- 1Department of Health and Kinesiology, University of Utah, Salt Lake City, UT, United States
- 2Department of Physical Therapy and Athletic Training, University of Utah, Salt Lake City, UT, United States
- 3Division of Epidemiology, Department of Internal Medicine, University of Utah, Salt Lake City, UT, United States
- 4Division of Physical Medicine and Rehabilitation, University of Utah School of Medicine, Salt Lake City, UT, United States
- 5Department of Orthopaedic Surgery Operations, University of Utah School of Medicine, Salt Lake City, UT, United States
Background: Deficits in neuromuscular control are widely reported after mild traumatic brain injury (mTBI). These deficits are speculated to contribute to the increased rate of musculoskeletal injuries after mTBI. However, a concrete mechanistic connection between post-mTBI deficits and musculoskeletal injuries has yet to be established. While impairments in some domains of balance control have been linked to musculoskeletal injuries, reactive balance control has received little attention in the mTBI literature, despite the inherent demand of balance recovery in athletics. Our central hypothesis is that the high rate of musculoskeletal injuries after mTBI is in part due to impaired reactive balance control necessary for balance recovery. The purpose of this study is to (1) characterize reactive postural responses to recover balance in athletes with recent mTBI compared to healthy control subjects, (2) determine the extent to which reactive postural responses remain impaired in athletes with recent mTBI who have been cleared to return to play, and (3) determine the relationship between reactive postural responses and acute lower extremity musculoskeletal injuries in a general sample of healthy collegiate athletes.
Methods: This two-phase study will take place at the University of Utah in coordination with the University of Utah Athletics Department. Phase 1 will evaluate student-athletes who have sustained mTBI and teammate-matched controls who meet all the inclusion criteria. The participants will be assessed at multiple time points along the return-to-play progress of the athlete with mTBI. The primary outcome will be measures of reactive postural response derived from wearable sensors during the Push and Release (P&R) test. In phase 2, student-athletes will undergo a baseline assessment of postural responses. Acute lower extremity musculoskeletal injuries for each participant will be prospectively tracked for 1 year from the date of first team activity. The primary outcomes will be the measures of reactive postural responses and the time from first team activity to lower extremity injury.
Discussion: Results from this study will further our understanding of changes in balance control, across all domains, after mTBI and identify the extent to which postural responses can be used to assess injury risk in collegiate athletes.
Introduction
Standing balance problems are well documented in collegiate athletes after mild traumatic brain injury (mTBI) (Guskiewicz, 2011; Broglio et al., 2014). However, the Balance Error Scoring System (BESS), the most common clinical balance assessment for a suspected mTBI, only subjectively measures postural control in a variety of static conditions (Riemann and Guskiewicz, 2000). The BESS and other clinical tests, such as the standard Romberg test (Guskiewicz, 2011), struggle to detect subtle balance deficits that persist throughout an athlete's recovery from mTBI (Buckley et al., 2018) and therefore may be limited in their ability to determine return-to-play (RTP) readiness. Additionally, postural demands during athletic pursuits are not static. Recent studies suggest clinical testing of dynamic balance using the timed tandem gait test has clinical utility in mTBI management (Oldham et al., 2018). However, other aspects of balance control remain untested in mTBI management.
Balance control can be separated into three domains based on the goal of the task: (1) maintaining a posture (e.g., standing balance), (2) transitioning between postures with voluntary movement (e.g., gait, turning, gait initiation/termination, standing from a chair), or (3) restoring stability (e.g., recovering from a trip, slip, or push) (Pollock et al., 2000). Objective measures of standing balance from instrumented force platforms (DeBeaumont et al., 2011; Quatman-Yates et al., 2015; Fino et al., 2016b) and inertial sensors (King et al., 2014; Parrington et al., 2019) suggest maintenance of posture can remain impaired beyond the clinical RTP decision in athletes, and gait and turning abnormalities suggest postural control during transitions may also be affected for up to 1 year after mTBI (Fino et al., 2016a, 2018). Yet, there is limited knowledge of how mTBI affects the restoration of stability after loss of balance. There is evidence to suggest that mTBI history affects stability after dynamic movement in athletes (Lynall et al., 2020). However, no study has examined reactive stabilization following an unexpected external disturbance in athletes after mTBI. Considering athletes are frequently required to recover balance after an external perturbation or sudden destabilizing event, examining the effects of mTBI on restoring stability is important for a comprehensive understanding of the impact of mTBI in real-world and sporting environments.
Reactive postural responses rely on short-, medium-, and long-latency responses, resulting from interaction among spinal circuits, the brainstem, and the cerebral cortex. The short-latency response is likely spinally mediated and is too small to stabilize balance, whereas medium- and long-latency responses are mediated by the brainstem and cortex and stabilize balance via whole-body, synergistic muscle activations (Jacobs and Horak, 2007). During change in support responses in particular, a transcortical loop through the motor cortex is involved in initiation (Jacobs and Horak, 2007). Yet, the response to a perturbation is also dependent on the preperturbation central set, which is the neuromotor state resulting from changes in initial contexts such as changes in cognitive state or sensorimotor conditions (Jacobs and Horak, 2007). Prior to a loss of balance, cortical loops update the central set to prime postural responses (Jacobs and Horak, 2007; Mochizuki et al., 2008) in an anticipatory manner according to stored visual information about the surrounding environment (Maki et al., 2001; Zettel et al., 2005, 2008a,b). Because attentional resources may be required to maintain an appropriate central set, concurrent cognitive demands can limit this anticipatory preparation; attentional resources switch from the concurrent cognitive task to the balance-recovery task after the postural disturbance (Maki and McIlroy, 2007), and delayed set-switching can compromise the ensuing stepping response (Maki et al., 2001). Concurrent cognitive tasks also attenuate the N1 perturbation-evoked response within the medial prefrontal cortex (Quant et al., 2004) associated with task-switching (Rushworth et al., 2002). Persistent executive and attentional dysfunction (Howell et al., 2013b) and lasting structural and functional neuroimaging abnormalities within the frontal and prefrontal cortex (Chamard et al., 2016; Churchill et al., 2017a,b,c; Bigler, 2018), may limit the cognitive reserve (Stern, 2009) for completing simultaneous tasks after mTBI and could be responsible for impaired dual-task balance. Based on these previous findings, we posit that persistently slower set-switching reaction times and larger switch costs after mTBI (Mayr et al., 2014; Moore et al., 2014) may adversely affect balance recovery, particularly in highly dynamic sporting environments with changing motor and cognitive demands. While dual-task paradigms involving simultaneous static or dynamic balance and cognitive tasks elicit large balance deficits in individuals with mTBI (Howell et al., 2013a; Lee et al., 2013; Register-Mihalik et al., 2013; Fino et al., 2018), the influence of cognitive demands on the ability to restore stability remains unclear after mTBI.
Numerous research groups suspect that there is a link between impaired neuromuscular control and musculoskeletal injuries after mTBI. Notably, both of these aspects have solid grounding in the literature; deficits in neuromuscular control after mTBI are widely reported in the literature (Howell et al., 2018b; Wilkerson et al., 2018) and musculoskeletal injuries are around two times more likely after mTBI (Nordström et al., 2014; Lynall et al., 2015, 2017; Pietrosimone et al., 2015; Brooks et al., 2016; Cross et al., 2016; Gilbert et al., 2016; Herman et al., 2017; Fino et al., 2019; Reneker et al., 2019). Recently, dual-task gait impairment after mTBI has been linked to musculoskeletal injury risk (Howell et al., 2018a). Despite the overlap and apparent connection, a concrete connection between post-mTBI deficits and musculoskeletal injuries has yet to be established. Although absent from mTBI literature to date, imprecise responses following a loss of balance may contribute to the high rate of musculoskeletal injuries after mTBI. In healthy collegiate athletes, postural responses following the release of a sustained force at the trunk, with the lower body fixed, are highly associated with future injuries to the low back (Cholewicki et al., 2005) and knee (Zazulak et al., 2007). Disrupted perception–action coupling has been suggested as an alternative potential mechanism for post-mTBI musculoskeletal injury. This theory suggests that neurophysiological dysfunction stemming from mTBI dysregulates the perception–action coupling loop (Eagle et al., 2020); misestimating one's abilities (both the participant's physical abilities related to the environment and the participant's perception based on repeated action in the environment) may manifest in improper timing of movement or body position and increase behavioral risk after mTBI (Eagle et al., 2020). However, direct experimental evidence is lacking.
Two major challenges in establishing the connection between musculoskeletal injury risk and neuromuscular deficits are the lack of sensitivity of assessments and obtaining adequate sample size. Clinical tests often lack sensitivity and specificity, particularly to subtle motor deficits that may have practical implications. There is growing interest in using neuroimaging and serum biomarkers to examine changes in cerebrovascular reactivity (Churchill et al., 2020) and systemic inflammation (Di Battista et al., 2019) after mTBI, but a direct connection between mTBI and musculoskeletal injury risk remains unclear. In a recent study, several clinical tests including the standard assessment of concussion (SAC), BESS, Immediate Post-Concussion Assessment and Cognitive Training (ImPACT), clinical reaction time, and the King-Devick failed to predict musculoskeletal injury risk after mTBI (Buckley et al., 2020). As a consequence, more sophisticated tests and instrumentation are often used to determine post-mTBI neuromuscular deficits. These assessments, often conducted within biomechanical laboratories, are not feasible in clinical settings, a necessary requirement for adequate power and future clinical translation. Recent technological advances in wearable, inertial sensors may be capable of addressing these limitations by enabling objective and sensitive assessments in clinical settings (Horak et al., 2015; Nouredanesh and Tung, 2015; El-Gohary et al., 2017). For example, instrumenting the BESS with a single inertial sensor identified acute mTBI more accurately than the clinical error count (King et al., 2017; Parrington et al., 2018). Inertial sensors can identify subtle deficits in gait, distinguishing between healthy controls and individuals within 72 h of mTBI and up to 2 weeks postinjury (Howell et al., 2015). Recently, objective qualities of gait, quantified with inertial sensors, differed between athletes with mTBI who went on to sustain a future lower extremity musculoskeletal injury and those who did not (Oldham et al., 2020). Similarly, worsening dual task cost of walking speed throughout mTBI recovery was associated with risk of incurring a sport-related injury during the year following mTBI (Howell et al., 2018a). Because inertial sensors are portable and clinically feasible, they can be used to facilitate clinical testing on large numbers of collegiate athletes.
Similar to static balance and gait, clinical assessments of reactive postural responses in other populations rely on scoring guidelines based on visual observation, but wireless, wearable inertial sensors can improve the accuracy of these assessments. For example, inertial sensors can detect differences in reactive postural responses between disease states [healthy control, multiple sclerosis (Smith et al., 2016; El-Gohary et al., 2017), Parkinson disease (Smith et al., 2016)]. Wearable sensors also provide information about quality of movement using a variety of objective characteristics. However, no study has used inertial sensors to quantify reactive postural responses in clinical mTBI settings. Improved understanding of how mTBI impacts the quality of balance recovery is critical to determine the implications of persistent balance impairments after mTBI, such as increased injury risk, and to develop clinical rehabilitation strategies to optimize athletes' neuromuscular control prior to return to competitive activity.
Our central hypothesis is that the high rate of musculoskeletal injuries after mTBI is in part due to impaired postural responses and balance recovery. Therefore, the purpose of this study is to (1) characterize reactive postural responses in athletes with recent mTBI compared to healthy control subjects, (2) determine the extent to which postural responses remain impaired in athletes with recent mTBI who have been cleared to RTP, and (3) determine the relationship between postural responses and acute lower extremity musculoskeletal injuries in a general sample of healthy collegiate athletes. We hypothesize that (1a) athletes with recent mTBI will take longer to regain balance during assessments of reactive postural responses compared to control athletes; (1b) athletes with recent mTBI will have larger deficits, in postural responses when simultaneously performing a dual task, relative to single task; (2) athletes with recent mTBI will improve reactive postural responses but will still demonstrate deficits compared to control subjects at all time points; (3a) that impaired postural responses at a baseline assessment will be associated with a faster time to acute musculoskeletal injury in healthy collegiate athletes; and (3b) objective measures of reactive postural responses will be better predictors of musculoskeletal injuries compared to clinical measures.
Methods
This study has two phases that will run concurrently. Phase 1 includes the first two aims: (1) to evaluate acute differences in postural responses after mTBI and (2) to evaluate persistent mTBI-related deficits in postural responses after RTP. Phase 2 contains the third aim: to characterize associations between baseline variations in postural responses and prospective lower extremity injuries in healthy athletes.
Phase 1: Characterization of Postural Responses After mTBI and Persistent mTBI-Related Deficits
Participants
For phase 1, 80 subjects will be recruited (40 athletes who experienced recent mTBI and 40 healthy teammate controls, matched on position and practice exposure). Sports medicine fellowship–trained team physicians with at least 10 years of training and clinical experience will diagnose mTBI based on history and physical examination, which is currently the gold standard. All subjects will be between the ages of 18 and 30 years and tested on site in the Athletic Training Clinics at the University of Utah. Informed written consent will be obtained from all participants.
Inclusion and Exclusion Criteria
For phase 1, inclusion criteria for mTBI recruitment are (1) age 18 to 30 years, (2) current participant in NCAA Division I athletics, and (3) mTBI within last 10 days (subjects must be assessed before beginning RTP protocol, even if acute time point is missed). Exclusion criteria for mTBI subjects are (1) previous history of vestibular or somatosensory pathology, (2) history of major injury to either leg requiring surgery within the last 2 years, and (3) body mass index (BMI) >40 kg/m2. Inclusion criteria for matched controls are (1) age 18–30 years, (2) current participant in NCAA Division I athletics. Exclusion criteria for healthy control subjects are (1) previous history of vestibular or somatosensory pathology, (2) recent mTBI (<1 year), (3) history of major injury to either leg requiring surgery within the last 2 years, and (4) BMI >40 kg/m2.
Assessment Procedures
For phase 1, athletes will be assessed at four time points: (1) within 48 h of mTBI (acute), (2) within 24 h of starting RTP protocol (PreRTP), (3) within 24 h of being medically cleared for RTP (PostRTP), and (4) ~6 months after injury (6Month). Assessments will take ~15 min and will be administered at the end of current Pac-12 CARE Affiliated Project (CAP) testing (Broglio et al., 2017). CAP testing is a tablet-based battery of measures that examine personal and family health history, neurocognitive assessments, neurological status, postural stability, symptoms, and oculomotor/oculovestibular function. Tests included in CAP testing are the Sport Concussion Assessment Tool, Fifth Edition; BESS; Brief Symptom Inventory 18; SAC; ImPACT; and EYESYNC (SyncThink, Palo Alto, CA) measures (Maruta et al., 2018). Assessments of matched control subjects will be tested within 24 h of the testing session of the athlete with mTBI. Return to play will follow guidelines set forth by the University of Utah Concussion Management Plan. Athletes will progress to RTP when, under the guidance of the physician and athletic trainer, the assessment battery including symptom assessment, ImPACT, and BESS has normalized. In the current study, athletes will be cleared to start RTP by only a limited number of team physicians.
Within 48 h from the time of the mTBI, informed written consent will be obtained from the athlete with mTBI and the matched control teammate. A total of 40 athletes with mTBI and 40 matched control athletes will complete assessments at four time points (Acute, PreRTP, PostRTP, 6Month). Each assessment will include questionnaires, clinical tests of function, and instrumented assessments of reactive postural responses (Table 1).
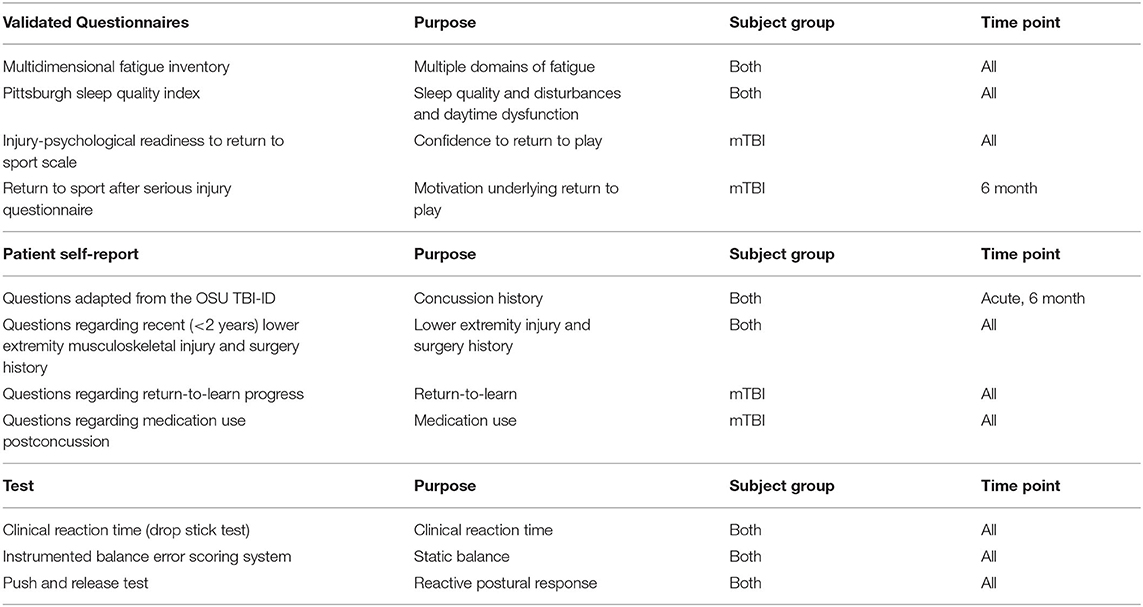
Table 1. Questionnaires, surveys, and tests utilized in the present study with description of subject group (mTBI, control, both) and time point (Acute, PreRTP, PostRTP, 6Month, all).
Questionnaires
At every time point, the athlete with mTBI will complete the following questionnaires: Multidimensional Fatigue Inventory (MFI), Pittsburgh Sleep Quality Index (PSQI), Injury-Psychological Readiness to Return to Sport (I-PRRS) scale. The MFI is a 20-item questionnaire designed to measure the following domains of fatigue: general fatigue, physical fatigue, mental fatigue, motivation, and activity (Smets et al., 1995). The PSQI is a 19-item questionnaire designed to assess sleep quality, sleep disturbances, and daytime dysfunction. In the present study we will exclude the five questions rated by the bed-partner or roommate (Buysse et al., 1989). The I-PRRS scale is a sport psychometric test to assess injured athletes' confidence and psychological readiness to return to play (Glazer, 2009). Athletes are asked to rate their confidence on a scale of 0 to 100 on six items related to competing in their sport. To determine motivation underlying return to sport, at the 6Month time point, the athlete with mTBI will also complete the 21-item Return to Sport after Serious Injury Questionnaire (Podlog and Eklund, 2005). Information regarding return-to-learn experience and medication use after mTBI will also be obtained. The matched control athlete will complete the MFI and PSQI at every time point.
Clinical reaction time
At every time point, both athletes with mTBI and matched control athletes will perform the Clinical Reaction Time test (RTclin). RTclin is a clinical test measuring simple reaction time using an 80-cm dowel with one end inserted in the middle of a hockey puck (Eckner et al., 2010). The participants will sit with their dominant arm on a table, leaving their wrist and hand off the side of the table. The investigator then brings the hockey puck to the level of the athlete's first finger and thumb. After a random duration between 2 and 5 s, the investigator releases the dowel, and the participant attempts to catch the dowel as soon as possible. The distance from the superior aspect of the hockey puck to the superior aspect of the participant's hand is measured. For the present study, two practice trials followed by eight measured trials will be completed by athletes at each assessment.
Instrumented BESS
Quiet standing balance will be assessed at every time point using the BESS (Bell et al., 2011). Both athletes with mTBI and matched control athletes will complete this test wearing five inertial measurement units (APDM Inc., Portland, OR) placed on the sternum, lumbar spine, right shank, and right and left feet. The footwear of the athletes will be recorded, and tests will be video recorded. The full BESS battery involves a double-leg stance, single-leg stance (on non-dominant foot), and tandem stance (nondominant behind dominant) performed on both firm and foam surfaces. Each trial will last 30 s, but errors will only be counted during the first 20 s.
Push and release reactive postural response paradigm
Reactive postural responses will be assessed using the Push and Release (P&R) test (Smith et al., 2016; El-Gohary et al., 2017). The P&R is a clinical test that examines reactive postural responses and compensatory stepping behavior after a loss of balance (Jacobs et al., 2006). Prior to each trial, a footplate (8″ long, 5.75″ wide at the toes, 4″ wide at the heels) will be placed on the floor, and the subject will be asked to press their feet firmly and symmetrically against each side of the footplate to ensure a standardized stance width at the beginning of each test. Subjects will lean, while supported by an investigator, until their center of mass is just beyond their base of support (Figure 1, left). After maintaining the supported leaning posture, the investigator will unexpectedly release their hands, requiring the subject to take a step (Figure 1, right). Subjects will perform the P&R, with eyes closed, in four directions: forward, backward, left, and right. Athletes demonstrate faster auditory and visual reaction times than healthy controls (Kaur et al., 2006) and typically perform better on balance tests than nonathletes (Davlin, 2004). Therefore, to increase difficulty of the P&R task for an athletic population, participants will be asked to close their eyes. During competition, athletes are often required to produce appropriate cognitive and motor responses simultaneously. Dual-task assessments are more sensitive to cognitive and motor impairments after mTBI than single-tasks beyond the acute phase of recovery (Register-Mihalik et al., 2013). Therefore, to make the P&R more functionally relevant and sensitive to cognitive and motor impairments, participants will be tested under single- and dual-task conditions. The dual task will be randomized and consists of serial subtraction by 3's, phonemic verbal fluency (FAS test), categorical verbal fluency (animal or fruit naming), and reciting every other letter of the alphabet (Tombaugh et al., 1999). During the dual-task condition, as soon as participants are in the supported leaning posture, they will be prompted with one of the four cognitive tasks. Participants will respond at least three times before being released unexpectedly by the investigator. Inertial measurement units (APDM Inc.) will be placed on the sternum, lumbar spine, right shank, and right and left feet. One sensor will be placed on the investigator's hand to determine release of support time. Data will be collected at 128 Hz and processed using custom-built algorithms in MATLAB (MathWorks, Natick, MA).
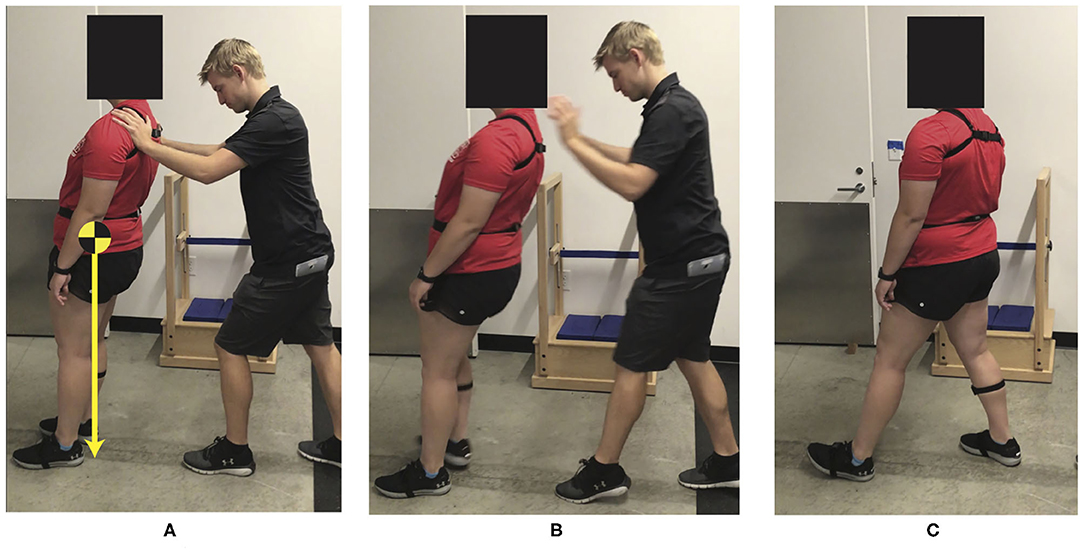
Figure 1. Push and release test (A) Prior to release, the subject's center of mass is beyond the subject's base of support (heels). (B) Immediately after release of support. (C) Subject recovered using one backward step.
Outcome Measures
Raw acceleration and angular velocity data from the five inertial measurement units will be used to calculate the following outcomes from the P&R test (Figure 2): step latency, step length, and time to stabilization.
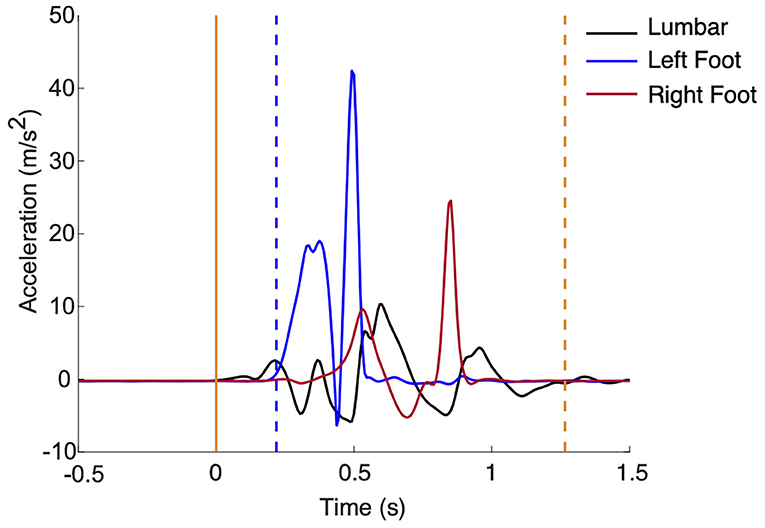
Figure 2. (Left) Support was released at time 0 (solid yellow). Step latency (blue dashed line) and time to stabilization (yellow dashed line) are marked.
Using data from the sensor on the investigator's hand, release of support (t0) will be identified when acceleration is >5% of gravity. Step latency will be calculated as the amount of time (seconds) from t0 to first foot movement. First foot movement will be detected when foot acceleration >7% of gravity and rotational rate is >7 degrees/s (El-Gohary et al., 2017). To determine step length (meters) of the first step, the double integral of acceleration of the foot from first foot movement to the next zero velocity instant will be calculated. Magnitude thresholds of 97.4 degrees/s for rotational rate and 0.8 m/s2 relative to gravity for acceleration will be used to determine the zero velocity instant (Rebula et al., 2013). Time to stabilization will be calculated as the amount of time (seconds) from t0 to stabilization. Stabilization will be detected when lumbar acceleration <7% of gravity and rotational rate <7 degrees/s, after the last step (El-Gohary et al., 2017).
The primary outcome measure will be time to stabilization. Secondary outcomes include step latency, step length, and the clinical score (number of steps) (Horak et al., 2009). The clinical score will be rated as follows: 0 = fall, 1 = recovers independently but takes more than 1 step, 2 = recovers independently with one step.
Sample Size
The sample size for aim 1 of phase 1 was calculated from differences between symptomatic concussed subjects and healthy controls in response to a tethered pull perturbation (Pan et al., 2015), with an estimated difference of 1.7 standard deviations (Cohen d = 1.7) between concussed subjects with residual balance complaints and healthy controls. With an effect size of 1.7, we will have >99% power to detect group differences at acute assessments with a 0.05 significance level and a two-sided t test with 40 subjects per group.
The sample size for aim 2 of phase 1 was based on an estimated effect size of Cohen d = 0.72 between asymptomatic, previously concussed individuals and healthy controls (Pan et al., 2015). If we observe a similar effect size of 0.72 for the P&R test, we expect 74% power to detect group differences at the primary PostRTP time point using a two-sided t test at a Bonferroni-corrected significance level of 0.0125 (0.05/4 for four outcomes: time to stabilization, step latency, step length and clinical score) with 40 subjects per group. Comparisons at PreRTP and 6Month assessments will also be performed but will be considered secondary. We also expect 88% power to detect group differences in the change between acute and subsequent assessments with 40 subjects per group.
Statistical Analysis
Aim 1 of phase 1: t tests will be used to determine if time to stabilization during the P&R differs between healthy subjects and subjects <48 h after concussion. Linear regression models will assess group differences while controlling for potential confounders of age, sex, contact/noncontact sport, and BMI. Concussion history will be recorded and explored as a covariate if necessary. As clinical scores are ordered with three levels, Wilcoxon Mann-Whitney U tests and generalized linear models for ordered multinomial outcomes (ordinal logistic regression) will compare clinical scores between groups. If the clinical scores exhibit a high ceiling effect, scores will be dichotomized and analyzed using χ2 tests and logistic models. t tests will assess whether concussed athletes had greater differences between single- and dual-task conditions relative to controls.
Aim 2 of phase 1: Bonferroni-adjusted t tests will be used to compare groups PostRTP to determine whether athletes with a recent mTBI take longer to stabilize during reactive postural responses after being medically cleared to RTP. Analogous secondary tests will be used for the PreRTP and 6Month assessments. We will also compute the difference in time to stabilization between assessments (e.g., change from Acute to PostRTP) to determine whether concussed athletes improved their postural responses over time. t tests will compare the group differences in the change between assessments. Longitudinal analysis using generalized estimating equations (GEEs) with compound symmetry working covariance models will be used to contrast each outcome of the P&R test at each assessment while controlling for lean direction; single vs. dual task; and possible confounders of age, sex, contact/noncontact sport, and BMI. A group-by-assessment interaction will determine if adjusted group differences changed between assessments. Two- and three-way interactions with task (group × task, group × task × assessment) will be included to test for group-difference in task, and if significant, final models will include these interactions. Ordinal logistic regression in a GEE framework will be used to assess changes in clinical scores between assessments.
Phase 2: Examining the Relationship Between Postural Responses and Acute Lower Extremity Musculoskeletal Injuries
Participants
For phase 2, a minimum of 200 subjects will be recruited before the start of their competitive season and prospectively tracked for 1 year. All subjects will be between the ages of 18 and 30 years and tested on site in the Athletic Training Clinics at the University of Utah. Informed written consent will be obtained from all participants.
Inclusion and Exclusion Criteria
For phase 2, inclusion criteria are as follows: (1) age 18 to 30 years and (2) current participant in NCAA Division I athletics. Exclusion criteria are as follows: (1) recent (within 6 months) or planned surgery that would result in future time loss and practice/competition exposure and (2) chronic conditions that could confound testing procedures (overuse injuries, medical conditions).
Assessment Procedures
Phase 2 assessments will be included as part of preseason physical baseline testing. Acute lower extremity musculoskeletal injuries for each athlete in phase 2 will be prospectively tracked for 1 year from the date of first team activity.
The phase 2 assessment will be identical to the phase 1 matched control athlete assessment. Additionally, mTBI history will be recorded using the Ohio State Traumatic Brain Injury Identification Method (OSU TBI-ID). While the OSU TBI-ID is not made specifically for mTBI, it is well validated (Corrigan and Bogner, 2007; Bogner and Corrigan, 2009) and recommended by the National Institute of Neurological Disorders and Stroke as a Common Data Element for mTBI studies. Lower extremity injury history, including the date of injury, location, type of injury (e.g., sprain, fracture), cause, time lost from sport, and relation of each injury to the athlete's primary sport, will be recorded.
Prospective Musculoskeletal Injury Risk
Acute lower extremity musculoskeletal injuries for each athlete in phase 2 will be prospectively tracked for 1 year from the date of their first team activity. Injury data will be queried from the athletes' electronic medical records documented by the University of Utah Athletic Training Staff and maintained for the Pac-12 Sports Injury Registry Management and Analytics Program. Injuries of interest will include any acute orthopedic injury of the lower extremity, pelvis, lumbar spine, or abdomen that resulted in time lost, including but not limited to joint sprains, musculotendinous strains, and fractures. Because abdominal and lumbar muscles are required for stability and movement control (Cholewicki et al., 1997), and the lumbar spine is a critical junction of postural control during sport with high musculoskeletal loads and compressive muscle forces, both lumbar spine and abdominal injuries will be included. Any injury that results in an athlete being unable to fully participate in training or match play will be considered a time loss injury (Fuller et al., 2007; Howell et al., 2018a). Overuse injuries and preexisting conditions will be excluded. The primary outcome measure will be time to first musculoskeletal injury. The number of injuries within 1 year and severity of injuries (time lost from a given injury) will be included as secondary outcomes.
Statistical Analysis
Time from first team activity to first musculoskeletal injury will be summarized using Kaplan–Meier methods. Cox proportional hazards models will be constructed to determine if time to stabilization during postural responses is associated with faster times to injury in the general collegiate athlete population (hypothesis 3a). Similar models will be performed for each secondary outcome. Models will first be unadjusted and then potentially adjusted for age, gender, sport, history of a recent musculoskeletal injury, and history of mTBI before enrollment. The number of variables that we will adjust for will depend on the number of musculoskeletal injuries observed. The inclusion of sport as a covariate will account for variation in the biomechanical demands, exposures, and schedules across different sports. To determine if time to stabilization is a better predictor of future injury than clinical score, similar models will be constructed for the P&R clinical score, and we will assess each model's predictive ability using c-statistics (hypothesis 3b). Models with the highest c-statistic value will indicate the outcome most predictive of time to injury.
Sample Size Justification
We will have 80% power to detect a minimum hazard ratio of 1.53 per 1-s increase in stabilization time with a total sample size of 200 healthy participants, an event rate of 22%, and a standard deviation of 1 s using a Cox regression model at the 0.05 significance level. The event rate was estimated from the average number of athletes who experienced musculoskeletal injuries at the University of Utah from 2015 to 2018.
Discussion
While a number of studies have examined static balance and gait after mTBI, reactive postural responses are an essential component of balance control for athletic performance and remain largely unstudied. An inability to accurately respond to postural disturbances could contribute to the increased musculoskeletal injury risk after mTBI. Therefore, the primary goal of this study is to determine if impaired reactive postural responses contribute to the increased musculoskeletal injury risk after mTBI. Using a two-phase approach, we will determine to what extent, and for how long, reactive postural responses are impaired after mTBI (phase 1), and we will determine the association between reactive postural responses and prospective musculoskeletal injury risk (phase 2). This study is unique in that it objectively measures reactive postural responses, an understudied component of balance, after mTBI.
Reactive postural responses are common in athletic competition and essential for athlete safety, but this domain of balance control has not been studied extensively after mTBI. Time to stability after dynamic movement can discriminate between those with history of mTBI and healthy controls (Lynall et al., 2020), suggesting that stability may be an important post-mTBI measure. However, no study has examined reactive postural responses in athletes with mTBI. We suspect the lack of knowledge concerning reactive postural responses after mTBI stems from two sources. First, reactive postural responses have been historically considered reflexive (subcortical) (Jacobs and Horak, 2007; Jacobs, 2014). However, it is likely that reactive postural responses rely on interaction among spinal circuits, the brainstem, and the cortex (Jacobs and Horak, 2007). As the focus of mTBI has traditionally been on cortical damage, postural responses may have been considered too automatic/reflexive to be disrupted by mTBI. The second possible reason for a gap in knowledge is logistical; traditional assessments of postural responses require sophisticated moving platforms (Zettel et al., 2008a,b) or tether-release (MacKey and Robinovitch, 2005; Inness et al., 2015) setups. Given some of the patient burden hurdles ingrained within clinical mTBI research, it is perhaps unsurprising that few studies have examined postural responses using these types of equipment. In fact, in designing this protocol, we considered implementing similarly controlled postural response paradigms (e.g., tether-release, moving platform, etc.). However, the need for a clinically feasible testing paradigm was critical to minimize the burden on student-athlete participants. Therefore, we selected the P&R test, a clinical test traditionally quantified using visual scoring. The notable weakness of this task is the relative simplicity for highly athletic individuals—few athletes should take multiple steps or struggle to recover. The use of wearable sensors is therefore essential and enables quantitative metrics of response latency, step length, and time to stabilization. The combination of wearable inertial sensors with the clinical P&R test facilitates assessments of reactive postural responses within an athletic training room with minimal added burden on the athlete. If we find athletes with recent mTBI take longer to regain stability or exhibit longer step latencies, it will provide evidence that mTBI impairs the reactive restoration of balance, demonstrating that mTBI affects all domains of balance control. Additionally, if we find differences between groups across time, it will support our hypothesis that postural responses are persistently impaired after mTBI. Such findings, coupled with the clinical feasibility of the P&R assessment, may spur the integration of postural response testing into the clinical management for mTBI. In the future, more work will be needed to develop outcome measures with established cutoff values for return-to-to play that could be incorporated into a testing battery.
The ability to carry out multiple tasks simultaneously, or to divide attention, is important in maintaining postural stability. Dual-task paradigms challenge the capacity for divided attention by requiring the participant to deal with competing cognitive and motor demands (Yogev-Seligmann et al., 2008). Because attentional capacity is limited, simultaneous performance of the tasks will cause a decline in one or both of the tasks (Posner and Boies, 1971; Kahneman, 1973). Correspondingly, dual-task deficits after mTBI during standing balance (Cavanaugh et al., 2005) and gait (Howell et al., 2013a, 2017) persist longer than motor tasks alone. If we find that reactive postural responses are impaired only in athletes with recent mTBI when performing a simultaneous cognitive task, it suggests that clinical evaluations of reactive balance control after mTBI should incorporate complex cognitive environments similar to gameplay. This finding would add support to existing literature demonstrating the need for dual-task assessments involving both physical and cognitive demands similar to those experienced during athletic competition.
A primary challenge in directly associating musculoskeletal injuries to underlying concussion deficits is adequate statistical power. To address this challenge, we considered the following to be true: if postural responses are associated with musculoskeletal within a population, the association will hold for a subgroup of that population. Therefore, if we find an association between reactive postural responses and the time to musculoskeletal injury in the general athletic population, irrespective of mTBI (phase 2), we expect that any impairment in reactive postural responses after mTBI will also associate with a faster time to injury. This assumption allows us to obtain adequate power for a longitudinal survival model within a reasonable timeframe (3 years). However, it does prompt a question about the validity of this assumption. The interpolation of a population-level association (all athletes) to a subgroup-level association (those with acute mTBI) will be directly tested by comparing the time to injury in athletes from phase 1 to test the validity of our assumption. Additionally, our concurrent two-phase approach will allow us to explore the association between changes in reactive postural responses from baseline-to-post-mTBI with time to injury using the small subset of athletes in which we will have baseline (pre-mTBI) and longitudinal post-mTBI measurements.
This study seeks to further our understanding of balance control after mTBI by examining a heretofore understudied, yet critically important aspect of balance, reactive postural responses. Through the innovative use of wearable sensor derived measures gathered during a clinical testing battery, the results of this study will improve our understanding of balance control post-mTBI and contribute to the foundation of relevant post-mTBI rehabilitation targets.
Ethics Statement
Written informed consent was obtained from the individual(s) for the publication of any potentially identifiable images or data included in this article.
Author Contributions
PF and LD led acquisition of funding from the PAC 12 that supports this project. AM and BC prepared the draft of the protocol with contributions from PF. AM, BC, RP, NF, AP, DC, NM, LD, and PF contributed to subsequent drafts and approved the final manuscript. All authors contributed to the article and approved the submitted version.
Funding
This project was supported with support from the Pac-12 Conference's Student-Athlete Health and Well-Being Initiative (PI: Fino, Dibble). Additional funding support was provided by the Eunice Kennedy Shiver National Institute of Child Health & Human Development of the National Institutes of Health under Award Number K12HD073945, and the University of Utah Study Design and Biostatistics Center, with funding in part from the National Center for Research Resources and the National Center for Advancing Translational Sciences, National Institutes of Health, through Grant UL1TR002538 (formerly 5UL1TR001067-05, 8UL1TR000105, and UL1RR025764). The content of this manuscript is solely the responsibility of the authors and does not necessarily represent the official views of the Pac-12 Conference, or its members, or of the National Institute of Health.
Conflict of Interest
The authors declare that the research was conducted in the absence of any commercial or financial relationships that could be construed as a potential conflict of interest.
Acknowledgments
The authors would like to thank the University of Utah Athletic Training Staff.
References
Bell, D. R., Guskiewicz, K. M., Clark, M. A., and Padua, D. A. (2011). Systematic review of the balance error scoring system. Sports Health. 3, 287–295. doi: 10.1177/1941738111403122
Bigler, E. D. (2018). Structural neuroimaging in sport-related concussion. Int. J. Psychophysiol. 132(Pt A), 105–23. doi: 10.1016/j.ijpsycho.2017.09.006
Bogner, J., and Corrigan, J. D. (2009). Reliability and predictive validity of the Ohio State University TBI identification method with prisoners. J. Head Trauma Rehab. 24, 279–291. doi: 10.1097/HTR.0b013e3181a66356
Broglio, S. P., Cantu, R. C., Gioia, G. A., Guskiewicz, K. M., Kutcher, J., Palm, M., et al. (2014). Management of sport concussion. J. Athletic Trai. 49, 245–265. doi: 10.4085/1062-6050-49.1.07
Broglio, S. P., McCrea, M., McAllister, T., Harezlak, J., Katz, B., Hack, D., et al. (2017). A national study on the effects of concussion in collegiate athletes and US military service academy members: the NCAA–DoD concussion assessment, research and education (CARE) Consortium Structure and Methods. Sports Med. 47, 1437–1451. doi: 10.1007/s40279-017-0707-1
Brooks, M. A., Peterson, K., Biese, K., Sanfilippo, J., Heiderscheit, B. C., and Bell, D. R. (2016). Concussion increases odds of sustaining a lower extremity musculoskeletal injury after return to play among collegiate athletes. Am J Sports Med. 44, 742–747. doi: 10.1177/0363546515622387
Buckley, T. A., Howard, C. M., Oldham, J. R., Lynall, R. C., Swanik, C. B., and Getchell, N. (2020). No clinical predictors of postconcussion musculoskeletal injury in college athletes. Med. Sci. Sports Exerc 52, 1256–1262. doi: 10.1249/MSS.0000000000002269
Buckley, T. A., Munkasy, B. A., and Clouse, B. P. (2018). Sensitivity and specificity of the modified balance error scoring system in concussed collegiate student athletes. Clin J Sport Med. 28, 174–176. doi: 10.1097/JSM.0000000000000426
Buysse, D. J., Reynolds, C. F., Monk, T. H., Berman, S. R., and Kupfer, D. J. (1989). The Pittsburgh sleep quality index: a new instrument for psychiatric practice and research. Psychiatry Res. 28, 193–213. doi: 10.1016/0165-1781(89)90047-4
Cavanaugh, J. T., Guskiewicz, K. M., Giuliani, C., Marshall, S., Mercer, V., and Stergiou, N. (2005). Detecting altered postural control after cerebral concussion in athletes with normal postural stability. Br. J. Sports Med 39, 805–811. doi: 10.1136/bjsm.2004.015909
Chamard, E., Lefebvre, G., Lassonde, M., and Theoret, H. (2016). Long-term abnormalities in the corpus callosum of female concussed athletes. J. Neurotrauma 33, 1220–1226. doi: 10.1089/neu.2015.3948
Cholewicki, J., Panjabi, M. M., and Khachatryan, A. (1997). Stabilizing function of trunk flexor-extensor muscles around a neutral spine posture. Spine 22, 2207–2212. doi: 10.1097/00007632-199710010-00003
Cholewicki, J., Silfies, S. P., Shah, R. A., Greene, H. S., Reeves, N. P., Alvi, K., et al. (2005). Delayed trunk muscle reflex responses increase the risk of low back injuries. Spine 30, 2614–2620. doi: 10.1097/01.brs.0000188273.27463.bc
Churchill, N., Hutchison, M., Richards, D., Leung, G., Graham, S., and Schweizer, T. A. (2017c). Brain structure and function associated with a history of sport concussion: a multi-modal magnetic resonance imaging study. J. Neurotrauma 34, 765–771. doi: 10.1089/neu.2016.4531
Churchill, N., Hutchison, M. G., Leung, G., Graham, S., and Schweizer, T. A. (2017b). Changes in functional connectivity of the brain associated with a history of sport concussion: a preliminary investigation. Brain Injury 31, 39–48. doi: 10.1080/02699052.2016.1221135
Churchill, N. W., Hutchison, M. G., Graham, S. J., and Schweizer, T. A. (2020). Cerebrovascular reactivity after sport concussion: from acute injury to 1 year after medical clearance. Front. Neurol 11:558. doi: 10.3389/fneur.2020.00558
Churchill, N. W., Hutchison, M. G., Richards, D., Leung, G., Graham, S. J., and Schweizer, T. A. (2017a). Neuroimaging of sport concussion: persistent alterations in brain structure and function at medical clearance. Sci. Rep 7:3. doi: 10.1038/s41598-017-07742-3
Corrigan, J. D., and Bogner, J. (2007). Initial reliability and validity of the Ohio State University TBI identification method. J Head Trauma Rehab. 22, 318–329. doi: 10.1097/01.HTR.0000300227.67748.77
Cross, M., Kemp, S., Smith, A., Trewartha, G., and Stokes, K. (2016). Professional rugby union players have a 60% greater risk of time loss injury after concussion: a 2-season prospective study of clinical outcomes. Br. J. Sports Med 50, 926–931. doi: 10.1136/bjsports-2015-094982
Davlin, C. D. (2004). Dynamic balance in high level athletes. Percep Motor Skills 98, 1171–76. doi: 10.2466/pms.98.3c.1171-1176
DeBeaumont, L., Mongeon, D., Tremblay, S., Messier, J., Prince, F., Leclerc, S., et al. (2011). Persistent motor system abnormalities in formerly concussed athletes. J. Athl. Train 46, 234–240. doi: 10.4085/1062-6050-46.3.234
Di Battista, A. P., Churchill, N., Rhind, S. G., Richards, D., and Hutchison, M. G. (2019). Evidence of a distinct peripheral inflammatory profile in sport-related concussion. J. Neuroinflammation 16:1. doi: 10.1186/s12974-019-1402-y
Eagle, S. R., Kontos, A. P., Pepping, G. J., Johnson, C. D., Sinnott, A., LaGoy, A., et al. (2020). Increased risk of musculoskeletal injury following sport-related concussion: a perception–action coupling approach. Sports Med. 50, 15–23. doi: 10.1007/s40279-019-01144-3
Eckner, J. T., Kutcher, J. S., and Richardson, J. K. (2010). Pilot evaluation of a novel clinical test of reaction time in national collegiate athletic association division i football players. J. Athl. Train 45, 327–332. doi: 10.4085/1062-6050-45.4.327
El-Gohary, M., Peterson, D., Gera, G., Horak, F. B., and Huisinga, J. M. (2017). Validity of the instrumented push and release test to quantify postural responses in persons with multiple sclerosis. Arch. Phys. Med. Rehabil 98, 1325–1331. doi: 10.1016/j.apmr.2017.01.030
Fino, P. C., Becker, L. N., Fino, N. F., Griesemer, B., Goforth, M., and Brolinson, P. G. (2019). Effects of recent concussion and injury history on instantaneous relative risk of lower extremity injury in division i collegiate athletes. Clin. J. Sport Med. 29, 218–223. doi: 10.1097/JSM.0000000000000502
Fino, P. C., Nussbaum, M. A., and Brolinson, P. G. (2016a). Locomotor deficits in recently concussed athletes and matched controls during single and dual-task turning gait: preliminary results. J. Neuroeng. Rehabil. 13:1. doi: 10.1186/s12984-016-0177-y
Fino, P. C., Nussbaum, M. A., and Brolinson, P. G. (2016b). Decreased high-frequency center-of-pressure complexity in recently concussed asymptomatic athletes. Gait Posture 50, 69–74. doi: 10.1016/j.gaitpost.2016.08.026
Fino, P. C., Parrington, L., Pitt, W., Martini, D. N., Chesnutt, J. C., Chou, L. S., et al. (2018). Detecting gait abnormalities after concussion or mild traumatic brain injury: a systematic review of single-task, dual-task, and complex gait. Gait Posture. 62, 157–166. doi: 10.1016/j.gaitpost.2018.03.021
Fuller, C. W., Molloy, M. G., Bagate, C., Bahr, R., Brooks, J. H. M., Donson, H., et al. (2007). Consensus statement on injury definitions and data collection procedures for studies of injuries in Rugby Union. Clin. J. Sport Med. 17, 177–181. doi: 10.1097/JSM.0b013e31803220b3
Gilbert, F. C., Burdette, G. T., Joyner, A. B., Llewellyn, T. A., and Buckley, T. A. (2016). Association between concussion and lower extremity injuries in collegiate athletes. Sports Health 8, 561–567. doi: 10.1177/1941738116666509
Glazer, D. D. (2009). Development and preliminary validation of the injury-psychological readiness to return to sport (I-PRRS) scale. J. Athl. Train 44, 185–189. doi: 10.4085/1062-6050-44.2.185
Guskiewicz, K. M. (2011). Balance assessment in the management of sport-related concussion. Clin. Sports Med. 30, 89–102. doi: 10.1016/j.csm.2010.09.004
Herman, D. C., Jones, D., Harrison, A., Moser, M., Tillman, S., Farmer, K., et al. (2017). Concussion may increase the risk of subsequent lower extremity musculoskeletal injury in collegiate athletes. Sports Med. 47, 1003–1010. doi: 10.1007/s40279-016-0607-9
Horak, F., King, L., and Mancini, M. (2015). Role of body-worn movement monitor technology for balance and gait rehabilitation. Phys. Ther 95, 461–470. doi: 10.2522/ptj.20140253
Horak, F. B., Wrisley, D. M., and Frank, J. (2009). The balance evaluation systems test (BESTest) to differentiate balance deficits. Phys. Ther. 89, 484–498. doi: 10.2522/ptj.20080071
Howell, D., Osternig, L., and Chou, L. S. (2015). Monitoring recovery of gait balance control following concussion using an accelerometer. J. Biomech 48, 3364–3368. doi: 10.1016/j.jbiomech.2015.06.014
Howell, D., Osternig, L., Van Donkelaar, P., Mayr, U., and Chou, L. S. (2013b). Effects of concussion on attention and executive function in adolescents. Med. Sci. Sports Exerc 45, 1030–1037. doi: 10.1249/MSS.0b013e3182814595
Howell, D. R., Buckley, T. A., Lynall, R. C., and Meehan, W. P. (2018a). Worsening dual-task gait costs after concussion and their association with subsequent sport-related injury. J. Neurotrauma 35, 1630–1636. doi: 10.1089/neu.2017.5570
Howell, D. R., Lynall, R. C., Buckley, T. A., and Herman, D. C. (2018b). Neuromuscular control deficits and the risk of subsequent injury after a concussion: a scoping review. Sports Med. 48, 1097–1115. doi: 10.1007/s40279-018-0871-y
Howell, D. R., Osternig, L. R., and Chou, L. S. (2013a). Dual-task effect on gait balance control in adolescents with concussion. Arch. Phys. Med. Rehabil 94, 1513–1520. doi: 10.1016/j.apmr.2013.04.015
Howell, D. R., Osternig, L. R., and Chou, L. S. (2017). Single-task and dual-task tandem gait test performance after concussion. J. Sci. Med. Sport 20, 622–626. doi: 10.1016/j.jsams.2016.11.020
Inness, E. L., Mansfield, A., Biasin, L., Brunton, K., Bayley, M., and McIlroy, W. E. (2015). Clinical implementation of a reactive balance control assessment in a sub-acute stroke patient population using a ‘lean-and-release' methodology. Gait Posture 41, 529–534. doi: 10.1016/j.gaitpost.2014.12.005
Jacobs, J. V. (2014). Why we need to better understand the cortical neurophysiology of impaired postural responses with age, disease, or injury. Front. Int. Neurosci. 8:69. doi: 10.3389/fnint.2014.00069
Jacobs, J. V., and Horak, F. B. (2007). Cortical control of postural responses. J. Neural Trans. 114:1339–48. doi: 10.1007/s00702-007-0657-0
Jacobs, J. V., Horak, F. B., Van Tran, K., and Nutt, J. G. (2006). An alternative clinical postural stability test for patients with Parkinson's Disease. J. Neurol 253, 1404–1413. doi: 10.1007/s00415-006-0224-x
Kaur, P., Paul, M., and Sandhu, J. S. (2006). Auditory and visual reaction time in athletes, healthy controls, and patients of type 1 diabetes mellitus: A comparative study. Int. J. Diabetes Dev. C 26, 112–115. doi: 10.4103/0973-3930.32170
King, L. A., Horak, F. B., Mancini, M., Pierce, D., Priest, K. C., Chesnutt, J., et al. (2014). Instrumenting the balance error scoring system for use with patients reporting persistent balance problems after mild traumatic brain injury. Arch. Phys. Med. Rehabil 95, 353–359. doi: 10.1016/j.apmr.2013.10.015
King, L. A., Mancini, M., Fino, P. C., Chesnutt, J., Swanson, C. W., Markwardt, S., et al. (2017). Sensor-based balance measures outperform modified balance error scoring system in identifying acute concussion. Ann. Biomed. Eng 45, 2135–2145. doi: 10.1007/s10439-017-1856-y
Lee, H., Sullivan, S. J., and Schneiders, A. G. (2013). The use of the dual-task paradigm in detecting gait performance deficits following a sports-related concussion: a systematic review and meta-analysis. J. Sci. Med. Sport. 16, 2–7. doi: 10.1016/j.jsams.2012.03.013
Lynall, R. C., Campbell, K. R., Mauntel, T. C., Blackburn, J. T., and Mihalik, J. P. (2020). Single-legged hop and single-legged squat balance performance in recreational athletes with a history of concussion. J. Athl. Train 55, 488–493. doi: 10.4085/1062-6050-185-19
Lynall, R. C., Mauntel, T. C., Padua, D. A., and Mihalik, J. P. (2015). Acute lower extremity injury rates increase after concussion in college athletes. Med. Sci. Sports Exerc 47, 2487–2492. doi: 10.1249/MSS.0000000000000716
Lynall, R. C., Mauntel, T. C., Pohlig, R. T., Kerr, Z. Y., Dompier, T. P., Hall, E. E., et al. (2017). Lower extremity musculoskeletal injury risk after concussion recovery in high school athletes. J. Athl. Train 52, 1028–1034. doi: 10.4085/1062-6050-52.11.22
MacKey, D. C., and Robinovitch, S. N. (2005). Postural steadiness during quiet stance does not associate with ability to recover balance in older women. Clin. Biomech. 20, 776–783. doi: 10.1016/j.clinbiomech.2005.05.002
Maki, B. E., and McIlroy, W. E. (2007). Cognitive demands and cortical control of human balance-recovery reactions. J. Neural. Transm. 114, 1279–96. doi: 10.1007/s00702-007-0764-y
Maki, B. E., Zecevic, A., Bateni, H., Kirshenbaum, N., and McIlroy, W. E. (2001). Cognitive demands of executing postural reactions: does aging impede attention switching? Neuroreport 12, 3583–3587. doi: 10.1097/00001756-200111160-00042
Maruta, J., Spielman, L. A., Rajashekar, U., and Ghajar, J. (2018). Association of visual tracking metrics with post-concussion symptomatology. Front. Neurol. 9:611. doi: 10.3389/fneur.2018.00611
Mayr, U., LaRoux, C., Rolheiser, T., Osternig, L., Chou, L. S., and Van Donkelaar, P. (2014). Executive dysfunction assessed with a task-switching task following concussion. PLoS ONE 9:e0091379. doi: 10.1371/journal.pone.0091379
Mochizuki, G., Sibley, K. M., Esposito, J. G., Camilleri, J. M., and McIlroy, W. E. (2008). Cortical responses associated with the preparation and reaction to full-body perturbations to upright stability. Clin. Neurophysiol. 119, 1626–1637. doi: 10.1016/j.clinph.2008.03.020
Moore, R. D., Hillman, C. H., and Broglio, S. P. (2014). The persistent influence of concussive injuries on cognitive control and neuroelectric function. J. Athl. Train 49, 24–35. doi: 10.4085/1062-6050-49.1.01
Nordström, A., Nordström, P., and Ekstrand, J. (2014). Sports-related concussion increases the risk of subsequent injury by about 50% in elite male football players. Br. J. Sports Med. 48, 1447–1450. doi: 10.1136/bjsports-2013-093406
Nouredanesh, M., and Tung, J. (2015). Machine learning based detection of compensatory balance responses to lateral perturbation using wearable sensors. In: IEEE Biomedical Circuits and Systems Conference: Engineering for Healthy Minds and Able Bodies, BioCAS 2015 - Proceedings. Atlanta, GA: Institute of Electrical and Electronics Engineers Inc. doi: 10.1109/BioCAS.2015.7348282
Oldham, J. R., Difabio, M. S., Kaminski, T. W., Dewolf, R. M., Howell, D. R., and Buckley, T. A. (2018). Efficacy of tandem gait to identify impaired postural control after concussion. Med. Sci. Sports Exerc. 50, 1162–1168. doi: 10.1249/MSS.0000000000001540
Oldham, J. R., Howell, D. R., Knight, C. A., Crenshaw, J. R., and Buckley, T. A. (2020). Gait performance is associated with subsequent lower extremity injury following concussion. Med Sci Sports Exerc. 1:2385. doi: 10.1249/mss.0000000000002385
Pan, T., Liao, K., Roenigk, K., Daly, J. J., and Walker, M. F. (2015). Static and dynamic postural stability in veterans with combat-related mild traumatic brain injury. Gait Post. 42, 550–557. doi: 10.1016/j.gaitpost.2015.08.012
Parrington, L., Fino, N. F., Fino, P. C., Murchison, C. F., Chesnutt, J. C., and King, L. A. (2018). Inflection points in longitudinal models: tracking recovery and return to play following concussion. Scand. J. Med. Sci. Sports 28, 2436–2442. doi: 10.1111/sms.13239
Parrington, L., Fino, P. C., Swanson, C. W., Murchison, C. F., Chesnutt, J., and King, L. A. (2019). Longitudinal assessment of balance and gait after concussion and return to play in collegiate athletes. J. Athl. Train 54, 429–438. doi: 10.4085/1062-6050-46-18
Pietrosimone, B., Golightly, Y. M., Mihalik, J. P., and Guskiewicz, K. M. (2015). Concussion frequency associates with musculoskeletal injury in retired NFL players. Med. Sci. Sports Exerc 47, 2366–2372. doi: 10.1249/MSS.0000000000000684
Podlog, L., and Eklund, R. C. (2005). Return to sport after serious injury: a retrospective examination of motivation and psychological outcomes. J. Sport Rehab. 14, 20–34. doi: 10.1123/jsr.14.1.20
Pollock, A. S., Durward, B. R., Rowe, P. J., and Paul, J. P. (2000). What Is Balance? Clin. Rehabil. 14, 402–406. doi: 10.1191/0269215500cr342oa
Posner, M. I., and Boies, S. J. (1971). Components of attention. Psychol. Rev. 78, 391–408. doi: 10.1037/h0031333
Quant, S., Adkin, A. L., Staines, W. R., Maki, B. E., and McIlroy, W. E. (2004). The effect of a concurrent cognitive task on cortical potentials evoked by unpredictable balance perturbations. BMC Neurosci. 5:18. doi: 10.1186/1471-2202-5-18
Quatman-Yates, C. C., Bonnette, S., Hugentobler, J. A., Méd,é, B., Kiefer, A. W., Kurowski, B. G., et al. (2015). Postconcussion postural sway variability changes in youth: the benefit of structural variability analyses. Pediatr. Phys. Ther. 27, 316–327. doi: 10.1097/PEP.0000000000000193
Rebula, J. R., Ojeda, L. V., Adamczyk, P. G., and Kuo, A. D. (2013). Measurement of foot placement and its variability with inertial sensors. Gait Posture 38, 974–980. doi: 10.1016/j.gaitpost.2013.05.012
Register-Mihalik, J. K., Littleton, A. C., and Guskiewicz, K. M. (2013). Are divided attention tasks useful in the assessment and management of sport-related concussion? Neuropsychol Rev. 23, 300–313. doi: 10.1007/s11065-013-9238-1
Reneker, J. C., Babl, R., and Flowers, M. M. (2019). History of concussion and risk of subsequent injury in athletes and service members: a systematic review and meta-analysis. Muscul. Sci. Prac. 42, 173–185. doi: 10.1016/j.msksp.2019.04.004
Riemann, B. L., and Guskiewicz, K. M. (2000). Effects of mild head injury on postural stability as measured through clinical balance testing. J. Athletic Train. 35, 19–25. https://pubmed.ncbi.nlm.nih.gov/16558603/.
Rushworth, M. F. S., Hadland, K. A., Paus, T., and Sipila, P. K. (2002). Role of the human medial frontal cortex in task switching: a combined FMRI and TMS study. J. Neurophysiol 87, 2577–2592. doi: 10.1152/jn.2002.87.5.2577
Smets, E. M. A., Garssen, B., Bonke, B., and De Haes, J. C. J. M. (1995). The multidimensional fatigue inventory (MFI) psychometric qualities of an instrument to assess fatigue. J. Psychosom. Res 39, 315–325. doi: 10.1016/0022-3999(94)00125-O
Smith, B. A., Carlson-Kuhta, P., and Horak, F. B. (2016). Consistency in administration and response for the backward push and release test: a clinical assessment of postural responses. Physiother. Res. Int. 21, 36–46. doi: 10.1002/pri.1615
Stern, Y. (2009). Cognitive reserve. Neuropsychologia. 47, 2015–2028. doi: 10.1016/j.neuropsychologia.2009.03.004
Tombaugh, T. N., Kozak, J., and Rees, L. (1999). Normative data stratified by age and education for two measures of verbal fluency. Arch. Clin. Neuropsychol. 14, 167–77.
Wilkerson, G. B., Nabhan, D. C., Prusmack, C. J., and Moreau, W. J. (2018). Detection of persisting concussion effects on neuromechanical responsiveness. Med. Sci. Sports Exerc 50, 1750–1756. doi: 10.1249/MSS.0000000000001647
Yogev-Seligmann, G., Hausdorff, J. M., and Giladi, N. (2008). The role of executive function and attention in gait. Mov Disord. 23, 329–342. doi: 10.1002/mds.21720
Zazulak, B. T., Hewett, T. E., Reeves, N. P., Goldberg, B., and Cholewicki, J. (2007). Deficits in neuromuscular control of the trunk predict knee injury risk: a prospective biomechanical-epidemiologic study. Am. J. Sports Med. 35, 1123–1130. doi: 10.1177/0363546507301585
Zettel, J. L., Holbeche, A., McIlroy, W. E., and Maki, B. E. (2005). Redirection of gaze and switching of attention during rapid stepping reactions evoked by unpredictable postural perturbation. Exp. Brain Res. 165, 392–401. doi: 10.1007/s00221-005-2310-1
Zettel, J. L., McIlroy, W. E., and Maki, B. E. (2008a). Effect of competing attentional demands on perturbation-evoked stepping reactions and associated gaze behavior in young and older adults. J. Gerontol. 63, 1370–1379. doi: 10.1093/gerona/63.12.1370
Keywords: wearable sensors, push and release test, return-to-play, compensatory stepping, concussion
Citation: Morris A, Cassidy B, Pelo R, Fino NF, Presson AP, Cushman DM, Monson NE, Dibble LE and Fino PC (2020) Reactive Postural Responses After Mild Traumatic Brain Injury and Their Association With Musculoskeletal Injury Risk in Collegiate Athletes: A Study Protocol. Front. Sports Act. Living 2:574848. doi: 10.3389/fspor.2020.574848
Received: 21 June 2020; Accepted: 11 September 2020;
Published: 29 October 2020.
Edited by:
Jeffrey B. Taylor, University of Tennessee Health Science Center (UTHSC), United StatesReviewed by:
Thomas Buckley, University of Delaware, United StatesRobert C. Lynall, University of Georgia, United States
Copyright © 2020 Morris, Cassidy, Pelo, Fino, Presson, Cushman, Monson, Dibble and Fino. This is an open-access article distributed under the terms of the Creative Commons Attribution License (CC BY). The use, distribution or reproduction in other forums is permitted, provided the original author(s) and the copyright owner(s) are credited and that the original publication in this journal is cited, in accordance with accepted academic practice. No use, distribution or reproduction is permitted which does not comply with these terms.
*Correspondence: Peter C. Fino, cGV0ZXIuZmlub0B1dGFoLmVkdQ==