastest rotation or pivot execution (Blanksby et al., 2004; Webster et al., 2011).
It has been reported that the optimization of the relationships between the kinematic, kinetic, and hydrodynamic variables can directly influence turn-out performance (Termin and Pendergast, 1998; Vilas-Boas et al., 2010; Pereira et al., 2015). The open turn turn-out performance mainly depends on the interaction between the kinetic variable (dominant push-off_Z force) and the four kinematic-temporal variables (first gliding distance, first gliding time, second gliding depth, and turn-out time). Theoretically, the peak perpendicular force, total impulse, and wall contact time kinetic features are key factors of swimming turns (Prins and Patz, 2006), with the dominant peak push-off_Z force being the key kinetic variable in this study. It tended to be slightly lower than data previously obtained in the breaststroke (557 ± 109 N; Blanksby et al., 1998), rollover backstroke (229 ± 70 N; Blanksby et al., 2004), and tumble turns (693 ± 228 N; Blanksby et al., 1998) in age-group swimmers. However, this is not particularly surprising considering that the age-group swimmers from our study depicted a slower rotation with a tendency to spend a short preparatory push-off time (33%), which could lead to a lower maximum normalized peak force and impulse.
From the perspective of turn-out efficacy, the optimization of the underwater gliding depth, gliding time, and gliding distance will directly affect turning performance (Termin and Pendergast, 1998; Chainok et al., 2021). The first gliding distance and time, second gliding depth, and turn-out time were identified as key variables and appeared to be advantageous for performing an open turn. In the current study, the first and second gliding distances, and the breakout distance and time, were slightly shorter in the open turn than in the other three turns.
Somersault Turn
The key mechanical features of the turn-in phase of the somersault turn mainly depended on the time-in (7.5 and 2.5 m) and rotation time. Given the high impact of the turn-in phase on the 15 m turning performance, the swimming approach (7.5 m and 2.5 m turn-in times), and rotation times should be more deeply considered. The somersault turn, compared to the open turn findings, suggests that a faster approach could directly influence the turn time. Since the execution of the somersault turn requires a hand touch at the wall before rotating from the supine to the prone position, the rotation is critical. At this backstroke to breaststroke transition, the rotation time tended to be slightly slower than those previously studied in the rollover backstroke (Blanksby et al., 2004) and breaststroke turns (Blanksby et al., 1998) by age-group swimmers.
The analysis of the turn-out variables revealed that the wall contact time, first gliding distance, breakout distance, breakout time, and turn-out time (kinematic-temporal), dominant push-off peak_Y force (kinetic and CD2 (hydrodynamic) variables were those affecting the 15 m turn time. Based on the pull-out strategy evidence, breakout distance, breakout time, and turn-out time were identified as the important variables, indicating that age-group swimmers should select their own individual strategies by considering the breakout distance and the time to maximize the pull-out performance (Blanksby et al., 2004). The longer first gliding distance in somersault turn may be related to a lower dominant peak push-off_Y coupled with a deeper foot plant, suggesting that age-group swimmers should try to minimize the up or down movement of the all body during push-off, which could lead to a longer and deeper gliding (Blanksby et al., 2004).
Contrary to the expectations, the dominant peak push-off_Y force (about 26% of the mean peak_Z force) was selected as a critical predictor of the 15 m turn time. Theoretically, the push-off force with the feet pushing up or down directly affects the push-off velocity and tends to be inversely related with rollover time (Blanksby et al., 2004; Pereira et al., 2015). The evidence from this study points to the notion that a suitable feet push-off position and wall contact time can directly affect the performance of the subsequent horizontal push-off force and impulse (Blanksby et al., 2004), and the push-off velocity (Pereira et al., 2015).
In the discussion of turn-out performance, it is essential to consider swimmers' hydrodynamic characteristics and pull-out strategy (Chainok et al., 2021). In the somersault turn, push-off from the wall that is completely ventral and without any relevant rotation of the body may eventually lead to lower hydrodynamic drag (Pereira et al., 2015). The current study CD2 of the somersault turn was slightly high, probably due to the lower foot plant index during the push-off phase that might directly affect the gliding path adopted during the pull-out phase (see Table 3). Even so, this value tended to be higher than those obtained in national-level breaststrokers (0.61–0.72; Vilas-Boas et al., 2010) and similar to data determined by computational fluid dynamics (0.85–1.06; Marinho et al., 2011).
Bucket Turn
Multiple linear regression analysis indicated that optimal turn-in performance mainly depends on the 7.5 and 2.5 m times-in and last upper limbs-wall distance. There was a direct relationship between 15 m turn time and 7.5 m time-in (r = 0.93) and 2.5 m time-in (r = 0.85), and a small inverse relationship between 15 m turn time and last upper limbs-wall distance (r = −0.13). As in the open and somersault turns, speed-in was an essential influencing factor of turning performance, in agreement with the previous literature on elite (Nicol et al., 2019) and Olympic swimmers (Mason and Cossor, 2001). The last upper limbs-wall distance was similar among the four turning techniques (range 0.45–0.57 m), evidencing a tendency for consistency in the approaching speed, resulting in an optimal last upper limbs wall distance and leading to faster turn-in.
The foot plant index, push-off velocity, second gliding distance, and turn-out time (kinematic-temporal) and CD1 (hydrodynamic) variables were identified as the key variables for the backstroke to breaststroke turning performance. From the perspective of push-off efficacy, it is advantageous to address the appropriate lower extremity at wall contact with a greater tuck index and optimal feet planting (30–40 cm depth), which will facilitate the best horizontal push-off velocity (Clothier et al., 2000; Prins and Patz, 2006). However, the turning technique showed no main effect on push-off velocity and the linking and interaction of the kinematic variables at the wall contact and push-off phase can be considered a partial contribution of the biomechanical variables to turning performance. In the current study, the tuck index and, concomitant with a longer wall contact time tended to be higher than those for the butterfly turn (0.56 ± 0.11 s and 0.37 ± 0.09 s; Ling et al., 2004) and for the breaststroke turn (0.58 ± 0.13 s and 0.39 ± 0.08 s; Blanksby et al., 1998), performed by age-group swimmers. The foot plant index (0.55 ± 0.18) was also higher than the one previously obtained in flip turn performed by university swimmers (0.45 ± 0.10; Prins and Patz, 2006).
As determined before using inverse dynamics, the first gliding position at the breaststroke underwater path was more hydrodynamic than the second one, allowing lower S, CD, and D values for the same range of speeds (Vilas-Boas et al., 2010). The CD1 calculated in the bucket turn tended to be higher than that calculated in national-level breaststrokers (0.46 ± 0.08; Vilas-Boas et al., 2010), probably due to the lower gliding velocity and anthropometric characteristics of our age-group swimmers. Our data and the available literature also suggest that age-group swimmers need to be concerned about minimizing hydrodynamic drag by controlling their gliding position (body shape and length) along with their optimal gliding depth (range 0.4–0.6 m) (Lyttle et al., 2000; Vilas-Boas et al., 2010; Chainok et al., 2021).
Crossover Turn
We have observed that the optimal crossover turn-in performance can be identified by the 7.5 and 2.5 m times-in, average stroke length, and rotation time, with the first two variables displaying strong direct relationships with 15 m turn time and the mean stroke length relating inversely with the 15 m turn time. Notably, the turn-in time and the wall approach stroke length were the key variables in all the backstroke to breaststroke turning techniques, indicating that the wall approach strategy was consistent among them.
Theoretically, from the turn-in efficacy improvement perspective, it is important to maximize the approach speed and minimize the rotation time. In the current study, the turning technique had no main effect on rotation time, which came out as a surprise because, from a theoretical and technical perspective, differences in body rotation actions—which are characteristic of the different studied techniques, may directly affect rotation speed and turning performance. Interestingly, the implemented training program significantly improved rotation in all the backstroke to breaststroke turning techniques, inclusively with higher values than those previously presented for the rollover backstroke (0.70 ± 0.10 s; Blanksby et al., 2004), pivot breaststroke (1.15 ± 0.22 s; Blanksby et al., 1998), pivot butterfly (1.11 ± 0.18 s; Ling et al., 2004), and tumble freestyle turns (2.01 m·s−1; Blanksby et al., 1996) performed by age-group swimmers.
Multiple linear regression analysis indicated that the breakout and turn out times, non-dominant peak push-off_Y and Z forces, and D1, D2, and CD2 are turn-out performance determinants and, due to the high impact of maximized breakout distance and streamlined position on the turn-out performance, the importance of those hydrodynamic variables should be emphasized. In fact, minimizing the hydrodynamic drag should be the primary consideration for improving backstroke to breaststroke turn-out performance. Typically, the first gliding position is more hydrodynamic than the second one, allowing lower S, D, and CD values for the same range of speeds (Vilas-Boas et al., 2010; Marinho et al., 2011; Chainok et al., 2021). The crossover turn had g higher D1, D2, and CD2 values than the other studied turns, which may be justified by: (i) a worst streamline performance due to the lateral body movements that occur from the wall push-off to the first gliding position may (Lyttle et al., 1998; Termin and Pendergast, 1998) and (ii) the lower gliding velocity and control of the body shape and length while gliding. The current study Crossover D1, D2, and CD2 values were also slightly higher than previous values obtained in national-level swimmers (Vilas-Boas et al., 2010).
Our push-off force results are consistent with Araujo et al. (2010) findings indicating the highest normalized horizontal peak force contributes the most to enhancing turning performance in freestyle flip turns performed by national and international level swimmers while increasing the upward or downward wall push-off was found to have a negative impact on turn-out performance during rollover backstroke turn in age-group swimmers (Blanksby et al., 2004). Interestingly, the non-dominant Y and Z push-off forces play a critical role in determining the symmetry of lower limb push-off and subsequent gliding orientation. This finding implies that the crossover, in which the swimmer lateral push-off against the wall, may need a powerful extension of one of the lower limbs—possibly the dominant limb—to generate a symmetric push-off force.
Conclusion
The determinant variables of the different backstroke to breaststroke transition techniques change during both the turn-in and turn-out phases. Some kinematic-temporal variables are more relevant during turn-in, some kinetic variables gain relevance during turn-out (highlighting the importance of the push-off phase), and the hydrodynamic variables are important for all the studied transition techniques. Finally, the rotation and push-off phases were the stronger determinants of turning performance among all studied backstroke to breaststroke turns. Considering the key biomechanical variables that influence each turning performance in the current data, the development of a specific training program aiming to enhance turning skills, particularly focusing on the rotation and push-off phases, should be reconsidered by coaches who work with age-group swimmers, even if it implies in a longer training intervention.
Data Availability Statement
The raw data supporting the conclusions of this article will be made available by the authors, without undue reservation.
Ethics Statement
The studies involving human participants were reviewed and approved by Faculty of Sport, University of Porto. Written informed consent to participate in this study was provided by the participants' legal guardian/next of kin.
Author Contributions
PC, RZ, RF, and JV-B: conceptualization, methodology, writing—original draft preparation, and project administration. PC, KJ, RZ, LM, and PF: data curation. PC, KJ, LM, PF, RZ, RF, and JV-B: review and editing. PC and RZ: visualization. JV-B: supervision. All the authors have read and agreed to the published version of the manuscript.
Funding
This study was supported by the Faculty of the Sport Science, Burapha University, Thailand (grant number 062/2554). RZ was founded by Research Center in Physical Activity, Health and Leisure—CIAFEL—Faculty of Sports, University of Porto—FADEUP (FCT UID/DTP/00617/2020 and Laboratory for Integrative and Translational Research in Population Health (ITR), Porto, Portugal (LA/P/0064/2020).
Conflict of Interest
The authors declare that the research was conducted in the absence of any commercial or financial relationships that could be construed as a potential conflict of interest.
Publisher's Note
All claims expressed in this article are solely those of the authors and do not necessarily represent those of their affiliated organizations, or those of the publisher, the editors and the reviewers. Any product that may be evaluated in this article, or claim that may be made by its manufacturer, is not guaranteed or endorsed by the publisher.
Acknowledgments
The authors extend special thanks to the technicians of the Porto Biomechanics Laboratory (LABIOMEP-UP) for their time, collaboration and commitment to this study.
References
Araujo, L., Pereira, S., Gatti, R., Freitas, E., Jacomel, G., Roesler, H., et al. (2010). Analysis of the lateral push-off in the freestyle flip turn. J. Sports Sci. 28, 1175–1181. doi: 10.1080/02640414.2010.485207
Arellano, R., Brown, P., Cappaert, J., and Nelson, R. C. (1994). Analysis of 50-, 100-, and 200-m freestyle swimmers at the 1992 Olympic Games. J. Appl. Biomech. 10, 189–199. doi: 10.1123/jab.10.2.189
Benjanuvatra, N., Blanksby, B. A., and Elliott, B. C. (2001). Morphology and hydrodynamic resistance in young swimmers. Pediatr. Exerc. Sci. 13, 246–255. doi: 10.1123/pes.13.3.246
Blanksby, B., Gathercole, D., and Marshall, R. (1996). Force plate and video analysis of the tumble turn by age-group swimmers. J. Swimm. Res. 11, 40–45.
Blanksby, B., Skender, S., Elliott, B., McElroy, K., and Landers, G. (2004). Swimming: an analysis of the rollover backstroke turn by age-group swimmers. Sports Biomech. 3, 1–14. doi: 10.1080/14763140408522826
Blanksby, B. A., Simpson, J. R., Elliott, B. C., and McElroy, K. (1998). Biomechanical factors influencing breaststroke turns by age-group swimmers. J. Appl. Biomech. 14, 180–189. doi: 10.1123/jab.14.2.180
Chainok, P., Machado, L., de Jesus, K., Abraldes, J. A., Borgonovo-Santos, M., Fernandes, R. J., et al. (2021). Backstroke to breaststroke turning performance in age-group swimmers: hydrodynamic characteristics and pull-out strategy. Int. J. Environ. Res. Public Health 18, 1858. doi: 10.3390/ijerph18041858
Clarys, J. P. (1979). “Human morphology and hydrodynamics,” in Swimming III, eds J. Terauds, E. W. Bedingfield (Baltimore, MD: University Park Press), 3–41.
Clothier, P. J., McElroy, K., Blanksby, B., and Payne, W. R. (2000). Traditional and modified exits following freestyle tumble turns by skilled swimmers. South Afr. J. Res. Sport Phys. Educ. Recreat. 22, 41–55.
de Jesus, K., de Jesus, K., Abraldes, J. A., Mourão, L., Borgonovo-Santos, M., Medeiros, A. I., et al. (2016). The effect of different foot and hand set-up positions on backstroke start performance. Sports Biomech. 15, 481–496. doi: 10.1080/14763141.2016.1182580
de Jesus, K., Mourão, L., Roesler, H., Viriato, N., de Jesus, K., Vaz, M., et al. (2019). 3D device for forces in swimming starts and turns. Appl. Sci. 9, 3559. doi: 10.3390/app9173559
Galbraith, H., Scurr, J., Hencken, C., Wood, L., and Graham-Smith, P. (2008). Biomechanical comparison of the track start and the modified one-handed track start in competitive swimming: an intervention study. J. Appl. Biomech. 24, 307–315. doi: 10.1123/jab.24.4.307
Lauer, J., Rouard, A. H., and Vilas-Boas, J. P. (2016). Upper limb joint forces and moments during underwater cyclical movements. J. Biomech. 49, 3355–3361. doi: 10.1016/j.jbiomech.2016.08.027
Ling, B., Blanksby, B., Elliott, B., and McElroy, G. (2004). Force-time characteristics of the butterfly turns by age-group swimmers. J. Hum. Mov. Stud. 47, 429–451.
Lyttle, A., Blanksby, B., Elliott, B., and Lloyd, D. (1998). The effect of depth and velocity on drag during the streamlined glide. J. Swim. Res. 13:15–22.
Lyttle, A. D., Blanksby, B. A., Elliott, B. C., and Lloyd, D. G. (2000). Net forces during tethered simulation of underwater streamlined gliding and kicking techniques of the freestyle turn. J. Sports Sci. 18, 801–807. doi: 10.1080/026404100419856
Marinho, D. A., Barbosa, T. M., Rouboa, A. I., and Silva, A. J. (2011). The hydrodynamic study of the swimming gliding: a two-dimensional computational fluid dynamics (CFD) analysis. J. Hum. Kinet. 29, 49. doi: 10.2478/v10078-011-0039-4
Mason, B., and Cossor, J. (2001). “Swim turn performance at the Sydney 2000 Olympic Games,” in Proceedings of Swim Sessions XIX International Symposium on Biomechanics in Sports, eds J. Blackwell and R. H. Sanders (San Francisco, CA: International Society of Biomechanics in Sports), 65–69.
McGibbon, K. E., Pyne, D., Shephard, M., and Thompson, K. (2018). Pacing in swimming: a systematic review. Sports Med. 48, 1621–1633. doi: 10.1007/s40279-018-0901-9
Mourão, L., De Jesus, K., Viriato, N., Fernandes, R. J., Vilas-Boas, J. P., and Vaz, M. A. (2016). Design and construction of a 3D force plate prototype for developing an instrumented swimming start block. J. Biomed. Eng. Inform. 2, 99. doi: 10.5430/jbei.v2n2p99
Nicol, E., Ball, K., and Tor, E. (2019). The biomechanics of freestyle and butterfly turn technique in elite swimmers. Sports Biomech. 20, 444–457. doi: 10.1080/14763141.2018.1561930
Pereira, S. M., Ruschel, C., Hubert, M., Machado, L., Roesler, H., Fernandes, R. J., et al. (2015). Kinematic, kinetic and EMG analysis of four front crawl flip turn techniques. J. Sports Sci. 33, 2006–2015. doi: 10.1080/02640414.2015.1026374
Porter, J. M., and Magill, R. A. (2010). Systematically increasing contextual interference is beneficial for learning sport skills. J. Sports Sci. 28, 1277–1285. doi: 10.1080/02640414.2010.502946
Prins, J. H., and Patz, A. (2006). “The influence of tuck index, depth of foot-plant, and wall contact time on the velocity of push-off in the freestyle flip turn,” in Biomechanics and Medicine in Swimming X, eds J. P. Vilas-Boas, F. Alves, and A. Marques (Porto Faculdade de Desporto da Universidade do Porto 2006), 6, 82–85.
Schmidt, R. A., and Lee, T. D. (2005). Motor Learning and Control: A Behavioral Emphasis, 4th Edn. Champaign, IL: Human Kinetics.
Tor, E., Pease, D. L., and Ball, K. A. (2015). Key parameters of the swimming start and their relationship to start performance. J. Sports Sci. 33, 1313–1321. doi: 10.1080/02640414.2014.990486
Vilas-Boas, J. P., Costa, L., Fernandes, R. J., Ribeiro, J., Figueiredo, P., Marinho, D., et al. (2010). Determination of the drag coefficient during the first and second gliding positions of the breaststroke underwater stroke. J. Appl. Biomech. 26, 324–331. doi: 10.1123/jab.26.3.324
Webster, J., West, A., Conway, P., and Cain, M. (2011). Development of a pressure sensor for swimming turns. Proc. Eng. 13, 126–132. doi: 10.1016/j.proeng.2011.05.062
Zacca, R., Azevedo, R., Chainok, P., Vilas-Boas, J. P., Castro, F. A. d. S., Pyne, D. B., et al. (2020b). Monitoring age-group swimmers over a training macrocycle: energetics, technique, and anthropometrics. J. Strength Condit. Res. 34, 818–827. doi: 10.1519/JSC.0000000000002762
Keywords: Exercise, aquatic locomotion, swimming, biomechanics, motion capture, force plate, hydrodynamics, performance
Citation: Chainok P, de Jesus K, Mourão L, Fonseca PFP, Zacca R, Fernandes RJ and Vilas-Boas JP (2022) Biomechanical Features of Backstroke to Breaststroke Transition Techniques in Age-Group Swimmers. Front. Sports Act. Living 4:802967. doi: 10.3389/fspor.2022.802967
Received: 27 October 2021; Accepted: 01 February 2022;
Published: 10 March 2022.
Edited by:
Carla McCabe, Ulster University, United KingdomReviewed by:
Santiago Veiga, Polytechnic University of Madrid, SpainHideki Takagi, University of Tsukuba, Japan
Copyright © 2022 Chainok, de Jesus, Mourão, Fonseca, Zacca, Fernandes and Vilas-Boas. This is an open-access article distributed under the terms of the Creative Commons Attribution License (CC BY). The use, distribution or reproduction in other forums is permitted, provided the original author(s) and the copyright owner(s) are credited and that the original publication in this journal is cited, in accordance with accepted academic practice. No use, distribution or reproduction is permitted which does not comply with these terms.
*Correspondence: Phornpot Chainok, cGhvcm5wb3QmI3gwMDA0MDtnby5idXUuYWMudGg=