- 1Département de kinésiologie, Université Laval, Quebec, QC, Canada
- 2Institut National du sport du Québec, Montreal, QC, Canada
Recent data suggests that peripheral adaptations, i.e., the muscle ability to extract and use oxygen, may be a stronger predictor of canoe-kayak sprint performance compared to VO2max or central adaptations. If maximizing the time near VO2max during high-intensity interval training (HIIT) sessions is believed to optimize central adaptations, maximizing the time near maximal levels of muscle desaturation could represent a critical stimulus to optimize peripheral adaptations.
Purpose: Therefore, the purpose of this study was to assess the VO2, muscle oxygenation and cardiac output responses to various HIIT sessions, and to determine which type of HIIT elicits the lowest muscle oxygenation and the longest cumulated time at low muscle O2 saturation.
Methods: Thirteen well-trained canoe-kayak athletes performed an incremental test to determine VO2max and peak power output (PPO), and 4 HIIT sessions (HIIT-15: 40x[15 s at 115%PPO, 15 s at 30%PPO]; HIIT-30: 20x[30 s at 115%PPO, 30 s at 30%PPO]; HIIT-60: 6x[1 min at 130%PPO, 3 min rest]; sprint interval training (SIT): 6x[30 s all-out, 3 min 30 rest]) on a canoe or kayak ergometer. Portable near-infrared spectroscopy monitors were placed on the Latissimus dorsi (LD), Biceps brachii (BB), and Vastus lateralis (VL) during every session to assess changes in muscle O2 saturation (SmO2, % of physiological range).
Results: HIIT-15 and HIIT-30 elicited a longer time >90%VO2max (HIIT-15: 8.1 ± 6.2 min, HIIT-30: 6.8 ± 4.6 min), compared to SIT (1.7 ± 1.3 min, p = 0.006 and p = 0.035) but not HIIT-60 (4.1 ± 1.7 min). SIT and HIIT-60 elicited the lowest SmO2 in the VL (SIT: 0 ± 1%, HIIT-60: 8 ± 9%) compared to HIIT-15 (26 ± 12%, p < 0.001 and p = 0.007) and HIIT-30 (25 ± 12%, p < 0.001 and p = 0.030). SIT produced the longest time at >90% of maximal deoxygenation in all 3 muscles, with effect sizes ranging from small to very large.
Conclusions: Short HIIT performed on a canoe/kayak ergometer elicits the longest time near VO2max, potentially conducive to VO2max improvements, but SIT is needed in order to maximize muscle deoxygenation during training, which would potentially conduct to greater peripheral adaptations.
Introduction
High-intensity interval training (HIIT) is considered one of the most effective training for improving performance in athletes from various sports (Buchheit and Laursen, 2013a). HIIT involves alternating short (<45 s) to long (2–4 min) bouts of high-intensity (usually >85% max HR) exercise with recovery periods (Weston et al., 2014). In the early 2000s, little was known on the optimal type of HIIT program for producing the greatest improvement in endurance performance in trained athletes (Laursen and Jenkins, 2002). Since then, a lot of research has focused on understanding the acute and chronic effects of different forms of HIIT to optimize its prescription in athletes.
In 1986, Wenger and Bell (1986) published a review of the literature, where they concluded that VO2max enhancement was positively related to exercise intensity, for intensities from 50 to 100% of VO2max, and showed that the greatest improvements occurred when training was performed at an intensity between 90 and 100% of VO2max. It led several authors to suggest that training near or at VO2max is an optimal stimulus to improve VO2max (Billat, 2001). From a physiological perspective, training at or near VO2max would impose a maximal stress to the physiological processes and structures limiting VO2max and should, therefore, promote adaptations in these structures and processes. For example, it has been shown that mechanical overload, the main stimulus for morphological adaptation of the myocardium and hence enhancement of the cardiac output, is maximal when exercising at the intensity eliciting VO2max (Åstrand et al., 1964). Thus, there has been great research interest in characterizing time spent at or near (>90%) VO2max in different training protocols, to identify the training sessions that would elicit the longest time near VO2max. In a literature review published in 2006, Midgley and McNaughton (2006) detailed the training characteristics that produce the longest time at or near VO2max. They concluded that, in order to maximize time spent near VO2max, work intervals of 15–30 s should be performed at an intensity of 90–105% of the minimal speed eliciting VO2max (vVO2max), and recovery intervals of 15–30 s should be performed at an intensity between 50% of VO2max and lactate threshold.
Sprint interval training (SIT), consisting of low volume all-out supramaximal (at a greater intensity than 100% VO2max) sprints of typically 20–30 s duration, interspersed by long (≥2 min) recovery intervals has been shown to improve endurance performance in trained cyclists (Laursen et al., 2002) and runners (Esfarjani and Laursen, 2007). Although time spent at >90% VO2max during SIT sessions is low (typically 0–60 s in trained cyclists for an entire training session), it has been suggested that muscle O2 demand is high, especially as the number of sprints increases, as suggested by low muscle oxygenation levels (Buchheit et al., 2012a). Therefore, SIT appears to be an optimal training stimulus to improve endurance performance through peripheral (muscular) adaptations. But all-out SIT is associated with high neuromuscular fatigue (Buchheit and Laursen, 2013b), which could limit the ability of the athletes to perform other training sessions. It is currently unknown if supramaximal efforts of lower intensity (120–130% maximal aerobic power) but longer duration (>45 s) could elicit the same peripheral demand, while inducing less neuromuscular fatigue. If so, it would be of great interest for athletes, decreasing the likeliness of interference between HIIT and other training sessions (moderate-intensity continuous training, strength training, speed training, power training, etc.). To our knowledge, acute response to longer supramaximal interval training has not been assessed so far.
In sprint canoe-kayak, Olympic individual events are 200- and 500-m (~38 to ~120 s) for women and 200-m and 1,000-m (~34 to ~220 s) for men. While VO2max is often considered a major performance factor in longer distance sprint canoe-kayak events (Michael et al., 2008), results from a recent study suggest that peripheral adaptations (as assessed via near infrared spectroscopy (NIRS) derived changes in muscle oxygenation) may be stronger predictors of canoe-kayak performance in both short and long events (Paquette et al., 2018). In fact, since 200 and 500-m events are performed at an intensity greater than VO2max, and since canoe-kayak is an upper body dominant sport, and skeletal muscles in the arms typically display larger cross sectional area of type II muscle fibers, it makes this discipline more reliant on muscle power than cardiorespiratory fitness. Therefore, there is a need to identify training sessions that elicit a high peripheral demand in this sport.
The oncoming of affordable and portable NIRS monitors has increased accessibility to muscle oxygenation measures during exercise. Researchers have used peak VO2 and time spent >90% VO2max during HIIT sessions to assess the central demand of HIIT, and we suggest that maximal muscle deoxygenation and cumulated time at >90% maximal muscle deoxygenation are good indicators of acute peripheral demand during HIIT sessions.
The purpose of this study was to assess the VO2, muscle oxygenation, and cardiac output responses to 4 types of interval training commonly used by canoe-kayak athletes, and to determine which type of interval training elicits the lowest muscle O2 levels and the longest cumulated time at low muscle O2 saturation.
Methods
Subjects
Thirteen canoe-kayak athletes participated in this study, of which 9 were kayakers (3 women, 6 men) and 4 were canoeists (2 women, 2 men). Participants were 22 ± 3 years of age (range 19–27 years old) and weighted 71.5 ± 8.3 kg. Four of them were members of the Canadian National Team in sprint or slalom, and nine were provincial-level athletes in sprint canoe or kayak. This study was approved by the local ethics committee and was conducted in accordance to the principles established in the Declaration of Helsinki, with verbal and written informed consent provided by all participants.
Experimental Design
Athletes performed a maximal incremental test on a canoe or kayak, for the determination of maximal oxygen consumption (VO2max), maximal cardiac output (Qmax), and peak power output (PPO). Next, they completed four interval training sessions on a canoe or kayak ergometer in a randomized order to determine the acute physiological response associated with each interval training type.
Methodology
Maximal Incremental Test
All participants performed a continuous incremental VO2max testing consisting of six 2-min stages of increasing intensity on a kayak or canoe ergometer (SpeedStroke Gym, KayakPro, Florida, USA). The ergometer was calibrated before each test, according to the manufacturer recommendations and tension in the ergometer's ropes was verified regularly (Tanner and Gore, 2013). Participants received stroke-by-stroke feedback during the test and were asked to maintain a constant intensity that would elicit effort perception of 2, 4, 5, 6, 8, and 10/10 during stages 1–6, respectively. Stroke rate ranges were given for the first 5 stages for canoe (30–35; 35–40; 40–45; 45–50; 50–55 strokes per min) and kayak (60–65; 65–75; 75–85; 85–95; 95–105 strokes per min) to help athletes select the right intensity in each stage. Power output (PO) was recorded on a computer, using the eMonitorPro2 software (KayakPro, Florida, USA).
Interval Training Sessions
The athletes completed four different HIIT sessions on the canoe or kayak ergometer in a randomized order. There was always more than 24 h and less than a week between HIIT sessions and athletes must refrain from doing any intense physical activity for 24 h before the training session. Training sessions are described in Table 1. Athletes were asked to rate their level of exertion and their level of enjoyment on a scale of 1 to 10 at the end of every HIIT session.
VO2 and Cardiac Output
During all sessions, expired air was continuously recorded using a breath-by-breath gas analyzer (Vmax Encore metabolic cart, CareFusion Corp, California, USA). PPO was the average power output on the last 2-min stage of the maximal incremental test. Heart rate (HR) and cardiac output (Q) were evaluated during all tests using thoracic electrical bioimpedance (Physioflow, Manatec Biomedical, France). All devices were calibrated according to manufacturer guidelines before every test. Arteriovenous O2 difference [(a-v)O2-diff (ml/dl)] was calculated using the following equation: (a-v)O2-diff = VO2 (L/min)/Q (L/min)*100 and stroke volume (SV) was calculated from Q and HR. VO2max, maximal Q (Qmax), maximal SV (SVmax), and maximal (a-v)O2-diff were defined as the highest values achieved over a 30-s period during the incremental test. Time over 90 and 95% of VO2max, Qmax, and HRmax were calculated for all sessions. Peak VO2, peak Q and peak HR were also calculated for each session, and defined as the highest 30-s average for VO2 and Q and highest 5-s average for HR value reached during the session.
Muscle Oxygenation
During all tests, Moxy NIRS monitors (Fortiori Design, Minnesota, USA) were placed on three active muscles: latissimus dorsi (LD)—midpoint between the inferior border of the scapula and posterior axillar fold (Borges et al., 2015) –, biceps brachii (BB)—middle of the BB muscle belly (8–12 cm above the elbow fold)—and vastus lateralis (VL)—distal part of the VL muscle belly (10–15 cm above the proximal border of the patella) (Billaut and Buchheit, 2013). NIRS monitors were placed on the athlete's dominant side for kayakers, and on the front leg and opposite BB and LD for canoeists, parallel to the muscle fiber orientation. They were attached and secured with a double-sided adhesive disk and an adhesive patch, and covered by a dark bandage to reduce the intrusion of extraneous light. The Moxy monitors position was marked on the athlete's skin to ensure the monitors were placed on the same site in every testing session. Skinfold thickness at each site was measured using a skinfold caliper (Harpenden Ltd) to ensure that the skinfold thickness was less than half the distance between the emitter and the detector (25 mm). The raw muscle O2 saturation (SmO2) signal was treated using a smooth spline filter to reduce the noise created by movement (Rodriguez et al., 2018). During exercise, SmO2 represents the balance between O2 delivery and extraction by the muscle (Ferrari et al., 2011). Minimum and maximum SmO2 were the absolute lowest and highest 5-s average SmO2 reached during any of the HIIT session. Minimum and maximum SmO2 values were determined from the lowest and highest observed values throughout the entire experiment for each subject. All SmO2 values were then normalized, so that 0 and 100% represent these minimum and maximum SmO2 of the participant, respectively. SmO2 values are presented in these normalized values in the results section and minimum SmO2 will be referred as deoxy max thereafter. As for VO2max and cardiac output values, time over 90 and 95% of deoxy max were calculated for all HIIT sessions and defined as the time spent at a SmO2 value of <10% and <5% in this normalized scale. Time spent above 90 and 95% deoxy max were determined during both work and rest intervals to obtain a complete picture of peripheral metabolic disturbances during the given sessions. Peak muscle deoxygenation (peak deoxy) was calculated for each muscle during each session, and was defined as the lowest 5-s average SmO2 reached during the session.
Statistical Analysis
Means and standard variations were calculated for physiological parameters, and ANOVAs with Tukey post-hoc tests were used to assess differences between groups. Cohen's d effect sizes (ES) and 90% confidence intervals were also computed for differences in means between sessions and ES of 0.2, 0.6, 1.2, and 2.0 were considered small, moderate, large, and very large differences, respectively. For the SmO2 data, a robust smallest worthwhile change (SWC) anchor is not evident, therefore the SWC was calculated using the standardized mean difference of 0.2 between subject standard deviations (SD).
Results
Athlete's Characteristics
Participants had a VO2max of 51.8 ± 7.7 ml/kg/min, or 3.7 ± 0.8 L/min, a maximal cardiac output of 25.4 ± 3.5 L/min, a maximal SV of 147 ± 24 ml/beat, a maximal (a-v)O2-diff of 14.5 ± 2.0 ml/dl and a maximal heart rate of 194 ± 5 bpm. Their PPO during the incremental test was 122 ± 36 W, or 1.69 ± 0.45 W/kg.
Cardiorespiratory and Subjective Responses
Figure 1 displays the average cardiorespiratory parameters at the end of each work and rest interval for the four training sessions. Table 2 shows the power output maintained, and the VO2 and cardiac output responses during each HIIT session. The highest RPE was reached in the SIT session, but it did not differ statistically from the other sessions RPE ratings. VO2peak was greater in HIIT-60 compared to HIIT-15 (ES 1.34 [0.62, 2.08], p = 0.02), HIIT-30 (ES 1.41 [0.70, 2.14], p = 0.01), and SIT (ES 2.32 [1.49, 3.16], p < 0.01). Peak Q was not different between sessions. Time spent >90% VO2max was higher in HIIT-15 (ES 1.71 [0.94,2.48], p < 0.01) and HIIT-30 (ES 1.74 [0.98,2.50], p < 0.01) compared to SIT. HIIT-15 and HIIT-30 also elicited a greater cumulated time at >90% Qmax compared to SIT (HIIT-15: ES 1.35 [0.46, 2.25], p = 0.04, HIIT-30: ES 1.09 [0.30, 1.88], p = 0.02) and greater cumulated time >90% HRmax (HIIT-15: ES 6.39 [4.46, 8.31], p < 0.01, HIIT-30: ES 4.19 [2.91, 5.47], p < 0.01).
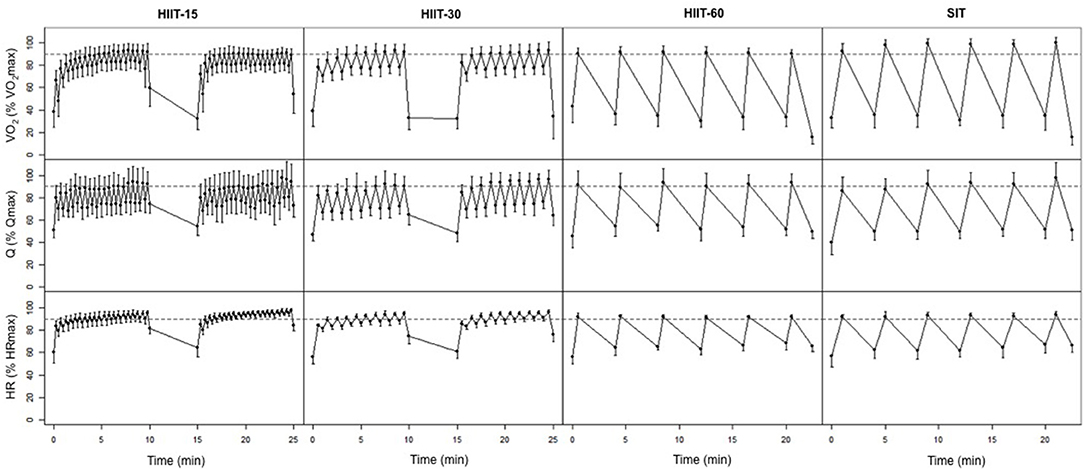
Figure 1. Average VO2, Q, and HR at the end of each work and rest interval during the four interval sessions. Horizontal dashed lines represent 90% of VO2max, Qmax and HRmax, respectively.
Muscle Oxygenation Response
Figure 2 displays the average SmO2 at the end of each work and rest interval for the four training sessions. Table 3 details the muscle oxygenation response to the 4 HIIT protocols. BB deoxy max was greater in SIT compared to HIIT-15 (ES −1.83 [−1.06, −2.59], p = 0.02) and HIIT-30 (ES −1.63 [−0.89, −2.38], p = 0.01) and LD deoxy max was greater in SIT compared to HIIT-15 (ES −1.24 [−0.54, −1.95], p = 0.04). VL deoxy max was greater in both SIT and HIIT-60 compared to HIIT-15 (SIT: ES 3.86 [−2.77, −4.95], p < 0.01, HIIT-60: ES 1.79 [−1.03, −2.55], p < 0.01) and HIIT-30 (SIT: ES −3.76 [−2.87, −4.83], p < 0.01, HIIT-60: ES −1.70 [−0.95, −2.45], p < 0.01). For all 3 investigated muscles, SIT resulted in the longest time spent near maximal muscle deoxygenation compared to session HIIT-15. Specifically in the VL, SIT resulted in the longest time spent at >90 and 95% maximal VL deoxygenation compared to HIIT-15 and HIIT-30, and to a smaller extent to HIIT-60.
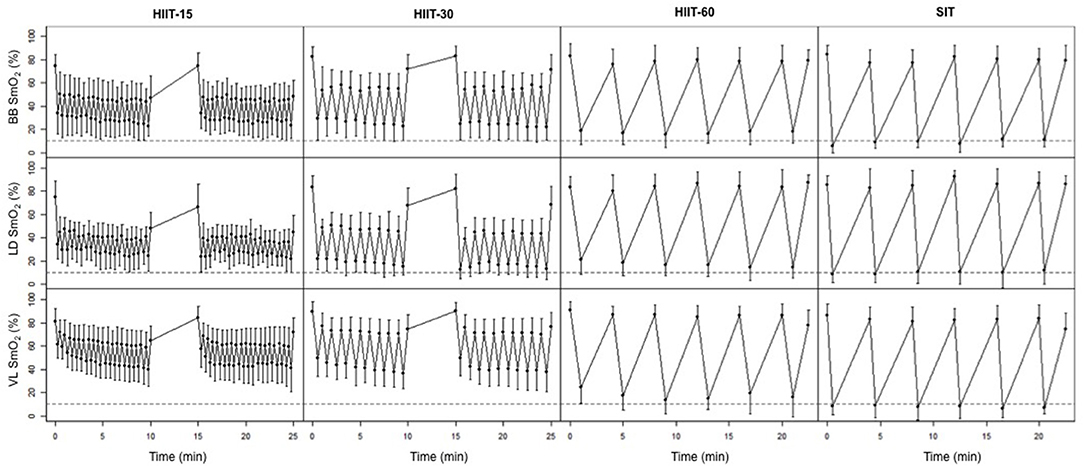
Figure 2. Average SmO2 in the BB, LD, and VL at the end of each work and rest interval during the four interval sessions. Horizontal dashed lines represent 10% SmO2 (90% of maximal deoxygenation).
Figure 3 shows the effect sizes and 90% confidence interval of differences in means between the different sessions for the time spent near maximal muscle deoxygenation. Clear very large differences were found for VL between sessions HIIT-15 and SIT and between sessions HIIT-30 and SIT, and clear large differences were found for VL between sessions HIIT-15 and HIIT-60, HIIT-30 and HIIT-60 and between SIT and HIIT-60 and for BB between sessions HIIT-15 and SIT. Moderate differences were found for BB between HIIT-15 and HIIT-60 and HIIT-30 and HIIT-60. Figure 4 shows the individual response for the time spent near maximal deoxygenation in each HIIT session, for the canoers and the kayakers.
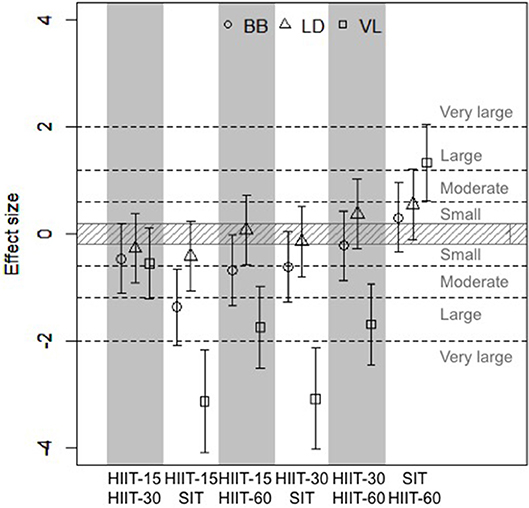
Figure 3. Effect sizes and 90% confidence interval of differences in means between cumulated time spent >90% maximal deoxygenation in the three studied muscles. Positive effect size reflects a greater time spent >90% deoxy max in the first session compared to the second, while negative effect size reflects a smaller time spent >90% deoxy max in the first session compared to the second.
Difference Between Muscles
Time spent >90% deoxy max was lower in the VL compared to the LD (ES −2.38 [−3.28, −1.48], p = 0.04) and tended to be lower compared to BB (ES −1.20 [−1.90, −0.50], p = 0.07) in HIIT-30 session. Peak deoxy values were higher in VL compared to BB and LD for both sessions HIIT-15 and HIIT-30 (all p < 0.01). There was no difference between investigated muscles for time spent >90% deoxy max or peak deoxy in HIIT-60 and SIT sessions.
Discussion
Physiological responses to 4 different HIIT sessions were assessed in well-trained canoe-kayak athletes and the most important findings were: (1) short interval training sessions (HIIT-15 and HIIT-30) elicit the most time at high levels of VO2, Q and HR, but did not elicit high or sustained levels of muscle deoxygenation; (2) SIT does not elicit much time at high levels of VO2, Q or HR, but elicit the highest levels of muscle deoxygenation and the most time spent at high levels of muscle deoxygenation; (3) long supramaximal interval training (HIIT-60) is an hybrid between short intervals and SIT, eliciting higher muscle deoxygenation than short intervals in the VL, and higher VO2peak than SIT.
HIIT and Central Demand
With no surprise, the short interval sessions elicited the highest time >90% VO2max compared to the other interval sessions. For short intervals, the ratio of time >90% VO2max on total exercise time has been shown to be ~30% (Buchheit and Laursen, 2013a), which is in line with our results (41% for HIIT-15 and 34% for HIIT-30). The lower ratio for HIIT-30 compared to HIIT-15 in our study might be explained by the relatively low intensity of recovery intervals (~30% PPO), which has been associated with reduced time >90% VO2max when recovery intervals are >20 s (Buchheit and Laursen, 2013a). The intensity of the recovery intervals was lower than what is usually recommended in short interval sessions, but was the highest intensity the athletes could practically maintain between work intervals, since the resistance from the ergometer elastics creates a high effort perception, even at low workloads. However, the lower intensity in the recovery intervals was compensated by a slightly higher intensity in the work intervals (~115% PPO).
It has been suggested that about 10 min must be spent around VO2max in order to elicit important cardiovascular adaptations in endurance athletes (Buchheit and Laursen, 2013a). With 8.1 min and 6.8 min, HIIT-15 and HIIT-30 sessions both come near this recommendation. Since RPE was not maximal at the end of the sessions (~8/10), we can speculate that a third set could have been performed, which would have brought the time >90% VO2max around 10–12 min.
In the SIT session, athletes reached 87 ± 5% of their VO2max and maintained 1.7 ± 1.3 min >90% VO2max. These results are in line with a study where trained cyclists performing 6 all-out 30-s sprints, interspersed by 2 min of passive recovery, reached 90 ± 3% VO2max and spent 22 ± 21 s (range 0–60 s) >90% VO2max (Buchheit et al., 2012a). Since time spent >90% VO2max is inversely related to subjects' VO2max (Buchheit et al., 2012a), the slightly higher time spent >90% VO2max in our study could be due to the generally lower VO2max achieved on kayaking compared to cycling exercise (Michael et al., 2008).
The highest VO2 values were reached during the long supramaximal interval session (HIIT-60), but athletes only cumulated 4.1 ± 1.7 min >90% VO2max during that session, which still represents a high percentage of the 6 × 1-min work intervals. The higher intensity of the session, accelerating VO2 kinetics (Hughson et al., 2000), coupled with the longer effort duration allowed for a high VO2 to be reached from the first effort bout. More repetitions would have been needed to accumulate more time >90% VO2max, but it would likely not be possible to reach 10 min with this session due to its difficulty. It was suggested that spending 5–7 min at >90% VO2max would likely be sufficient for team-sports athletes or for maintenance in endurance athletes. Therefore, such a session could be planned for sprint kayakers wishing to develop 500-m specific speed while maintaining VO2max.
Hence, as pointed out by other authors (Midgley and McNaughton, 2006; Buchheit and Laursen, 2013a), short intervals are effective for accumulating training time near VO2max and would therefore be a good choice when the goal is to increase VO2max.
HIIT and Peripheral Demand
It has been suggested that repeated fluctuations of muscular O2 consumption during training sessions is necessary for muscular oxidative capacity adaptations (Daussin et al., 2008a,b), and that low O2 partial pressure at the muscle level is needed to induce mitochondrial biogenesis (Hoppeler et al., 2003). Hence, training sessions that elicit repeated high levels of muscle deoxygenation might present an adequate stimulus to foster peripheral adaptations. Understanding the degree of muscle deoxygenation that occurs during training is therefore important, as it is likely that localized O2 availability and extraction triggers the cascade of signals responsible for the marked metabolic adaptations witnessed after high-intensity exercise (Coffey and Hawley, 2007). Muscle deoxygenation during both effort and rest periods were considered in this study, to obtain a complete picture of peripheral metabolic disturbances during the given sessions.
The short interval sessions were the ones eliciting the least deoxygenation and the lowest time spent at high levels of muscle deoxygenation. HIIT-60 elicited a higher muscle deoxygenation than short interval sessions in the VL, but did not elicit a greater time at high deoxygenation levels. SIT was the most effective session to elicit both high and sustained levels of muscle deoxygenation. This is in line with Buchheit et al. (2012a) study, showing muscle deoxygenation during six 30-s all-out efforts to be similar to values observed during maximal voluntary leg contractions or occlusion, known to produce near-to-maximal muscle desaturation. Our results also suggest that effort bout intensity is the most important factor determining the time spent at high levels of muscle deoxygenation. This is in line with results from Stöcker et al. (2015), where a higher cycling intensity was associated with greater muscle deoxygenation. The duration of the work intervals would also have an impact on the degree of muscle deoxygenation. For example, repeated 4-s sprints would reduce SmO2 by about 12% (Buchheit et al., 2012b), compared to a reported reduction of 27% for longer sprints (30 s) (Buchheit et al., 2012a).
NIRS SmO2 value represents the balance between O2 delivery and extraction within the muscle (Ferrari et al., 2011). Thus, a decreased SmO2 value could be due to both decreased O2 delivery and/or increased O2 extraction by the muscle. In supramaximal efforts, it is likely that strong muscle contraction limit O2 delivery (Bhambhani, 2004), as suggested by decreased total hemoglobin concentration during SIT (Jones et al., 2015). But deoxyhemoglobin concentration also increased during SIT, suggesting that an increase in muscle O2 extraction also occurs (Jones et al., 2015). We chose to only present SmO2 here, since recent studies have found that the Moxy monitor provides credible and reliable SmO2 values (McManus et al., 2018), but that total hemoglobin values had low variation during exercise and were probably not a valid indicator of blood volume (Crum et al., 2017).
Taken together, these results suggest that SIT elicits a high muscle O2 demand, and while the optimal amount of time spent at high muscle deoxygenation in training is not known, it appears that SIT would be an ideal training session to elicit peripheral adaptations. These findings are in line with previous studies showing various peripheral adaptations following SIT (Gibala and McGee, 2008) and a study where muscle deoxygenation was increased in hockey players during SIT following 6 sessions of cycling SIT, potentially indicative of an increase muscle O2 extraction capacity with SIT (Jones et al., 2015).
We suggested that HIIT-60 session would represent a good stimulus to improve peripheral adaptations without requiring a maximal effort from the athletes. However, we found that even though maximal deoxygenation was not different from SIT session, time spent at high deoxygenation levels tended to be lower compared to SIT. This is explained by the fact that maximal deoxygenation was only reached near the end of the 1-min work intervals at 130% PPO. We could hypothesize that increasing the intensity during the first 10–15 s of the HIIT-60 work intervals could induce a faster deoxygenation and potentially higher time spent near maximal deoxygenation. Also, HIIT-60 allowed for greater time spent at high deoxygenation level compared to sessions HIIT-15 and HIIT-30 only for the VL, and therefore would be a good hybrid between high central demand and high peripheral demand sessions only for this muscle.
Upper-body muscles deoxygenated to a greater extent in short interval sessions compared to the lower-body muscle investigated. These differences between muscle groups may be explained by a difference in contribution of these muscles. It is possible that upper-body muscles contribute more compared to lower-body muscles to the effort; however, this difference seems to dissipate at higher intensities (>130% PPO). This difference may also come from the lower oxidative capacity of upper body motor units compared to leg muscles (Bhambhani et al., 1998).
We hypothesized that SIT would be an optimal stimulus for peripheral adaptations, but that it would be associated with a higher effort perception. However, while RPE rating was higher following the SIT session compared to the other HIIT sessions, the difference did not reach statistical significance (p = 0.08). Session enjoyment was thus similar in all 4 HIIT sessions, suggesting athletes' compliance would not be an issue for any of these sessions (Scanlan et al., 1993). More research is needed to understand the effect of HIIT type on fatigue and recovery.
Even though interval sessions were not matched for interval duration (effort duration varied from 180 to 600 s between sessions), central and peripheral responses were compared between sessions using absolute time-derived variables. Indeed, training sessions were not matched by duration or energy expenditure, but by effort, which is how coaches prescribe training sessions in the field. So if physiological adaptations are driven by the time spent in a given acute physiological state, we believe using typical training sessions and looking at absolute time-derived variables is the best approach in an applied sport research context. Mixing canoeing and kayaking in this study is a potential limitation, given that muscle activity differs between disciplines.
Practical Applications
The present study highlights the different acute physiological responses to various types of HIIT. Coaches and athletes who wish to improve VO2max through central adaptations should include short intervals to their program. In canoe-kayak, and likely in other upper-body dominant sports, peripheral adaptations are associated with performance and, therefore, SIT sessions targeting the muscle oxidative adaptations should also be included in the training program. Longer supramaximal intervals could be used to train race specific speed while still stimulating VO2max, but should probably be modified in order to really target peripheral adaptations.
Conclusions
Time spent near VO2max is high during short-interval HIIT sessions performed on a canoe or kayak ergometer. However, all-out 30-s sprints are required to elicit high and sustained levels of muscle deoxygenation. These findings regarding the acute physiological changes associated with different types of HIIT give insights on potential physiological adaptations following different HIIT protocols. Future studies should assess the chronic effect of HIIT and SIT sessions to ascertain whether time spent near VO2max and time spent near maximal muscle deoxygenation are important determinants of VO2max and maximal deoxygenation adaptations, respectively, and performance in canoe-kayak athletes.
Data Availability
The datasets generated for this study are available on request to the corresponding author.
Ethics Statement
This study was carried out in accordance with the recommendations of Ethics Committee for Health Sciences research of Laval University with written informed consent from all subjects. All subjects gave written informed consent in accordance with the Declaration of Helsinki. The protocol was approved by the Ethics Committee for Health Sciences research of Laval University.
Author Contributions
All authors listed have made a substantial, direct and intellectual contribution to the work, and approved it for publication.
Funding
This research received fundings from the Institut National du Sport du Québec, including funds for open access publication fees.
Conflict of Interest Statement
The authors declare that the research was conducted in the absence of any commercial or financial relationships that could be construed as a potential conflict of interest.
Acknowledgments
Preliminary results from this study were presented at the European College of Sport Science hosted in Dublin, Ireland in 2018 (MP, FB, FB muscle oxygenation response to high-intensity interval training in sprint canoe-kayak. 23rd annual Congress of the European College of Sport Science, Dublin, Ireland, 2018).
References
Åstrand, P., Cuddy, T., Saltin, B., and Stenberg, J. (1964). Cardiac output during submaximal and maximal work. J. Appl. Physiol. 19, 268–274. doi: 10.1152/jappl.1964.19.2.268
Bhambhani, Y., Maikala, R., and Buckley, S. (1998). Muscle oxygenation during incremental arm and leg exercise in men and women. Eur. J. Appl. Physiol. Occup. Physiol. 78, 422–431. doi: 10.1007/s004210050441
Bhambhani, Y. N. (2004). Muscle oxygenation trends during dynamic exercise measured by near infrared spectroscopy. Can. J. Appl. Physiol. 29, 504–523. doi: 10.1139/h04-033
Billat, V. (2001). Interval training for performance: a scientific and Empirical Practice. Special recommendations for middle- and long-distance running. Part II: anaerobic interval training. Sports Med. 31, 75–90. doi: 10.2165/00007256-200131020-00001
Billaut, F., and Buchheit, M. (2013). Repeated-sprint performance and vastus lateralis oxygenation: effect of limited O(2) availability. Scand. J. Med. Sci. Sports. 23, e185–193. doi: 10.1111/sms.12052
Borges, T. O., Dascombe, B., Bullock, N., and Coutts, A. J. (2015). Physiological characteristics of well-trained junior sprint kayak athletes. Int. J. Sports Physiol. Perform. 10, 593–599. doi: 10.1123/ijspp.2014-0292
Buchheit, M., Abbiss, C. R., Peiffer, J. J., and Laursen, P. B. (2012a). Performance and physiological responses during a sprint interval training session: relationships with muscle oxygenation and pulmonary oxygen uptake kinetics. Eur. J. Appl. Physiol. 112, 767–779. doi: 10.1007/s00421-011-2021-1
Buchheit, M., Hader, K., and Mendez-Villanueva, A. (2012b). Tolerance to high-intensity intermittent running exercise: do oxygen uptake kinetics really matter? Front. Physiol. 3:406. doi: 10.3389/fphys.2012.00406
Buchheit, M., and Laursen, P. B. (2013a). High-intensity interval training, solutions to the programming puzzle: Part I: cardiopulmonary emphasis. Sports Med. 43, 313–338. doi: 10.1007/s40279-013-0029-x
Buchheit, M., and Laursen, P. B. (2013b). High-intensity interval training, solutions to the programming puzzle. Part II: anaerobic energy, neuromuscular load and practical applications. Sports Med. 43, 927–954. doi: 10.1007/s40279-013-0066-5
Coffey, V. G., and Hawley, J. A. (2007). The molecular bases of training adaptation. Sports Med. 37, 737–763. doi: 10.2165/00007256-200737090-00001
Crum, E. M., O'Connor, W. J., Van Loo, L., Valckx, M., and Stannard, S. R. (2017). Validity and reliability of the Moxy oxygen monitor during incremental cycling exercise. Eur. J. Sport Sci. 2017, 1–7. doi: 10.1080/17461391.2017.1330899
Daussin, F. N., Zoll, J., Dufour, S. P., Ponsot, E., Lonsdorfer-Wolf, E., Doutreleau, S., et al. (2008a) Effect of interval versus continuous training on cardiorespiratory and mitochondrial functions: relationship to aerobic performance improvements in sedentary subjects. Am. J. Physiol. Regulat. Integr. Comparat. Physiol. 295, R264–R272. doi: 10.1152/ajpregu.00875.2007
Daussin, F. N., Zoll, J., Ponsot, E., Dufour, S. P., Doutreleau, S., Lonsdorfer, E., et al. (2008b). Training at high exercise intensity promotes qualitative adaptations of mitochondrial function in human skeletal muscle. J. Appl. Physiol. 104, 1436–1441. doi: 10.1152/japplphysiol.01135.2007
Esfarjani, F., and Laursen, P. B. (2007). Manipulating high-intensity interval training: effects on VO2max, the lactate threshold and 3000 m running performance in moderately trained males. J. Sci. Med. Sport. 10, 27–35. doi: 10.1016/j.jsams.2006.05.014
Ferrari, M., Muthalib, M., and Quaresima, V. (2011). The use of near-infrared spectroscopy in understanding skeletal muscle physiology: recent developments. Philosoph Transact. Seri. Mathemat. Phys. Eng. Sci. 369, 4577–4590. doi: 10.1098/rsta.2011.0230
Gibala, M. J., and McGee, S. L. (2008). Metabolic adaptations to short-term highintensity interval training: a little pain for a lot of gain? Exerc. Sport Sci. Rev. 36:58–63. doi: 10.1097/JES.0b013e318168ec1f
Hoppeler, H., Vogt, M., Weibel, E. R., and Flück, M. (2003). Response of skeletal muscle mitochondria to hypoxia. Exp. Physiol. 88, 109–119. doi: 10.1113/eph8802513
Hughson, R. L., O'Leary, D. D., Betik, A. C., and Hebestreit, H. (2000). Kinetics of oxygen uptake at the onset of exercise near or above peak oxygen uptake. J. Appl. Physiol. 88, 1812–1818. doi: 10.1152/jappl.2000.88.5.1812
Jones, B., Hamilton, D. K., and Cooper, C. E. (2015). Muscle oxygen changes following sprint interval cycling training in elite field hockey players. PLoS ONE 10:e0120338. doi: 10.1371/journal.pone.0120338
Laursen, P. B., and Jenkins, D. G. (2002). The scientific basis for high-intensity interval training: optimising training programmes and maximising performance in highly trained endurance athletes. Sports Med. 32, 53–73. doi: 10.2165/00007256-200232010-00003
Laursen, P. B., Shing, C. M., Peake, J. M., Coombes, J. S., and Jenkins, D. G. (2002). Interval training program optimization in highly trained endurance cyclists. Med. Sci. Sports Exer. 34, 1801–1807. doi: 10.1097/00005768-200211000-00017
McManus, C. J., Collison, J., and Cooper, C. E. (2018). Performance comparison of the MOXY and PortaMon near-infrared spectroscopy muscle oximeters at rest and during exercise. J. Biomed. Opt. 23, 1–14. doi: 10.1117/1.JBO.23.1.015007
Michael, J. S., Ronney, K. B., and Smith, R. (2008). The metabolic demands of kayaking: a review. J. Sports Sci. Med. 7, 1–7.
Midgley, A. W., and McNaughton, L. R. (2006). Time at or near VO2max during continuous and intermittent running: a review with special reference to considerations for the optimisation of training protocols to elicit the longest time at or near VO2max. J. Sports Med. Phys. Fitness. 46, 1–14.
Paquette, M., Bieuzen, F., and Billaut, F. (2018). Muscle oxygenation rather than VO2max as a strong predictor of performance in sprint canoe-kayak. Int. J. Sports Physiol. Perform. 2018:1–9. doi: 10.1123/ijspp.2018-0077
Rodriguez, R. F., Townsend, N. E., Aughey, R. J., and Billaut, F. (2018). Influence of averaging method on muscle deoxygenation interpretation during repeated-sprint exercise. Scand. J. Med. Sci. Sports 28, 2263–2271. doi: 10.1111/sms.13238
Scanlan, T. K., Carpenter, P. J., Lobel, M., and Simons, P. (1993). Sources of enjoyment for youth sport athletes. Pediatr. Exer. Sci. 5, 275–285. doi: 10.1123/pes.5.3.275
Stöcker, F., Von Oldershausen, C., Paternoster, F. K., Schulz, T., and Oberhoffer, R. (2015). End-exercise ΔHHb/ΔVO2 and post-exercise local oxygen availability in relation to exercise intensity. Clin. Physiol. Funct. Imaging. 37, 384–393. doi: 10.1111/cpf.12314
Tanner, R., and Gore, C. (2013). Physiological Tests for Elite Athletes. 2 Edn. Canberra: Australian Institute of Sport (AIS).
Wenger, H. A., and Bell, G. J. (1986). The interactions of intensity, frequency and duration of exercise training in altering cardiorespiratory fitness. Sports Med. 3, 346–356. doi: 10.2165/00007256-198603050-00004
Keywords: oxygen saturation, peripheral adaptations, aerobic fitness, sprint kayak, sprint canoe, interval training
Citation: Paquette M, Bieuzen F and Billaut F (2019) Sustained Muscle Deoxygenation vs. Sustained High VO2 During High-Intensity Interval Training in Sprint Canoe-Kayak. Front. Sports Act. Living 1:6. doi: 10.3389/fspor.2019.00006
Received: 16 April 2019; Accepted: 12 July 2019;
Published: 31 July 2019.
Edited by:
Franck Brocherie, Institut national du sport, de l'expertise et de la performance, FranceReviewed by:
Mohammed Ihsan, Aspetar Hospital, QatarBen Jones, University of Essex, United Kingdom
Stylianos N. Kounalakis, Evelpidon Military Academy, Greece
Copyright © 2019 Paquette, Bieuzen and Billaut. This is an open-access article distributed under the terms of the Creative Commons Attribution License (CC BY). The use, distribution or reproduction in other forums is permitted, provided the original author(s) and the copyright owner(s) are credited and that the original publication in this journal is cited, in accordance with accepted academic practice. No use, distribution or reproduction is permitted which does not comply with these terms.
*Correspondence: Myriam Paquette, bXlyaWFtLnBhcXVldHRlLjImI3gwMDA0MDt1bGF2YWwuY2E=