- Department of Chemistry and Biochemistry, University of Mississippi, Oxford, MS, United States
One of the most abundant Al-containing molecules detected in the interstellar medium (ISM) is AlOH. Over the past several years, there have been various pathways proposed for the formation of AlOH in the ISM, including reactions between AlO and H2 or H2O. However, these pathways include an energetic barrier from a transition state that likely prevents the reaction from progressing efficiently in the low temperature/low pressure environment of the ISM. Recently, a barrierless pathway for formation of AlOH from AlO and AlH has been proposed for the formation of AlOH. Even so, only one of these species really needs to contain an aluminum atom. To account for this, alternative but related pathways reacting the known interstellar molecule AlO with XH and AlH with XO (X = Mg, Si, P, or S) to form AlOH are explored with high accuracy quantum chemical calculations via CCSD(T)-F12b/cc-pVTZ-F12. Each third row element has at least one pair of reactants that lead to exothermic formation of AlOH. These reactions can go on to form other aluminum oxides and aluminum oxide clusters that may, in part, lead to the formation of interstellar dust grains.
1 Introduction
Despite aluminum being the 11th most abundant element in the solar system (Asplund et al., 2009), aluminum levels in the interstellar medium (ISM) are still typically so low that this atom is not even considered “abundant” (Costantini et al., 2019). As aluminum-containing molecules are believed to be some of the first to form in circumstellar envelopes (CSEs) of AGB stars; a common hypothesis is that these aluminum molecules form corundum (Al2O3) and then larger complex dust grains (Clayton, 1978; Costantini et al., 2019). Aluminum being locked in dust grains would account for the low presence of gas-phase aluminum in the ISM. A possible starting material for corundum is AlOH. Current data shows AlOH to be one of the most abundant aluminum species in the CSEs of Al-rich AGB stars. However, the relative abundance of AlOH greatly depends on which region is being observed (Tenenbaum and Ziurys, 2010; Decin et al., 2017; Takigawa et al., 2017; Kamiński et al., 2016). The relative abundance of AlOH tends to be inversely related to the abundance of AlO, leading to the theory of AlO being involved in the formation of AlOH (Parnis et al., 1989; Sharipov et al., 2011; Mangan et al., 2021; Firth et al., 2024). AlO has been found in the CSEs of several O-rich AGB stars (Decin et al., 2017; Tachibana et al., 2019; Karovicova et al., 2013; Takigawa et al., 2017; Danilovich et al., 2020), suggesting the CSEs of these stars as a viable location for the initial steps of dust grain nucleation. In addition to AGB stars, AlO is also well known in cool (Kamiński et al., 2013) and variable stars (Banerjee et al., 2003; Tylenda et al., 2005; Banerjee et al., 2012; Kamiński et al., 2016) as well as in sunspots (Sriramachandran et al., 2013). AlO has also been tentatively detected in the atmospheres of several exoplanet atmospheres (Bowesman et al., 2021) including the hot Jupiter exoplanets WASP-33b (von Essen et al., 2019), WASP-43b (Chubb et al., 2020), HAT-P-41n (Lewis et al., 2020; Sheppard et al., 2021), and the sub-Saturn KELT-11b (Colón et al., 2020). A recent proposed pathway of formation for AlOH from the reaction of AlO and AlH, shows a fully exothermic reaction with an Al atom as a leaving group (Firth et al., 2024). While this pathway is an improvement over prior methods of forming AlOH that include energetic barriers along the pathway (Parnis et al., 1989; Sharipov et al., 2011; Mangan et al., 2021), it heavily depends on limited quantities of aluminum as it requires two aluminum-containing species to produce a molecule containing only one aluminum atom. To remedy this, variations on this reaction are explored following the form of Equations 1, 2 below, where X
Again, where X
Of the alternate metals explored in this work, magnesium is the most common at the 8th most abundant element in the Universe (Rogantini et al., 2020). Despite this, the diatomic hydrides and oxides (MgH and MgO) have limited instances of detection. Optical spectra of bright mid-L dwarf stars obtained with the W. M. Keck Observatory show the presence of MgH as well as other metal hydrides and oxides (Kirkpatrick, 2005). Submillimeter observations of IRC + 10216 also show weak evidence of the MgH line (Avery et al., 1994). Recent work has also shown the possible existence of MgH in the atmosphere of the hot Jupiter HAT-P-41b (Jiang et al., 2024). Lastly, MgH has been see in sunspot spectra (Sotirovski, 1972) which suggests it could still be present in other stars (Yurchenko et al., 2017). MgO has also been observed in spectra of sunspots (Sotirovski, 1972), but there have been confirmed nondetections of MgO in IK Tau (Decin et al., 2018), R Dor (Decin et al., 2018), and a series of other O-rich AGB stars (Wallström et al., 2024) Both MgO and MgH are also considered to be important contenders for the molecular makeup of the atmospheres of hot super-Earth exoplanets (Tennyson and Yurchenko, 2017).
While silicon is less abundant than magnesium in the universe as the 9th most abundant element (Rogantini et al., 2020), there are still several instances of detection for SiH and SiO. Even though the diatomic hydride (SiH) has reported nondetections in IRC + 10216 (Siebert et al., 2020) and VY Canis Majoris (Siebert et al., 2020), there are tentative detections in both Orion-KL with use of the Caltech Submillimeter Telescope (Schilke et al., 2001) and in the solar disk spectrum (Sauval, 1969). SiH has also been found in the sun (Wöhl, 1971), implying it could still be present in other stars (Yurchenko et al., 2017). In contrast with SiH, SiO has been detected in a multitude of sources. SiO was initially detected in Sagittarius B2 (Wilson et al., 1971; Dickinson, 1972). Since the initial detection, SiO has also been detected toward diffuse interstellar molecular clouds (Lucas and Liszt, 2000; Turner, 1998), IRC + 10216 (Prieto et al., 2015), and in the CSEs of many O-rich AGB stars (Massalkhi et al., 2020). SiO is also theorized to be important in hot super-Earth exoplanet atmospheres (Tennyson and Yurchenko, 2017).
Phosphorus and sulfur are less abundant than magnesium, silicon, and aluminum. However, phosphorus is still one of the 20 most abundant elements in the solar photosphere (Caffau et al., 2011) and the known gaseous forms of sulfur only make up <1% of the elemental sulfur in the ISM (Kama et al., 2019; Costantini et al., 2019). Phosphorous hydride (PH) has not yet been detected, but PH is still theorized to exist. Reported nondetections of PH include observations of IRC + 10216 and VY Canis Majoris (Siebert et al., 2020). In contrast with PH, PO has been detected in several sources. PO Has been detected in the star-forming region L1157-B1 (Lefloch et al., 2016), the CSE of several O-rich AGB stars such as IK Tau (Ziurys et al., 2018; De Beck et al., 2013), R Cas (Ziurys et al., 2018), and TX Cam (Ziurys et al., 2018). The supergiant NML Cyg (Ziurys et al., 2018) has also shown signatures of the presence of PO. Additional studies on PO and other phosphorus-bearing molecules agree with the theory that phosphorus appears to be extremely depleted in inactive molecular gas by multiple orders of magnitude (Lefloch et al., 2016; Fontani et al., 2016; Rivilla et al., 2016). Sulfur has been detected in dust near C-rich AGB stars, planetary nebulae (Hony et al., 2002), and protoplanetary disks (Keller et al., 2002), primarily in the form of FeS (Costantini et al., 2019; Westphal et al., 2014). Other forms of sulfur have also been found in lower abundances. The diatomic hydride (SH) has been detected toward the submillimeter source W49N with SOFIA (Neufeld et al., 2012), while the oxide (SO) has been found in Orion KL as well as the CSEs of several O-rich AGB stars (Massalkhi et al., 2020). SO is also a contender for the molecular composition of atmospheres of hot super-Earth atmospheres (Tennyson and Yurchenko, 2017). Several other sulfur-containing molecules are also known (McGuire, 2018; McGuire, 2021), and this element has even been the subject of discussion for the so-called “missing sulfur” problem (Henry et al., 2004).
Beyond their abundances, chemical reactions in the inter- and circumstellar media have more limitations to be considered viable compared to reactions on Earth. For instance, bimolecular reactions become the primary contributors to chemical reactions due to the low number densities in these environments making it statistically unlikely for more than two molecules to collide at once (Puzzarini, 2022). The nature of these regions also prevents chemical reactions from accessing additional energy to overcome energetic barriers. This means that the only energy available for the reaction to occur is the energy from the initial starting materials (Tinacci et al., 2023). Due to this, the chemical reactions must also be exothermic overall with an initial barrierless association of the starting materials (Puzzarini, 2022). The overall exothermic quality of the reactions, along with the low number densities, require a viable reaction to give off a leaving group to dispel the excess kinetic energy from the collision (Puzzarini, 2022). If there is no leaving group, the molecule must undergo vibrational relaxation to dispel the excess energy, or it will dissociate back to starting materials. The association of two molecules without the loss of a leaving group, radiative association, is considered note to be feasible as the probability of the molecules to simply fragment apart increases instead of them undergoing vibrational relaxation (Tinacci et al., 2023). Consequently, all of the reaction pathways discussed in this work are evaluated based on the requirements of viable reactions in the ISM/CSM as well as the abundance for each set of starting materials.
2 Computational methods
The reaction pathways explored in this work are based in the highly accurate CCSD(T)-F12b/cc-pVTZ-F12 level of theory, hereinafter referred to as F12-TZ. F12-TZ is based on coupled cluster theory at the singles, doubles, and perturbative triples level [CCSD(T)] (Raghavachari et al., 1989) along with the inclusion of the explicitly correlated F12b formalism (Adler et al., 2007; Knizia et al., 2009) in conjunction with the triple-
The starting materials, intermediate minima, and final products undergo a geometry optimization and harmonic frequency computation at the F12-TZ level of theory using the Molpro 2022.3, 2023.2, and 2024.1 quantum chemistry program suites (Werner et al., 2012). The harmonic zero-point vibrational energy is included in the total energy of the structure. Transition states along the pathway undergo a transition state optimization in Gaussian16 (Frisch et al., 2016) where a geometric structure and harmonic frequencies are computed. This transition state optimization is computed utilizing density functional theory (DFT) at the B3LYP/aug-cc-pVTZ (Dunning and Thom, 1989; Kendall et al., 1992; Woon et al., 1993) level of theory. The B3LYP computed geometry then undergoes a single-point energy (SPE) calculation via F12-TZ in Molpro to maintain consistent energies across the pathway for relative energy determination. The use of coupled cluster energies for DFT geometries has been previously shown not to affect greatly (less than 1 kcal mol−1 the energies for the coupled cluster comparison (Ramal-Olmedo et al., 2021; Ramal-Olmedo et al., 2023a; Ramal-Olmedo et al., 2023b; Nguyen et al., 2020). For transition states, the B3LYP computed harmonic zero-point energy is added to the F12-TZ energy from the SPE computation to determine the vibrationally-corrected energies. Intermediates along the pathway are located with the use of the motif shown in Firth et al. (2024). Relative energies included on the pathways are computed relative to one pair of starting materials for each pathway.
3 Results and discussion
3.1 Magnesium
Figure 1 shows the pathways to create AlOH from combinations of both sets of magnesium-bearing reactants: AlH/MgO and MgH/AlO. The MgH/AlO pair is lower in energy by 23.6 kcal mol−1 compared to the AlH/MgO pair. This is the smallest relative energy gap for all sets of reactants explored in this work. The relative energies in Figure 1 are relative to MgO/AlH. Beginning with the higher energy reactants, MgO and AlH are able to combine to form
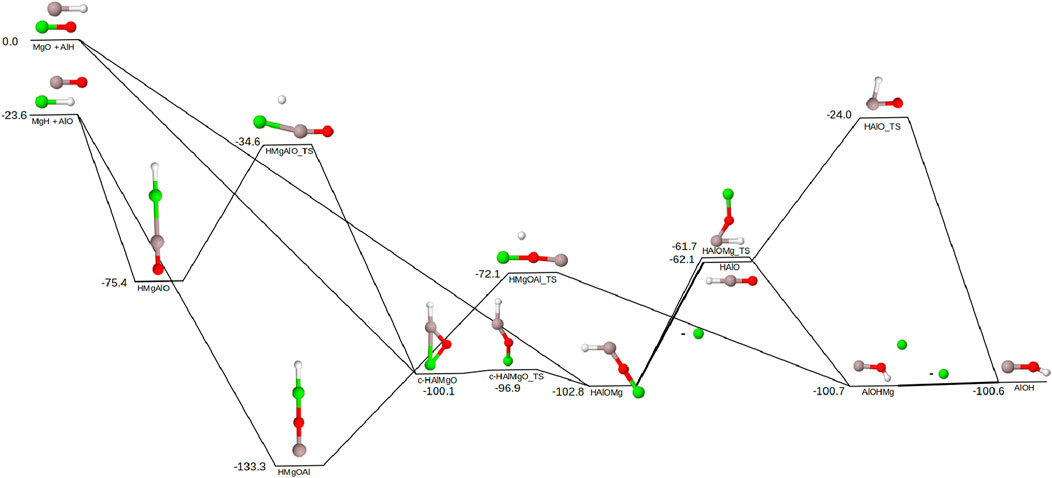
Figure 1. Reaction pathway for the formation of AlOH from AlO + MgH and AlH + MgO. Relative energies are expressed in kcal mol−1. Color scheme as follows: Al (grey), Mg (green), O (red), H (white). Lines on the pathway are bold where the Mg dissociation occurs. All molecules in this pathway are singlets except for MgH and AlO which are both doublets.
If the lower energy reactants are present instead, there are two possible intermediates depending on the orientation of AlO. Both of these structures (HMgAlO and HMgOAl) are completely linear, though the relative energies are vastly different. HMgOAl has significantly more stabilization from the additional oxygen
3.2 Silicon
The reaction pathways for the formation of AlOH with the silicon reactant pairs (SiH/AlO and SiO/AlH) are shown in Figure 2. In contrast with the magnesium reactants, the SiO/AlH pair is significantly lower in energy with a difference of 65.7 kcal mol−1 between the two sets of reactants. The relative energies in Figure 2 are determined relative to SiH/AlO. When considering, first, the reactant pair of SiO/AlH, the intermediates
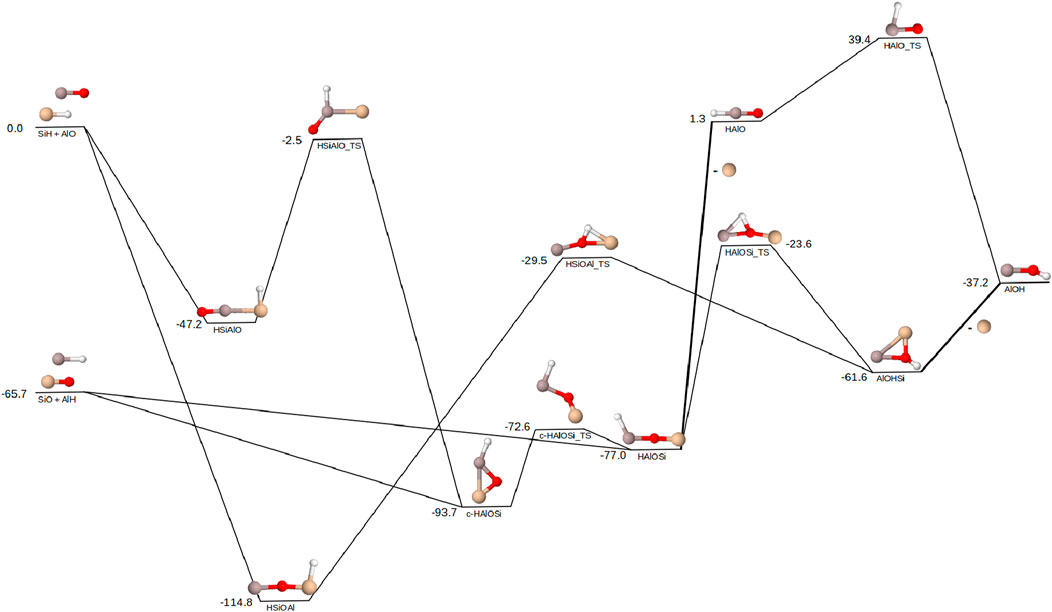
Figure 2. Reaction pathway for the formation of AlOH from AlO + SiH and AlH + SiO. Relative energies are expressed in kcal mol−1. Color scheme as follows: Al (grey), Si (tan), O (red), H (white). Lines on the pathway are bold where the Si dissociation occurs. All molecules in this pathway are singlets except for SiH and AlO which are both doublets.
If, instead, the SiH/AlO reactant pair is considered, the orientation of AlO produces two possible intermediate structures (HSiAlO and HSiOAl). The creation of an Si
3.3 Phosphorus
Possible reaction pathways for the formation of AlOH with the phosphorus-containing reactant pairs (PH/AlO and PO/AlH) are shown in Figure 3. The phosphorus reactant pairs follow a similar pattern to the silicon pairs with the PO/AlH pair existing 44.1 kcal mol−1 lower in energy than PH/AlO. Both reactant pairs for phosphorus follow similar motifs as both Mg and Si, with a couple differences as well as additional routes of formation for AlOH/P. The product pair is lower in energy than both sets of reactants, suggesting it will be thermodynamically possible to create AlOH/P from both sets of starting materials. As with silicon, loss of the phosphorus atom too early pushes the energy of the system above the energy of the reactants, which makes this route not ideal for interstellar or circumstellar reactions.
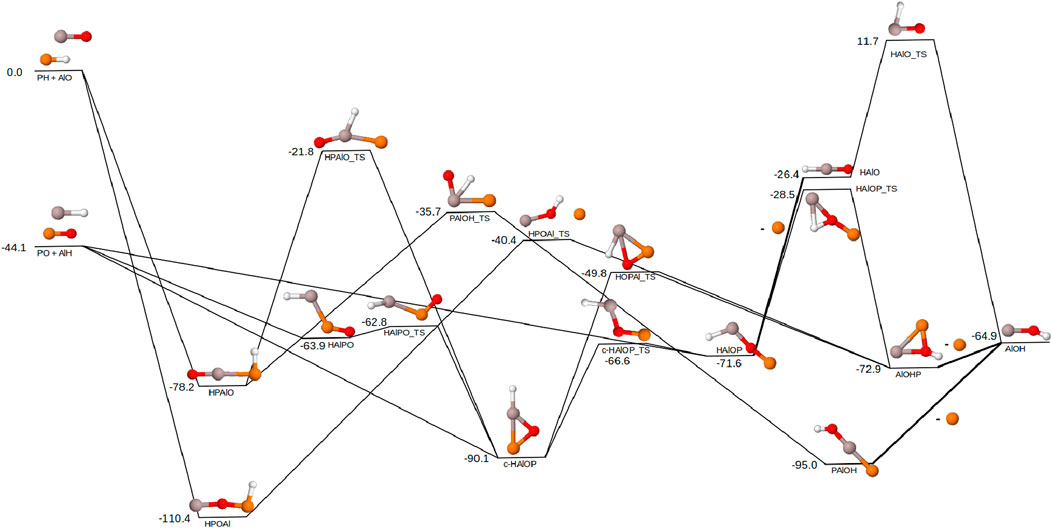
Figure 3. Reaction pathway for the formation of AlOH from AlO + PH and AlH + PO. Relative energies are expressed in kcal mol−1. Color scheme as follows: Al (grey), P (orange), O (red), H (white). Lines on the pathway are bold where the P dissociation occurs. All molecules in this pathway are doublets except for PH, AlH, and AlOH which are singlets.
In comparing the phosphorus reaction pathways to those previously shown for silicon and magnesium, the
When starting with the PH/AlO reactants instead, there is once again very strong stabilization from the addition of these reactants. HPOAl is able to follow the same motif of a hydrogen migration followed by the loss of a phosphorus atom to form AlOH/P. Additionally, HPAlO is able to follow the same previously discussed hydrogen migration and ring closing mechanism to form
3.4 Sulfur
Finally, the reaction pathways for the sulfur-containing reactant pairs SO/AlH and SH/AlO are shown in Figure 4. The sulfur reactant pairs follow the same pattern as the magnesium pairs with the SH/AlO pair 35.4 kcal mol−1 lower in energy than SO/AlH. Both sets of reactant pairs are able to follow the motifs present in the phosphorus pathway (Figure 3). Beginning with the reactions of SH/AlO, the initial intermediates, HSOAl and HSAlO, are flipped energetically compared to the analogs with Mg, Si, and P. Where previously the species with the terminal aluminum would be lower in energy, the S-analog shows the terminal oxygen as 15.7 kcal mol−1 lower in energy. This could be due to oxygen and sulfur both being in the chalcogen family and their strong propensity to form bonds with one another (Doerksen and Fortenberry, 2020). The similar chemistry between these two elements minimizes the stabilization effects of the additional bond with oxygen shown in Figures 1–3. Both HSOAl and HSAlO are able to proceed on to form AlOH/S without exceeding the initial energy of SH/AlO. The hydrogen migration transition states to move the hydrogen onto the oxygen are both
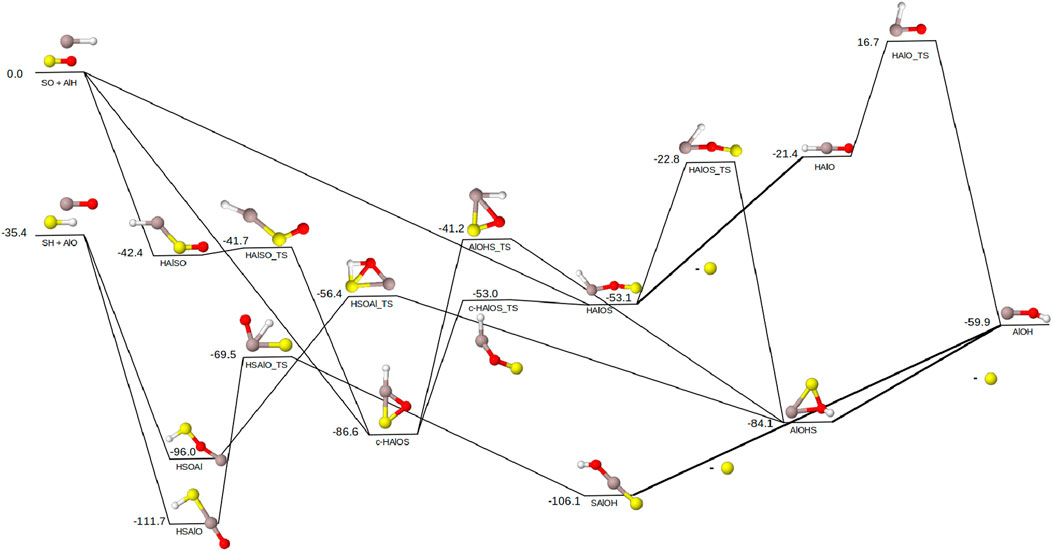
Figure 4. Reaction pathway for the formation of AlOH from AlO + SH and AlH + SO. Relative energies are expressed in kcal mol−1. Color scheme as follows: Al (grey), S (yellow), O (red), H (white). Lines on the pathway are bold where the S dissociation occurs. All molecules in this pathway are singlets except for SH and AlO which are both doublets.
Depending upon the orientation of the reactants when SO and AlH collide, there are three possible initial intermediates: HAlSO,
4 Astrophysical implications
Based on the previously discussed detections of the initial diatomics, MgH has a known presence in a variety of locations while MgO has yet to be detected in regions of high dust nucleation. This suggests MgH and AlO as the more likely pair of starting materials for AlOH creation in this manner. However, MgO and AlH cannot be discounted completely as the difficulty in detecting MgO could be related to MgO quickly reacting in the manner described here or creating larger magnesium structures such as Mg2O (Costantini et al., 2019; Flint et al., 2024). In the event these AlH/MgO and MgH/AlO reactants are present in a high-temperature region, such as the atmosphere of a hot exoplanet, the reactions in Figure 1 will likely still occur. The higher temperature will provide the reactants more energy to cascade through the pathways, submerging the barriers even further below the energy of the reactants.
While SiO is observed more readily than SiH in circumstellar and interstellar regions, SiO is so low in relative energy (Figure 2) that it will likely not contribute to the formation of AlOH. Thus, SiO is likely to participate in the formation of larger silicates instead of AlOH (Flint and Fortenberry, 2023). While SiH has fewer instances of detection than SiO, SiH has been found in locations where AlO has also been detected such as VY Canis Majoris (Tenebaum and Ziurys, 2009). This, along with the lone energetically favorable path of formation stemming from SiH, suggests reactions of SiH with AlO to be the most likely method to form AlOH with silicon diatomics. SiH has also been the focus of other theoretical reaction pathways including the possible formation of SiS (Fortenberry and McGuire, 2024), and detection of molecules in pathways where it could be invoked imply its (as-of-yet undetected) presence. High temperature environments such as hot and ultra-hot exoplanets can reach temperatures up to and exceeding 2,500 K (Garhart et al., 2020; Baxter et al., 2020; Jones et al., 2022), which could potentially provide sufficient energy to the reactants to overcome the barriers. A Boltzmann population analysis indicates a temperature of 4,600 K would be requisite to provide enough energy to the SiO/AlH reactants for 1% of the reactants to surpass the energy barriers shown in Figure 2 and form AlOH. Along a similar vein, for 1% of the HAlO intermediate to surpass the HAlO_TS barrier and form AlOH, the environment would need to be at a temperature of 4,200 K. The most extreme member of the ultra-hot Jupiter classification and the hottest known exoplanet to date is KELT-9b with an equilibrium temperature of 4,050
The complete lack of detection of PH suggests that the PO/AlH reactants will be more likely to react and form AlOH. However, the nondetections of PH could be due to the hydride reacting extremely quickly to form larger structures. Hence, the PH/AlO reactants should not be fully discounted. The PO/AlH reactants show a pathway that prevents the formation of AlOH with the HAlOP_TS barrier 15.6 kcal mol−1 above the energy of the PO/AlH reactants. According to a Boltzmann population analysis, this barrier is small enough for temperatures of 1,700 K to allow for 1% of the reactants to surpass the barrier and form AlOH. Hot Jupiters have equilibrium temperatures in the range of 1,500–2,000 K (Baxter et al., 2020), suggesting such exoplanets as suitable environments for the PO/AlH reactants to progress through the
The detections of both SH and SO support the viability of each set of reactants to form AlOH. While much of the sulfur in space is believed to be tied up in FeS (Costantini et al., 2019), the likelihood of the sulfur reactants participating in the formation of AlOH is lower than the magnesium or silicon reactants. However, the greater understanding of how SH and SO react with other species will aid in future studies of the sulfur diatomics in inter- and circumstellar regions, such as the proposed formation of SiS using SH (Fortenberry and McGuire, 2024).
5 Conclusion
This work proposes a series of gas-phase routes of formation for AlOH in CSEs of AGB stars from a combination of third row diatomic hydrides and oxides. Nearly every combination of reactants explored has at least one energetically favorable route of formation to the AlOH/X products. Each pathway follows a series of similar motifs for the rearrangements while the phosphorus and sulfur pathways begin to add more paths that are unavailable to magnesium and silicon. In truth AlOH is likely formed from a mixture of these pathways depending upon the abundance of various required starting materials. While some of the proposed reactants have not yet been identified in CSEs or other astronomical regions of space, these reactions can not be completely discounted as the abundances of those diatomics may be low due to quick reactions to form other species. Future kinetic studies are necessary to determine the rates of the rearrangements occurring during these proposed reaction pathways and predict the fastest path. Additional observations of AGB stars where some of these diatomic molecules could exist are crucial to try to confirm the existence of these diatomics in such environments.
Data availability statement
The original contributions presented in the study are included in the article/Supplementary Material, further inquiries can be directed to the corresponding author.
Author contributions
ReF: Conceptualization, Data curation, Formal Analysis, Investigation, Methodology, Validation, Visualization, Writing–original draft, Writing–review and editing. RyF: Conceptualization, Data curation, Funding acquisition, Methodology, Project administration, Resources, Supervision, Validation, Writing–review and editing.
Funding
The author(s) declare that financial support was received for the research, authorship, and/or publication of this article. This work is funded through NASA Grants NNH22ZHA004C and 22-A22ISFM-0009 as well as the University of Mississippi’s College of Liberal Arts and Graduate School. The Mississippi Center for Supercomputing Research funded in part by NSF grant OIA-1757220 provided the computational hardware needed for this work.
Conflict of interest
The authors declare that the research was conducted in the absence of any commercial or financial relationships that could be construed as a potential conflict of interest.
The author(s) declared that they were an editorial board member of Frontiers, at the time of submission. This had no impact on the peer review process and the final decision.
Publisher’s note
All claims expressed in this article are solely those of the authors and do not necessarily represent those of their affiliated organizations, or those of the publisher, the editors and the reviewers. Any product that may be evaluated in this article, or claim that may be made by its manufacturer, is not guaranteed or endorsed by the publisher.
Supplementary material
The Supplementary Material for this article can be found online at: https://www.frontiersin.org/articles/10.3389/fspas.2024.1466975/full#supplementary-material
References
Adler, T. B., Knizia, G., and Werner, H.-J. (2007). A simple and efficient CCSD(T)-F12 approximation. J. Chem. Phys. 127, 221106. doi:10.1063/1.2817618
Asplund, M., Grevesse, N., Jacques, S. A., and Scott, P. (2009). The chemical composition of the sun. Annu. Rev. Astron. Astrophys. 47, 481–522. doi:10.1146/annurev.astro.46.060407.145222
Avery, L. W., Bell, M. B., Cunningham, C. T., Feldman, P. A., Hayward, R. H., McLeod, J. M., et al. (1994). Submillimeter molecular line observations of IRC +10216: searches for MgH, SiH2, and HCO+, and detection of hot HCN. Astrophys. J. 426, 737. doi:10.1086/174110
Banerjee, D. P. K., Varricatt, W. P., Ashok, N. M., and Launila, O. (2003). Remarkable changes in the near-infrared spectrum of the nova-like variable V4332 sagittarii. Astrophys. J. 598, L31–L34. doi:10.1086/380389
Banerjee, D. P. K., Varricatt, W. P., Mathew, B., Launila, O., and Ashok, N. M. (2012). The A-X infrared bands of aluminum oxide in stars: search and new detections. Astrophys. J. Lett. 753, L20. doi:10.1088/2041-8205/753/1/L20
Baxter, C., Désert, J.-M., Parmentier, V., Line, M., Fortney, J., Arcangeli, J., et al. (2020). A transition between the hot and the ultra-hot jupiter atmospheres. Astron. Astrophys. 639, A36. doi:10.1051/0004-6361/201937394
Bowesman, C. A., Shuai, M., Yurchenko, S. N., and Tennyson, J. (2021). A high-resolution line list for AlO. Mon. Not. R. Astron. Soc. 508, 3181–3193. doi:10.1093/mnras/stab2525
Caffau, E., Bonifacio, P., Faraggiana, R., and Steffen, M. (2011). The galactic evolution of phosphorus. Astron. Astrophys. 532, A98. doi:10.1051/0004-6361/201117313
Chubb, K. L., Min, M., Kawashima, Y., Helling, C., and Waldmann, I. (2020). Aluminium oxide in the atmosphere of hot jupiter WASP-43b. Astron. Astrophys. 639, A3. doi:10.1051/0004-6361/201937267
Clayton, D. D. (1978). “Precondensed matter: key to the early solar system,”, Paper presesnted at the conference on protostars and planets, held at the planetary science Institute, 19. Tucson, Arizona: University of Arizona, 109–137. between January 3 and 7, 1978.). Moon and Planets. doi:10.1007/BF00896983
Colón, K. D., Kreidberg, L., Welbanks, L., Line, M. R., Madhusudhan, N., Beatty, T., et al. (2020). An unusual transmission spectrum for the sub-saturn KELT-11b suggestive of a subsolar water abundance. Astron. J. 160, 280. doi:10.3847/1538-3881/abc1e9
Costantini, E., Zeegers, S. T., Rogantini, D., de Vries, C. P., Tielens, A. G. G. M., and Waters, L. B. F. M. (2019). X-Ray extinction from interstellar dust - prospects of observing carbon, sulfur, and other trace elements. Astron. Astrophys. 629, A78. doi:10.1051/0004-6361/201833820
Danilovich, T., Gottlieb, C. A., Decin, L., Richards, A. M. S., Lee, K. L. K., Kamiński, T., et al. (2020). Rotational spectra of vibrationally excited AlO and TiO in oxygen-rich stars. Astrophys. J. 904, 110. doi:10.3847/1538-4357/abc079
De Beck, E., Kamiński, T., Patel, N. A., Young, K. H., Gottlieb, C. A., Menten, K. M., et al. (2013). PO and PN in the wind of the oxygen-rich AGB star Ik tauri. Astron. Astrophys. 558, A132. doi:10.1051/0004-6361/201321349
Decin, L., Danilovich, T., Gobrecht, D., Plane, J. M. C., Richards, A. M. S., Gottlieb, C. A., et al. (2018). Constraints on metal oxide and metal hydroxide abundances in the winds of AGB stars: potential detection of FeO in R dor. Astrophys. J. 855, 113. doi:10.3847/1538-4357/aaab6a
Decin, L., Richards, A. M. S., Waters, L. B. F. M., Danilovich, T., Gobrecht, D., Khouri, T., et al. (2017). Study of the aluminium content in AGB winds using ALMA: indications for the presence of gas-phase (Al2O3)nclusters. Astron. Astrophys. 608, A55. doi:10.1051/0004-6361/201730782
Deegan, M. J. O., and Knowles, P. J. (1994). Perturbative corrections to account for triple excitations in closed and open shell coupled cluster theories. Chem. Phys. Lett. 227, 321–326. doi:10.1016/0009-2614(94)00815-9
Dickinson, D. F. (1972). Detection of silicon monoxide at 87 GHz. Astrophys. J. Lett. 175, L43. doi:10.1086/180981
Doerksen, E. S., and Fortenberry, R. C. (2020). Coincidence between bond strength, atomic abundance, and the composition of rocky materials. ACS Earth Space Chem. 4, 812–817. doi:10.1021/acsearthspacechem.0c00029
Dunning, J., and Thom, H. (1989). Gaussian basis sets for use in correlated molecular calculations. I. The atoms boron through neon and hydrogen. J. Chem. Phys. 90, 1007–1023. doi:10.1063/1.456153
Firth, R. A., Bell, K. M., and Fortenberry, R. C. (2024). Formation of AlO, AlOH, and Al(OH)3 in the interstellar medium and circumstellar envelopes of AGB stars. ACS Earth Space Chem. 8, 974–982. doi:10.1021/acsearthspacechem.3c00335
Flint, A. R., and Fortenberry, R. C. (2023). Formation and destruction of Si6O12 nanostructures in the gas phase: applications to grain nucleation and water generation. ACS Earth Space Chem. 7, 2119–2128. doi:10.1021/acsearthspacechem.3c00207
Flint, A. R., Westbrook, B. R., and Fortenberry, R. C. (2024). Theoretical rotational and vibrational spectra data for the hypermagnesium oxide species Mg2O and Mg2O and Mg2O+. Chem. Phys. Chem. 25, e202400479. doi:10.1002/cphc.202400479
Fontani, F., Rivilla, V. M., Caselli, P., Vasyunin, A., and Palau, A. (2016). Phosphorus-bearing molecules in massive dense cores. Astrophys. J. Lett. 822, L30. doi:10.3847/2041-8205/822/2/L30
Fortenberry, R. C., and McGuire, B. A. (2024). A possible additional formation pathway for the interstellar diatomic SiS. Astrophys. J. 971, 101. doi:10.3847/1538-4357/ad4d94
Frisch, M. J., Trucks, G. W., Schlegel, H. B., Scuseria, G. E., Robb, M. A., Cheeseman, J. R., et al. (2016). Gaussian 16 revision C.01. Wallingford CT: Gaussian Inc.
Garhart, E., Deming, D., Mandell, A., Knutson, H. A., Wallack, N., Burrows, A., et al. (2020). Statistical characterization of hot jupiter atmospheres using spitzer’s secondary eclipses. Astron. J. 159, 137. doi:10.3847/1538-3881/ab6cff
Gaudi, B. S., Stassun, K. G., Collins, K. A., Beatty, T. G., Zhou, G., Latham, D. W., et al. (2017). A giant planet undergoing extreme-ultraviolet irradiation by its hot massive-star host. Nature 546, 514–518. doi:10.1038/nature22392
Grosselin, D., and Fortenberry, R. C. (2022). Formation of magnesium and aluminum oxides from water and metal hydrides: creation of the smallest ruby. ACS Earth Space Chem. 6, 18–24. doi:10.1021/acsearthspacechem.1c00324
Győrffy, W., and Werner, H.-J. (2018). Analytical energy gradients for explicitly correlated wave functions. II. Explicitly correlated coupled cluster singles and doubles with perturbative triples corrections: CCSD(T)-F12. J. Chem. Phys. 148, 114104. doi:10.1063/1.5020436
Hampel, C., Peterson, K. A., and Werner, H.-J. (1992). A comparison of the efficiency and accuracy of the quadratic configuration interaction (QCISD), coupled cluster (CCSD), and brueckner coupled cluster (BCCD) methods. Chem. Phys. Lett. 190, 1–12. doi:10.1016/0009-2614(92)86093-W
Helgaker, T., Ruden, T. A., Jørgensen, P., Olsen, J., and Klopper, W. (2004). A priori calculation of molecular properties to chemical accuracy. J. Phys. Org. Chem. 17, 913–933. doi:10.1002/poc.841
Henry, R. B. C., Kwitter, K. B., and Balick, B. (2004). Sulfur, chlorine, and argon abundances in planetary nebulae. IV. Synthesis and the sulfur anomaly. Astron. J. 127, 2284–2302. doi:10.1086/382242
Herbig, G. H. (1956). Identification of aluminum hydride as the emitter of bright lines observed in Cygni near minimum light. Publ. Astron. Soc. Pac. 68, 204. doi:10.1086/126916
Hill, J. G., and Peterson, K. A. (2010). Correlation consistent basis sets for explicitly correlated wavefunctions: valence and core valence basis sets for Li, Be, Na, and Mg. Phys. Chem. Chem. Phys. 12, 10460–10468. doi:10.1039/c0cp00020e
Hony, S., Waters, L. B. F. M., and Tielens, A. G. G. M. (2002). The Carrier of the “30” the carrier of the “30” μm emission feature in evolved stars: a simple model using magnesium sulfidem Emission Feature in Evolved Stars. A Simple Model Using Magnesium Sulfide. Astron. Astrophys. 390, 533–553. doi:10.1051/0004-6361:20020603
Jiang, C., Chen, G., Murgas, F., Pallé, E., Parviainen, H., and Ma, Y. (2024). Confirmation of TiO absorption and tentative detection of MgH and CrH in the atmosphere of HAT-P-41b. Astron. Astrophys. 682, A73. doi:10.1051/0004-6361/202347989
Johnson, H. R., and Sauval, A. J. (1982). Molecules in red-giant stars. I - column densities in models for K and M stars. Astron. Astrophys. Suppl. Ser. 49, 77–87.
Jones, K., Morris, B. M., Demory, B.-O., Heng, K., Hooton, M. J., Billot, N., et al. (2022). The stable climate of KELT-9b. Astron. Astrophys. 666, A118. doi:10.1051/0004-6361/202243823
Kama, M., Shorttle, O., Jermyn, A. S., Folsom, C. P., Furuya, K., Bergin, E. A., et al. (2019). Abundant refractory sulfur in protoplanetary disks. Astrophys. J. 885, 114. doi:10.3847/1538-4357/ab45f8
Kamiński, T., Schmidt, M. R., and Menten, K. M. (2013). Aluminium oxide in the optical spectrum of VY Canis Majoris. Astron. Astrophys. 549, A6. doi:10.1051/0004-6361/201220650
Kamiński, T., Wong, K. T., Schmidt, M. R., Müller, H. S. P., Gottlieb, C. A., Cherchneff, I., et al. (2016). An observational study of dust nucleation in mira (o ceti) I. Variable features of AlO and other Al-bearing species. Astron. Astrophys. 592, A53. doi:10.1051/0004-6361/201628664
Karovicova, I., Wittkowski, M., Ohnaka, K., Boboltz, D. A., Fossat, E., and Sholz, M. (2013). New insights into the dust formation of oxygen-rich AGB stars. Astron. Astrophys. 560, A75. doi:10.1051/0004-6361/201322376
Karthikeyan, B., Rajamanickam, N., and Bagare, S. P. (2010). On the rotational temperature of AlH in sunspots. Sol. Phys. 264, 279–285. doi:10.1007/s11207-010-9590-8
Keller, L. P., Hony, S., Bradley, J. P., Molster, F. J., Waters, L. B. F. M., Bouwman, J., et al. (2002). Identification of iron sulphide grains in protoplanetary disks. Nature 417, 148–150. doi:10.1038/417148a
Kendall, R. A., Dunning, J., Thom, H., and Harrison, R. J. (1992). Electron affinities of the first-row atoms revisited. Systematic basis sets and wave functions. J. Chem. Phys. 96, 6796–6806. doi:10.1063/1.462569
Kirkpatrick, J. D. (2005). New spectral types L and T. Ann. Rev. Astron. Astrophys. 43, 195–245. doi:10.1146/annurev.astro.42.053102.134017
Knizia, G., Adler, T. B., and Werner, H.-J. (2009). Simplified CCSD(T)-F12 methods: theory and benchmarks. J. Chem. Phys. 130, 054104. doi:10.1063/1.3054300
Knowles, P. J., Hampel, C., and Werner, H.-J. (1993). Coupled cluster theory for high spin, open shell reference wave functions. J. Chem. Phys. 99, 5219–5227. doi:10.1063/1.465990
Lefloch, B., Vastel, C., Viti, S., Jimenez-Serra, I., Codella, C., Podio, L., et al. (2016). Phosphorus-bearing molecules in solar-type star-forming regions: first PO detection. Mon. Not. R. Astron. Soc. 462, 3937–3944. doi:10.1093/mnras/stw1918
Lewis, N. K., Wakeford, H. R., MacDonald, R. J., Goyal, J. M., Sing, D. K., Barstow, J., et al. (2020). Into the UV: the atmosphere of the hot jupiter HAT-P-41b revealed. Astrophys. J. Lett. 902, L19. doi:10.3847/2041-8213/abb77f
Lucas, R., and Liszt, H. S. (2000). SiO in diffuse, translucent and ‘spiral-arm’ clouds. Astron. Astrophys. 355, 327–332.
Mangan, T. P., Douglas, K. M., Lade, R. E., Gobrecht, D., Decin, L., and Plane, J. M. C. (2021). Kinetic study of the reactions of AlO with H2O and H2; precursors to stellar dust formation. ACS Earth Space Chem. 5, 3385–3395. doi:10.1021/acsearthspacechem.1c00225
Massalkhi, S., Agúndez, M., Cernicharo, J., and Velilla-Prieto, L. (2020). The abundance of S- and Si-bearing molecules in O-rich circumstellar envelopes of AGB stars. Astron. Astrophys. 641, A57. doi:10.1051/0004-6361/202037900
McGuire, B. A. (2018). 2018 census of interstellar, circumstellar, extragalactic, protoplanetary disk, and exoplanetary molecules. Astrophys. J. Suppl. Ser. 239, 17. doi:10.3847/1538-4365/aae5d2
McGuire, B. A. (2021). 2021 census of interstellar, circumstellar, extragalactic, protoplanetary disk, and exoplanetary molecules. Astrophys. J. Suppl. Ser. 259, 30. doi:10.3847/1538-4365/ac2a48
Neufeld, D. A., Falgarone, E., Gerin, M., Godard, B., Herbst, E., Pineau des Forêts, G., et al. (2012). Discovery of interstellar mercapto radicals (SH) with the GREAT instrument on SOFIA. Astron. Astrophys. 542, L6. doi:10.1051/0004-6361/201218870
Nguyen, Q. L. D., Peters, W. K., and Fortenberry, R. C. (2020). Highly-excited state properties of cumulenone chlorides in the vacuum-ultraviolet. Phys. Chem. Chem. Phys. 22, 11838–11849. doi:10.1039/D0CP01835J
Parnis, J. M., Mitchell, S. A., Kanigan, T. S., and Hackett, P. A. (1989). Gas-phase reactions of aluminum monoxide with small molecules. J. Phys. Chem. 93, 8045–8052. doi:10.1021/j100361a017
Pavlenko, Y. V., Tennyson, J., Yurchenko, S. N., Schmidt, M. R., Jones, H. R. A., Lyubchik, Y., et al. (2022). AlH lines in the blue spectrum of Proxima Centauri. Mon. Not. R. Astron. Soc. 516, 5655–5673. doi:10.1093/mnras/stac2588
Prieto, L. V., Cernicharo, J., Quintana-Lacaci, G., Agúndez, M., Castro-Carrizo, A., Fonfría, J. P., et al. (2015). Si-bearing molecules toward IRC+10216: ALMA unveils the molecular envelope of CWLeo. Astrophys. J. Lett. 805, L13. doi:10.1088/2041-8205/805/2/L13
Puzzarini, C. (2022). Gas-phase chemistry in the interstellar medium: the role of laboratory astrochemistry. Front. Astron. Space Sci. 8, 811342. doi:10.3389/fspas.2021.811342
Raghavachari, K., Trucks, G. W., Pople, J. A., and Head-Gordon, M. (1989). A fifth-order perturbation comparison of electron correlation theories. Chem. Phys. Lett. 157, 479–483. doi:10.1016/s0009-2614(89)87395-6
Ramal-Olmedo, J. C., Menor-Salván, C. A., and Fortenberry, R. C. (2021). Mechanisms for gas-phase molecular formation of neutral formaldehyde (H2CO) in cold astrophysical regions. Astron. Astrophys. 656, A148. doi:10.1051/0004-6361/202141616
Ramal-Olmedo, J. C., Menor-Salván, C. A., Miyoshi, A., and Fortenberry, R. C. (2023a). Gas-phase molecular formation mechanisms of cyanamide (NH2CN) and its tautomer carbodiimide (HNCNH) under sgr B2(N) astrophysical conditions. Astron. Astrophys. 672, A49. doi:10.1051/0004-6361/202245811
Ramal-Olmedo, J. C., Menor-Salván, C. A., Miyoshi, A., and Fortenberry, R. C. (2023b). Possible gas-phase synthesis of neutral malononitrile (C3H2N2) and isocyanoacetonitrile (NCCH2NC) under the upper atmospheric conditions of titan. ACS Earth Space Chem. 7, 1694–1712. doi:10.1021/acsearthspacechem.3c00107
Rivilla, V. M., Fontani, F., Beltrán, M. T., Vasyunin, A., Caselli, P., Martín-Pintado, J., et al. (2016). The first detections of the key prebiotic molecule PO in star-forming regions. Astrophys. J. 826, 161. doi:10.3847/0004-637X/826/2/161
Rogantini, D., Costantini, E., Zeegers, S. T., Mehdipour, M., Psaradaki, I., Raassen, A. J. J., et al. (2020). Magnesium and silicon in interstellar dust: X-ray overview. Astron. Astrophys. 641, A149. doi:10.1051/0004-6361/201936805
Sauval, A. J. (1969). Identification of SiH lines in the solar disk spectrum. Sol. Phys. 10, 319–329. doi:10.1007/BF00145520
Schilke, P., Benford, D. J., Hunter, T. R., Lis, D. C., and Phillips, T. G. (2001). A line survey of orion-KL from 607 to 725 GHz. Astrophys. J. Suppl. Ser. 132, 281–364. doi:10.1086/318951
Sharipov, A., Titov, N., and Starik, A. (2011). Kinetics of Al + H2O reaction: theoretical study. J. Phys. Chem. A 115, 4476–4481. doi:10.1021/jp111826y
Sheppard, K. B., Welbanks, L., Mandell, A. M., Madhusudhan, N., Nikolov, N., Deming, D., et al. (2021). The hubble PanCET program: a metal-rich atmosphere for the inflated hot jupiter hat-P-41b. Astron. J. 161, 51. doi:10.3847/1538-3881/abc8f4
Siebert, M. A., Simon, I., Shingledecker, C. N., Carroll, P. B., Burkhardt, A. M., Booth, S. T., et al. (2020). A search for light hydrides in the envelopes of evolved stars. Astrophys. J. 901, 22. doi:10.3847/1538-4357/abac0e
Sotirovski, P. (1972). Table of solar diatomic molecular lines spectral range 4900 - 6441 Å. Astron. Astrophys. Suppl. 6, 85.
Sriramachandran, P., Viswanathan, B., and Shanmugavel, R. (2013). Occurrence of AlO molecular lines in sunspot umbral spectra. Sol. Phys. 286, 315–326. doi:10.1007/s11207-013-0264-1
Swinnen, S., Nguyen, V. S., Sakai, S., and Nguyen, M. T. (2009). Calculations suggest facile hydrogen release from water using boranes and alanes as catalysts. Chem. Phys. Lett. 472, 175–180. doi:10.1016/j.cplett.2009.02.078
Tachibana, S., Kamizuka, T., Hirota, T., Sakai, M., Oya, Y., Takigawa, A., et al. (2019). Spatial distribution of AlO in a high-mass protostar candidate orion source I. Astrophys. J. Lett. 875, L29. doi:10.3847/2041-8213/ab1653
Takigawa, A., Kamizuka, T., Tachibana, S., and Yamamura, I. (2017). Dust Formation and wind acceleration around the aluminum oxide-rich AGB star W hydrae. Sci. Adv 3, eaao2149. doi:10.1126/sciadv.aao2149
Tenebaum, E. D., and Ziurys, L. M. (2009). Millimeter detection of AlO X2Σ+: metal oxide chemistry in the envelope of VY Canis Majoris. Astrophys. J. Lett. 694, L59–L63. doi:10.1088/0004-637x/694/1/l59
Tenenbaum, E. D., and Ziurys, L. M. (2010). Exotic metal molecules in oxygen-rich envelopes: detection of AlOH X1Σ+ in VY Canis Majoris. Astrophys. J. Lett. 712, L93–L97. doi:10.1088/2041-8205/712/1/l93
Tennyson, J., and Yurchenko, S. N. (2017). Laboratory spectra of hot molecules: data needs for hot super-Earth exoplanets. Mol. Astrophys. 8, 1–18. doi:10.1016/j.molap.2017.05.002
Tinacci, L., Ferrada-Chamorro, S., Ceccarelli, C., Pantaleone, S., Ascenzi, D., Maranzana, A., et al. (2023). The GRETOBAPE gas-phase reaction network: the importance of being exothermic. Astrophys. J. Suppl. Ser. 266, 38. doi:10.3847/1538-4365/accae9
Turner, B. E. (1998). The Physics and chemistry of small translucent molecular clouds. X. SiO. Astrophys. J. 495, 804–820. doi:10.1086/305319
Tylenda, R., Crause, L. A., Górny, S. K., and Schmidt, M. R. (2005). V4332 sagittarii revisited. Astron. Astrophys. 439, 651–661. doi:10.1051/0004-6361:20041581
von Essen, C., Mallonn, M., Welbanks, L., Madhusudhan, N., Pinhas, A., Bouy, H., et al. (2019). An optical transmission spectrum of the ultra-hot jupiter WASP-33 b. First indication of aluminum oxide in an exoplanet. Astron. Astrophys. 622, A71. doi:10.1051/0004-6361/201833837
Wallace, L., Hinkle, K., and Livingston, W. (2000). An atlas of sunspot umbral spectra in the visible, from 15,000 to 25,500 cm, Angstrom, (3920-6664.
Wallström, S. H. J., Danilovich, T., Müller, H. S. P., Gottlieb, C. A., Maes, S., Van de Sande, M., et al. (2024). ATOMIUM: molecular inventory of 17 oxygen-rich evolved stars observed with ALMA. Astron. Astrophys. 681, A50. doi:10.1051/0004-6361/202347632
Werner, H.-J., Knowles, P. J., Knizia, G., Manby, F. R., and Schütz, M. (2012). Molpro: a general-purpose quantum chemistry program package. WIREs Comput. Mol. Sci. 2, 242–253. doi:10.1002/wcms.82
Westphal, A. J., Stroud, R. M., Bechtel, H. A., Brenker, F. E., Butterworth, A. L., Flynn, G. J., et al. (2014). Evidence for interstellar origin of seven dust particles collected by the stardust spacecraft. Science 345, 786–791. doi:10.1126/science.1252496
Wilson, R. W., Penzias, A. A., Jefferts, K. B., Kutner, M., and Thaddeus, P. (1971). Discovery of interstellar silicon monoxide. Astrophys. J. Lett. 167, L97. doi:10.1086/180769
Woon, D. E., Dunning, J., and Thom, H. (1993). Gaussian basis sets for use in correlated molecular calculations. III. The atoms aluminum through argon. J. Chem. Phys. 98, 1358–1371. doi:10.1063/1.464303
Yurchenko, S. N., Sinden, F., Lodi, L., Hill, C., Gorman, M. N., and Tennyson, J. (2017). ExoMol line lists xxiv: a new hot line list for silicon monohydride, SiH. Mon. Not. R. Astron. Soc. 473, 5324–5333. doi:10.1093/mnras/stx2738
Zhao, Y., and Truhlar, D. G. (2007). The M06 suite of density functionals for main group thermochemistry, thermochemical kinetics, noncovalent interactions, excited states, and transition elements: two new functionals and systematic testing of four M06- class functionals and 12 other functionals. Theor. Chem. Acc. 120, 215–241. doi:10.1007/s00214-007-0310-x
Keywords: reaction pathways, submerged barriers, aluminum chemistry, third row chemistry, diatomic reactants, coupled cluster theory
Citation: Firth RA and Fortenberry RC (2024) Alternate formation of AlOH from third row diatomic hydrides and oxides. Front. Astron. Space Sci. 11:1466975. doi: 10.3389/fspas.2024.1466975
Received: 18 July 2024; Accepted: 09 September 2024;
Published: 25 September 2024.
Edited by:
Tom Millar, Queen’s University Belfast, United KingdomReviewed by:
Helgi Rafn Hrodmarsson, Laboratoire Inter-universitaire des Systemes Atmospheriques (CNRS UMR 7583)–Université Paris Est Créteil (UPEC), FranceGerman Molpeceres De Diego, Spanish National Research Council (CSIC), Spain
Copyright © 2024 Firth and Fortenberry. This is an open-access article distributed under the terms of the Creative Commons Attribution License (CC BY). The use, distribution or reproduction in other forums is permitted, provided the original author(s) and the copyright owner(s) are credited and that the original publication in this journal is cited, in accordance with accepted academic practice. No use, distribution or reproduction is permitted which does not comply with these terms.
*Correspondence: Ryan C. Fortenberry, cjQxMEBvbGVtaXNzLmVkdQ==