- 1Department of Dietetics and Human Nutrition, University of Kentucky, Lexington, KY, United States
- 24th Department of Internal Medicine and Institute of Medical Biochemistry and Laboratory Diagnostics, 1st Faculty of Medicine, Charles University and General University Hospital in Prague, Prague, Czechia
- 3Department of Athletic Training and Clinical Nutrition, University of Kentucky College of Medicine, Lexington, KY, United States
- 4Center for Muscle Biology, University of Kentucky College of Medicine, Lexington, KY, United States
- 5Department of Physiology & Biophysics, Cardiorenal, and Metabolic Diseases Research Center, University of Mississippi Medical Center, Jackson, MS, United States
- 6Department of Pharmacology and Nutritional Sciences, University of Kentucky College of Medicine, Lexington, KY, United States
- 7Barnstable Brown Diabetes Center, University of Kentucky College of Medicine, Lexington, KY, United States
- 8Markey Cancer Center, University of Kentucky, Lexington, KY, United States
Exercise performance is dependent on many factors, such as muscular strength and endurance, cardiovascular capacity, liver health, and metabolic flexibility. Recent studies show that plasma levels of bilirubin, which has classically been viewed as a liver dysfunction biomarker, are elevated by exercise training and that elite athletes may have significantly higher levels. Other studies have shown higher plasma bilirubin levels in athletes and active individuals compared to general, sedentary populations. The reason for these adaptions is unclear, but it could be related to bilirubin's antioxidant properties in response to a large number of reactive oxygen species (ROS) that originates from mitochondria during exercise. However, the mechanisms of these are unknown. Current research has re-defined bilirubin as a metabolic hormone that interacts with nuclear receptors to drive gene transcription, which reduces body weight. Bilirubin has been shown to reduce adiposity and improve the cardiovascular system, which might be related to the adaption of bilirubin increasing during exercise. No studies have directly tested if elevating bilirubin levels can influence athletic performance. However, based on the mechanisms proposed in the present review, this seems plausible and an area to consider for future studies. Here, we discuss the importance of bilirubin and exercise and how the combination might improve metabolic health outcomes and possibly athletic performance.
Introduction
Exercise training can promote the physiological health of every organ system in the body, carrying a myriad of benefits, including improving blood glucose control, cardiovascular capacity, arterial compliance, skeletal muscle function, and energy metabolism (1–4). In fact, 35 chronic diseases or conditions have been independently linked to physical inactivity (5). Most health outcomes of regular exercise, such as improving aspects of the metabolic syndrome, depend on skeletal muscle adaptations (6). However, recent data has pointed to exercise-induced benefits in liver metabolism and function playing a vital role (7–9). Exercise increases hepatic glycogen mobilization when exercise bouts are sustained beyond short bursts of high-intensity activity that rely on intramuscular stores of glucose and fat (9, 10). As hepatic glycogen is reduced with extended exercise, the liver is also responsible for the uptake of gluconeogenic precursors such as lactate, pyruvate, ketones, and glycerol (10–13). This is accomplished, in part, by exercise-induced reductions in lipogenic processes and a simultaneous increase in the lipid oxidation (14, 15), a potential mechanism for how exercise can prevent liver diseases such as non-alcoholic fatty liver disease (NAFLD) (16, 17). Interestingly, a classical liver disease biomarker, bilirubin (11), has been shown to increase with exercise (18). Studies also show that increasing bilirubin levels decreases liver fat content and reduces oxidative stress in obese mice, improving adiposity and blood glucose (19–22). Other work has shown that aerobic exercise protects the liver and cardiometabolic health and adipose tissue remodeling under metabolic stress (23). These adaptations might be linked to glucose and fatty acid metabolism during exercise, which points to well-controlled crosstalk between the liver and skeletal muscle, exchanging substrates and maintaining metabolic homeostasis. Thus, exercise-induced adaptations centered on improving substrate utilization, also termed metabolic flexibility, are not solely dependent on the skeletal muscle metabolism (9, 10).
Exercise can also play an important role in weight control by aiding in attaining an energy deficit and the metabolic adaptations in the glucose and fatty-acid metabolism (24, 25). Although other aspects of metabolic syndrome can be improved with exercise alone (without weight loss), these benefits are substantially greater when significant weight loss occurs (26). While we later discuss that plasma bilirubin levels are elevated with exercise, another facet is that it also increases during weight loss (27). With the continually prevalent obesity epidemic, exercising for weight loss will continue to be a prevailing theme in research trials. It will be interesting to see whether bilirubin will be a measurable component of future works, especially since it has many protective properties that reduce oxidative stress.
An additional adaptation to exercise that may influence substrate utilization is the upregulation of antioxidant defense systems (18, 28, 29); this is partially due to increased ROS, and reactive nitrogen species (RNS) observed with exercise (30, 31). Such free radical production during exercise can have key regulatory roles in mediating various signaling processes. However, when increases in free radicals are not met with increases in antioxidant defense, pathophysiological states such as inflammatory, cardiovascular, and neurodegenerative diseases may manifest (32). Recent research has focused on oxidative stress and exercise mechanisms, with many exploring the utility of additional antioxidant supplementation when engaging in consistent exercise (18, 33, 34). New findings have revealed that the antioxidant bilirubin may be significantly elevated in athletes (18, 35). Other recent works have shown that bilirubin has a hormonal function that reduces body weight and may be related to exercise capacity (19, 36–44). These findings point to bilirubin as an underlying mediator of exercise-induced alterations in substrate oxidation, weight loss, antioxidant status, and a surrogate to the aforementioned health outcomes (37, 38, 42, 44). Herein, we will delve into the recent literature investigating the link between bilirubin, exercise, and physiological health.
Bilirubin and exercise
Traditionally viewed as a marker for liver damage, bilirubin is becoming recognized as an important endocrine hormone and a potent antioxidant that activates nuclear receptors to control gene transcription that promotes many aspects of physiological health (cardiovascular health, blood glucose control, oxidative stress, and improves liver function) (37, 39, 43, 45). The medical community has defined “normal” total plasma bilirubin levels as 1.7–20 µmol/L, while the Child-Pugh index indicates a value of >51 µmol/L is indicative of decompensated liver cirrhosis. Large variations in plasma bilirubin are exhibited among the general population due to age, sex, ethnicity, and other biological factors. Thus, it is difficult to define a particular range for other non-clinical conditions such as long-term exercise, acute exercise, obesity, and lean individuals (46). The concept of hypobilirubinemia has been recently proposed at levels of plasma/serum bilirubin <10 µmol/L [discussed further in (37)].
Bilirubin originates from hemoglobin released from myoglobin and other hemoproteins during the destruction of senescent red blood cells. When a blood cell dies and is lysed, which occurs mostly in the spleen, heme is released and converted to biliverdin by heme oxygenase (HO), which is further metabolized to bilirubin by biliverdin reductase A (BVRA) (Figure 1) (47). Blood bilirubin levels have previously been thought only to be derived from reticuloendothelial cells in the spleen (37). However, studies in mice lacking BVRA (21, 48–50) have shown that bilirubin generation also occurs in many other tissues. Lastly, bilirubin is conjugated by the UDP-glucuronosyltransferase enzyme, UGT1A1 (51), which then deposits the conjugated bilirubin in the bile (43). Thus, it is possible to regulate plasma bilirubin levels by regulating HO, BVRA, or UGT1A1. Recently published work showed that high-capacity running rats (HCR), compared to low-capacity running rats (LCR), had significantly higher plasma bilirubin, which was likely due to hepatic BVRA being raised and UGT1A1 lowered (52). These ultimately cause higher bilirubin production by BVRA and less bilirubin clearance via UGT1A1 conjugation.
Although research connecting bilirubin and exercise is in its infancy, a limited number of studies have demonstrated that bilirubin may be increased with both acute and regular (long-term) endurance exercise in animal models and humans (52–55). This was observed in the Dose-Response to Exercise in Women Trial (DREW Trial), where participants were placed in three groups of varying exercise volumes (4, 8, or 12 kcal.kg.week) for 12 weeks, demonstrating bilirubin only increased in the 12 kcal.kg.week group, equivalent to an average of 169 min per week (54). This dose-response relationship is supported by a separate trial where 12 weeks of exercise training that progressed to 120 min per week did not influence bilirubin levels (56). Thus, exercise meeting or slightly exceeding the recommended 150 min of moderate to vigorous physical activity per week appears necessary to observe physiological (beneficial) increases in the plasma bilirubin (57). This is also supported in less controlled trials, where bilirubin increases after 3 months of soccer or rugby training in competitive athletes (58, 59) and is elevated in competitive athletes compared to the general population (35, 60). Associations have also been drawn between usual exercise behavior, where aerobic and strength training participation was positively related to plasma bilirubin levels among women. In contrast, only aerobic training participation was positively correlated in the men (61). There is also evidence that an acute bout of exercise (often exhaustive) can upregulate plasma bilirubin. This was demonstrated in trained and untrained adults and adolescents after a running time trial test to exhaustion (62). A maximal exercise test also increased plasma bilirubin among football players (63) and was increased 4 days after an ultra-marathon among trained runners (64).
An important question yet to be fully elucidated is the mechanisms induced by exercise that cause the reciprocal increase in plasma bilirubin (Figure 2). One theory is that heme catabolism could result from exercise (especially aerobic exercise) induced damage such as repeated foot strikes, elevated core temps, and skeletal muscle breakdown (myoglobin release) (65, 66). In this scenario, red blood cells may become lysed and release heme (hemolysis). This released heme can be broken down to biliverdin by heme oxygenase-1 (HO-1) and further catabolized by BVRA to eventually form a stable, unconjugated bilirubin (43). This view is supported by several of the findings above, where only the highest dose of exercise, which had the greatest exposure to factors associated with exercise-induced hemolysis, observed increases in the plasma bilirubin (54). This logic could also be applied to trained athletes exposed to very high levels of factors that may induce hemolysis to promote the observed elevations in bilirubin levels (35, 58–60). However, Swift et al. did not detect changes in hemoglobin or hematocrit following exercise training (54). This has been supported by Witek et al.'s work on athletes, who concluded that the hematological parameters did not indicate the occurrence of increased hemolysis, with no significant relationship between the total bilirubin concentration and the number of red blood cells, hemoglobin, or iron levels in the blood of trained athletes (60). These results are similar to those of Andelkovic et al., where 3 months of soccer training did not increase serum iron (likely to reflect hemolysis) nor transferrin (likely to reflect erythropoiesis due to increased hemolysis) (58). Although this study also demonstrated increased serum ferritin after training and positive correlations between bilirubin and ferritin post-training (58). Since ferritin is known to sequester iron in the blood, increased ferritin levels may mask the elevations in iron resulting from exercise-induced hemolysis; an antioxidant adaptation of ferritin has been previously demonstrated (67, 68). However, this has not been consistent across studies, with many showing no changes or decreases in ferritin after long-term exercise training in athletes’ (69–71). Other arguments against exercise-induced hemolysis driving greater bilirubin levels seen in athletes or after a long-term intervention are the notion that markers of hemolysis are typically present only immediately after intense exercise (63, 66), which would support why plasma bilirubin can increase after a bout of acute exercise (62–64).
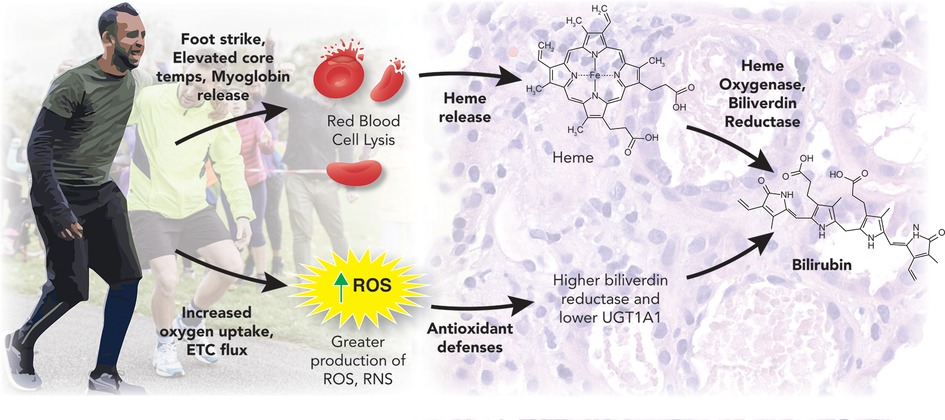
Figure 2. Exercise and bilirubin production. This figure was created by Matthew Hazzard at the University of Kentucky College of Medicine.
Another hypothesis is that exercise-induced increases in bilirubin are the result of a feedback mechanism to regulate the increased oxidative stress that accompanies physical training (35). As noted, bilirubin is a powerful antioxidant, and if following other antioxidant defense systems, it should increase with long-term exercise training to better control exercise-induced free radical damage (18, 28, 29). Indeed, the long-term exercise effect on bilirubin is associated with an increase in other antioxidant reserves as well, including total antioxidant status (35). Such increases in bilirubin would likely result from greater HO activity, which is increased with exercise training (72). Since HO is the rate-limiting enzyme necessary for converting heme to biliverdin (73), greater HO levels could force the observed increase in plasma bilirubin after long-term exercise or in physical activity individuals/athletes. Other mechanisms promoting an exercise-induced increase in plasma bilirubin could involve the enzyme that converts biliverdin to bilirubin (BVRA) (74) or the enzyme that is responsible for the removal of bilirubin from the blood into bile (UGT1A1). As noted, HCR mice demonstrated higher plasma bilirubin and increased BVRA expression while UGT1A1 was decreased compared to control animals (52). Interestingly, hepatic HO-1 was not different between the HCR mice and control, despite large differences in distance and time run to exhaustion. This indicates that exercise-induced increases in bilirubin can stem from changes in several different enzymes, including HO-1, BVRA, and UGT1A1 (Figure 1). It seems likely that long-term adaptations to exercise training promote antioxidant defenses, including bilirubin, while short-term adaptations include those related to exercise-induced damage and increased heme availability.
Gilbert’s syndrome and exercise
Although it seems that regular physical training leads to an elevation in serum bilirubin concentrations, additional considerations need to be given to Gilbert's Syndrome (GS), a genetic polymorphism that reduces UGT1A1 expression, increasing plasma bilirubin levels to potentially influence athletic performance (53). This has been demonstrated in elite Czech athletes, where elite sportsmen and sportswomen had significantly greater serum bilirubin concentrations (8.5–16 µmol/L) compared to the general population (53). At the same time, the prevalence rate of phenotypic GS syndrome was also much higher in elite athletes, suggesting that a mild elevation of serum bilirubin might predispose to better sports performance. In other words, mildly hyperbilirubinemic elite athletes could have been selected based on this biochemical trait to reach the sport's elite. This provides further evidence that bilirubin may promote athletic performance, likely related to bilirubin's role as an endocrine hormone, inducing gene transcription that modulates metabolic functions. Increased systemic concentrations of bilirubin may represent a feedback mechanism to:
a) cope with the increased oxidative stress that accompanies the training process (30),
b) provide signaling stimuli to the muscle (75) and cardiovascular system (76), improve adaptation to physical training stress, and simultaneously,
c) provide substantial metabolic advantages regarding fatty acid oxidation associated with regular exercise (77).
These conclusions are based on recent observations. Regular exercise has been associated with increased antioxidant capacity, similar to a previous report documenting an exercise-induced increase in other body antioxidant reserves (62). In addition, bilirubin is an important signaling molecule (78, 79), fulfilling parameters of the endocrine substance (45). Therefore, these activities are highly likely to contribute to the beneficiary metabolic adaptations associated with regular training.
Bilirubin and cardiovascular system as a benefit for exercise
Increased plasma bilirubin levels can have several beneficial effects on the cardiovascular system in the context of exercise (Figure 3). First, bilirubin is a potent antioxidant compound that can scavenge ROS both directly and through the inhibition of the NAD(P)H oxidase (80, 81). One of the main targets of the ROS product superoxide anion (O2•) is nitric oxide (NO). Superoxide interacts with NO to form peroxynitrite radical, damaging DNA and nitrosylate tyrosine residues, which disrupts protein function. By limiting the production and actions of superoxide, bilirubin can increase the bioavailability of NO to preserve the blood flow (82–84). The preservation of blood flow through enhanced NO bioavailability may mediate the improvement in athletic performance observed with increased levels of plasma bilirubin (35). Bilirubin mimics the protective actions of HO-1 induction and restores attenuated eNOS expression after exposure to oxLDL and TNF-α (85). The hyperbilirubinemic Gunn rat is resistant to the pressor actions of angiotensin II, and bilirubin can attenuate the release of endothelin-1 (86, 87). These findings demonstrate that bilirubin has vasoprotective actions, which could be beneficial to maintaining blood flow during exercise.
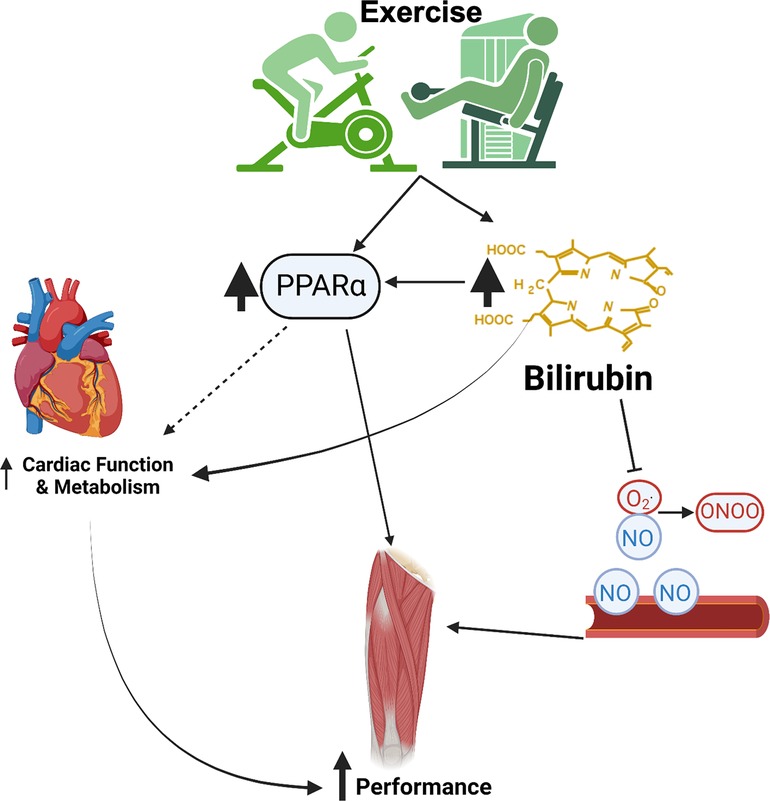
Figure 3. Cardiovascular benefits of exercise and bilirubin. This image was made using Biorender.com.
Recent studies have indicated that bilirubin functions as a hormone to activate the nuclear receptor peroxisome proliferator-activated receptor alpha (PPARα). It has been proposed that low plasma bilirubin levels should be considered a pathological state (37, 44). PPARα activation in the liver is a contributory factor to the exercise-related improvements in the whole-body metabolism (88). In fact, induction of PPARα in the vasculature by exercise has recently been proposed as a therapy to fight COVID-19 infection (89). Gene polymorphisms in PPARα increase physical and aerobic performance and are associated with muscle fiber type composition in athletes’ (90, 91). Twice a day, close proximity exercise is associated with enhanced mitochondrial biogenesis, fat oxidation, and upregulation of skeletal muscle PPARα (92). Likewise, treatment with the PPARα agonist, fenofibrate, increases soleus muscle weight and enhances musculoskeletal training response during estrogen deficiency in ovariectomized (OVX) Sprague Dawley rats (93). Exercise training also decreases the age-related decline in cardiac PPARα levels in rats (94). PPARα knockout mice exhibited reduced lipolysis and anti-inflammatory responses in adipose tissue following exercise (95). The adipose-specific PPARα KO (96) and liver-specific PPARα KO (97) animals exhibited adiposity in the null tissues, which further indicates the importance of the bilirubin-PPARα circuit.
PPARα affects changes in metabolism central to exercise adaptation and muscle stem cell dynamics. Satellite cells, the bonafide muscle stem cell, support skeletal muscle exercise adaptation through activation and fusion into muscle fibers (98–100). Exercise-induced satellite cell activation is reliant on dynamic metabolic reprogramming culminating in robust activation of oxidative metabolism during the terminal differentiation (101). PPARα is a critical regulator of muscle lipid homeostasis to facilitate differentiation of human satellite cells in vitro to support subsequent fusion into muscle fibers to facilitate exercise-induced adaptation (102, 103). Furthermore, skeletal muscle is a mosaic of different fiber “types” uniquely defined by their metabolic requirement. The targeting by PPARα of genes involved in cellular fatty acid import and binding help define a unique cellular identity for PPARα in oxidative type I fibers versus the predominantly glycolytic type II muscle fibers (104). Greater demand for mitochondrial biogenesis and oxidative metabolism that occur in response to chronic exercise supports a fiber type-specific role for PPARα-mediated transcription. Variance in human type I fiber distribution is closely associated with PPARα expression, offering further support for PPARα in the distinct metabolic requirements of oxidative, slow twitch type I fibers (105). Further studies in PPARα deficient animals are needed in order to fully elucidate the role of PPARα activation in response to increases in bilirubin production in exercise.
Bilirubin is also cardio-protective, and increased bilirubin levels during exercise may benefit the heart. For example, studies in hyperbilirubinemic Gunn rats demonstrate that bilirubin protects the heart from reperfusion injury and beneficially influences aortic ejection velocities and pressures, improving cardiac performance during exercise (106, 107). Recent studies have demonstrated that bilirubin can increase the production of hepatic ketone beta-hydroxybutyrate (BOHB) (19), which likely occurred via PPARα mechanisms. A diet supplemented with BOHB precursors improved exercise performance in rats (108). Ketones may play an important role in the metabolic adaptation of the heart to exercise, especially in type II diabetic patients who are unable to effectively utilize glucose as a cardiac energy source. While the protective actions of bilirubin on the heart have largely been explained through its potent antioxidant activity, the effects of bilirubin on cardiac metabolism remain to be thoroughly studied. It is possible that bilirubin plays an important role in the metabolic adaptation of the heart to exercise both directly and indirectly through its action on PPARα and hepatic production of BOHB.
There is mounting evidence pointing to bilirubin as an important hormonal molecule and antioxidant, a departure from the traditional view that the role of bilirubin was limited to a marker for liver dysfunction. Bilirubin's role in mediating metabolic adaptations and protecting from oxidative stress is now evident, most notably in the context of cardiovascular disease and obesity. The present review has further explored the role exercise training appears to have on bilirubin levels, outlining two primary metabolic pathways activated by exercise that promote slight elevations in plasma bilirubin. The first of these pathways are related to heme catabolism, where exercise-induced damage such as muscle strain causing myoglobin release, elevated core temperature, and repetitive foot strike causes red blood cell lysis and heme release. Using heme as a precursor, through actions of the HO and BVRA enzymes, bilirubin synthesis is increased. This pathway seems to be impacted primarily by acute exercise, as reductions in hemolysis can be a long-term training adaptation observed among athletes (69–71). An additional pathway that can increase exercise-induced elevations in plasma bilirubin is related to an upregulation of antioxidant defense mechanisms. Just as other antioxidant enzymes are increased in response to elevations in ROS and RNS that accompany exercise (62), including total antioxidant status (35), BVRA can be increased while the enzyme UGT1A1 is decreased, thus promoting the synthesis and increased plasma levels of bilirubin (52). The combination of both pathways explains how both long-term and acute exercise can promote bilirubin levels and why athletes have consistently demonstrated greater plasma bilirubin levels compared to the general population.
Conclusion
Although no studies have directly tested if increasing plasma bilirubin levels promote improved exercise performance, this hypothesis seems probable with the evidence presented and an area for future research exploration. Preliminary evidence that supports this hypothesis is related to studies on GS and elite athlete performance. These individuals have a specific genetic polymorphism that causes elevated plasma bilirubin, where a far greater prevalence of GS is observed in elite athletes. This suggests that individuals with greater bilirubin levels might be predisposed to greater athletic performance. This could be related to bilirubin's role as a hormonal signaling molecule, where bilirubin interacts with PPARα to stimulate gene transcription related to fatty acid oxidative and mitochondrial capacities, important mediators in muscle function and exercise performance (102, 103). Improving antioxidant defenses through elevations in bilirubin is also desirable for athletic performance, likely related to enhanced bioavailability of NO and increased blood flow (35, 82–84). Controlled trials in humans testing the potential utility of bilirubin playing an ergogenic role in exercise performance are lacking and, thus, an additional avenue for future investigation. The optimal level of plasma bilirubin has also not been defined for health or athletic performance, another important question that research may address. Altogether, future work to determine whether increasing plasma bilirubin levels are useful for enhancing athletic performance is needed before research can focus on ergogenic aids to increase plasma bilirubin. In the least, bilirubin is an important molecule and new hormone that improves metabolic function and could be an essential metabolite of exercise performance and weight loss.
Author contributions
All authors listed have made a substantial, direct, and intellectual contribution to the work and approved it for publication.
Funding
This work was supported by the National Institutes of Health R01DK121797 (TH), R01DK126884 (DS), R01AR072061 (CF), and the National Heart, Lung and Blood Institute P01 HL05197-11 (DS) and K01HL125445 (TH), and the National Institute of General Medical Sciences P20GM104357-02 (DS). This study was also supported by a grant MH CZ-DRO-VFN64165 (LV) from the Czech Ministry of Health.
Conflict of interest
TH and DS have submitted patents on bilirubin and obesity-related disorders. The remaining authors declare that the research was conducted in the absence of any commercial or financial relationships that could be construed as a potential conflict of interest.
Publisher's note
All claims expressed in this article are solely those of the authors and do not necessarily represent those of their affiliated organizations, or those of the publisher, the editors and the reviewers. Any product that may be evaluated in this article, or claim that may be made by its manufacturer, is not guaranteed or endorsed by the publisher.
References
1. Sargeant JA, Gray LJ, Bodicoat DH, Willis SA, Stensel DJ, Nimmo MA, et al. The effect of exercise training on intrahepatic triglyceride and hepatic insulin sensitivity: a systematic review and meta-analysis. Obes Rev. (2018) 19(10):1446–59. doi: 10.1111/obr.12719
2. Fiuza-Luces C, Santos-Lozano A, Joyner M, Carrera-Bastos P, Picazo O, Zugaza JL, et al. Exercise benefits in cardiovascular disease: beyond attenuation of traditional risk factors. Nat Rev Cardiol. (2018) 15(12):731–43. doi: 10.1038/s41569-018-0065-1
3. Ho SS, Dhaliwal SS, Hills AP, Pal S. The effect of 12 weeks of aerobic, resistance or combination exercise training on cardiovascular risk factors in the overweight and obese in a randomized trial. BMC Public Health. (2012) 12:704. doi: 10.1186/1471-2458-12-704
4. Holloszy JO. Adaptations of skeletal muscle mitochondria to endurance exercise: a personal perspective. Exerc Sport Sci Rev. (2004) 32(2):41–3. doi: 10.1097/00003677-200404000-00001
5. Booth FW, Roberts CK, Thyfault JP, Ruegsegger GN, Toedebusch RG. Role of inactivity in chronic diseases: evolutionary insight and pathophysiological mechanisms. Physiol Rev. (2017) 97(4):1351–402. doi: 10.1152/physrev.00019.2016
6. Smedlund KB, Sanchez ER, Hinds TD Jr. FKBP51 And the molecular chaperoning of metabolism. Trends Endocrinol Metab. (2021) 32(11):862–74. doi: 10.1016/j.tem.2021.08.003
7. Denou E, Marcinko K, Surette MG, Steinberg GR, Schertzer JD. High-intensity exercise training increases the diversity and metabolic capacity of the mouse distal gut microbiota during diet-induced obesity. Am J Physiol Endocrinol Metab. (2016) 310(11):E982–93. doi: 10.1152/ajpendo.00537.2015
8. Carbajo-Pescador S, Porras D, García-Mediavilla MV, Martínez-Flórez S, Juarez-Fernández M, Cuevas MJ, et al. Beneficial effects of exercise on gut microbiota functionality and barrier integrity, and gut-liver crosstalk in an in vivo model of early obesity and non-alcoholic fatty liver disease. Dis Model Mech. (2019) 12(5):dmm039206. doi: 10.1242/dmm.039206
9. Thyfault JP, Bergouignan A. Exercise and metabolic health: beyond skeletal muscle. Diabetologia. (2020) 63(8):1464–74. doi: 10.1007/s00125-020-05177-6
10. Trefts E, Williams AS, Wasserman DH. Exercise and the regulation of hepatic metabolism. Prog Mol Biol Transl Sci. (2015) 135:203–25. doi: 10.1016/bs.pmbts.2015.07.010
11. Badmus OO, Hillhouse SA, Anderson CD, Hinds TD, Stec DE. Molecular mechanisms of metabolic associated fatty liver disease (MAFLD): functional analysis of lipid metabolism pathways. Clin Sci. (2022) 136(18):1347–66. doi: 10.1042/CS20220572
12. Hu C, Hoene M, Plomgaard P, Hansen JS, Zhao X, Li J, et al. Muscle-liver substrate fluxes in exercising humans and potential effects on hepatic metabolism. J Clin Endocrinol Metab. (2020) 105(4):1196–1209. doi: 10.1210/clinem/dgz266
13. Marino JS, Stechschulte LA, Stec DE, Nestor-Kalinoski A, Coleman S, Hinds TD Jr. Glucocorticoid receptor beta induces hepatic steatosis by augmenting inflammation and inhibition of the peroxisome proliferator-activated receptor (PPAR) alpha. J Biol Chem. (2016) 291(50):25776–788. doi: 10.1074/jbc.M116.752311
14. Rector RS, Uptergrove GM, Morris EM, Borengasser SJ, Laughlin MH, Booth FW, et al. Daily exercise vs. caloric restriction for prevention of nonalcoholic fatty liver disease in the OLETF rat model. Am J Physiol Gastrointest Liver Physiol. (2011) 300(5):G874–83. doi: 10.1152/ajpgi.00510.2010
15. Linden MA, Fletcher JA, Morris EM, Meers GM, Laughlin MH, Booth FW, et al. Treating NAFLD in OLETF rats with vigorous-intensity interval exercise training. Med Sci Sports Exerc. (2015) 47(3):556–67. doi: 10.1249/MSS.0000000000000430
16. Thyfault JP, Rector RS. Exercise combats hepatic steatosis: potential mechanisms and clinical implications. Diabetes. (2020) 69(4):517–24. doi: 10.2337/dbi18-0043
17. Creeden JF, Kipp ZA, Xu M, Flight RM, Moseley HNB, Martinez GJ, et al. Hepatic kinome atlas: an in-depth identification of kinase pathways in liver fibrosis of humans and rodents. Hepatology. (2022) 76(5):1376–88. doi: 10.1002/hep.32467
18. Thomas DT, DelCimmuto NR, Flack KD, Stec DE, Hinds TD Jr. Reactive oxygen Species (ROS) and antioxidants as immunomodulators in exercise: implications for heme oxygenase and bilirubin. Antioxidants. (2022) 11(2):179. doi: 10.3390/antiox11020179
19. Hinds TD Jr, Creeden JF, Gordon DM, Stec DF, Donald MC, Stec DE. Bilirubin nanoparticles reduce diet-induced hepatic steatosis, improve fat utilization, and increase plasma beta-hydroxybutyrate. Front Pharmacol. (2020) 11:594574. doi: 10.3389/fphar.2020.594574
20. Hinds TD Jr, Hosick PA, Hankins MW, Nestor-Kalinoski A, Stec DE. Mice with hyperbilirubinemia due to Gilbert's syndrome polymorphism are resistant to hepatic steatosis by decreased serine 73 phosphorylation of PPARalpha. Am J Physiol Endocrinol Metab. (2017) 312(4):E244–E252. doi: 10.1152/ajpendo.00396.2016
21. Hinds TD Jr, Burns KA, Hosick PA, McBeth L, Nestor-Kalinoski A, Drummond HA, et al. Biliverdin reductase A attenuates hepatic steatosis by inhibition of glycogen synthase kinase (GSK) 3beta phosphorylation of serine 73 of peroxisome proliferator-activated receptor (PPAR) alpha. J Biol Chem. (2016) 291(48):25179–191. doi: 10.1074/jbc.M116.731703
22. Gordon DM, Adeosun SO, Ngwudike SI, Anderson CD, Hall JE, Hinds TD Jr, et al. CRISPR Cas9-mediated deletion of biliverdin reductase A (BVRA) in mouse liver cells induces oxidative stress and lipid accumulation. Arch Biochem Biophys. (2019) 672:108072. doi: 10.1016/j.abb.2019.108072
23. Kim YJ, Kim HJ, Lee SG, Kim DH, In Jang S, Go HS, et al. Aerobic exercise for eight weeks provides protective effects towards liver and cardiometabolic health and adipose tissue remodeling under metabolic stress for one week: a study in mice. Metab Clin Exp. (2022) 130:155178. doi: 10.1016/j.metabol.2022.155178
24. Flack KD, Hays HM, Moreland J, Long DE. Exercise for weight loss: further evaluating energy compensation with exercise. Med Sci Sports Exerc. (2020) 52(11):2466–75. doi: 10.1249/MSS.0000000000002376
25. Berggren JR, Hulver MW, Dohm GL, Houmard JA. Weight loss and exercise: implications for muscle lipid metabolism and insulin action. Med Sci Sports Exerc. (2004) 36(7):1191–5. doi: 10.1249/01.MSS.0000074670.03001.98
26. Mora-Rodriguez R, Ortega JF, Ramirez-Jimenez M, Moreno-Cabanas A, Morales-Palomo F. Insulin sensitivity improvement with exercise training is mediated by body weight loss in subjects with metabolic syndrome. Diabetes Metab. (2019) 46(3):210–218. doi: 10.1016/j.diabet.2019.05.004
27. Andersson C, Weeke P, Fosbøl EL, Brendorp B, Køber L, Coutinho W, et al. Acute effect of weight loss on levels of total bilirubin in obese, cardiovascular high-risk patients: an analysis from the lead-in period of the sibutramine cardiovascular outcome trial. Metab Clin Exp. (2009) 58(8):1109–15. doi: 10.1016/j.metabol.2009.04.003
28. Ji LL. Exercise-induced modulation of antioxidant defense. Ann N Y Acad Sci. (2002) 959:82–92. doi: 10.1111/j.1749-6632.2002.tb02085.x
29. Vargas-Mendoza N, Morales-Gonzalez A, Madrigal-Santillan EO, Madrigal-Bujaidar E, Alvarez-Gonzalez I, Garcia-Melo LF, et al. Antioxidant and adaptative response mediated by Nrf2 during physical exercise. Antioxidants. (2019) 8(6):196. doi: 10.3390/antiox8060196
30. Radak Z, Chung HY, Koltai E, Taylor AW, Goto S. Exercise, oxidative stress and hormesis. Ageing Res Rev. (2008) 7(1):34–42. doi: 10.1016/j.arr.2007.04.004
31. Radak Z, Chung HY, Goto S. Exercise and hormesis: oxidative stress-related adaptation for successful aging. Biogerontology. (2005) 6(1):71–5. doi: 10.1007/s10522-004-7386-7
32. Pingitore A, Lima GP, Mastorci F, Quinones A, Iervasi G, Vassalle C. Exercise and oxidative stress: potential effects of antioxidant dietary strategies in sports. Nutrition. (2015) 31(7-8):916–22. doi: 10.1016/j.nut.2015.02.005
33. Mason SA, Trewin AJ, Parker L, Wadley GD. Antioxidant supplements and endurance exercise: current evidence and mechanistic insights. Redox Biol. (2020) 35:101471. doi: 10.1016/j.redox.2020.101471
34. Higgins MR, Izadi A, Kaviani M. Antioxidants and exercise performance: with a focus on vitamin E and C supplementation. Int J Environ Res Public Health. (2020) 17(22):8452. doi: 10.3390/ijerph17228452
35. Woronyczova J, Novakova M, Lenicek M, Batovsky M, Bolek E, Cifkova R, et al. Serum bilirubin concentrations and the prevalence of gilbert syndrome in elite athletes. Sports Med Open. (2022) 8(1):84. doi: 10.1186/s40798-022-00463-6
36. Gordon DM, Hong SH, Kipp ZA, Hinds TD Jr. Identification of binding regions of bilirubin in the ligand-binding pocket of the peroxisome proliferator-activated receptor-A (PPARalpha). Molecules. (2021) 26(10):2975. doi: 10.3390/molecules26102975
37. Creeden JF, Gordon DM, Stec DE, Hinds TD Jr. Bilirubin as a metabolic hormone: the physiological relevance of low levels. Am J Physiol Endocrinol Metab. (2021) 320(2):E191–207. doi: 10.1152/ajpendo.00405.2020
38. Gordon DM, Neifer KL, Hamoud AA, Hawk CF, Nestor-Kalinoski AL, Miruzzi SA, et al. Bilirubin remodels murine white adipose tissue by reshaping mitochondrial activity and the coregulator profile of peroxisome proliferator-activated receptor alpha. J Biol Chem. (2020) 295(29):9804–22. doi: 10.1074/jbc.RA120.013700
39. Hinds TD Jr, Stec DE. Bilirubin safeguards cardiorenal and metabolic diseases: a protective role in health. Curr Hypertens Rep. (2019) 21(11):87. doi: 10.1007/s11906-019-0994-z
40. Gordon DM, Blomquist TM, Miruzzi SA, McCullumsmith R, Stec DE, Hinds TD Jr. RNA sequencing in human HepG2 hepatocytes reveals PPAR-alpha mediates transcriptome responsiveness of bilirubin. Physiol Genomics. (2019) 51(6):234–40. doi: 10.1152/physiolgenomics.00028.2019
41. Weaver L, Hamoud AR, Stec DE, Hinds TD Jr. Biliverdin reductase and bilirubin in hepatic disease. Am J Physiol Gastrointest Liver Physiol. (2018) 314(6):G668–G76. doi: 10.1152/ajpgi.00026.2018
42. Hinds TD Jr, Stec DE. Bilirubin, a cardiometabolic signaling molecule. Hypertension. (2018) 72(4):788–95. doi: 10.1161/HYPERTENSIONAHA.118.11130
43. Hamoud AR, Weaver L, Stec DE, Hinds TD Jr. Bilirubin in the liver-gut signaling axis. Trends Endocrinol Metab. (2018) 29(3):140–150. doi: 10.1016/j.tem.2018.01.002
44. Stec DE, John K, Trabbic CJ, Luniwal A, Hankins MW, Baum J, et al. Bilirubin binding to PPARalpha inhibits lipid accumulation. PLoS One. (2016) 11(4):e0153427. doi: 10.1371/journal.pone.0153427
45. Vitek L, Tiribelli C. Bilirubin: the yellow hormone? J Hepatol. (2021) 75(6):1485–90. doi: 10.1016/j.jhep.2021.06.010
46. Fischbach F. A manual of laboratory and diagnostic tests. 9th ed Philadelphia, PA: Lippincott Williams & Wilkins (2014).
47. O'Brien L, Hosick PA, John K, Stec DE, Hinds TD Jr. Biliverdin reductase isozymes in metabolism. Trends Endocrinol Metab. (2015) 26(4):212–20. doi: 10.1016/j.tem.2015.02.001
48. Stec DE, Gordon DM, Nestor-Kalinoski AL, Donald MC, Mitchell ZL, Creeden JF, et al. Biliverdin reductase A (BVRA) knockout in adipocytes induces hypertrophy and reduces mitochondria in white fat of obese mice. Biomolecules. (2020) 10(3):387. doi: 10.3390/biom10030387
49. Chen W, Tumanov S, Fazakerley DJ, Cantley J, James DE, Dunn LL, et al. Bilirubin deficiency renders mice susceptible to hepatic steatosis in the absence of insulin resistance. Redox Biol. (2021) 47:102152. doi: 10.1016/j.redox.2021.102152
50. Chen W, Maghzal GJ, Ayer A, Suarna C, Dunn LL, Stocker R. Absence of the biliverdin reductase-a gene is associated with increased endogenous oxidative stress. Free Radic Biol Med. (2018) 115:156–65. doi: 10.1016/j.freeradbiomed.2017.11.020
51. Sundararaghavan VL, Sindhwani P, Hinds TD Jr. Glucuronidation and UGT isozymes in bladder: new targets for the treatment of uroepithelial carcinomas? Oncotarget. (2017) 8(2):3640–8. doi: 10.18632/oncotarget.12277
52. Hinds TD Jr, Creeden JF, Gordon DM, Spegele AC, Britton SL, Koch LG, et al. Rats genetically selected for high aerobic exercise capacity have elevated plasma bilirubin by upregulation of hepatic biliverdin reductase-A (BVRA) and suppression of UGT1A1. Antioxidants. (2020) 9(9):889. doi: 10.3390/antiox9090889
53. Woronyczova J, Novákova M, Lenicek M, Batovsky M, Bolek E, Cifkova R, et al. Serum bilirubin concentrations and the prevalence of gilbert syndrome in elite athletes. Sports Med Open Access. (2022) 8(1):84. doi: 10.1186/s40798-022-00463-6
54. Swift DL, Johannsen NM, Earnest CP, Blair SN, Church TS. Effect of different doses of aerobic exercise training on total bilirubin levels. Med Sci Sports Exerc. (2012) 44(4):569–74. doi: 10.1249/MSS.0b013e3182357dd4
55. Kabasakalis A, Tsalis G, Zafrana E, Loupos D, Mougios V. Effects of endurance and high-intensity swimming exercise on the redox status of adolescent male and female swimmers. J Sports Sci. (2014) 32(8):747–56. doi: 10.1080/02640414.2013.850595
56. Devries MC, Samjoo IA, Hamadeh MJ, Tarnopolsky MA. Effect of endurance exercise on hepatic lipid content, enzymes, and adiposity in men and women. Obesity. (2008) 16(10):2281–8. doi: 10.1038/oby.2008.358
57. Stamatakis E, Straker L, Hamer M, Gebel K. The 2018 physical activity guidelines for Americans: what's new? Implications for clinicians and the public. J Orthop Sports Phys Ther. (2019) 49(7):487–90. doi: 10.2519/jospt.2019.0609
58. Anđelković M, Baralić I, Đorđević B, Stevuljević JK, Radivojević N, Dikić N, et al. Hematological and biochemical parameters in elite soccer players during A competitive half season. J Med Biochem. (2015) 34(4):460–6. doi: 10.2478/jomb-2014-0057
59. Banfi G, Di Gaetano N, Lopez RS, Melegati G. Decreased mean sphered cell volume values in top-level rugby players are related to the intravascular hemolysis induced by exercise. Lab Hematol. (2007) 13(3):103–7. doi: 10.1532/LH96.07012
60. Witek K, Scislowska J, Turowski D, Lerczak K, Lewandowska-Pachecka S, Pokrywka A. Total bilirubin in athletes, determination of reference range. Biol Sport. (2017) 34(1):45–8. doi: 10.5114/biolsport.2017.63732
61. Fragala MS, Bi C, Chaump M, Kaufman HW, Kroll MH. Associations of aerobic and strength exercise with clinical laboratory test values. PLoS One. (2017) 12(10):e0180840. doi: 10.1371/journal.pone.0180840
62. Zalavras A, Fatouros IG, Deli CK, Draganidis D, Theodorou AA, Soulas D, et al. Age-related responses in circulating markers of redox status in healthy adolescents and adults during the course of a training macrocycle. Oxid Med Cell Longev. (2015) 2015:283921. doi: 10.1155/2015/283921
63. Hammouda O, Chtourou H, Chaouachi A, Chahed H, Ferchichi S, Kallel C, et al. Effect of short-term maximal exercise on biochemical markers of muscle damage, total antioxidant status, and homocysteine levels in football players. Asian J Sports Med. (2012) 3(4):239–46. doi: 10.5812/asjsm.34544
64. Fallon KE, Sivyer G, Sivyer K, Dare A. The biochemistry of runners in a 1600 km ultramarathon. Br J Sports Med. (1999) 33(4):264–9. doi: 10.1136/bjsm.33.4.264
65. Senturk UK, Gunduz F, Kuru O, Kocer G, Ozkaya YG, Yesilkaya A, et al. Exercise-induced oxidative stress leads hemolysis in sedentary but not trained humans. J Appl Physiol. (2005) 99(4):1434–41. doi: 10.1152/japplphysiol.01392.2004
66. Miller BJ, Pate RR, Burgess W. Foot impact force and intravascular hemolysis during distance running. Int J Sports Med. (1988) 9(1):56–60. doi: 10.1055/s-2007-1024979
67. Orino K, Lehman L, Tsuji Y, Ayaki H, Torti SV, Torti FM. Ferritin and the response to oxidative stress. Biochem J. (2001) 357(Pt 1):241–7. doi: 10.1042/bj3570241
68. Meneghini R. Iron homeostasis, oxidative stress, and DNA damage. Free Radic Biol Med. (1997) 23(5):783–92. doi: 10.1016/S0891-5849(97)00016-6
69. Pizza FX, Flynn MG, Boone JB, Rodriguez-Zayas JR, Andres FF. Serum haptoglobin and ferritin during a competitive running and swimming season. Int J Sports Med. (1997) 18(4):233–7. doi: 10.1055/s-2007-972625
70. Deruisseau KC, Roberts LM, Kushnick MR, Evans AM, Austin K, Haymes EM. Iron status of young males and females performing weight-training exercise. Med Sci Sports Exerc. (2004) 36(2):241–8. doi: 10.1249/01.MSS.0000113483.13339.7B
71. Malcovati L, Pascutto C, Cazzola M. Hematologic passport for athletes competing in endurance sports: a feasibility study. Haematologica. (2003) 88(5):570–81. doi: 10.3324/%25x
72. Abraham NG, Kappas A. Pharmacological and clinical aspects of heme oxygenase. Pharmacol Rev. (2008) 60(1):79–127. doi: 10.1124/pr.107.07104
73. Stec DE, Hinds TD Jr. Natural product heme oxygenase inducers as treatment for nonalcoholic fatty liver disease. Int J Mol Sci. (2020) 21(24):9493. doi: 10.3390/ijms21249493
74. Adeosun SO, Moore KH, Lang DM, Nwaneri AC, Hinds TD Jr, Stec DE. A novel fluorescence-based assay for the measurement of biliverdin reductase activity. React Oxyg Species. (2018) 5(13):35–45. doi: 10.20455/ros.2018.809
75. Petriz BA, Gomes CP, Almeida JA, de Oliveira GP Jr, Ribeiro FM, Pereira RW, et al. The effects of acute and chronic exercise on skeletal muscle proteome. J Cell Physiol. (2017) 232(2):257–69. doi: 10.1002/jcp.25477
76. Hellsten Y, Nyberg M. Cardiovascular adaptations to exercise training. Comp Physiol. (2016) 6:1–32. doi: 10.1002/cphy.c140080
77. Ferraro E, Giammarioli AM, Chiandotto S, Spoletini I, Rosano G. Exercise-induced skeletal muscle remodeling and metabolic adaptation: redox signaling and role of autophagy. Antioxid Redox Signal. (2014) 21(1):154–76. doi: 10.1089/ars.2013.5773
78. Vitek L. Bilirubin as a signaling molecule. Med Res Rev. (2020) 40(4):1335–51. doi: 10.1002/med.21660
79. Hinds TD Jr, Adeosun SO, Alamodi AA, Stec DE. Does bilirubin prevent hepatic steatosis through activation of the PPARalpha nuclear receptor? Med Hypotheses. (2016) 95:54–7. doi: 10.1016/j.mehy.2016.08.013
80. Stocker R, Yamamoto Y, McDonagh AF, Glazer AN, Ames BN. Bilirubin is an antioxidant of possible physiological importance. Science. (1987) 235(4792):1043–6. doi: 10.1126/science.3029864
81. Lanone S, Bloc S, Foresti R, Almolki A, Taille C, Callebert J, et al. Bilirubin decreases nos2 expression via inhibition of NAD(P)H oxidase: implications for protection against endotoxic shock in rats. FASEB J. (2005) 19(13):1890–2. doi: 10.1096/fj.04-2368fje
82. Vera T, Granger JP, Stec DE. Inhibition of bilirubin metabolism induces moderate hyperbilirubinemia and attenuates ANG II-dependent hypertension in mice. Am J Physiol Regul Integr Comp Physiol. (2009) 297(3):R738–R43. doi: 10.1152/ajpregu.90889.2008
83. Stec DE, Hosick PA, Granger JP. Bilirubin, renal hemodynamics, and blood pressure. Front Pharmacol. (2012) 3:18. doi: 10.3389/fphar.2012.00018
84. Vera T, Stec DE. Moderate hyperbilirubinemia improves renal hemodynamics in ANG II-dependent hypertension. Am J Physiol Regul Integr Comp Physiol. (2010) 299(4):R1044–R9. doi: 10.1152/ajpregu.00316.2010
85. Kawamura K, Ishikawa K, Wada Y, Kimura S, Matsumoto H, Kohro T, et al. Bilirubin from heme oxygenase-1 attenuates vascular endothelial activation and dysfunction. Arterioscler Thromb Vasc Biol. (2005) 25(1):155–60. doi: 10.1161/01.ATV.0000148405.18071.6a
86. Pflueger A, Croatt AJ, Peterson TE, Smith LA, d'Uscio LV, Katusic ZS, et al. The hyperbilirubinemic gunn rat is resistant to the pressor effects of angiotensin II. Am J Physiol Renal Physiol. (2005) 288(3):F552–F8. doi: 10.1152/ajprenal.00278.2004
87. Bakrania BA, Spradley FT, Satchell SC, Stec DE, Rimoldi JM, Gadepalli RSV, et al. Heme oxygenase-1 is a potent inhibitor of placental ischemia-mediated endothelin-1 production in cultured human glomerular endothelial cells. Am J Physiol Regul Integr Comp Physiol. (2018) 314(3):R427–R32. doi: 10.1152/ajpregu.00370.2017
88. Zhang S, Liu Y, Li Q, Dong X, Hu H, Hu R, et al. Exercise improved rat metabolism by raising PPAR-alpha. Int J Sports Med. (2011) 32(8):568–73. doi: 10.1055/s-0031-1271755
89. Heffernan KS, Ranadive SM, Jae SY. Exercise as medicine for COVID-19: on PPAR with emerging pharmacotherapy. Med Hypotheses. (2020) 143:110197. doi: 10.1016/j.mehy.2020.110197
90. Ahmetov II, Mozhayskaya IA, Flavell DM, Astratenkova IV, Komkova AI, Lyubaeva EV, et al. PPARalpha gene variation and physical performance in Russian athletes. Eur J Appl Physiol. (2006) 97(1):103–8. doi: 10.1007/s00421-006-0154-4
91. Tural E, Kara N, Agaoglu SA, Elbistan M, Tasmektepligil MY, Imamoglu O. PPAR-alpha and PPARGC1A gene variants have strong effects on aerobic performance of turkish elite endurance athletes. Mol Biol Rep. (2014) 41(9):5799–804. doi: 10.1007/s11033-014-3453-6
92. Andrade-Souza VA, Ghiarone T, Sansonio A, Santos Silva KA, Tomazini F, Arcoverde L, et al. Exercise twice-a-day potentiates markers of mitochondrial biogenesis in men. FASEB J. (2020) 34(1):1602–19. doi: 10.1096/fj.201901207RR
93. Mosti MP, Ericsson M, Erben RG, Schuler C, Syversen U, Stunes AK. The PPARalpha agonist fenofibrate improves the musculoskeletal effects of exercise in ovariectomized rats. Endocrinology. (2016) 157(10):3924–34. doi: 10.1210/en.2016-1114
94. Iemitsu M, Miyauchi T, Maeda S, Tanabe T, Takanashi M, Irukayama-Tomobe Y, et al. Aging-induced decrease in the PPAR-alpha level in hearts is improved by exercise training. Am J Physiol Heart Circ Physiol. (2002) 283(5):H1750–60. doi: 10.1152/ajpheart.01051.2001
95. Cabral-Santos C, Silveira LS, Chimin P, Rosa-Neto JC, Lira FS. Moderate aerobic exercise-induced cytokines changes are disturbed in PPARalpha knockout mice. Cytokine. (2020) 134:155207. doi: 10.1016/j.cyto.2020.155207
96. Hinds TD Jr, Kipp ZA, Xu M, Yiannikouris FB, Morris AJ, Stec DF, et al. Adipose-specific PPARalpha knockout mice have increased lipogenesis by PASK-SREBP1 signaling and a polarity shift to inflammatory macrophages in white adipose tissue. Cells. (2021) 11(1):4. doi: 10.3390/cells11010004
97. Stec DE, Gordon DM, Hipp JA, Hong S, Mitchell ZL, Franco NR, et al. The loss of hepatic PPARalpha promotes inflammation and serum hyperlipidemia in diet-induced obesity. Am J Physiol Regul Integr Comp Physiol. (2019) 317(5):R733–R745. doi: 10.1152/ajpregu.00153.2019
98. Fry CS, Lee JD, Jackson JR, Kirby TJ, Stasko SA, Liu HL, et al. Regulation of the muscle fiber microenvironment by activated satellite cells during hypertrophy. FASEB J. (2014) 28(4):1654–65. doi: 10.1096/fj.13-239426
99. Goh Q, Millay DP. Requirement of myomaker-mediated stem cell fusion for skeletal muscle hypertrophy. Elife. (2017) 6:e20007. doi: 10.7554/eLife.20007
100. Goh Q, Song T, Petrany MJ, Cramer AA, Sun C, Sadayappan S, et al. Myonuclear accretion is a determinant of exercise-induced remodeling in skeletal muscle. Elife. (2019) 8:e44876. doi: 10.7554/eLife.44876
101. Ryall JG. Metabolic reprogramming as a novel regulator of skeletal muscle development and regeneration. FEBS J. (2013) 280(17):4004–13. doi: 10.1111/febs.12189
102. Kase ET, Andersen B, Nebb HI, Rustan AC, Thoresen GH. 22-Hydroxycholesterols regulate lipid metabolism differently than T0901317 in human myotubes. Biochim Biophys Acta. (2006) 1761(12):1515–22. doi: 10.1016/j.bbalip.2006.09.010
103. Muoio DM, Way JM, Tanner CJ, Winegar DA, Kliewer SA, Houmard JA, et al. Peroxisome proliferator-activated receptor-alpha regulates fatty acid utilization in primary human skeletal muscle cells. Diabetes. (2002) 51(4):901–9. doi: 10.2337/diabetes.51.4.901
104. Russell AP, Feilchenfeldt J, Schreiber S, Praz M, Crettenand A, Gobelet C, et al. Endurance training in humans leads to fiber type-specific increases in levels of peroxisome proliferator-activated receptor-gamma coactivator-1 and peroxisome proliferator-activated receptor-alpha in skeletal muscle. Diabetes. (2003) 52(12):2874–81. doi: 10.2337/diabetes.52.12.2874
105. Krämer DK, Ahlsén M, Norrbom J, Jansson E, Hjeltnes N, Gustafsson T, et al. Human skeletal muscle fibre type variations correlate with PPAR alpha, PPAR delta and PGC-1 alpha mRNA. Acta Physiol. (2006) 188(3-4):207–16. doi: 10.1111/j.1748-1716.2006.01620.x
106. Bakrania B, Du Toit EF, Ashton KJ, Wagner KH, Headrick JP, Bulmer AC. Chronically elevated bilirubin protects from cardiac reperfusion injury in the Male gunn rat. Acta Physiol. (2017) 220(4):461–70. doi: 10.1111/apha.12858
107. Bakrania B, Du Toit EF, Ashton KJ, Kiessling CJ, Wagner KH, Headrick JP, et al. Hyperbilirubinemia modulates myocardial function, aortic ejection, and ischemic stress resistance in the gunn rat. Am J Physiol Heart Circ Physiol. (2014) 307(8):H1142–9. doi: 10.1152/ajpheart.00001.2014
Keywords: exercise performance, heme oxygenase, biliverdin reductase, bilirubin, reactive oxygen species, oxidative stress, HO-1, BLVRA
Citation: Flack KD, Vítek L, Fry CS, Stec DE and Hinds TD (2023) Cutting edge concepts: Does bilirubin enhance exercise performance?. Front. Sports Act. Living 4:1040687. doi: 10.3389/fspor.2022.1040687
Received: 9 September 2022; Accepted: 19 December 2022;
Published: 11 January 2023.
Edited by:
Marco Beato, University of Suffolk, United KingdomReviewed by:
Ruoyu Yang, Shanghai University of Medicine and Health Sciences, ChinaMelinda M. Manore, Oregon State University, United States
© 2023 Flack, Vítek, Fry, Stec and Hinds. This is an open-access article distributed under the terms of the Creative Commons Attribution License (CC BY). The use, distribution or reproduction in other forums is permitted, provided the original author(s) and the copyright owner(s) are credited and that the original publication in this journal is cited, in accordance with accepted academic practice. No use, distribution or reproduction is permitted which does not comply with these terms.
*Correspondence: Kyle D. Flack a3lsZS5mbGFja0B1a3kuZWR1 Terry D. Hinds dGVycnkuaGluZHNAdWt5LmVkdQ==
Specialty Section: This article was submitted to Elite Sports and Performance Enhancement, a section of the journal Frontiers in Sports and Active Living