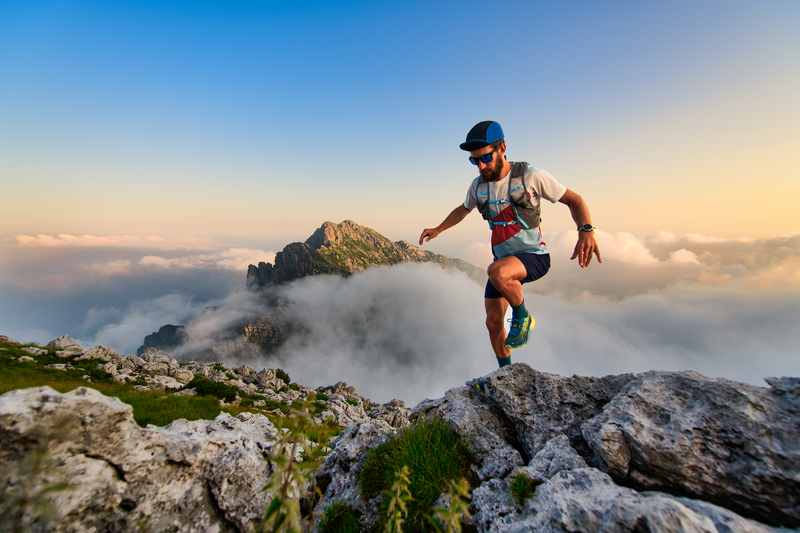
95% of researchers rate our articles as excellent or good
Learn more about the work of our research integrity team to safeguard the quality of each article we publish.
Find out more
MINI REVIEW article
Front. Sports Act. Living , 26 April 2021
Sec. Elite Sports and Performance Enhancement
Volume 3 - 2021 | https://doi.org/10.3389/fspor.2021.663674
This article is part of the Research Topic From Physiological Adaptations to Endurance Performance: It is Time to Bridge the Gap View all 9 articles
Exercise-induced hypoxemia (EIH) is well-described in endurance-trained athletes during both maximal and submaximal exercise intensities. Despite the drop in oxygen (O2) saturation and provided that training volumes are similar, athletes who experience EIH nevertheless produce the same endurance performance in normoxia as athletes without EIH. This lack of a difference prompted trainers to consider that the phenomenon was not relevant to performance but also suggested that a specific adaptation to exercise is present in EIH athletes. Even though the causes of EIH have been extensively studied, its consequences have not been fully characterized. With the development of endurance outdoor activities and altitude/hypoxia training, athletes often train and/or compete in this stressful environment with a decrease in the partial pressure of inspired O2 (due to the drop in barometric pressure). Thus, one can reasonably hypothesize that EIH athletes can specifically adapt to hypoxemic episodes during exercise at altitude. Although our knowledge of the interactions between EIH and acute exposure to hypoxia has improved over the last 10 years, many questions have yet to be addressed. Firstly, endurance performance during acute exposure to altitude appears to be more impaired in EIH vs. non-EIH athletes but the corresponding physiological mechanisms are not fully understood. Secondly, we lack information on the consequences of EIH during chronic exposure to altitude. Here, we (i) review research on the consequences of EIH under acute hypoxic conditions, (ii) highlight unresolved questions about EIH and chronic hypoxic exposure, and (iii) suggest perspectives for improving endurance training.
For many years, cardiovascular responses and muscle metabolism were the only acknowledged limiting factors in exercise performance. However, research has now clearly shown that the respiratory system is far from perfect and does not always have the capacity to meet the demands of intense endurance training (Amann, 2012). Dempsey et al.'s (2020) elegant review analyses the consequences of an “underpowered” respiratory system in endurance-trained athletes, where the excessive strain placed on to the respiratory system (relative to its capacity) leads to several problems, among them a phenomenon called exercise-induced hypoxemia (EIH).
EIH was first described in detail by Dempsey et al. in the 1980s. EIH occurs in some (but not all) endurance-trained athletes performing exercise at sea level (i.e. under normoxic conditions) (Dempsey et al., 1984; Dempsey and Wagner, 1999). The phenomenon involves a decrease in arterial oxygen pressure (PaO2) and a concomitant drop in arterial oxygen saturation (SaO2) between rest and maximal exercise. This hypoxemia is associated with abnormal gas exchange, as reflected by an increase in the alveolo-arterial oxygen pressure difference [D(A-a)O2] (Dempsey and Wagner, 1999; Prefaut et al., 2000). Since PaO2 and SaO2, together with the hemoglobin concentration, impact the arterial O2 content (CaO2), the oxygen (O2) supply to the muscles and thus aerobic exercise performance at sea level are impacted. In this context, EIH has attracted interest from sports scientists and physiologists.
Historically, EIH has been studied with invasive measurements of PaO2 and SaO2. The O2 dissociation curve for hemoglobin shows that the fall in PaO2 is linked to O2 desaturation, i.e., a fall in SaO2. Many studies have found that SaO2 ranges from 87 to 94% in EIH athletes (Powers et al., 1984, 1988; Sheel et al., 2001; Stewart et al., 2003). This involved that the fall of PaO2 is large enough to be located to the sloping part of the Barcroft's curve. Several physiological aspects must be considered in the strict definition of EIH. A fall in PaO2 of at least 10 mmHg between rest and maximal exercise is required, considering that (i) hypoxemia at rest is defined as a fall of 5 mmHg (Préfaut et al., 1988), and (ii) there is a 2–3 mmHg error in the PaO2 measurement during a maximal exercise test (due to the shift in Barcroft's curve with increasing temperature) (Holmgren and McIlroy, 1964). However, PaO2 is not the major determinant of CaO2 as opposed to SaO2, and due to the flat part of the Barcroft's curve some drop in PaO2 could not induced any fall in SaO2. So, SaO2 may be a greater marker to define EIH and the first studies to use SaO2 as a tool for measuring EIH considered SaO2 < 94% to be abnormal and so set thresholds with a certain margin of error (Powers et al., 1988; Harms and Stager, 1995).
After showing that VO2max decreases when SaO2 goes below 95%, Dempsey and Wagner (1999) suggested the following classification: mild EIH for SaO2 values between 95 and 93%, moderate EIH for 93–88%, and severe EIH for below 88%. To avoid invasive method, the strong relationship between SaO2 and oxygen pulse saturation values (SpO2) at rest and during exercise was considered. The use of pulse oximetry to study EIH was validated (Mollard et al., 2010). Considering inter-individual differences in the resting SpO2 values, taking into account (i) the inherent rightward shift of Barcroft's curve during exercise, and (ii) the accuracy of oximeters, Prefaut et al. (2000) defined EIH as a drop in SpO2 of at least 4% between rest and exercise. Since transient SpO2 drops are typically observed during exercise (Préfaut et al., 1994), the persistent nature of the desaturation must also be taken into account. Hence, the most suitable definition of EIH is a drop of at least 4% in SpO2 during one or more of the last three steps in an incremental exercise test to exhaustion (Durand et al., 2000; Prefaut et al., 2000). However, steps during a test to exhaustion vary in duration and workload charge, thus to avoid confusion between transient SpO2 drops and EIH a minimum of 3 min of desaturation could be recommend. The magnitude of the fall in SpO2 reported during EIH is the same as for SaO2 (Grataloup et al., 2005; Stewart and Pickering, 2007; Kyparos et al., 2012; Riganas et al., 2019).
Since the 1990s, several studies showed that athletes with EIH (EIH athletes) and without (NEIH athletes) with similar training volumes have the same VO2max (Legrand et al., 2005; Gaston et al., 2016; Raberin et al., 2019a; Durand et al., 2020). This prompted leading sports scientists to dismiss EIH as not being relevant. However, beyond a SaO2 decrement of 4% in men and 3% in women, each additional 1% decrement results in a 1% decrement in VO2max (Powers et al., 1993; Harms et al., 2000). EIH appears to be a multifactorial phenomenon, with the involvement of ventilation/perfusion mismatch, shunts (intrapulmonary or intracardiac), relative hypoventilation and diffusion limitation (Dempsey and Wagner, 1999). The contribution of each factor is still unclear, although it could partly depend on the intensity of the exercise (Durand et al., 2000). Anyway the purpose of this review is not to discuss around the pathophysiology of EIH but its consequences.
Few researchers have focused on the prevalence of EIH and an early study reported that 52% of male athletes with a VO2max > 68 ml.min−1.kg−1 displayed EIH during an incremental exercise test on a cycle ergometer (Powers et al., 1988). Some publication have reported a similar prevalence (Mucci et al., 2000; Connes et al., 2004a; Grataloup et al., 2005; Gaston et al., 2016), whereas others found higher values (60–75%) (Connes et al., 2004b; Guenette et al., 2004; Connes and Boucher, 2010) or lower values (30–35%) (Sheel et al., 2000; Alis et al., 2015). Recently, a prevalence of 70% was reported in a large cohort (n = 79) of male athletes with a VO2max > 68 ml.min−1.kg−1 performing a progressive-grade treadmill exercise test to exhaustion (Constantini et al., 2017). Due to a dysanapsis phenomenon with smaller relative lung size, women would be more prone to develop EIH than men (St Croix et al., 1998; Dominelli et al., 2013). Richards et al. (2004) reported that 67% of young active women (VO2max range: 28–61 ml.kg−1.min−1) present EIH during a cycle test to exhaustion.
EIH is also present at submaximal exercise intensities in some individuals (Dominelli and Sheel, 2018). In this context the “Demand vs. Capacity” theory is unable to explain the phenomenon. There is some evidence to show that hypoxemia during submaximal exercise is the result of relative alveolar hypoventilation while a non-ventilatory mechanism seemed to affect gas exchanges beyond the second ventilatory threshold and thereby enhancing EIH (Durand et al., 2000). The relative hypoventilation is probably at least a result of metabolic changes and different ventilatory responsiveness due to a high level of endurance training (Brooks and Mercier, 1994; Mucci et al., 1998; Granger et al., 2020).
Despite the presence of this large body of evidence, one group of researchers has claimed that EIH is a fallacy because most studies did not correct hypoxemia considering the rightward shift of the oxy-hemoglobin dissociation curve due to temperature, CO2 and pH (Scroop and Shipp, 2010). Furthermore, EIH is typically observed during exercises in a laboratory at sea level. On this basis, studies have suggested that EIH reduces performance and induces fatigue (Powers et al., 1988; Romer et al., 2006). However, laboratory conditions do not reflect ecological condition of training or competition, and so EIH and its consequences have not been studied during real training sessions. As a result, the athletes and coaches have likely underestimated the importance of EIH.
Some endurance athletes are required to compete at altitude. For example, trail running has become particularly popular in recent years and the craze is prompting more and more athletes to exercise at moderate altitude, often without acclimatization. In this context of acute exposure to moderate altitude, it has been reported that EIH athletes perform less well than NEIH athletes (Chapman et al., 1999; Gaston et al., 2016; Raberin et al., 2019a). Studies that monitored EIH during an acute exposure to hypoxia were reviewed in Table 1. These studies must have to include a control group in order to investigate the interaction between EIH and exposure to altitude during acute exposure. The studies were carried out under simulated altitudes of 1000–3000 m (Chapman et al., 1999; Grataloup et al., 2007; Raberin et al., 2019a), or under naturally hypoxic conditions at 2150 m (Gaston et al., 2016). All the studies found that the drop in VO2max during exercise at altitude was greater in EIH athletes. According to some researchers, this drop could be explained (at least in part) by the fact that the EIH athletes had a lower maximal heart rate (HRmax) at altitudes of 2150 m (Gaston et al., 2016), 2400 m (Raberin et al., 2019a), 3000 m (Grataloup et al., 2007), and 5000 m (Benoit et al., 2003). In contrast, Chapman et al. (1999) did not find a difference in HRmax between EIH and NEIH athletes at an altitude of 1000 m. Some researchers have suggested that the reduction in VO2max in EIH athletes at altitude is associated with the fall in SaO2 observed at sea level (Gavin et al., 1998; Chapman et al., 1999, 2011). Indeed, SpO2 during exercise at altitude is lower in EIH athletes than in NEIH athletes in some studies (Grataloup et al., 2007; Raberin et al., 2019a). A decrease in the ventilatory response to hypoxia can likely explain the lower SaO2 measured in EIH athletes. EIH athletes might fail to have an adequate ventilation during exercise at altitude, which might contribute to hypoxemia (Harms and Stager, 1995; Derchak et al., 2000). However, some studies did not find a relationship between the ventilatory response to hypoxia and SaO2 (Hopkins and McKenzie, 1989; Guenette et al., 2004). Recent studies of the ventilatory response to hypoxia and hypercapnia in EIH athletes have generated interesting results (Granger et al., 2020). Indeed, athletes with the lowest SpO2 during exercise showed a lower ventilatory response to hypercapnia (but not to hypoxia) than athletes with a higher SpO2. This blunted hypercapnic ventilatory response might have consequences on ventilatory acclimatization to altitude. Nevertheless, other studies did not find greater hypoxemia values in EIH athletes (vs. NEIH athletes) during hypoxic exercise (Benoit et al., 2003; Verges et al., 2005; Gaston et al., 2016). In the study by Gaston et al. (2016), the larger decrease in VO2max in EIH athletes (22 vs. 16% in NEIH athletes) could not be wholly explained by a greater reduction in HRmax and so suggested the involvement of another factor and probably specific adaptation to hypoxia in EIH athletes. More recently, it was reported that EIH athletes displayed exacerbated changes in cerebral deoxygenation and a low limb muscle blood volume at sea level; these results were accentuated by the severity of O2 arterial desaturation, and in contrast to NEIH athletes, were the same in hypoxia and normoxia (Raberin et al., 2019a).
In another way, since the Mexico Olympics, scientists have been particularly interested in altitude training. The traditional “live high, train high” paradigm, i.e., a training camp situated at moderate altitude (1800–2500 m) is usually implemented two or three times a year (Millet et al., 2010). Historically, it was expected that the altitude-induced hematological response would increase O2 transport and thus aerobic performance back at sea level. However, the nature of other responses requires further investigation. The “live high, train low” (LHTL) altitude training mode was developed in which athletes live at moderate altitude but train at sea level or at low altitude (Levine and Stray-Gundersen, 1997). So, LHTL enables athletes to benefit from both the effects of chronic exposure to altitude and from maintaining a level of training intensity close to that used typically at sea level (Levine and Stray-Gundersen, 1997). In this initial study, Levine and Stray-Gundersen (1997) described significant hematological adaptations (notably elevated erythropoietin, hemoglobin, and hematocrit) associated with a gain in performance. Several follow-up studies gave essentially similar results (Hahn et al., 2001; Stray-Gundersen et al., 2001; Dehnert et al., 2002; Chapman et al., 2014) although other researchers observed no (or only small) hematological changes after LHTL (Ashenden et al., 1999, 2000). It is well-known that endurance performance (i.e., VO2max) does not depend solely on hematological parameters. Indeed, peripheral factors like exercise economy and/or buffering capacity (Gore et al., 2001, 2007; Saunders et al., 2004) might also contribute to post-LHTL performance. The initial LHTL model has evolved, with the development of new devices for artificially creating altitude conditions (i.e., normobaric hypoxia). At present, athletes no longer need to go up into the mountains to find a hypoxic environment. Regardless of the simulation method, not all endurance athletes respond in the same way to altitude training, and considerable inter-individual variations in responses have been documented (Chapman, 2013; Nummela et al., 2021). In this context, several methodological, training-related, and physiological factors have been identified but the putative impact of EIH on individual responses has never been investigated. Only a study evaluated the impact of intermittent hypoxia for 10 days (90 min.day−1, with FiO2 giving a SaO2 of 80%) on EIH athletes while others followed the same protocol under normoxic conditions (Marshall et al., 2008). The researchers found that exposure to intermittent hypoxia reduced the severity of EIH (94% after exposure, compared with 91% before). The increase in SaO2 might have been mediated by increased chemosensitivity at altitude, which in turn might increase hyperventilation (Katayama et al., 2001; Ainslie et al., 2003). Indeed, EIH athletes are thought to be poorly chemosensitive (Harms and Stager, 1995; Constantini et al., 2017; Granger et al., 2020). However, no improvements in VO2max or performance were reported. Thus, the interactions between EIH and altitude during the acclimatization phase remain to be explored.
There are few studies on this topic. It was recently reported that EIH athletes presented lower resting and maximal SpO2 values after 5 days of exposure to 2400 m (Durand et al., 2019). During this prolonged hypoxia, EIH athletes maintained a greater maximal cardiac output (vs. NEIH athletes), suggesting specific cardiovascular adaptations that enabled the achievement of the same VO2max. During the same protocol, EIH athletes exhibit an exacerbated oxidative stress at sea level compared to NEIH athletes while there was no between group difference after 1 day of exposure (Raberin et al., 2021). This result suggests that the impact of EIH on oxidative stress was blunted by acute altitude. However after 5 days of exposure, the reappearance of the between group difference suggest that EIH athletes may elicit a greater ventilatory acclimatization due to higher oxidative stress, since oxidative stress has been shown to modulate the hypoxic ventilatory response (Pialoux et al., 2009). However, the study failed to conclude on a role of oxidative stress at the onset of EIH due to its impact on pulmonary endothelial permeability (Nielsen, 2003).
One could also suspect that the mechanisms at the origin of EIH at sea level could be exacerbated during prolonged altitude exposure. The low chemosensitivity of EIH athletes could impact the kinetic of ventilatory acclimatation and be at the onset of a greater relative hypoventilation during exercise in hypoxia. An exacerbation of the ventilation/perfusion mismatch and extravascular pulmonary fluid movements may also occur during prolonged exposure. Indeed, pulmonary vessels constrict in response to alveolar hypoxia, this phenomenon known as hypoxic pulmonary vasoconstriction (HPV) aims to divert blood to better-oxygenated lung segments, thereby optimizing ventilation/perfusion matching and systemic O2 delivery (Swenson, 2013). HPV is known to persist during prolonged exposure to hypoxia and to increase pulmonary arterial pressure and pulmonary arterial resistance (Raberin et al., 2019b). These hemodynamic changes might be triggered intrapulmonary arteriovenous anastomosis (IPAVAS) recruitment leading to an arterial O2 desaturation due to shunt. Some evidences show that hypoxia could have a role in the opening of IPAVAS but whether O2 tension regulates IPAVAS remains unclear (Laurie et al., 2010; Lovering et al., 2015). Further studies are needed to understand how EIH changes during chronic exposure to altitude. Finally, the Figure 1 provides a summary of the physiological determinants of EIH in relation to the modalities of exposure to hypoxia.
Figure 1. Putative impact of hypoxia (black rectangle) on physiological determinants thought to be involved in the onset of EIH at sea level (gray rectangle). Dotted line refers to the “stress failure theory.”.
The roles of EIH following diffusion limitation (Chapman et al., 1999), excessive work of breathing (Amann et al., 2007), and increased pulmonary vascular resistance and arterial pressure (Eldridge et al., 2006; Faoro et al., 2009) are clear and raise the question of whether EIH has an additional role in the context of hypoxic training.
However, it seems essential to better understand EIH in ecological condition since athletes rarely reach maximal aerobic capacities during training. In addition, the course of EIH during altitude/hypoxic training and its consequences on training goals should be investigated. We can suggest different avenues to develop this knowledge: (i) monitor SpO2 during ecological training session to understand the frequency, duration, and severity of the hypoxemic episode and which kind of training might induce significant hypoxemia, (ii) evaluate whether EIH could impair or facilitate acclimatization to altitude and thereafter, (iii) investigate the putative role of EIH in the great variability of the response to altitude/hypoxia training whatever the method. In order to collect and analyze the large amounts of data and their interaction, openness to the science complex system is essential. In recent years, mathematics has been increasingly used in physiology (Lloyd et al., 2016; Pereira et al., 2018). The integration of sports science analysis with complex networks is called “complex sports analytics” and makes it possible to approach complex network structures as a very promising model prediction tool.
Individualization of the altitude dose is well-known to be the key of altitude/hypoxic training to ultimately reduce inter-individual variability. In the context, monitor the EIH during training has to be considered as a main factor of this individualization to find the best practice to increase performance.
The original conception of the work was conducted and the manuscript was drafted by FD and AR. Both authors read and approved the final manuscript.
The authors declare that the research was conducted in the absence of any commercial or financial relationships that could be construed as a potential conflict of interest.
Ainslie, P. N., Kolb, J. C., Ide, K., and Poulin, M. J. (2003). Effects of five nights of normobaric hypoxia on the ventilatory responses to acute hypoxia and hypercapnia. Respir. Physiol. Neurobiol. 138, 193–204. doi: 10.1016/S1569-9048(03)00190-3
Alis, R., Sanchis-Gomar, F., Ferioli, D., La Torre, A., Blesa, J. R., and Romagnoli, M. (2015). Exercise effects on erythrocyte deformability in exercise-induced arterial hypoxemia. Int. J. Sports Med. 36, 286–291. doi: 10.1055/s-0034-1394395
Amann, M. (2012). Pulmonary system limitations to endurance exercise performance in humans. Exp. Physiol. 97, 311–318. doi: 10.1113/expphysiol.2011.058800
Amann, M., Pegelow, D. F., Jacques, A. J., and Dempsey, J. A. (2007). Inspiratory muscle work in acute hypoxia influences locomotor muscle fatigue and exercise performance of healthy humans. Am. J. Physiol. Regul. Integr. Comp. Physiol. 293, R2036–R2045. doi: 10.1152/ajpregu.00442.2007
Ashenden, M. J., Gore, C. J., Dobson, G. P., Boston, T. T., Parisotto, R., Emslie, K. R., et al. (2000). Simulated moderate altitude elevates serum erythropoietin but does not increase reticulocyte production in well-trained runners. Eur. J. Appl. Physiol. 81, 428–435. doi: 10.1007/s004210050064
Ashenden, M. J., Gore, C. J., Martin, D. T., Dobson, G. P., and Hahn, A. G. (1999). Effects of a 12-day “live high, train low” camp on reticulocyte production and haemoglobin mass in elite female road cyclists. Eur. J. Appl. Physiol. Occup. Physiol. 80, 472–478. doi: 10.1007/s004210050620
Benoit, H., Busso, T., Castells, J., Geyssant, A., and Denis, C. (2003). Decrease in peak heart rate with acute hypoxia in relation to sea level VO(2max). Eur. J. Appl. Physiol. 90, 514–519. doi: 10.1007/s00421-003-0899-y
Brooks, G. A., and Mercier, J. (1994). Balance of carbohydrate and lipid utilization during exercise: the “crossover” concept. J. Appl. Physiol. (1985) 76, 2253–2261. doi: 10.1152/jappl.1994.76.6.2253
Chapman, R. F. (2013). The individual response to training and competition at altitude. Br. J. Sports Med. 47, i40–i44. doi: 10.1136/bjsports-2013-092837
Chapman, R. F., Emery, M., and Stager, J. M. (1999). Degree of arterial desaturation in normoxia influences VO2max decline in mild hypoxia. Med. Sci. Sports Exerc. 31, 658–663. doi: 10.1097/00005768-199905000-00006
Chapman, R. F., Laymon Stickford, A. S., Lundby, C., and Levine, B. D. (2014). Timing of return from altitude training for optimal sea level performance. J. Appl. Physiol. 116, 837–843. doi: 10.1152/japplphysiol.00663.2013
Chapman, R. F., Stager, J. M., Tanner, D. A., Stray-Gundersen, J., and Levine, B. D. (2011). Impairment of 3000-m run time at altitude is influenced by arterial oxyhemoglobin saturation. Med. Sci. Sports Exerc. 43, 1649–1656. doi: 10.1249/MSS.0b013e318211bf45
Connes, P., and Boucher, J. H. (2010). Echinocytosis in athletes with exercise-induced hypoxemia. Clin. Hemorheol. Microcirc. 44, 107–114. doi: 10.3233/CH-2010-1258
Connes, P., Bouix, D., Durand, F., Kippelen, P., Mercier, J., Prefaut, C., et al. (2004a). Is hemoglobin desaturation related to blood viscosity in athletes during exercise? Int. J. Sports Med. 25, 569–574. doi: 10.1055/s-2004-821118
Connes, P., Bouix, D., Py, G., Caillaud, C., Kippelen, P., Brun, J.-F., et al. (2004b). Does exercise-induced hypoxemia modify lactate influx into erythrocytes and hemorheological parameters in athletes? J. Appl. Physiol. 97, 1053–1058. doi: 10.1152/japplphysiol.00993.2003
Constantini, K., Tanner, D. A., Gavin, T. P., Harms, C. A., Stager, J. M., and Chapman, R. F. (2017). Prevalence of exercise-induced arterial hypoxemia in distance runners at sea level. Med. Sci. Sports Exerc. 49, 948–954. doi: 10.1249/MSS.0000000000001193
Dehnert, C., Hütler, M., Liu, Y., Menold, E., Netzer, C., Schick, R., et al. (2002). Erythropoiesis and performance after two weeks of living high and training low in well trained triathletes. Int. J. Sports Med. 23, 561–566. doi: 10.1055/s-2002-35533
Dempsey, J. A., Hanson, P. G., and Henderson, K. S. (1984). Exercise-induced arterial hypoxaemia in healthy human subjects at sea level. J. Physiol. 355, 161–175. doi: 10.1113/jphysiol.1984.sp015412
Dempsey, J. A., La Gerche, A., and Hull, J. H. (2020). Is the healthy respiratory system built just right, overbuilt, or underbuilt to meet the demands imposed by exercise? J. Appl. Physiol. (1985) 129, 1235–1256. doi: 10.1152/japplphysiol.00444.2020
Dempsey, J. A., and Wagner, P. D. (1999). Exercise-induced arterial hypoxemia. J. Appl. Physiol. 87, 1997–2006. doi: 10.1152/jappl.1999.87.6.1997
Derchak, P. A., Stager, J. M., Tanner, D. A., and Chapman, R. F. (2000). Expiratory flow limitation confounds ventilatory response during exercise in athletes. Med. Sci. Sports Exerc. 32, 1873–1879. doi: 10.1097/00005768-200011000-00009
Dominelli, P. B., Foster, G. E., Dominelli, G. S., Henderson, W. R., Koehle, M. S., McKenzie, D. C., et al. (2013). Exercise-induced arterial hypoxaemia and the mechanics of breathing in healthy young women. J. Physiol. (Lond.) 591, 3017–3034. doi: 10.1113/jphysiol.2013.252767
Dominelli, P. B., and Sheel, A. W. (2018). Exercise-induced arterial hypoxemia; some answers, more questions. Appl. Physiol. Nutr. Metab. 44, 571–579 doi: 10.1139/apnm-2018-0468
Durand, F., Gaston, A.-F., Vicenzi, M., Deboeck, G., Subirats, E., and Faoro, V. (2020). Noninvasive pulmonary hemodynamic evaluation in athletes with exercise-induced hypoxemia. Chest 157, 1568–1578. doi: 10.1016/j.chest.2020.01.037
Durand, F., Mucci, P., and Préfaut, C. (2000). Evidence for an inadequate hyperventilation inducing arterial hypoxemia at submaximal exercise in all highly trained endurance athletes. Med. Sci. Sports Exerc. 32, 926–932. doi: 10.1097/00005768-200005000-00008
Durand, F., Raberin, A., Meric, H., Mucci, P., and Lopez Ayerbe, J. (2019). “Exercise-induced hypoxemia leads to specific adaptations in cardiovascular system during acclimatization to moderate hypoxia,” in Book of abstracts−24th Annual Congress of the European College of Sport Science (Prague: European College of Sport Science), 237. Available online at: https://www.dropbox.com/s/7z3xr5rblm5l069/Prague_BOA_Web.pdf?dl=0 (accessed January 29, 2021).
Eldridge, M. W., Braun, R. K., Yoneda, K. Y., and Walby, W. F. (2006). Effects of altitude and exercise on pulmonary capillary integrity: evidence for subclinical high-altitude pulmonary edema. J. Appl. Physiol. 100, 972–980. doi: 10.1152/japplphysiol.01048.2005
Faoro, V., Boldingh, S., Moreels, M., Martinez, S., Lamotte, M., Unger, P., et al. (2009). Bosentan decreases pulmonary vascular resistance and improves exercise capacity in acute hypoxia. Chest 135, 1215–1222. doi: 10.1378/chest.08-2222
Gaston, A.-F., Durand, F., Roca, E., Doucende, G., Hapkova, I., and Subirats, E. (2016). Exercise-induced hypoxaemia developed at sea-level influences responses to exercise at moderate altitude. PLoS ONE 11:e0161819. doi: 10.1371/journal.pone.0161819
Gavin, T. P., Derchak, P. A., and Stager, J. M. (1998). Ventilation's role in the decline in VO2max and SaO2 in acute hypoxic exercise. Med. Sci. Sports Exerc. 30, 195–199. doi: 10.1097/00005768-199802000-00004
Gore, C. J., Clark, S. A., and Saunders, P. U. (2007). Nonhematological mechanisms of improved sea-level performance after hypoxic exposure. Med. Sci. Sports Exerc. 39, 1600–1609. doi: 10.1249/mss.0b013e3180de49d3
Gore, C. J., Hahn, A. G., Aughey, R. J., Martin, D. T., Ashenden, M. J., Clark, S. A., et al. (2001). Live high:train low increases muscle buffer capacity and submaximal cycling efficiency. Acta Physiol. Scand. 173, 275–286. doi: 10.1046/j.1365-201X.2001.00906.x
Granger, E. A., Cooper, T. K., Hopkins, S. R., McKenzie, D. C., and Dominelli, P. (2020). Peripheral chemoresponsiveness during exercise in male athletes with exercise-induced arterial hypoxaemia. Exp. Physiol. 105, 1960–1970. doi: 10.1113/EP088639
Grataloup, O., Busso, T., Castells, J., Denis, C., and Benoit, H. (2007). Evidence of decrease in peak heart rate in acute hypoxia: effect of exercise-induced arterial hypoxemia. Int. J. Sports Med. 28, 181–185. doi: 10.1055/s-2006-924216
Grataloup, O., Prieur, F., Busso, T., Castells, J., Favier, F. B., Denis, C., et al. (2005). Effect of hyperoxia on maximal O2 uptake in exercise-induced arterial hypoxaemic subjects. Eur. J. Appl. Physiol. 94, 641–645. doi: 10.1007/s00421-005-1361-0
Guenette, J. A., Diep, T. T., Koehle, M. S., Foster, G. E., Richards, J. C., and Sheel, A. W. (2004). Acute hypoxic ventilatory response and exercise-induced arterial hypoxemia in men and women. Respir. Physiol. Neurobiol. 143, 37–48. doi: 10.1016/j.resp.2004.07.004
Hahn, A. G., Gore, C. J., Martin, D. T., Ashenden, M. J., Roberts, A. D., and Logan, P. A. (2001). An evaluation of the concept of living at moderate altitude and training at sea level. Comp. Biochem. Physiol. A Mol. Integr. Physiol. 128, 777–789. doi: 10.1016/S1095-6433(01)00283-5
Harms, C. A., McClaran, S. R., Nickele, G. A., Pegelow, D. F., Nelson, W. B., and Dempsey, J. A. (2000). Effect of exercise-induced arterial O2 desaturation on VO2max in women. Med. Sci. Sports Exerc. 32, 1101–1108. doi: 10.1097/00005768-200006000-00010
Harms, C. A., and Stager, J. M. (1995). Low chemoresponsiveness and inadequate hyperventilation contribute to exercise-induced hypoxemia. J. Appl. Physiol. 79, 575–580. doi: 10.1152/jappl.1995.79.2.575
Holmgren, A., and McIlroy, M. B. (1964). Effect of temperature on arterial blood gas tensions and pH during exercise. J. Appl. Physiol. 19, 243–245. doi: 10.1152/jappl.1964.19.2.243
Hopkins, S. R., and McKenzie, D. C. (1989). Hypoxic ventilatory response and arterial desaturation during heavy work. J. Appl. Physiol. 67, 1119–1124. doi: 10.1152/jappl.1989.67.3.1119
Katayama, K., Sato, Y., Morotome, Y., Shima, N., Ishida, K., Mori, S., et al. (2001). Intermittent hypoxia increases ventilation and Sa(O2) during hypoxic exercise and hypoxic chemosensitivity. J. Appl. Physiol. 90, 1431–1440. doi: 10.1152/jappl.2001.90.4.1431
Kyparos, A., Riganas, C., Nikolaidis, M. G., Sampanis, M., Koskolou, M. D., Grivas, G. V., et al. (2012). The effect of exercise-induced hypoxemia on blood redox status in well-trained rowers. Eur. J. Appl. Physiol. 112, 2073–2083. doi: 10.1007/s00421-011-2175-x
Laurie, S. S., Yang, X., Elliott, J. E., Beasley, K. M., and Lovering, A. T. (2010). Hypoxia-induced intrapulmonary arteriovenous shunting at rest in healthy humans. J. Appl. Physiol. 109, 1072–1079. doi: 10.1152/japplphysiol.00150.2010
Legrand, R., Ahmaidi, S., Moalla, W., Chocquet, D., Marles, A., Prieur, F., et al. (2005). O2 arterial desaturation in endurance athletes increases muscle deoxygenation. Med. Sci. Sports Exerc. 37, 782–788. doi: 10.1249/01.MSS.0000161806.47058.40
Levine, B. D., and Stray-Gundersen, J. (1997). “Living high-training low”: effect of moderate-altitude acclimatization with low-altitude training on performance. J. Appl. Physiol. 83, 102–112. doi: 10.1152/jappl.1997.83.1.102
Lloyd, A., Raccuglia, M., Hodder, S., and Havenith, G. (2016). Interaction between environmental temperature and hypoxia on central and peripheral fatigue during high-intensity dynamic knee extension. J. Appl. Physiol. (1985) 120, 567–579. doi: 10.1152/japplphysiol.00876.2015
Lovering, A. T., Duke, J. W., and Elliott, J. E. (2015). Intrapulmonary arteriovenous anastomoses in humans—response to exercise and the environment. J. Physiol. 593:507. doi: 10.1113/jphysiol.2014.275495
Marshall, H. C., Hamlin, M. J., Hellemans, J., Murrell, C., Beattie, N., Hellemans, I., et al. (2008). Effects of intermittent hypoxia on SaO(2), cerebral, and muscle oxygenation during maximal exercise in athletes with exercise-induced hypoxemia. Eur. J. Appl. Physiol. 104, 383–393. doi: 10.1007/s00421-007-0616-3
Millet, G. P., Roels, B., Schmitt, L., Woorons, X., and Richalet, J. P. (2010). Combining hypoxic methods for peak performance. Sports Med. 40, 1–25. doi: 10.2165/11317920-000000000-00000
Mollard, P., Bourdillon, N., Letournel, M., Herman, H., Gibert, S., Pichon, A., et al. (2010). Validity of arterialized earlobe blood gases at rest and exercise in normoxia and hypoxia. Respir. Physiol. Neurobiol. 172, 179–183. doi: 10.1016/j.resp.2010.05.017
Mucci, P., Durand, F., Lebel, B., Bousquet, J., and Préfaut, C. (2000). Interleukins 1-beta,−8, and histamine increases in highly trained, exercising athletes. Med. Sci. Sports Exerc. 32, 1094–1100. doi: 10.1097/00005768-200006000-00009
Mucci, P., Prioux, J., Hayot, M., Ramonatxo, M., and Préfaut, C. (1998). Ventilation response to CO2 and exercise-induced hypoxaemia in master athletes. Eur. J. Appl. Physiol. Occup. Physiol. 77, 343–351. doi: 10.1007/s004210050343
Nielsen, H. B. (2003). Arterial desaturation during exercise in man: implication for O2 uptake and work capacity. Scand. J. Med. Sci. Sports 13, 339–358. doi: 10.1046/j.1600-0838.2003.00325.x
Nummela, A., Eronen, T., Koponen, A., Tikkanen, H., and Peltonen, J. E. (2021). Variability in hemoglobin mass response to altitude training camps. Scand. J. Med. Sci. Sports 31, 44–51. doi: 10.1111/sms.13804
Pereira, V. H., Gobatto, C. A., Lewis, T. G., Ribeiro, L. F. P., Beck, W. R., Dos Reis, I. G. M., et al. (2018). Computational and complex network modeling for analysis of sprinter athletes' performance in track field tests. Front. Physiol. 9:843. doi: 10.3389/fphys.2018.00843
Pialoux, V., Brugniaux, J. V., Fellmann, N., Richalet, J.-P., Robach, P., Schmitt, L., et al. (2009). Oxidative stress and HIF-1 alpha modulate hypoxic ventilatory responses after hypoxic training on athletes. Respir. Physiol. Neurobiol. 167, 217–220. doi: 10.1016/j.resp.2009.04.012
Powers, S. K., Dodd, S., Lawler, J., Landry, G., Kirtley, M., McKnight, T., et al. (1988). Incidence of exercise induced hypoxemia in elite endurance athletes at sea level. Eur. J. Appl. Physiol. Occup. Physiol. 58, 298–302. doi: 10.1007/BF00417266
Powers, S. K., Dodd, S., Woodyard, J., Beadle, R. E., and Church, G. (1984). Haemoglobin saturation during incremental arm and leg exercise. Br. J. Sports Med. 18, 212–216. doi: 10.1136/bjsm.18.3.212
Powers, S. K., Martin, D., and Dodd, S. (1993). Exercise-induced hypoxaemia in elite endurance athletes. Incidence, causes and impact on VO2max. Sports Med. 16, 14–22. doi: 10.2165/00007256-199316010-00003
Préfaut, C., Anselme, F., Caillaud, C., and Massé-Biron, J. (1994). Exercise-induced hypoxemia in older athletes. J. Appl. Physiol. 76, 120–126. doi: 10.1152/jappl.1994.76.1.120
Préfaut, C., Bourgouin-Karaouni, D., Ramonatxo, M., Michel, F. B., and Macabies, J. (1988). A one year double blind follow-up of blood gas tensions and haemodynamics in almitrine bismesylate therapy. Eur. Respir. J. 1, 41–50.
Prefaut, C., Durand, F., Mucci, P., and Caillaud, C. (2000). Exercise-induced arterial hypoxaemia in athletes: a review. Sports Med. 30, 47–61. doi: 10.2165/00007256-200030010-00005
Raberin, A., Meric, H., Mucci, P., Lopez Ayerbe, J., and Durand, F. (2019a). Muscle and cerebral oxygenation during exercise in athletes with exercise-induced hypoxemia: a comparison between sea level and acute moderate hypoxia. Eur. J. Sport Sci. 20, 803–812. doi: 10.1080/17461391.2019.1669717
Raberin, A., Nader, E., Ayerbe, J. L., Mucci, P., Connes, P., and Durand, F. (2019b). Evolution of blood rheology and its relationship to pulmonary hemodynamic during the first days of exposure to moderate altitude. Clin. Hemorheol. Microcirc. 74, 201–208. doi: 10.3233/CH-190671
Raberin, A., Nader, E., Lopez Ayerbe, J., Alfonsi, G., Mucci, P., Rytz, C. L., et al. (2021). Pro-Oxidant/Antioxidant balance during a prolonged exposure to moderate altitude in athletes exhibiting exercise-induced hypoxemia at sea-level. Life 11:228. doi: 10.3390/life11030228
Richards, J. C., McKenzie, D. C., Warburton, D. E. R., Road, J. D., and Sheel, A. W. (2004). Prevalence of exercise-induced arterial hypoxemia in healthy women. Med. Sci. Sports Exerc. 36, 1514–1521. doi: 10.1249/01.MSS.0000139898.30804.60
Riganas, C., Papadopoulou, Z., Margaritelis, N. V., Christoulas, K., and Vrabas, I. S. (2019). Inspiratory muscle training effects on oxygen saturation and performance in hypoxemic rowers: effect of sex. J. Sports Sci. 37, 2513–2521. doi: 10.1080/02640414.2019.1646582
Romer, L. M., Haverkamp, H. C., Lovering, A. T., Pegelow, D. F., and Dempsey, J. A. (2006). Effect of exercise-induced arterial hypoxemia on quadriceps muscle fatigue in healthy humans. Am. J. Physiol. Regul. Integr. Comp. Physiol. 290, R365–R375. doi: 10.1152/ajpregu.00332.2005
Saunders, P. U., Telford, R. D., Pyne, D. B., Cunningham, R. B., Gore, C. J., Hahn, A. G., et al. (2004). Improved running economy in elite runners after 20 days of simulated moderate-altitude exposure. J. Appl. Physiol. (1985) 96, 931–937. doi: 10.1152/japplphysiol.00725.2003
Scroop, G. C., and Shipp, N. J. (2010). Exercise-induced hypoxemia: fact or fallacy? Med. Sci. Sports Exerc. 42, 120–126. doi: 10.1249/MSS.0b013e3181ad0117
Sheel, A. W., Edwards, M. R., Hunte, G. S., and McKenzie, D. C. (2001). Influence of inhaled nitric oxide on gas exchange during normoxic and hypoxic exercise in highly trained cyclists. J. Appl. Physiol. 90, 926–932. doi: 10.1152/jappl.2001.90.3.926
Sheel, A. W., Edwards, M. R., and McKenzie, D. C. (2000). Relationship between decreased oxyhaemoglobin saturation and exhaled nitric oxide during exercise. Acta Physiol. Scand. 169, 149–156. doi: 10.1046/j.1365-201x.2000.00729.x
St Croix, C. M., Harms, C. A., McClaran, S. R., Nickele, G. A., Pegelow, D. F., Nelson, W. B., et al. (1998). Effects of prior exercise on exercise-induced arterial hypoxemia in young women. J. Appl. Physiol. 85, 1556–1563. doi: 10.1152/jappl.1998.85.4.1556
Stewart, I. B., Labreche, J. M., and McKenzie, D. C. (2003). Effect of a long- and short-acting beta2-agonist on exercise-induced arterial hypoxemia. Med. Sci. Sports Exerc. 35, 603–607. doi: 10.1249/01.MSS.0000058439.11005.38
Stewart, I. B., and Pickering, R. L. (2007). Effect of prolonged exercise on arterial oxygen saturation in athletes susceptible to exercise-induced hypoxemia. Scand. J. Med. Sci. Sports 17, 445–451. doi: 10.1111/j.1600-0838.2006.00563.x
Stray-Gundersen, J., Chapman, R. F., and Levine, B. D. (2001). “Living high-training low” altitude training improves sea level performance in male and female elite runners. J. Appl. Physiol. (1985) 91, 1113–1120. doi: 10.1152/jappl.2001.91.3.1113
Swenson, E. R. (2013). Hypoxic pulmonary vasoconstriction. High Alt. Med. Biol. 14, 101–110. doi: 10.1089/ham.2013.1010
Keywords: endurance, performance, exercise induced hypoxemia, altitude, O2 desaturation, altitude/hypoxia training, endurance performance
Citation: Durand F and Raberin A (2021) Exercise-Induced Hypoxemia in Endurance Athletes: Consequences for Altitude Exposure. Front. Sports Act. Living 3:663674. doi: 10.3389/fspor.2021.663674
Received: 03 February 2021; Accepted: 25 March 2021;
Published: 26 April 2021.
Edited by:
Franck Brocherie, Institut national du sport, de l'expertise et de la performance (INSEP), FranceReviewed by:
Martin Burtscher, University of Innsbruck, AustriaCopyright © 2021 Durand and Raberin. This is an open-access article distributed under the terms of the Creative Commons Attribution License (CC BY). The use, distribution or reproduction in other forums is permitted, provided the original author(s) and the copyright owner(s) are credited and that the original publication in this journal is cited, in accordance with accepted academic practice. No use, distribution or reproduction is permitted which does not comply with these terms.
*Correspondence: Fabienne Durand, ZmR1cmFuZEB1bml2LXBlcnAuZnI=
Disclaimer: All claims expressed in this article are solely those of the authors and do not necessarily represent those of their affiliated organizations, or those of the publisher, the editors and the reviewers. Any product that may be evaluated in this article or claim that may be made by its manufacturer is not guaranteed or endorsed by the publisher.
Research integrity at Frontiers
Learn more about the work of our research integrity team to safeguard the quality of each article we publish.