- 1Department of Earth and Space Sciences, University of Washington, Seattle, WA, United States
- 2Institute of Planetary Research, German Aerospace Center (DLR), Berlin, Germany
- 3Institute of Aerospace Medicine, German Aerospace Center (DLR), Cologne, Germany
- 4Institute of Geological Sciences, Freie Universität Berlin, Berlin, Germany
- 5Geophysical Imaging and Monitoring, RWTH Aachen University, Aachen, Germany
- 6Faculty of Aerospace Engineering, FH Aachen University of Applied Sciences, Aachen, Germany
- 7Institut für Bioengineering, FH Aachen University of Applied Sciences, Jülich, Germany
- 8Experimental Space Science and Biophysics, Freie Universität Berlin, Berlin, Germany
- 9GSI - Gesellschaft für Systementwicklung and Instrumentierung mbH, Aachen, Germany
- 10German Space Agency at DLR, German Aerospace Center (DLR), Bonn, Germany
- 11Physics Institute III B, RWTH Aachen University, Aachen, Germany
- 12Institute of Space Systems, University of Stuttgart, Stuttgart, Germany
- 13Alfred-Wegener-Institute, Helmholtz Centre for Polar and Marine Research, Bremerhaven, Germany
- 14Institute of Optical Sensor Systems, German Aerospace Center (DLR), Berlin, Germany
- 15Astrobiology Group, Center of Astronomy and Astrophysics, Technische Universität Berlin, Berlin, Germany
- 16Section Geomicrobiology, GFZ German Research Center for Geosciences, Potsdam, Germany
- 17Department of Plankton and Microbial Ecology, Leibniz-Institute of Freshwater Ecology and Inland Fisheries (IGB), Stechlin, Germany
- 18School of the Environment, Washington State University, Pullman, WA, United States
- 19Microgravity User Support Center (MUSC), Space Operations and Astronaut Training, German Aerospace Center (DLR), Cologne, Germany
Icy bodies with subsurface oceans are a prime target for astrobiology investigations, with an increasing number of scientists participating in the planning, development, and realization of space missions to these worlds. Within Germany, the Ocean Worlds and Icy Moons working group of the German Astrobiology Society provides an invaluable platform for scientists and engineers from universities and other organizations with a passion for icy ocean worlds to share knowledge and start collaborations. We here present an overview about astrobiology research activities related to icy ocean worlds conducted either in Germany or in strong collaboration with scientists in Germany. With recent developments, Germany offers itself as a partner to contribute to icy ocean world missions.
1 Introduction: past and future exploration of icy ocean worlds
The German space science community and particularly the German Astrobiology Society (Deutsche Astrobiologische Gesellschaft; DAbG) has a deep scientific interest in space exploration. In 2023, the German Government published its space program strategy (BMWK, 2023) by explicitly mentioning the value of space research, technology developments and exploration for the society in general. The strategic view formulated in this document is to support synergy of different competences, expertise and resources to allow on the one hand space research and space exploration leading to international space missions and on the other hand the use of space related technology via technology transfer also in society relevant topics such as climate and environmental protection as well as discovering and using sustainable resources. We will here start with an overview on the general scientific interest and space missions as well as showing the German participation and/or interest in the present and future exploration missions particularly to the icy moons of Jupiter and Saturn. We further describe the technology developments and scientific strategy including planetary analog field research, laboratory experiments, numerical simulation investigations and even space experiments in Earth’s orbit. At the end, the context of German space exploration activities to the German political strategy in reference to the exploration of the icy ocean worlds will be highlighted. Technology developed for future missions will be able to be transferred to disciplines related to polar-, ocean-, deep sea- and climate change-research to address fundamental scientific questions related to our home planet Earth.
The first enlightening visits of the giant planets and their moons by spacecraft (Pioneer 10/11 in 1973–1990, Voyager 1/2 in 1979–1989) led to an increasing interest to further investigate those planetary systems as key elements for understanding the Solar System as a whole. Measurements by Galileo in the Jovian (in 1995–2003) and Cassini-Huygens in the Saturnian system (in 2004–2017) revealed the existence of subsurface oceans under the ice shells of, for example, Jupiter’s moons Europa and Ganymede as well as Saturn’s moon Enceladus (e.g., Nimmo and Pappalardo, 2016). The Cassini-Huygens mission discovered a plume emanating from the south pole of Saturn’s moon Enceladus (Porco et al., 2006). This plume emerges from cracks in the ice shell and consequent studies revealed its composition to be mainly water vapor and ice particles originating from a subsurface ocean, with a salinity slightly lower than Earth’s oceans (Postberg et al., 2009). Finding analogue sites for extraterrestrial icy vents and plumes here on Earth is challenging due to the triple point conditions present inside the icy vents of Enceladus (Schmidt et al., 2008), derived from the drastic difference in pressure between the interior and exterior of the moon. Analogies to the environment near terrestrial, submarine hydrothermal vents, a potential site for the origin of life on the early Earth were striking and the icy moons became promising candidates for habitable worlds and prime targets for astrobiology investigations.
Alike Enceladus, Europa harbors a global subsurface liquid water ocean that is in contact with a rocky core (e.g., Kivelson et al., 2000). Ganymede’s subsurface ocean is probably sandwiched between two layers of ice (Vance et al., 2014; Saur et al., 2015), making material exchange between the liquid ocean and the core less likely. The same applies to Jupiter’s moon Callisto (Hartkorn and Saur, 2017). Saturn’s moon Titan has a liquid, likely stratified (Idini and Nimmo, 2024), water ocean underneath its organic-covered ice shell (Goossens et al., 2024). Neptune’s moon Triton is another, yet widely unexplored, ocean world and a compelling destination for future space mission (Frazier et al., 2020; Hansen et al., 2021).
Our current understanding of the oceans of the icy moons and the fascinating discoveries by spacecraft in the recent past (e.g., Enceladus’s plume) will be discussed in some detail in the following chapters. However, many questions could not be answered so far, which led to the development of numerous mission proposals to further investigate the oceans of the icy moons (e.g., Howell and Pappalardo, 2020; Barnes et al., 2021; Mousis et al., 2022).
After the end of the Cassini-Huygens mission in 2017, the flybys of Ganymede and Europa of the Juno spacecraft in 2022 and 2023, as part of its extended mission, are the only occasions of a spacecraft visiting ocean moons in the 2020s. This lack is partially mitigated by the spectacular observations of the James Webb Space Telescope of the moons Europa (Villanueva et al., 2023a; Trumbo and Brown, 2023) and Enceladus (Villanueva et al., 2023b). Although these observations yield better data than any other telescopic observation, the spatial resolution of the James Webb Space Telescope, even for the Jovian moons, is still four to five orders of magnitude below what can be achieved with a close flyby.
However, starting in 2030, ocean moons will become more important targets of both NASA’s and ESA’s space programs. In 2030 and 2031 respectively, two flagship missions - Europa Clipper (NASA) and the JUpiter Icy Moons Explorer (JUICE; ESA) - will arrive in the Jovian System to observe Europa, Ganymede, and Callisto as their prime targets. Both missions Europa Clipper (Howell and Pappalardo, 2020) and JUICE (Grasset et al., 2013) have significant German contributions, advancing the research in this field at German research facilities (Figure 1).
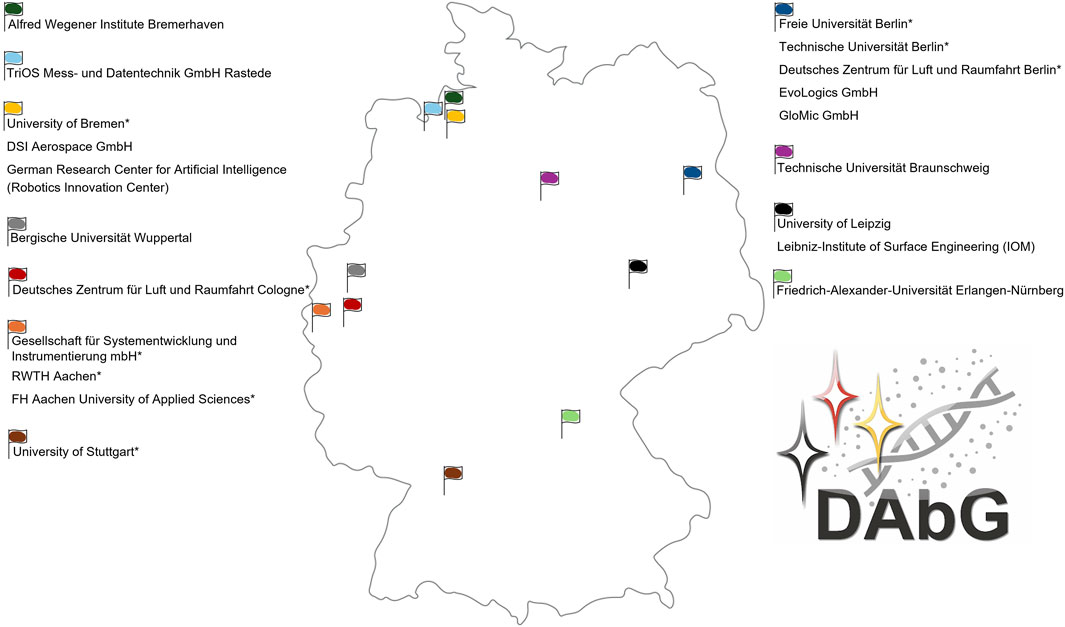
Figure 1. Locations of research groups in Germany with ongoing astrobiology research and collaborations related to icy ocean worlds. Research themes are summarized in Table 1. The German Astrobiology Society (Deutsche Astrobiologische Gesellschaft; DAbG) logo is shown on the bottom right. Institutions where DAbG members are currently located are marked with an asterisk.
Europa Clipper is scheduled for launch in October 2024 and has the overarching goal to constrain the habitability of Europa’s subsurface ocean with about 50 close flybys within the 4 years of its prime mission while in orbit around Jupiter (Vance et al., 2023). The locations of these flybys are all fixed in form of a flyby sequence, but a potential extended mission could allow for targeted flybys over regions that have turned out to be of specific interest for Europa’s habitability.
JUICE (launched 14 April 2023) has a somewhat broader scope. During the first part of the mission the spacecraft will stay in a Jovian orbit with two flybys of Europa and tens of flybys of each Callisto and Ganymede. In December 2034, ESA plans to go into Ganymede orbit for a detailed investigation of the largest moon in the Solar System. After up to 1 year in Ganymede orbit a crash onto the moon’s surface will then end the JUICE mission.
Titan will be visited by the spectacular Dragonfly mission in the mid 2030s. Currently, the launch of this NASA New Frontier class mission is foreseen in 2028 with an arrival in 2034. The spacecraft is a rotorcraft lander weighing about 450 kg, that - driven by radioisotope thermoelectric generators (RTG) – will sample the moon’s atmosphere and surface composition at different landing sites to assess Titan’s potentially prebiotic organic chemistry. The combination of low gravity and dense atmosphere allow the drone-like lander to investigate various sites and altitudes during its 3.5-year prime mission by flying distances of up to 100 km while climbing to altitudes of up to several kilometers (Barnes et al., 2023).
Beyond these approved missions, there are long term strategies of space agencies, most notably NASA, ESA and the China National Space Administration (CNSA), to investigate the giant planets and their icy moons. On the U.S. side, this is outlined in the Planetary Science and Astrobiology Decadal Survey 2023–2032 (National Academies of Sciences, Engineering, and Medicine, 2023) as well as in reports written by the Outer Planets Assessment Group (OPAG), established in 2004 (Beauchamp et al., 2009). The NASA competitive programs, Discovery and New Frontiers (NF), include the possibility to propose missions to icy ocean worlds. New Horizons (NF1) performed a flyby of dwarf planet Pluto. JUNO (NF2) is in orbit around Jupiter. The aforementioned Dragonfly has been selected as the fourth New Frontiers mission (Barnes et al., 2021). All those missions have relevance to icy ocean worlds exploration.
The Planetary Science and Astrobiology Decadal Survey 2023–2032 foresees up to three major missions in the near future. Firstly, the next New Frontiers mission (NF5) lists Enceladus as a potential target. Moreover, the next two flagship missions should be a Uranus orbiter and an Enceladus lander. Following the Orbilander concept (MacKenzie et al., 2021), the latter would be a mission that looks for life on Saturn’s active ocean moon with a planned arrival in the early 2050s. Already sometime earlier the Uranus orbiter would arrive and the exploration of the Uranian moons, some of which are potential ocean worlds, will certainly be part of the mission goals. ESA considers contributing to this Uranus mission in a similar fashion as it has been successfully implemented for the joint Cassini-Huygens mission where ESA provided the Huygens probe that landed on Titan in 2005 (Lebreton et al., 2005).
At ESA, the long-term priorities in space sciences are described in the Voyage 2050 report (Tacconi et al., 2021), which identifies a mission to “moons of the giant planets” as the fourth large mission (L4) in the science program (following JUICE, Athena and LISA) as well as a so-called “Inspirator Mission”, aiming for sample return from one of the icy moons (Rapley et al., 2022). With a launch date in the early 2040s, Enceladus is the most likely target for this L4 mission, as recently announced by ESA (Martins et al., 2024).
China is also planning to investigate the Jovian system with the Tianwen-4 mission (also referred to as Gan De, after a Chinese astronomer of the 4th century). As a possible launch date, 2029 has been announced (Lei et al., 2021). It is worth noting that currently there are no concrete plans to land on a Jovian satellite, and even less so to physically penetrate the ice crust. Although many ideas were addressed and the concept of a Europa lander mission was studied (Hand et al., 2022), this concept was not rated as a high-priority mission. Nevertheless, some astrobiological questions will only be answered by lander missions or melting/drilling probes. For example, limiting elements of life, such as iron, may be remotely detectable on the surface in flybys, but in situ analyses with a lander provides spatially better resolved and much more detailed and sensitive measurements. Other space agencies, e.g., Russia, Japan, or India, currently do not have plans for ocean moon exploration.
2 Activities in Germany - general overview
In the last decades there has been increasing activities in Germany to investigate icy ocean moons to realize future space missions to these worlds. DAbG Members (Figure 1; Table 1) are heavily involved in both missions to the Jovian system JUICE and Europa Clipper. Involvements include participations in various instrument teams, such as the Particle Environment Package-Neutral Ion Mass Spectrometer (PEP-NIM; Barabash et al., 2013), the JANUS multispectral camera (Della Corte et al., 2014) and the Ganymede Laser Altimeter GALA (Enya et al., 2022) for JUICE as well as the SUrface Dust Analyzer (SUDA) for Europa Clipper (Kempf et al., 2024).
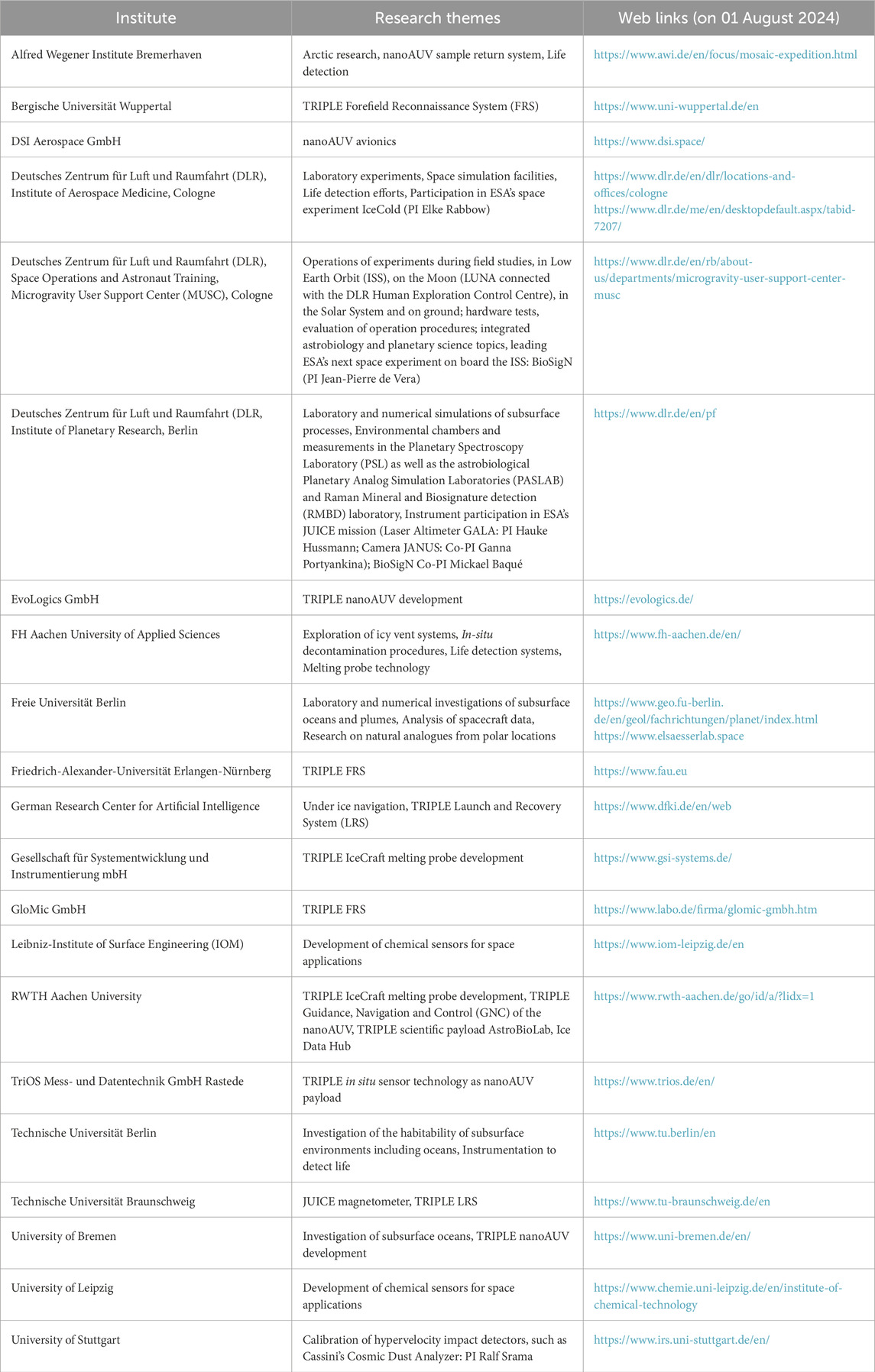
Table 1. Institutes in Germany (see Figure 1) and their icy ocean world research themes.
2.1 Explorer Initiatives at the German Aerospace Center
Since 2012, the Explorer Initiatives at the German Aerospace Center (DLR) have been supporting universities, research institutions and commercial companies from all over Germany in specially created project lines. The aim of these project lines is to develop innovative technologies to enable future space missions to astrobiologically interesting celestial bodies in our Solar System (Funke and Horneck, 2018). Each project line is based on a central, albeit currently fictitious, mission scenario:
In the EnEx initiative (“EnEx - Enceladus Explorer”; Kowalski et al., 2016), technologies are being developed for taking a H2O sample from a vent on Saturn’s moon Enceladus. The sample can be taken without completely melting through the moon’s outer ice shield, which is several kilometers thick.
Jupiter’s moon Europa is also receiving special attention, here in the project lines “EurEx - Europa Explorer”, and “TRIPLE - Technologies for Rapid Ice Penetration and subglacial Lake Exploration”:
In EurEx, an autonomous underwater vehicle (AUV) is being developed to explore the seabed of the deep global ocean on Europa completely independently (Hildebrandt et al., 2022). The challenges are enormous, particularly in terms of the AI required for this task, and successful implementation is not expected before the middle of this century.
While EurEx has a long-term focus, the TRIPLE project line has a medium-term objective (Waldmann and Funke, 2020): The plan is to develop an AUV that is even more miniaturized than the EurEx AUV. This nanoAUV is designed as a payload for a melting probe, which is designed to penetrate the ice sheet of Europa into the global ocean below (see also Section 3). The melting probe will be anchored in the ice at the point of entry into the ocean and will serve as a base to ensure communication to the surface and from there to Earth. The nanoAUV payload will then be deployed into the ocean as a mobile unit and will be used for exploration within a radius of approximately 100 m around the base. The nanoAUV will also be used to take samples from the bottom side of the ice and bring these samples to the melting probe for further analysis within the AstroBioLab, which is another payload of the probe. It is planned to demonstrate the technological readiness of the complete TRIPLE system consisting of three major parts: The melting probe TRIPLE-IceCraft (Figure 2), the mobile exploration unit TRIPLE-nanoAUV and the TRIPLE-AstroBioLab.
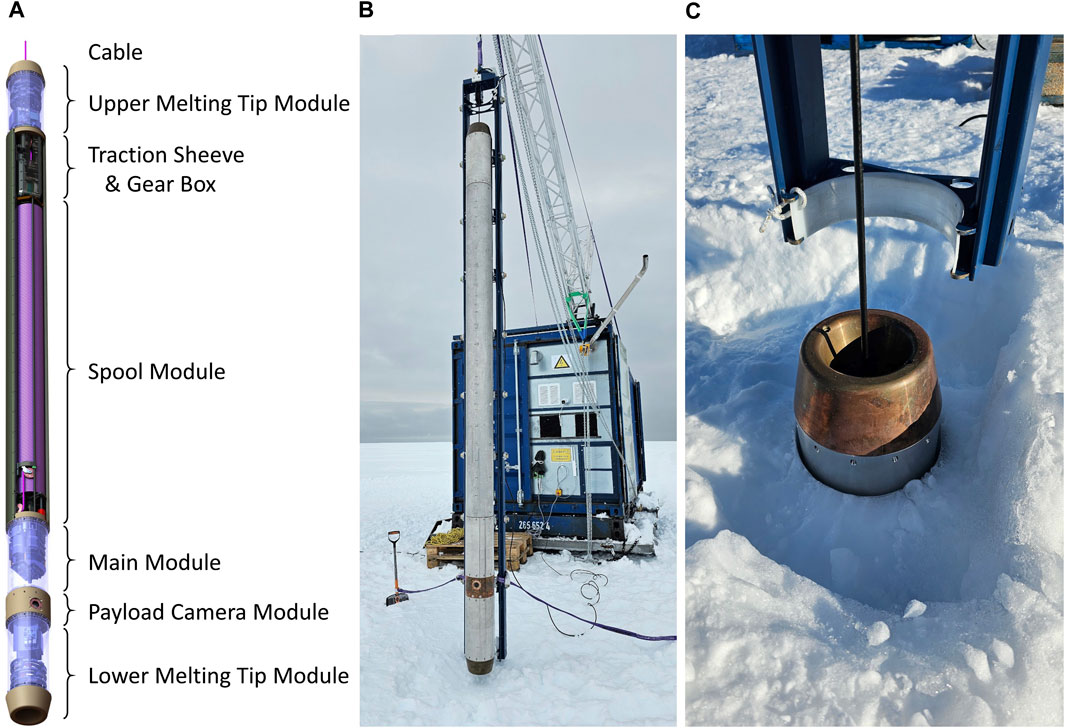
Figure 2. TRIPLE-IceCraft melting probe. (A) Technical drawing showing the different parts of the melting probe, which is 4 m in length and 20 cm in diameter. (B) and (C) Photographs of the melting probe during a test campaign on the Ekström Ice Shelf, Antarctica in 2023/2024. (C) shows the Upper Melting Tip Module and the cable after the probe melted through the ice.
These three main components are designed to work together as consecutive stages of the TRIPLE comprehensive exploration strategy. With its state-of-the-art instrumentation, the AstroBioLab serves as the portable analytical field hub for analyzing samples previously collected under the ice and transported to the surface.
2.2 The AstroBioLab
The AstroBioLab analytical pipeline consists of four modalities: (a) fluorescence spectroscopy, (b) fluorescence microscopy, (c) DNA sequencing and (d) mass spectrometry interconnected by a sophisticated microfluidics system.
Fluorescence spectroscopy (a), especially when coupled with Chromophoric Dissolved Organic Matter (CDOM) analysis principles, emerges as a powerful tool in astrobiology, particularly for scrutinizing extraterrestrial environments or seeking signs of life (Barker et al., 2009; Smith et al., 2018). Unique combinations of fluorescence intensity, peak shapes and exact positions produce characteristic spectral patterns that shed light on CDOM’s composition, concentration and even its origin. Extensive testing in Arctic ice, Antarctic lakes and deep-sea hydrothermal vents has demonstrated the effectiveness of fluorescence spectroscopy in studying complex aquatic ecosystems and in detecting organic compounds under conditions analogous to those found on other planets (Storrie-Lombardi and Sattler, 2009). For instance, fluorescence analysis of water samples from Antarctic lakes revealed significant photosynthetic and biodegradation activities, (De Laurentiis et al., 2013). To meet the future mission requirements regarding miniaturization, energy consumption, robustness and easy handling, a semi-automatic fluorimeter module has been designed and manufactured by the FH Aachen University of Applied Sciences and GSI mbH (Figure 3).
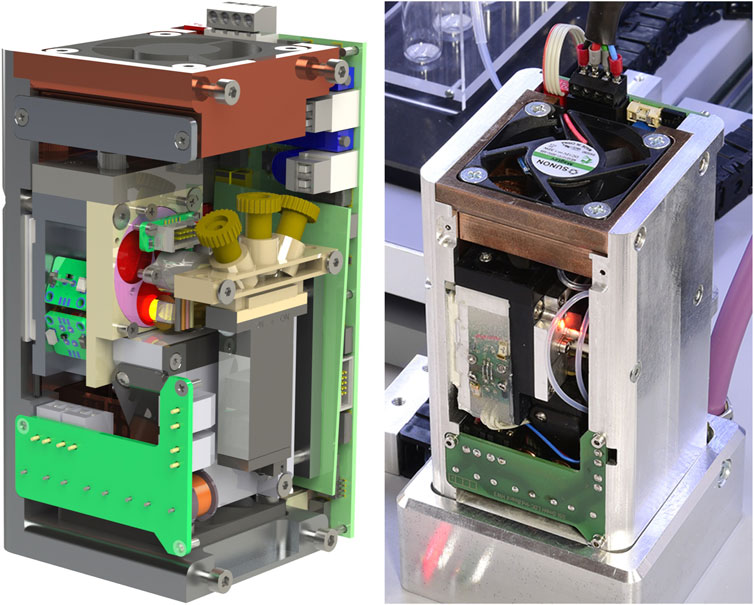
Figure 3. Computer Aided Design (CAD; left) and photograph (right) of a miniaturized UV-fluorescence spectrometer.
Fluorescence microscopy (b) will enable direct visualization of particulate matter, ranging from submicron to submillimeter size (e.g., Mulyukin et al., 2014). Studies in Earth’s polyextreme environments like the Atacama Desert have shown the potential of this technology in visualizing and identifying microbial life forms in various microhabitats (Wierzchos et al., 2018). In future field missions, miniaturized automated fluorescence microscopy systems such as OpenFlexure (which can be 3D printed), can be deployed for in situ exploration, thereby diminishing the necessity for sample return. Furthermore, employing advanced fluorescence techniques, such as lifetime imaging microscopy, may provide additional insights into the microenvironment of (extra) terrestrial water samples (Nadeau et al., 2016).
DNA sequencing (c) is yet another valuable tool for exploring icy ocean habitats (e.g., Carré et al., 2024). Field tests in locations like Antarctica’s subglacial lakes and Canadian high arctic permafrost ice wedge have validated the feasibility of using portable sequencers in remote and harsh environments (Goordial et al., 2017). Nanopore sequencers, exemplified by the Oxford Nanopore MinION, are available as extremely compact devices for environmental water sampling (Li et al., 2023). However, further progress must be made in automatization of real-time DNA sequencing where a critical step remains in the preparation of a DNA library, which currently needs to be performed manually.
The mass spectrometry unit (d) will provide crucial information of elemental and molecular composition of the sample (O'Donnell et al., 2016). Criteria related to compactness, energy efficiency and robustness define the range of the available options. Currently we consider the portable field mass spectrometer EcoSys-P (ESS Ltd., Ireland) to be a promising system.
Obvious challenges to address include extremely low temperatures, broad magnitudes of pressures, limited available power, as well as limitations in space and weight. All this demands comprehensive interdisciplinary collaboration, coupled with advanced engineering and AI solutions.
The scientific payload is planned to be tested by the end of this decade in a major terrestrial analog scenario field campaign at the Dome C region in Antarctica: There, the IceCraft (Figure 2) has to melt through ∼3.3 km of ice, avoiding any possible obstacles within its vertical trajectory by a specially designed forefield reconnaissance system (implemented into the melting head), and to finally get access to a subglacial lake that has been hermetically sealed from the environment for about 1 million years.
2.3 Beyond the Explorer Initiatives
In addition to these specific technological developments, laboratory setups and sophisticated computer simulations are being designed in Germany to help understand processes on icy ocean worlds and inform instruments on board space missions (Section 4). Other projects in Germany include, for example, at DLR and the Berlin universities, research on organics, biosignature detection and extremophiles (Sections 5, 6). Instruments on board space missions are calibrated in laboratory experiments at, for example,, Freie Universität Berlin and University of Stuttgart.
Another Recent development is the formation of the international team “Bridging the Gap: From Terrestrial to Icy Moons Cryospheres” sponsored by the International Space Science Institute (ISSI) in Bern. The team is led by researchers from German institutions and investigates active processes on icy moons with the aim of bridging the temporal and spatial scales between terrestrial and extraterrestrial cryospheres (Kowalski et al., 2024).
3 Technology and sensor developments
Accessing ocean worlds is not trivial but crucial for in situ astrobiological investigations. The water reservoirs of the currently known ocean worlds like Europa or Enceladus are situated below thick icy crusts. In fact, a recent study suggests that Europa’s ice shell may still be growing (Shibley and Goodman, 2024).
While Ground-Penetrating-Radar is useful to better understand the icy shell and underlying oceans of Solar System bodies (e.g., Heggy et al., 2017), yet nobody drilled or melted through an icy moon’s shell to gather in situ information from the ocean water. Technology to penetrate the ice is, hence, a key technology for future missions. Thermal melting seems to be a promising technology to achieve this task due to its mechanical simplicity and robustness (Dachwald et al., 2023). Also, thermal melting probes can be operated autonomously, and the removed ice is transported as meltwater to the back of the thermal drill. In contrast, mechanical drilling requires less energy (Rinaldi et al., 1990), but it faces the issue that cut ice chips must be mechanically transported to the back of a mechanical drill. Whatever penetration method would be used, considerable infrastructure on the surface and high energy supply will be required (Dachwald et al., 2020).
The discovery of a plume at the south pole of Enceladus that is sourced from a liquid water ocean in the early 2000s (Hansen et al., 2006; Porco et al., 2006) inspired the development of space mission concepts that include thermal melting probes (Konstantinidis et al., 2015) and the development of new types of melting probes (Dachwald et al., 2020). Activities in Germany are mainly organized within the Explorer Initiatives led by the DLR (Section 2.1) and include the EnEx-IceMole concept that is maneuverable due to its ice screw and differential heating (Dachwald et al., 2014; Kowalski et al., 2016; Baader et al., 2024), tiny laboratory scale probes (Baader et al., 2016), and small helper probes for localization (Weinstock et al., 2021). Another probe is the large TRIPLE-IceCraft (Heinen et al., 2021; see Section 2.1) that will allow to carry small autonomous underwater vehicles (TRIPLE-nanoAUV) as a payload (Waldmann and Funke, 2020). To describe the trajectories of such probes, modeling efforts are ongoing (Schüller and Kowalski, 2019; Boxberg et al., 2023). A major success was the use of the EnEx-IceMole to retrieve clean samples from a water reservoir that feeds the Blood Falls at Taylor Glacier in Antarctica in November 2014 (Kowalski et al., 2016; Lyons et al., 2019; Mikucki et al., 2023). However, the probe only melted through about 17 m of ice to reach the subglacial water. Although the thicknesses of the ice layers on top of the icy ocean worlds are not yet well constrained, it is very likely that melting probes must overcome ice layers of several kilometers. Therefore, current developments like the TRIPLE-IceCraft are focusing on increasing the range and the level of autonomy of such melting probes and establishing a standardized interface to enable an easy integration of arbitrary scientific payloads or sensors. A first polar test of the TRIPLE-IceCraft was performed on the shelf-ice close to the German Neumayer Station III in Antarctica in 2023. One of the main goals of the current TRIPLE project (Figure 2) is to develop a melting probe that is able to penetrate ice with a thickness of 3–4 km, which is the maximum thickness available in analogue missions on Earth, at Dome C in Antarctica. Within the same project, the nanoAUV is developed for autonomous investigation of subglacial water reservoirs (Waldmann and Funke, 2020). Tests in partly frozen lakes and below shelf-ice are planned.
Recent improvements in onboard computing capacities and power storage used within AUVs is allowing far more detailed studies of environmental conditions and flora and fauna distributions within the oceans of Earth and beneath the permanent ice caps of both poles. Societal interest in global environmental changes is driving some of these developments (Benway et al., 2019).
There is also industrial interest in studying remote, high pressure and variable temperature locations such as deep-sea polymetallic nodule fields and hydrothermal provinces. The application of these sensor systems into high pressure platforms (both automated and tethered) for use in the underice environments is ongoing. Permanent underice monitoring systems such as the F/Photometric Robotic Atmospheric Monitor (FRAM) observatory network combines traditional “Conductivity, Temperature and Depth” sensors, cameras and direct sampling with these new systems to inform on life below the ice – from the microscopic bacterial life to megafauna – as well as to monitor the ongoing effects of climate change in these underice environments.
Promising techniques for in situ analysis of the water ice and accompanying compounds include spectroscopic techniques, such as Laser Induced Breakdown Spectroscopy (LIBS) and Raman spectroscopy. Both methods rely on the use of a laser and detect the light of a laser-induced micro plasma for elemental analysis (LIBS) or inelastically backscattered laser light for information about molecules and lattices (Raman). They are thus greatly complementary in giving information about the elemental and molecular compositions of targeted samples. Both methods have the advantage of requiring only optical access to the target of interest with rapid data acquisition and are developed at DLR with a focus on extraterrestrial applications including the analysis of ices (e.g., Pavlov et al., 2011; Schröder et al., 2013; Böttger et al., 2017; Hagelschuer et al., 2022). Salts as well as other inorganic compounds, minerals, but also organics and complex biomolecules can be detected and identified, even after space exposure (Baqué et al., 2022). The instruments could serve as payload for robots investigating the surface of an icy moon or be integrated into systems exploring the subsurface. For example, the Raman spectrometer RAX that was developed for the small Martian Moons eXploration (MMX) rover weighs only 1.5 kg in a volume of about 10 dm3 (Hagelschuer et al., 2022) and LIBS instruments of similar weight are currently under development (Rapin et al., 2023).
4 Analogues, experimental and numerical simulations in the field, laboratory, and space
4.1 Field analogues
Cassini’s measurements revealed geochemical evidence for hydrothermal vents at Enceladus’s ocean floor (Hsu et al., 2015; Waite et al., 2017). Terrestrial analogues sites for extraterrestrial hydrothermal systems include deep sea vents around mid-ocean ridges (e.g., Lost City in the Atlantic Ocean; Kelley et al., 2005), arcs (e.g., Tonga-Kermadec and Le Havre-Lau back arc system; Smith and Price, 2006), and hot spots in volcanic active areas (e.g., Iceland).
The icy shells of several icy satellites, such as Europa, Enceladus and Ganymede are heavily affected by brittle and ductile deformation (e.g., Collins et al., 2010). The analysis of the resulting structural patterns is essential for understanding the driving forces, such as tidally-induced stresses (Tufts et al., 1991; Hoppa et al., 2000). Terrestrial analogues for tectonic deformation of ice volumes support the study of icy satellites and include mountain glaciers, ice caps, and marine ice shelves (e.g., Blankenship and Morse, 2004). Of particular interest are faults and fractures as pathways for ascending fluids which could deliver biosignatures from liquid water reservoirs within the icy shells to the surface to make these signatures available for spacecraft analysis.
The surfaces of icy ocean worlds are exposed to high doses of radiation (Cassidy et al., 2021). The epitome of this is the tidally locked moon Europa, where the leading and trailing hemispheres of the moon present stark contrast due to the differences in surface irradiation. Terrestrial analogues to icy moon’s surfaces include the Greenland Ice Sheet, maritime and continental Antarctica (e.g., McMurdo Ice Shelf, Deception island and the South Pole) and altitude glaciers found in several mountain ranges (e.g., Southern Ice Field in Patagonia and Andean or Himalayan glaciers).
The interaction between ice and the waterbed can be studied through terrestrial analogue sites. Subglacial lakes such as Lake Untersee (Eastern Antarctica) present anoxic, methane rich, stratified waters. Similar lakes, such as Lake Vida (McMurdo Dry Valleys) or Lake Vostok (East Antarctic ice sheet) are also considered important analogues. Lastly, Gypsum Hill Springs in Axel Heiberg Island (Canadian Arctic) is adjacent to highly saline ice, rich in perennial saline springs (Heldmann et al., 2005), and is relevant for studying both Enceladus and Europa.
Field tests are not only valuable for testing drilling and melting probes, such as the TRIPLE-IceCraft (see Sections 2.1, 3), but also for studying the compositions, including organics and microbes, of sites analogous to icy ocean worlds. The Planetary Sciences & Remote Sensing group at Freie Universität Berlin recently conducted icy moon analogue research in coastal Antarctica, with a field campaign at the beginning of 2024 (Hortal Sánchez et al., 2024) carried out in collaboration with the Centro de Astrobiología in Spain and the Instituto Antártico Uruguayo. The goal was to retrieve icy samples in King George Island, in the South Shetlands islands in the Antarctica peninsula. Samples were collected in the Southwestern region of Collins Glacier (a.k.a Bellingshausen Glacier), at four sampling points: by the seaside, at the dome, by the land front and in cryoconite pockets. Cryoconite pockets are of special interest due to their high organic content. They usually form during summer due to the lower albedo of the accumulated dark dust (i.e., soot, ash and rock particles), and the resulting higher temperature that melts the glacier ice directly under it. Alpine and McMurdo Dry Valley glaciers with cryoconite surfaces and holes have proven to be unique niches for psychrophiles (Porazinska et al., 2004; Margesin et al., 2012). Deposition of micrometeorites on the surface of icy moons may create similar conditions for putative life-forms ejected from the subsurface oceans of these moons.
At the sampling site by the seaside, deposition of ocean-borne aerosols is expected. The action of wind on the sea around polar regions on Earth creates sub-micron aerosols: ice particles that are organic-rich and salt-poor (Burrows et al., 2014). These organic-laden ice particles are similar in composition to the organic rich Type II ice grains found in Enceladus plume (Postberg et al., 2018). The deposition of these ice particles on the glacier’s surface and subsequent sampling presents an excellent analogue for the study of ice grains from icy ocean worlds.
Sampling carried out at the dome of the glacier as well as by the land front could present interesting cases for organic-poor ices and organic-rich ices, respectively, the latter having a bigger organic input from non-microbial species (e.g., algae). This expected difference in concentration of the target analyte allows further assessment on the limitations and capabilities of spaceborne instruments on board future space missions to icy ocean moons.
The collected samples include both deep ice cores (Figure 4) and surface ice. They will be analyzed with a range of analytical techniques to evaluate the organic content and presence of biosignatures that could be detected with spaceborne instrumentation on board missions to icy moons (e.g., Klenner et al., 2019). A detailed comparison of the data obtained by the different analytical techniques will provide analogue data for the detections of biosignatures by combining several instruments onboard spacecraft.
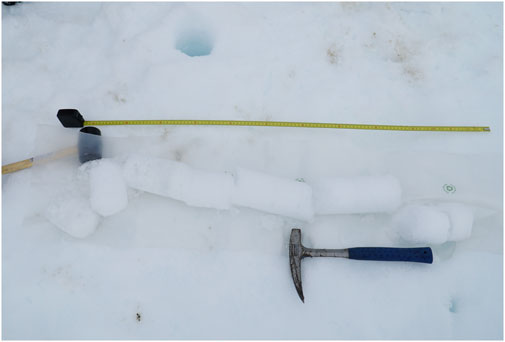
Figure 4. Ice core collected at the dome of Collins glacier by the Planetary Sciences & Remote Sensing group at Freie Universität Berlin at the beginning of 2024.
4.2 Experimental studies
Laboratory-based simulations complement our understanding of icy ocean worlds, their interaction with different radiation fields and allow us to examine specific chemical reaction pathways in much more detail. Some experimental setups are based on an ultra-high vacuum (UHV) chamber that is equipped with a cryostat and linked to radiation sources that provide X-rays, UV photons, or particle radiation. Ice analog samples are created via background condensation of gas-phase molecules on suitable substrates. For example, one such facility is operated at Freie Universität Berlin, where various ice mixtures on top of organic layers are irradiated using either an electron gun, a solar simulator, or both simultaneously. Detailed analysis of the samples is performed using Fourier-transform infrared (FT-IR) transmission spectroscopy and mass spectrometry, which are available both during the irradiation process and the warming-up process.
Another setup, newly built at the Planetary Spectroscopy Laboratory at DLR Berlin, aims at performing bi-directional reflectance measurements under cryogenic conditions to support current and upcoming missions to the outer Solar System, including ESA’s JUICE and NASA’s Lucy. The setup allows for measurements from UV to far infrared for up to four samples at temperatures lower than −150°C under vacuum down to 10−6 hPa (Helbert et al., 2023).
A setup (PI Nozair Khawaja) is being designed that can simulate the conditions in the only known extraterrestrial hydrothermal vent system, i.e., Enceladus seafloor (Figure 5). A detailed hydrothermal experimental plan is made to process several species in this reactor to investigate the effects of hydrothermal processing of compounds before incorporation in ice grains (Khawaja et al., 2024a). DAbG members at US institutions are involved in experimental studies that address the formation of these ice grains (Klenner et al., 2024a) as well as fractionation processes occurring upon eruption of an ocean world plume (Fifer et al., 2024).
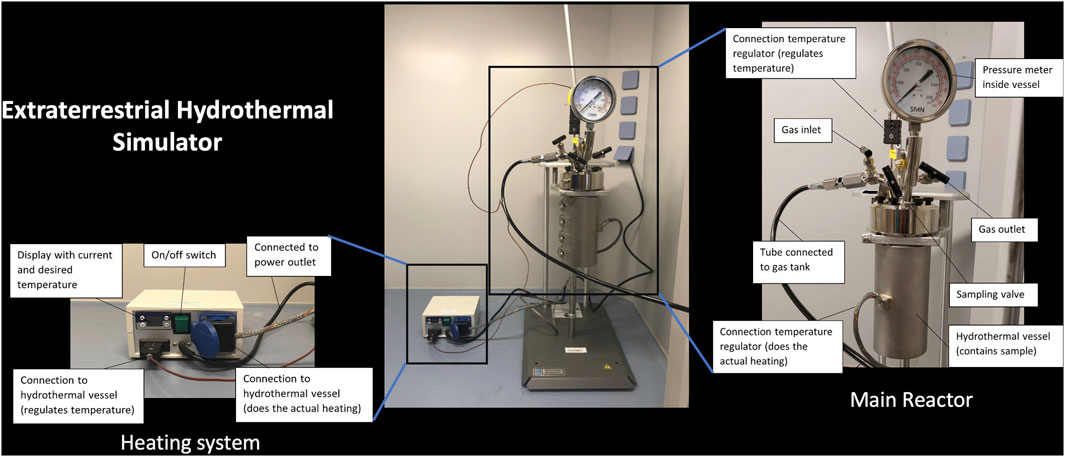
Figure 5. Experimental hydrothermal reactor used to simulate the conditions in Enceladus’s interior. More information about this setup can be found in Khawaja et al. (2024a)
The analysis of ice grains in space can be achieved by impact ionization mass spectrometers, such as SUDA on board Europa Clipper (Kempf et al., 2024). However, a detailed analysis of ice grain mass spectra requires laser-based analogue experiments conducted at Freie Universität Berlin (Klenner et al., 2019; 2022; Sanderink et al., 2023). This approach has successfully been used to analyze ice grain mass spectra recorded by Cassini (e.g., Postberg et al., 2018; Postberg et al., 2023) and predict mass spectra of ice grains containing molecules essential for the emergence of water- and carbon-based life, such as amino acids (Klenner et al., 2020a). Mass spectral data from impact ionization instruments can also be simulated using dust accelerators in a laboratory environment or quantum chemistry techniques in computer simulations (O’Sullivan et al., 2024).
However, the duration of particle exposure to the space environment before being analyzed can have an influence on their composition and on the preservation and detectability of complex organic molecules as potential traces of life. Laboratory-based simulations of certain space factors are necessary but generally not enough to fully recreate a complex space environment, especially in terms of radiation. Thus, several exposure experiments involving space platforms have been conducted by space agencies since humanity’s presence in Low Earth Orbit (LEO) and beyond (Horneck et al., 2010). Among the latest ones, ESA’s EXPOSE platform allowed a long-term exposure (12–18 months) in LEO of biological and chemical samples outside the International Space Station (ISS). It completed its third and final mission called EXPOSE-R2 in 2016 (Rabbow et al., 2017). New active and passive exposure experiments in LEO are currently being developed and prepared for flight by ESA and partners: the new payload EXPO on the Bartholomeo platform will include several experiments dedicated in part to the exposure of ice particles and their components: BioSigN (BioSignatures and habitable Niches), IceCold, OREOcube (ORganics Exposure in Orbit cube) and ExoCube (Exposure of organics/organisms cube). On these platforms, in situ analytical methods (including spectroscopy) are crucial tools for understanding photochemical and radiation-biological processes for studying organics and potential biosignatures. Simulating icy moon conditions through space experiments presents a challenge, particularly in maintaining low temperatures throughout the space exposure phase. New concepts for low-temperature platforms that enable irradiation and exposure of samples to space conditions exist (Cottin et al., 2022) but face implementation challenges.
4.3 Numerical simulations
Another important aspect in assessing the habitability of icy ocean worlds is to gain further understanding of the subsurface environment on a large scale. The cryo-subsurface environment includes the uppermost ice crust, the ocean as well as high-pressure ice layers. Whether an ocean or high-pressure ice layers are likely to occur depends on the individual icy moon (see review in Soderlund et al., 2020). Numerical models predicting the interior structure (e.g., Sohl et al., 2002) are based on observational constraints (e.g., the bodies mass, the moment of inertia factor or the tidal love number) and high-pressure experiments to investigate the material properties at high pressures and temperatures. The presence of liquids in these layers and the exchange of heat and material between them to address the overall habitability is subject to ongoing studies.
The uppermost ice crust may contain salt-rich liquids (brine) in the form of, for example, small subsurface lakes (e.g., Schmidt et al., 2011), cracks (e.g., Rudolph et al., 2022) or interstitial water at grain boundaries (e.g., Wolfenbarger et al., 2022). Salt is an essential ingredient in these cryo-environments as it efficiently decreases the melting temperature and thus prevents liquids from freezing in these otherwise extremely cold conditions. Liquid inclusions may originate from the ocean beneath the ice crust (e.g., Soderlund et al., 2020).
Recent modeling attempts address the uptake of salt-enriched liquids at the ice-ocean interface by investigating the physics of a mushy layer between the (mainly) solid ice crust and the ocean (Buffo et al., 2020; Buffo et al., 2021). A connected and emerging field of research is the interplay of the dynamics of the meso-scale mushy layer and the large-scale ice crust. Coupled models, developed by DLR scientists, are able to tell whether liquids trapped at the ice-ocean interface can move within the ice crust and remain liquid over longer periods of time (Myrs to Gyrs; Rückriemen-Bez et al., 2023). Other ways to create liquids are local melting of the ice crust due to heat sources such as tidal heating in the case of Europa (Sotin et al., 2009) and Enceladus (Spencer and Nimmo, 2013) or heating by impacts (Cox and Bauer, 2015). In both cases, large-scale models of the ice shell - being developed now - can investigate how the associated heat anomalies and liquid fractions are evolving, which ultimately provides an estimate of the lifecycle of habitable niches in the ice shell.
The ocean is of particular interest when the ocean floor is expected to be in direct contact with rocks (see Sections 4.1, 5). The suspected aqueous alteration of silicates is thought to be responsible for most of the ocean’s salinity and organic molecules (Zolotov and Shock, 2001; Zolotov and Kargel, 2009). Fluid motions in the ocean influence the degree of mixing of these salty and organic compounds, and thus the distribution of chemical and thermal gradients. Numerical studies of ocean dynamics cover topics such as global circulation (e.g., Soderlund, 2019; Terra-Nova et al., 2023) and ocean tides (e.g., Matsuyama et al., 2018; Rovira-Navarro et al., 2019).
Especially for larger icy moons such as Ganymede, Callisto or Titan, high-pressure ice layers may occur above the rocky core of the moons effectively shielding any potential ocean from being in direct contact with rocks (Journaux et al., 2020). In these scenarios it is not immediately clear if the ocean can be continuously fueled with salts and organics, which is crucial for creating a habitable environment. A primary interest is thus studying the transport of heat and material across the high-pressure ice layers. Recent models have investigated whether (and at which rate) liquids emerging at the interface between the high-pressure ice and the rocky core are released into the overlying ocean (Choblet et al., 2017; Kalousová and Sotin, 2018; Lebec et al., 2024). Phenomena like magmatic volcanism originating from the rocky core and its effect on the ocean as well as the high-pressure ice layers have only recently come into focus (Bland and Elder, 2022; Kervazo et al., 2022).
Currently, in Germany, individual modeling efforts focus mostly on Europa and Enceladus. These efforts are being undertaken at the Freie Universität Berlin (e.g., Matteoni et al., 2023; Schmidt et al., 2024), University of Münster (e.g., Wong et al., 2022), University of Braunschweig (e.g., Gundlach et al., 2018), and at the DLR in Berlin (e.g., Plesa et al., 2024) in collaboration with RWTH Aachen (Rückriemen-Bez et al., 2022) and with the University of Münster (Holm et al., 2024). Future research needs to combine different modeling strategies to tackle overarching research topics that may be addressed in large collaborative projects. A crucial part of future planetary ocean models should be the influence of boundaries (ice shell at the top, rocky core/high-pressure ice at the bottom) on the ocean and vice versa.
5 Organics and detection of biosignatures
Besides the presence of liquid water, chemical disequilibria, available energy and organic molecules are important conditions and ingredients of life as we know it. Water is an excellent medium to initiate and facilitate biochemical reactions as well as nutrient transport, important for originating and sustaining life. Therefore, the discovery of subsurface liquid water oceans on icy moons triggered the search for organics and biosignatures in recent decades. DAbG members are involved in these efforts through various international projects and play a significant role in developing a systematic way to life detection by combining laboratory and field work with Low Earth Orbit (LEO) experiments (de Vera, 2019; de Vera et al., 2019; Baqué et al., 2022).
Organic and inorganic material can be leached from a rocky core into the subsurface ocean through water-mineral interactions at the seafloor. On Enceladus, such interactions may even take place throughout the whole rocky interior because of the relatively high porosity of the core (Kisvárdai et al., 2023). Further heat and chemical diversity could be provided through serpentinization reactions at the seafloor (e.g., Farkas-Takács et al., 2022).
Active cryovolcanism on Enceladus (Porco et al., 2006), and potentially Europa (e.g., Sparks et al., 2017; Bradák et al., 2023), provide a means of making material from the subsurface water ocean accessible for in situ analyses by spacecraft. On Titan, organic surface material may be delivered to the subsurface water ocean through impact cratering (Neish et al., 2024).
With the instruments already sent to icy moons (e.g., Srama et al., 2004), a large variety of organic compounds could be found in Enceladus’s ocean by the analysis of mass spectra of emitted ice grains (Postberg et al., 2018; Khawaja et al., 2019). Some of these organics could potentially act as amino acid precursors. Cassini’s data recently revealed more organics emerging from the subsurface ocean of Enceladus. These organics suggest an alternative pathway for organic synthesis that could lead to the formation of more complex organics, such as Polycyclic Aromatic Hydrocarbons (PAHSs) (Khawaja et al., 2024b). Because of these discoveries, many laboratory efforts are ongoing to better understand the processing and detectability of organics, including potential biosignatures (Malaterre et al., 2023), on icy ocean worlds (e.g., Klenner et al., 2020a; Klenner et al., 2020b; Napoleoni et al., 2023a; Napoleoni et al., 2023b; Dannenmann et al., 2023; Khawaja et al., 2023). The results of these experiments show that many organics and biosignatures, including biotic fatty acid abundance patterns or DNA molecules, will be identifiable with future spaceborne instruments, such as SUDA (Kempf et al., 2024) or the High Ice Flux Instrument (Mousis et al., 2022), thereby advancing the search for life in the Solar System. Moreover, methods are being developed to combine data from different analytical techniques in a complementary way to elucidate the composition of unknown organic species emerging from extraterrestrial oceans (Khawaja et al., 2022). Such laboratory investigations are primordial to guide future space missions and make recommendations for the detection of organic molecules or even life. These recommendations are particularly relevant to Europa Clipper (Pappalardo et al., 2024) or potential future Enceladus missions (Cable et al., 2021; Mousis et al., 2022).
In addition to terrestrial laboratory calibrations, experiments conducted on the International Space Station (ISS) involve organic molecules that are exposed to cosmic radiation. Several DAbG members are actively planning these experiments to support the exploration of Enceladus, Europa, Mars and beyond (e.g., Elsaesser et al., 2023).
6 Extremophile research
Icy ocean worlds present a potential abode for habitability, but their environments are challenging for the origin and persistence of life. Jupiter’s icy moons, such as Europa and Ganymede but also Saturn’s satellite Enceladus are subject to huge amounts of radiation on their surface, which could alter potential biomolecules that originate from their interiors. Not much is known about the environment within the subsurfaces of icy moons, but sub-zero temperatures, high pressure, low light levels, and probably high salt concentration beneath the ice crusts could be inferred (e.g., Marusiak et al., 2021). The thriving microbial life discovered in some of the most extreme environments on Earth serves as a potential analogue for habitats on these moons, particularly deep-sea hydrothermal vents (McCliment et al., 2006) and zones of accumulated sea water beneath and within Antarctic ice (Garrison, 1991), suggesting that life may also be able to exist under similar conditions in the subsurface oceans of some of the icy moons (Russel et al., 2017; Martin and McMinn, 2018; Weber et al., 2023). Hypothetical models of organisms that might live under the ice crusts of the icy moons have been advanced (e.g., Schulze-Makuch and Irwin, 2002). However, a special emphasis has been the study of polyextremophiles, which are adapted to thrive under multiple environmental stresses and serve as useful models for studying the limits of life. They provide valuable insights into the potential habitability of extreme environments, on both Earth and beyond (Thombre et al., 2020). These environments are also of special interest to the study of the origin of life. Can life originate under a hydrothermal vent scenario as has been suggested by multiple authors for Earth (e.g., Martin et al., 2008) or are land areas required for an origin of life as suggested by other authors (e.g., Schulze-Makuch and Irwin, 2018; Damer and Deamer, 2020; Toner and Catling, 2020; Haas et al., 2024)? Extremophile research is a rapidly growing field involving DAbG members from diverse disciplines, including microbiology, biochemistry, geology, and planetary sciences (e.g., Klenner et al., 2024b).
Recent advancements in technology have significantly improved our ability to study extremophiles (e.g., Caro-Astorga et al., 2024). Innovations in deep-sea robotics, high-pressure cultivation techniques, and advanced/new molecular biology tools are changing how we approach this research. These technologies not only allow for a deeper understanding of extremophiles but also equip us with tools for the search for potential life in space, especially on icy ocean moons.
The study of extremophiles is essential in astrobiology. By studying and comprehending how life adapts and thrives in Earth’s most extreme conditions, we can better speculate about life’s potential beyond Earth. This knowledge influences the design of future space missions, including the development of instruments capable of detecting life (e.g., Klenner et al., 2024b) and understanding its biochemistry.
7 Cleanliness and decontamination controls
In exploring icy ocean moons, we also face ethical issues and challenges. One major concern is the possibility of contaminating other celestial bodies with Earth’s life forms (forward contamination) or bringing extraterrestrial organisms to Earth (backward contamination; e.g., Rettberg et al., 2019). This situation requires strict procedures to ensure a balance between scientific exploration and protecting potential ecosystems. The equipment that may touch pristine icy moon environments must be cleaned and verified. Applying a rigorous witness plan, for example, by using witness plates, provides a possibility to passively record the contamination level during the fabrication or testing of spacecraft instrumentation (e.g., Weissbrodt et al., 1994).
Novel thermoelectric melt probes such as the EnEx-IceMole, developed with funding from the German Federal Ministry for Economic Affairs and Energy (BMWi) can be specifically designed and optimized for (self)cleaning (Dachwald et al., 2014; Kowalski et al., 2016). They utilize no fuel, have a significantly smaller logistical footprint, and therefore offer potentially cleaner means of accessing the subglacial environment than many alternative ice penetration approaches.
The measures required for mitigation of contamination risks can be broadly divided into two groups, that should go hand-in-hand: (I) a complex of assays for contamination monitoring and assessment and (II) a set of procedures aimed at biological and chemical burden reduction. In terms of biological or organic contamination, celestial environments are under protection by the guiding principles of the Planetary Protection Policy (Kminek et al., 2020).
Many aspects and components of the Planetary Protection Policy are reflected in further official standards regulating exploration of polar terrestrial regions. This includes the Protocol on Environmental Protection within the Antarctic Treaty, and the Code of Conduct (CoC) for the Exploration and Research of Subglacial Aquatic Environments (Doran und Vincent, 2011; Siegert und Kennicutt, 2018). The principles set in both these documents also were applied in the Clean Access Plan for the EnEx field tests performed in Antarctica (Mikucki et al., 2023).
The tasks and recommendations regarding contamination management and control, published in the abovementioned documents, are derived from the necessity to reduce the number of cells and biological molecules on the instruments that enter (sub)glacial environments. Efficient biological and chemical burden reduction has required developments of multiple-step procedures for each hardware component. Usually, sampling equipment is sterilized before its transportation to the application site. However, this approach does not prevent contamination appearing shortly after the mission begins, for instance, if the ice-melting probe must penetrate contaminated upper ice layers on its way to the subglacial locations of interest. In this situation, two action classes must be defined: (a) pre- and inter-mission hardware decontamination in the stationary laboratory conditions and (b) in situ measures and protocols applied during the (sub)glacial exploration. The actions in the first group (a) can be designed very flexibly and use numerous classical disinfection and decontamination methods, combined and applied in a consecutive way. In addition to cleaning with various detergents and organic solvents, physical removal of bioburden can be assisted by autoclaving, dry heat, exposure of surfaces to ozone, ethylene oxide, ultraviolet or gamma radiation, gas plasmas or hydrogen peroxide (Rummel and Pugel, 2019).
The in situ decontamination (b) is especially important when multiple independent sampling acts have to be performed within the same deployment, without return to the laboratory. Main requirements for efficient in situ chemical decontamination in glacial environments are: high killing rate and activity against a broad range of microorganisms (spores, fungi, viruses, small insects, etc.), fast decomposing, environmentally friendly end products, easy handling, good solubility in water at any temperatures, good penetration ability, activity in cold environments, easy storage and easy application. Several successful decontamination protocols are developed and validated, for example, in the laboratories at FH Aachen University of Applied Sciences, using specially designed compositions based on 3%–5% (v/v) hydrogen peroxide and 3% (v/v) sodium hypochlorite (Leimea et al., 2010).
8 Relevance to systems on Earth
Considering the challenging work for realizing missions to the outer Solar System and to investigate particularly the habitable and potentially inhabited icy ocean worlds around Jupiter and Saturn, it is clear that the technology developments and realization of such missions have also a significant impact on the realization of tools necessary to explore environments on Earth (see also Section 4.1). An excellent side effect of this fact is the collaboration of engineers, natural scientists and information scientists from the three main research fields, such as polar research, ocean/deep sea research and space research, who finally are able to use different facilities and develop instruments for society relevant topics such as climate change studies, economic energy use, exploration of the ocean, exploration of the remote polar areas and also the exploration of space for finding new resources.
The oceans of Earth are far more dynamic and varied than is commonly assumed (Olbers et al., 2012), with the ice-covered regions doubly so. Taking the Arctic and Antarctic in turn, the Arctic ocean is in general an area with reasonably thin ice cover (several meters thick the general maximum thickness) that waxes and wanes in coverage throughout the year, with the central high Arctic permanently covered in moving sea ice (Kwok, 2018). The recent Multidisciplinary drifting Observatory for the Study of Arctic Climate (MOSAiC) expedition locked the German Polarstern research icebreaker of the Alfred Wegener Institute for Polar and Marine Research (AWI) in Bremerhaven into this moving ice to monitor firsthand the highly variable under and above ice environmental conditions taking place throughout the polar summer and polar night (Nicolaus et al., 2022). Below the ice, the water depth of the Arctic varies from a few centimeters at the coast to 5,500 m in the Molloy Deep. Topography is also highly variable, with flat plains interspersed with steep hills, cliffs, seamount chains, isolated seamounts, ridges and rises (Jakobsson et al., 2012). Of particular interest to icy moon research may be the Gakkel Ridge, a 4,000 m and ultraslow constructive plate margin running across the central Arctic, touching 85°N, and supporting a variety of active hydrothermal vents at depth, each with a unique community of life (Ramirez-Llodra et al., 2023). Shallow 1,000 m–2,000 m seamounts adjacent to the ridge are 100% covered by thick, extremely slow growing and long living sponge communities (Stratmann et al., 2022). Other sponge communities seem to bloom in the deeps, downstream of hydrothermal sources. Other Arctic areas include the extended Lomonsov Ridge and numerous isolated basins.
The Antarctic ocean contrasts with the Arctic in that it has a continental landmass center, covered with kilometers of glacial ice, containing many hundreds of isolated lakes of briny waters that cannot be reached by the sunlight (Dorschel et al., 2022). The waters around the Antarctic continent also differ from those surrounding the Arctic. According to current knowledge, they lack active hydrothermalism and are covered by glacial ice of hundreds of meters thickness, which has broken from the continent and may stay floating or grounded in the open ocean for decades (Wesche and Dierking, 2016). Beneath this ice, which can form hundreds of kilometers of ice tongues out over the ocean, no life penetrates but advected nutrients support diverse ecosystems, as new sensor equipped robotic platforms are starting to discover (Griffiths et al., 2021).
The great variety of polar life, food sources and environmental niches is still largely unknown. In 2021, the vast, several hundred km square array of actively nesting icefish was discovered in one of the more studied regions of the Weddell Sea (Purser et al., 2022). How these complex and diverse ecosystems interact in the underice environment can only inform icy ocean world research.
9 Conclusion and outlook: near future strategies
We here presented an overview about astrobiology research related to icy ocean worlds conducted in Germany. Due to involvements in ESA’s JUICE and NASA’s Europa Clipper, astrobiology investigations of icy ocean worlds are getting more and more attention within Germany and beyond. Through various institutions, such as the German Aerospace Center or German universities, Germany offers itself as a partner for international collaborations in icy ocean world research and space missions. The German Astrobiology Society represents a valuable platform for scientists and engineers to exchange ideas and start collaborations in icy moon research, involving international institutions, from space mission participations to individual research at universities and other institutions. Efforts include the development of melting probes to melt through the outer layer of an icy ocean world as well as instrumentation for life detection on these worlds. These projects feed upcoming missions, such as Europa Clipper, JUICE and potential future missions to Enceladus, Europa or any other icy ocean world.
One important aspect of icy moon research is the impact on society through themes like climate change, biodiversity and the exploration of new resources, as it is also supported by the general space program strategy of the German Government. In the near future the synergistic strategy in space exploration will be a significant gain for science as well as for innovative inventions for terrestrial applications and technology developments, particularly in increasing the benefit for the human society and the environment on our home planet Earth. Future missions to the icy ocean worlds with their resulting exploration technologies will provide opportunities to further support the exploration of Earth’s oceans and polar regions and to understand the role of these environments for the climate and the biosphere of the whole planet.
A new way of common cooperation should be consolidated on the long term because of its multi-useable technology and scientific outcome. Collaborations with international institutions are key to realizing space missions and exploiting the full potential of icy ocean world research. Field studies beyond Germany are easier to realize when researchers from other locations, meaning from the field site, are involved. Biosignature detection represents another realm in which various international institutions, particularly US institutions, are collaborating with researchers from Germany. In the near future, one particular focus should lie on modeling efforts to investigate the interactions between a subsurface ocean and a rocky core or an ice shell.
It is clear that the sequence of field investigations, lab research and space tests should be maintained and further supported. Synergy is key and will lead to collaborative actions as well as to visible solutions of common problems. To get there, politicians, national and international foundations, entrepreneurs and stakeholders should foster this promising collaborative interdisciplinary approach by significantly increasing their budgets for supporting such cooperative synergistic activities, technology developments and transfers.
Author contributions
FK: Conceptualization, Project administration, Resources, Supervision, Visualization, Writing–original draft, Writing–review and editing. MB: Resources, Writing–original draft, Writing–review and editing. KB-V: Resources, Writing–original draft, Writing–review and editing. JB: Resources, Writing–original draft, Writing–review and editing. MB: Resources, Writing–original draft, Writing–review and editing. BD: Resources, Writing–original draft, Writing–review and editing. ID: Resources, Writing–original draft, Writing–review and editing. AE: Resources, Writing–original draft, Writing–review and editing. CE: Resources, Writing–original draft, Writing–review and editing. OF: Resources, Writing–original draft, Writing–review and editing. EH: Resources, Writing–original draft, Writing–review and editing. DH: Resources, Writing–original draft, Writing–review and editing. FH: Resources, Writing–original draft, Writing–review and editing. LHS: Resources, Writing–original draft, Writing–review and editing. NK: Resources, Writing–original draft, Writing–review and editing. MN: Resources, Writing–original draft, Writing–review and editing. A-CP: Resources, Writing–original draft, Writing–review and editing. FP: Resources, Writing–original draft, Writing–review and editing. AP: Resources, Writing–original draft, Writing–review and editing. TR-B: Resources, Writing–original draft, Writing–review and editing. SS: Resources, Writing–original draft, Writing–review and editing. DS-M: Resources, Writing–original draft, Writing–review and editing. SU: Resources, Writing–original draft, Writing–review and editing. J-PdV: Conceptualization, Project administration, Resources, Writing–original draft, Writing–review and editing.
Funding
The author(s) declare that financial support was received for the research, authorship, and/or publication of this article. FK acknowledges support from the NASA Habitable Worlds Program grant No. 80NSSC19K0311. The DLR-internal institutes acknowledge the programmatic support in the development proposal “IMOTEC (Icy Moons Technologievorbereitung)”. The Institut für Bioengineering at FH Aachen acknowledges support from German Federal Ministry of Education (BMBF) and German Federal Research Centre for Aeronautics and Space Research (DLR) within the grant 50RK2351C: “TRIPLELifeDetect-Technologies for Rapid Ice Penetration and subglacial Lake Exploration - Life Detection A holistic, technological concept to Life Detection Missions in Extreme Cryosphere Environments.” AE acknowledges support from the Ministry of Economics and Energy (Projekträger Deutsches Zentrum für Luft-und Raumfahrt, grants 50WB1623 and 50WB2023. Furthermore, funding and support from the Forschungskomission (via TEAMS funding) of Freie Universität Berlin is gratefully acknowledged. FH is supported by Volkswagen Foundation and its Freigeist Program (AE, FH).
Acknowledgments
This publication emerged in the framework of the Ocean Worlds and Icy Moons working group of the German Astrobiology Society (DAbG). The authors note that the institutions, missions, and projects with German contributions to icy ocean world astrobiology research discussed in this paper are without any claim to completeness.
Conflict of interest
The authors declare that the research was conducted in the absence of any commercial or financial relationships that could be construed as a potential conflict of interest.
The author(s) declared that they were an editorial board member of Frontiers, at the time of submission. This had no impact on the peer review process and the final decision.
The author who is an editorial board member had no editorial role in the present paper and also no personal relations to the editor of this article.
Publisher’s note
All claims expressed in this article are solely those of the authors and do not necessarily represent those of their affiliated organizations, or those of the publisher, the editors and the reviewers. Any product that may be evaluated in this article, or claim that may be made by its manufacturer, is not guaranteed or endorsed by the publisher.
References
Baader, F., Boxberg, M. S., Chen, Q., Förstner, R., Kowalski, J., and Dachwald, B. (2024). Field-test performance of an ice-melting probe in a terrestrial analogue environment. Icarus 409, 115852. doi:10.1016/j.icarus.2023.115852
Baader, F., Dachwald, B., Espe, C., et al. (2016). Testing of a miniaturized subsurface icecraft for an Enceladus lander mission under enceladus-like conditions. LPI Contrib. No. 1927. Abstract #3029.
Baqué, M., Backhaus, T., Meeßen, J., Hanke, F., Böttger, U., Ramkissoon, N., et al. (2022). Biosignature stability in space enables their use for life detection on Mars. Sci. Adv. 8, eabn7412. doi:10.1126/sciadv.abn7412
Barabash, S., Wurz, P., Brandt, P., et al. (2013). Particle environment package (PEP). Eur. Planet. Sci. Congr. 8, EPSC2013–709.
Barker, J. D., Sharp, M. J., and Turner, R. J. (2009). Using synchronous fluorescence spectroscopy and principal components analysis to monitor dissolved organic matter dynamics in a glacier system. Hydrol. Process. 23, 1487–1500. doi:10.1002/hyp.7274
Barnes, J. W., Turtle, E. P., Trainer, M. G., Lorenz, R. D., MacKenzie, S. M., Brinckerhoff, W. B., et al. (2021). Science goals and objectives for the dragonfly titan rotorcraft relocatable lander. Planet. Sci. J. 2, 130. doi:10.3847/psj/abfdcf
Beauchamp, P. M., McKinnon, W., Magner, T., et al. (2009). Technologies for outer planet missions: a companion to the outer planet assessment group (OPAG) strategic exploration white paper.
Benway, H. M., Lorenzoni, L., White, A. E., Fiedler, B., Levine, N. M., Nicholson, D. P., et al. (2019). Ocean time series observations of changing marine ecosystems: an era of integration, synthesis, and societal applications. Front. Mar. Sci. 6, 393. doi:10.3389/fmars.2019.00393
Bland, M. T., and Elder, C. M. (2022). Silicate volcanism on Europa's seafloor and implications for habitability. Geophys. Res. Lett. 49 (5), e2021GL096939. doi:10.1029/2021gl096939
Blankenship, D. D., and Morse, D. L. (2004). “Earth's ice sheets and ice shelves as an analog for europa's icy shell,” in Workshop on europa's icy shell: past, present, and future. February 6-8, Houston, Texas, abstract no.7053.
BMWK (2023). Raumfahrtstrategie der Bundesregierung, Bundesministerium für Wirtschaft und Klimaschutz (BMWK). Berlin, 1–60.
Böttger, U., Bulat, S. A., Hanke, F., Pavlov, S. G., Greiner-Bär, M., and Hübers, H. (2017). Identification of inorganic and organic inclusions in the subglacial antarctic Lake Vostok ice with Raman spectroscopy. J. Raman Spectrosc. 48, 1503–1508. doi:10.1002/jrs.5142
Boxberg, M. S., Chen, Q., Plesa, A.-C., and Kowalski, J. (2023). Ice transit and performance analysis for cryorobotic subglacial access missions on Earth and Europa. Astrobiology 23, 1135–1152. doi:10.1089/ast.2021.0071
Bradák, B., Kereszturi, Á., and Gomez, C. (2023). Tectonic analysis of a newly identified putative cryovolcanic field on Europa. Adv. Space Res. 72, 4064–4073. doi:10.1016/j.asr.2023.07.062
Buffo, J. J., Schmidt, B. E., Huber, C., and Meyer, C. (2021). Characterizing the ice-ocean interface of icy worlds: a theoretical approach. Icarus 360, 114318. doi:10.1016/j.icarus.2021.114318
Buffo, J. J., Schmidt, B. E., Huber, C., and Walker, C. C. (2020). Entrainment and dynamics of ocean-derived impurities within Europa's ice shell. J. Geophys. Res.:Planets 125, e2020JE006394. doi:10.1029/2020je006394
Burrows, S. M., Ogunro, O., Frossard, A. A., Russell, L. M., Rasch, P. J., and Elliott, S. M. (2014). A physically based framework for modeling the organic fractionation of sea spray aerosol from bubble film Langmuir equilibria. Atmos. Chem. Phys. 14, 13601–13629. doi:10.5194/acp-14-13601-2014
Cable, M. L., Porco, C., Glein, C. R., German, C. R., MacKenzie, S. M., Neveu, M., et al. (2021). The Science case for a return to Enceladus. Planet. Sci. J. 2, 132. doi:10.3847/psj/abfb7a
Caro-Astorga, J., Meyerowitz, J. T., Stork, D. A., Nattermann, U., Piszkiewicz, S., Vimercati, L., et al. (2024). Polyextremophile engineering: a review of organisms that push the limits of life. Front. Microbiol. 15, 1341701. doi:10.3389/fmicb.2024.1341701
Carré, L., Henneke, G., Henry, E., Flament, D., Girard, É., and Franzetti, B. (2024). DNA polymerization in icy moon abyssal pressure conditions. Astrobiology 24, 151–162. doi:10.1089/ast.2021.0201
Cassidy, T., Coll, P., Raulin, F., Carlson, R. W., Johnson, R. E., Loeffler, M. J., et al. (2021). Radiolysis and photolysis of icy satellite surfaces: experiments and theory. Space Sci. Rev. 153, 299–315. doi:10.1007/s11214-009-9625-3
Choblet, G., Tobie, G., Kalousove, K., Kalousová, K., and Grasset, O. (2017). Heat transport in the high-pressure ice mantle of large icy moons. Icarus 285, 252–262. doi:10.1016/j.icarus.2016.12.002
Collins, G., McKinnon, W. B., Moore, J. M., et al. (2010). “Tectonics of the outer planet satellites,” in Planetary tectonics. Editors T. Watters, and R. Schultz (Cambridge University Press), 264–350.
Cottin, H., Stalport, F., Grand, N., et al. (2022). “The IR-COASTER project for astrobiology experiments oustide the International Space Station or as a payload for 6U CubeSats,” in 44th COSPAR scientific assembly abstract F3.2-0007-22.
Cox, R., and Bauer, A. W. (2015). Impact breaching of Europa's ice: constraints from numerical modeling. J. Geophys. Res.:Planets 120, 1708–1719. doi:10.1002/2015je004877
Dachwald, B., Mikucki, J., Tulaczyk, S., Digel, I., Espe, C., Feldmann, M., et al. (2014). IceMole: a maneuverable probe for clean in situ analysis and sampling of subsurface ice and subglacial aquatic ecosystems. Ann. Glaciol. 55, 14–22. doi:10.3189/2014aog65a004
Dachwald, B., Ulamec, S., Kowalski, J., et al. (2023). “Ice melting probes,” in Handbook of space resources. Editors V. Badescu, K. Zacny, and Y. Bar-Cohen (Springer), 955–996.
Dachwald, B., Ulamec, S., Postberg, F., Sohl, F., de Vera, J. P., Waldmann, C., et al. (2020). Key technologies and instrumentation for subsurface exploration of Ocean Worlds. Space Sci. Rev. 216, 83–45. doi:10.1007/s11214-020-00707-5
Damer, B., and Deamer, D. (2020). The hot spring hypothesis for an origin of life. Astrobiology 20, 429–452. doi:10.1089/ast.2019.2045
Dannenmann, M., Klenner, F., Bönigk, J., Pavlista, M., Napoleoni, M., Hillier, J., et al. (2023). Toward detecting biosignatures of DNA, lipids, and metabolic intermediates from bacteria in ice grains emitted by Enceladus and Europa. Astrobiology 23, 60–75. doi:10.1089/ast.2022.0063
De Laurentiis, E., Buoso, S., Maurino, V., Minero, C., and Vione, D. (2013). Optical and photochemical characterization of chromophoric dissolved organic matter from lakes in terra nova bay, Antarctica. Evidence of considerable photoreactivity in an extreme environment. Environ. Sci. Technol. 47, 14089–14098. doi:10.1021/es403364z
Della Corte, V., Schmitz, N., Zusi, M., et al. (2014). The JANUS camera onboard JUICE mission for Jupiter system optical imaging. Proc. SPIE 9143, Space Telesc. Instrum. 2014 Opt. Infrared, Millim. Wave 9143, 1062–1073. doi:10.1117/12.2056353
de Vera, J.-P.The Life Detection Group of BIOMEX/BIOSIGN (2019). “A systematic way to life detection: combining field, lab and space research in low Earth orbit,” in Biosignatures for astrobiology. Advances in astrobiology and biogeophysics. Editors B. Cavalazzi, and F. Westall (Springer), 111–122.
de Vera, J.-P., Alawi, M., Backhaus, T., Baqué, M., Billi, D., Böttger, U., et al. (2019). Limits of life and the habitability of Mars: the ESA space experiment BIOMEX on the ISS. Astrobiology 19, 145–157. doi:10.1089/ast.2018.1897
Doran, P. T., and Vincent, W. F. (2011). “Environmental protection and stewardship of subglacial aquatic environments,” in Antarctic subglacial aquatic environments 192. Editors M. J. Siegert, and I. I. M. C. Kennicutt (American Geophysical Union), 149–157.
Dorschel, B., Hehemann, L., Viquerat, S., Warnke, F., Dreutter, S., Tenberge, Y. S., et al. (2022). The international bathymetric chart of the southern ocean version 2. Sci. Data 9, 275. doi:10.1038/s41597-022-01366-7
Elsaesser, A., Burr, D. J., Mabey, P., Urso, R. G., Billi, D., Cockell, C., et al. (2023). Future space experiment platforms for astrobiology and astrochemistry research. Microgravity 9, 43. doi:10.1038/s41526-023-00292-1
Enya, K., Kobayashi, M., Kimura, J., Araki, H., Namiki, N., Noda, H., et al. (2022). The Ganymede laser altimeter (GALA) for the jupiter icy moons explorer (JUICE): mission, science, and instrumentation of its receiver modules. Adv. Space Res. 69, 2283–2304. doi:10.1016/j.asr.2021.11.036
Farkas-Takács, A., Kiss, C., Góbi, S., and Kereszturi, Á. (2022). Serpentinization in the thermal evolution of icy kuiper belt objects in the early solar system. Planet. Sci. J. 3, 54. doi:10.3847/psj/ac5175
Fifer, L. M., Toner, J. D., Ford, K., et al. (2024). Measuring exsolution rates of gases in a laboratory analog for Enceladus plume formation. Eur. Sci. Congr. 17, EPSC2024–1314. doi:10.5194/epsc2024-1314
Frazier, W., Bearden, D., Mitchell, K. L., et al. (2020). Trident: the path to Triton on a discovery budget. IEEE Aerosp. Conf., 1–12. doi:10.1109/AERO47225.2020.9172502
Funke, O., and Horneck, G. (2018). “The search for signatures of life and habitability on planets and moons of our solar system,” in Biological, physical and technical basics of cell engineering. Editors G. M. Artmann, A. Artmann, A. A. Zhubanova, and I. Digel (Springer), 457–481.
Goordial, J., Altshuler, I., Hindson, K., Chan-Yam, K., Marcolefas, E., and Whyte, L. G. (2017). In situ field sequencing and life detection in remote (79°26′N) Canadian high arctic permafrost ice wedge microbial communities. Front. Microbiol. 8, 2594. doi:10.3389/fmicb.2017.02594
Goossens, S., van Noort, B., Mateo, A., Mazarico, E., and van der Wal, W. (2024). A low-density ocean inside Titan inferred from Cassini data. Nat. Astron 8, 846–855. online ahead of print. doi:10.1038/s41550-024-02253-4
Grasset, O., Dougherty, M. K., Coustenis, A., Bunce, E. J., Erd, C., Titov, D., et al. (2013). JUpiter ICy moons Explorer (JUICE): an ESA mission to orbit Ganymede and to characterise the Jupiter system. Planet. Space Sci. 78, 1–21. doi:10.1016/j.pss.2012.12.002
Griffiths, H. J., Anker, P., Linse, K., Maxwell, J., Post, A. L., Stevens, C., et al. (2021). Breaking all the rules: the first recorded hard substrate sessile benthic community far beneath an antarctic ice shelf. Front. Mar. Sci. 8, 76. doi:10.3389/fmars.2021.642040
Gundlach, B., Ratte, J., Blum, J., Oesert, J., and Gorb, S. N. (2018). Sintering and sublimation of micrometre-sized water-ice particles: the formation of surface crusts on icy Solar System bodies. Mon. Not. R. Astron. Soc. 479, 5272–5287. doi:10.1093/mnras/sty1839
Haas, S., Sinclair, K. P., and Catling, D. C. (2024). Biogeochemical explanations for the world’s most phosphate-rich lake, an origin-of-life analog. Commun. Earth Environ. 5, 28. doi:10.1038/s43247-023-01192-8
Hagelschuer, T., Böttger, U., Buder, M., et al. (2022). “RAX: the Raman spectrometer for the MMX phobos rover,” in 73rd international astronautical congress.
Hand, K. P., Phillips, C. B., Murray, A., Garvin, J. B., Maize, E. H., Gibbs, R. G., et al. (2022). Science goals and mission architecture of the Europa lander mission concept. Planet. Sci. J. 3, 22. doi:10.3847/psj/ac4493
Hansen, C. J., Castillo-Rogez, J., Grundy, W., Hofgartner, J. D., Martin, E. S., Mitchell, K., et al. (2021). Triton: fascinating moon, likely ocean world, compelling destination. Planet Sci. J. 2, 137. doi:10.3847/psj/abffd2
Hansen, C. J., Esposito, L., Stewart, A. I. F., Colwell, J., Hendrix, A., Pryor, W., et al. (2006). Enceladus’ water vapor plume. Science 311, 1422–1425. doi:10.1126/science.1121254
Hartkorn, O., and Saur, J. (2017). Induction signals from Callisto's ionosphere and their implications on a possible subsurface ocean. J. Geophys. Res.:Space Phys. 122, 677–697. doi:10.1002/2017ja024269
Heggy, E., Scabbia, G., Bruzzone, L., and Pappalardo, R. T. (2017). Radar probing of Jovian icy moons: understanding subsurface water and structure detectability in the JUICE and Europa missions. Icarus 285, 237–251. doi:10.1016/j.icarus.2016.11.039
Heinen, D., Audehm, J., Becker, F., et al. (2021). The TRIPLE melting probe - an electro-thermal drill with a forefield reconnaissance system to access subglacial lakes and oceans. San Diego, CA, USA: OCEANS, 1–7.
Helbert, J., Lorek, A., Maturilli, A., et al. (2023). Cryogenic reflectance spectroscopy under high vacuum conditions for outer planets exploration. Infrared Remote Sens. Instrum. XXXI 12686, 79–85. doi:10.1117/12.2678910
Heldmann, J. L., Toon, O. B., Pollard, W. H., Mellon, M. T., Pitlick, J., McKay, C. P., et al. (2005). Formation of Martian gullies by the action of liquid water flowing under current Martian environmental conditions. J. Geophys. Res.:Planets 110, E05004. doi:10.1029/2004je002261
Hildebrandt, M., Creutz, T., Wehbe, B., et al. (2022). Under-ice field tests with an AUV in abisko/torneträsk. OCEANS 1–7. doi:10.1109/OCEANS47191.2022.9977094
Holm, A., Plesa, A.-C., Rückriemen-Bez, T., et al. (2024). Solid-state convection in Europa’s rocky core. Eur. Sci. Congr. 17, EPSC2024–699. doi:10.5194/epsc2024-699
Hoppa, G., Greenberg, R., Tufts, B. R., Geissler, P., Phillips, C., and Milazzo, M. (2000). Distribution of strike-slip faults on Europa. J. Geophys. Res.:Planets 105, 22617–22627. doi:10.1029/1999je001156
Horneck, G., David, M. K., and Mancinelli, R. L. (2010). Space microbiology. Microbiol. Mol. Biol. Rev. 74, 121–156. doi:10.1128/mmbr.00016-09
Hortal Sánchez, L., Napoleoni, M., Finkel, P. L., et al. (2024). Detection of molecular biosignatures in polar ices with mass spectrometry: implications for Europa Clipper. Eur. Sci. Congr. 17, EPSC2024–835. doi:10.5194/epsc2024-835
Howell, S. M., and Pappalardo, R. T. (2020). NASA’s Europa Clipper-a mission to a potentially habitable ocean world. Nat. Commun. 11, 1311. doi:10.1038/s41467-020-15160-9
Hsu, H.-W., Postberg, F., Sekine, Y., Shibuya, T., Kempf, S., Horányi, M., et al. (2015). Ongoing hydrothermal activities within Enceladus. Nature 519, 207–210. doi:10.1038/nature14262
Idini, B., and Nimmo, F. (2024). Resonant stratification in titan's global ocean. Planet. Sci. J. 5, 15. doi:10.3847/psj/ad11ef
Jakobsson, M., Mayer, L., Coakley, B., Dowdeswell, J. A., Forbes, S., Fridman, B., et al. (2012). The international bathymetric chart of the Arctic Ocean (IBCAO) version 3.0. Geophys. Res. Lett. 39, L12609. doi:10.1029/2012gl052219
Journaux, B., Kalousová, K., Sotin, C., Tobie, G., Vance, S., Saur, J., et al. (2020). Large Ocean worlds with high-pressure ices. Space Sci. Rev. 216, 7. doi:10.1007/s11214-019-0633-7
Kalousová, K., and Sotin, C. (2018). Melting in high-pressure ice layers of large ocean worlds — implications for volatiles transport. Geophys. Res. Lett. 45, 8096–8103. doi:10.1029/2018gl078889
Kelley, D. S., Karson, J. A., Fruh-Green, G. L., Yoerger, D. R., Shank, T. M., Butterfield, D. A., et al. (2005). A serpentinite-hosted ecosystem: the Lost city hydrothermal field. Science 307 (5714), 1428–1434. doi:10.1126/science.1102556
Kempf, S., Tucker, S., Altobelli, N., et al. (2024). SUDA: a SUrface dust analyser for compositional mapping of the galilean moon Europa. Space Sci. Rev. in press.
Kervazo, M., Běhounková, M., Tobie, G., et al. (2022). Impact of melt accumulation on tidal heat production in Europa’s mantle. Eur. Sci. Congr. Abstr. 16, EPSC2022–234. doi:10.5194/epsc2022-234
Khawaja, N., Hillier, J., Klenner, F., Nölle, L., Zou, Z., Napoleoni, M., et al. (2022). Complementary mass spectral analysis of isomeric O-bearing organic compounds and fragmentation differences through analog techniques for spaceborne mass spectrometers. Planet. Sci. J. 3, 254. doi:10.3847/psj/ac97ed
Khawaja, N., Hortal Sánchez, L., O’Sullivan, T. R., Bloema, J., Napoleoni, M., Klenner, F., et al. (2024a). Laboratory characterization of hydrothermally processed oligopeptides in ice grains emitted by Enceladus and Europa. Philos. Trans. R. Soc. A 382, 20230201. doi:10.1098/rsta.2023.0201
Khawaja, N., O’Sullivan, T. R., Klenner, F., Sanchez, L. H., and Hillier, J. (2023). Discriminating aromatic parent compounds and their derivative isomers in ice grains from Enceladus and Europa using a laboratory analogue for spaceborne mass spectrometers. Earth Space Sci. 10, e2022EA002807. doi:10.1029/2022ea002807
Khawaja, N., Postberg, F., Hillier, J., Klenner, F., Kempf, S., Nölle, L., et al. (2019). Low-mass nitrogen-oxygen-bearing, and aromatic compounds in Enceladean ice grains. Mon. Not. R. Astron. Soc. 489, 5231–5243. doi:10.1093/mnras/stz2280
Khawaja, N., Postberg, F., O’Sullivan, T. R., et al. (2024b). Cassini’s new look at organic material in Enceladus’ plume ice grains with CDA: implication for the habitability of Ocean Worlds. Eur. Sci. Congr. 17, EPSC2024–1055. doi:10.5194/epsc2024-1055
Kisvárdai, I., Pál, B. D., and Kereszturi, Á. (2023). Investigating the porosity of Enceladus. Mon. Not. R. Astron. Soc. 525, 1246–1253. doi:10.1093/mnras/stad2333
Kivelson, M. G., Khurana, K. K., Russel, C. T., Volwerk, M., Walker, R. J., and Zimmer, C. (2000). Galileo magnetometer measurements: a stronger case for a subsurface ocean at Europa. Science 289, 1340–1343. doi:10.1126/science.289.5483.1340
Klenner, F., Bönigk, J., Napoleoni, M., Hillier, J., Khawaja, N., Olsson-Francis, K., et al. (2024b). How to identify cell material in a single ice grain emitted from Enceladus or Europa. Sci. Adv. 10, eadl0849. doi:10.1126/sciadv.adl0849
Klenner, F., Fifer, L. M., Journaux, B., et al. (2024a). Toward a better understanding of ice grain formation from Enceladus’s salty ocean. Eur. Sci. Congr. 17, EPSC2024–657. doi:10.5194/epsc2024-657
Klenner, F., Postberg, F., Hillier, J., Khawaja, N., Cable, M. L., Abel, B., et al. (2020b). Discriminating abiotic and biotic fingerprints of amino acids and fatty acids in ice grains relevant to ocean worlds. Astrobiology 20, 1168–1184. doi:10.1089/ast.2019.2188
Klenner, F., Postberg, F., Hillier, J., Khawaja, N., Reviol, R., Srama, R., et al. (2019). Analogue spectra for impact ionization mass spectra of water ice grains obtained at different impact speeds in space. Rapid Commun. Mass Spectrom. 33, 1751–1760. doi:10.1002/rcm.8518
Klenner, F., Postberg, F., Hillier, J., Khawaja, N., Reviol, R., Stolz, F., et al. (2020a). Analog experiments for the identification of trace biosignatures in ice grains from extraterrestrial ocean worlds. Astrobiology 20, 179–189. doi:10.1089/ast.2019.2065
Klenner, F., Umair, M., Walter, S. H. G., Khawaja, N., Hillier, J., Nölle, L., et al. (2022). Developing a laser induced liquid beam ion desorption spectral database as reference for spaceborne mass spectrometers. Earth Space Sci. 9, e2022EA002313. doi:10.1029/2022ea002313
Kminek, G., Hedman, N., Ammannito, E., et al. (2020). COSPAR policy on planetary protection. Space Res. Today 208, 10–22. doi:10.1016/j.srt.2020.07.009
Konstantinidis, K., Flores Martinez, C. L., Dachwald, B., Ohndorf, A., Dykta, P., Bowitz, P., et al. (2015). A lander mission to probe subglacial water on Saturn’s moon Enceladus for life. Acta Astronaut. 106, 63–89. doi:10.1016/j.actaastro.2014.09.012
Kowalski, J., Linder, P., Zierke, S., von Wulfen, B., Clemens, J., Konstantinidis, K., et al. (2016). Navigation technology for exploration of glacier ice with maneuverable melting probes. Cold Reg. Sci. Technol. 123, 53–70. doi:10.1016/j.coldregions.2015.11.006
Kowalski, J., Plesa, A.-C., Boxberg, M., et al. (2024). Compiling analysis-ready ice data across cryosphere disciplines. European Geosciences Union General Assembly. EGU24-21117. doi:10.5194/egusphere-egu24-21117
Kwok, R. (2018). Arctic sea ice thickness, volume, and multiyear ice coverage: losses and coupled variability (1958–2018). Environ. Res. Lett. 13, 105005. doi:10.1088/1748-9326/aae3ec
Lebec, L., Labrosse, S., Morison, A., Bolrão, D. P., and Tackley, P. J. (2024). Effects of salts on the exchanges through high-pressure ice layers of large ocean worlds. Icarus 412, 115966. doi:10.1016/j.icarus.2024.115966
Lebreton, J.-P., Witasse, O., Sollazzo, C., Blancquaert, T., Couzin, P., Schipper, A. M., et al. (2005). An overview of the descent and landing of the Huygens probe on Titan. Nature 438, 758–764. doi:10.1038/nature04347
Lei, L., Jäggi, A., Wang, Y., et al. (2021). Gan de: a mission to search for the origins and workings of the jupiter system. 43th COSPAR Sci. Assem. Abstract B0.7-0016-21.
Leimea, W., Artmann, G. M., Dachwald, B., et al. (2010). Feasibility of an in-situ microbial decontamination of an ice-melting probe. Eur. Chem. Tech. J. 12, 145–150. doi:10.18321/ectj37
Li, X., Miao, Z., Dan, C., Wu, Z., and Xia, Y. (2023). In situ Nanopore sequencing reveals metabolic characteristics of the Qilian glacier meltwater microbiome. Environ. Sci. Pollut. Res. 30, 84805–84813. doi:10.1007/s11356-023-28250-0
Lyons, W. B., Mikucki, J. A., German, L. A., Welch, K. A., Welch, S. A., Gardner, C. B., et al. (2019). The geochemistry of englacial brine from Taylor Glacier, Antarctica. J. Geophys. Res.:Biogeosciences 124, 633–648. doi:10.1029/2018jg004411
MacKenzie, S. M., Neveu, M., Davila, A. F., Lunine, J. I., Craft, K. L., Cable, M. L., et al. (2021). The Enceladus Orbilander mission concept: balancing return and resources in the search for life. Planet. Sci. J. 2, 77. doi:10.3847/psj/abe4da
Malaterre, C., ten Kate, I. L., Baqué, M., Debaille, V., Grenfell, J. L., Javaux, E. J., et al. (2023). Is there such as thing as a biosignature? Astrobiology 23, 1213–1227. doi:10.1089/ast.2023.0042
Margesin, R., Schumann, P., Zhang, D.-C., Redzic, M., Zhou, Y. G., Liu, H. C., et al. (2012). Arthrobacter cryoconiti sp. nov., a psychrophilic bacterium isolated from alpine glacier cryoconite. Int. J. Syst. Evol. Microbiol. 62, 397–402. doi:10.1099/ijs.0.031138-0
Martin, A., and McMinn, A. (2018). Sea ice, extremophiles and life on extra-terrestrial ocean worlds. Int. J. Astrobiol. 17, 1–16. doi:10.1017/s1473550416000483
Martin, W., Baross, J., Kelley, D., and Russell, M. J. (2008). Hydrothermal vents and the origin of life. Nat. Rev. Microbiol. 6, 805–814. doi:10.1038/nrmicro1991
Martins, Z., Bunce, E., Grasset, O., et al. (2024). Report of the Expert Committee for the Large-class mission in ESA’s Voyage 2050 plan covering the science theme “Moons of the Giant Planets”. Moons Giant Planets, 1–45.
Marusiak, A. G., Vance, S., Panning, M. P., Běhounková, M., Byrne, P. K., Choblet, G., et al. (2021). Exploration of icy Ocean Worlds using geophysical approaches. Planet. Sci. J. 2, 150. doi:10.3847/psj/ac1272
Matsuyama, I., Beuthe, M., Hay, HCFC, Nimmo, F., and Kamata, S. (2018). Ocean tidal heating in icy satellites with solid shells. Icarus 312, 208–230. doi:10.1016/j.icarus.2018.04.013
Matteoni, P., Neesemann, A., Jaumann, R., Hillier, J., and Postberg, F. (2023). Ménec fossae on Europa: a strike-slip tectonics origin above a possible shallow water reservoir. J. Geophys. Res.:Planets 128, e2022JE007623. doi:10.1029/2022je007623
McCliment, E. A., Voglesonger, K. M., O’Day, P. A., Dunn, E. E., Holloway, J. R., and Cary, S. C. (2006). Colonization of nascent, deep-sea hydrothermal vents by a novel Archaeal and Nanoarchaeal assemblage. Environ. Microbiol. 8, 114–125. doi:10.1111/j.1462-2920.2005.00874.x
Mikucki, J. A., Schuler, C. G., Digel, I., Kowalski, J., Tuttle, M., Chua, M., et al. (2023). Field-based planetary protection operations for melt probes: validation of clean access into the Blood Falls, Antarctica, englacial ecosystem. Astrobiology 23, 1165–1178. doi:10.1089/ast.2021.0102
Mousis, O., Bouquet, A., Langevin, Y., André, N., Boithias, H., Durry, G., et al. (2022). Moonraker: Enceladus multiple flyby mission. Planet. Sci. J. 3, 268. doi:10.3847/psj/ac9c03
Mulyukin, A. L., Demkina, E. V., Manucharova, N. A., Akimov, V. N., Andersen, D., McKay, C., et al. (2014). The prokaryotic community of subglacial bottom sediments of Antarctic Lake Untersee: detection by cultural and direct microscopic techniques. Microbiology 83, 77–84. doi:10.1134/s0026261714020143
Nadeau, J., Lindensmith, C., Deming, J. W., Fernandez, V. I., and Stocker, R. (2016). Microbial morphology and motility as biosignatures for outer planet missions. Astrobiology 16, 755–774. doi:10.1089/ast.2015.1376
Napoleoni, M., Klenner, F., Hortal Sánchez, L., Khawaja, N., Hillier, J. K., Gudipati, M. S., et al. (2023b). Mass spectrometric fingerprints of organic compounds in sulfate-rich ice grains: implications for Europa clipper. ACS Earth Space Chem. 7, 1675–1693. doi:10.1021/acsearthspacechem.3c00098
Napoleoni, M., Klenner, F., Khawaja, N., Hillier, J. K., and Postberg, F. (2023a). Mass spectrometric fingerprints of organic compounds in NaCl-rich ice grains from Europa and Enceladus. ACS Earth Space Chem. 7, 735–752. doi:10.1021/acsearthspacechem.2c00342
National Academies of Sciences, Engineering, and Medicine (2023). Origins, worlds, and life: a decadal strategy for planetary science and astrobiology 2023-2032. Washington, DC: The National Academies Press.
Neish, C., Malaska, M. J., Sorin, C., Lopes, R. M., Nixon, C. A., Affholder, A., et al. (2024). Organic input to titan's subsurface ocean through impact cratering. Astrobiology 24, 177–189. doi:10.1089/ast.2023.0055
Nicolaus, M., Perovich, D. K., Spreen, G., Granskog, M. A., von Albedyll, L., Angelopoulos, M., et al. (2022). Overview of the MOSAiC expedition: snow and sea ice. Elem. Sci. Anth. 10, 000046. doi:10.1525/elementa.2021.000046
Nimmo, F., and Pappalardo, R. T. (2016). Ocean worlds in the outer solar system. J. Geophys. Res.:Planets 121, 1378–1399. doi:10.1002/2016je005081
O’Donnell, E. C., Wadham, J. L., Lis, G. P., Tranter, M., Pickard, A. E., Stibal, M., et al. (2016). Identification and analysis of low-molecular-weight dissolved organic carbon in subglacial basal ice ecosystems by ion chromatography. Biogeoscience 13, 3833–3846. doi:10.5194/bg-13-3833-2016
O’Sullivan, T. R., Bera, P. P., Khawaja, N., and Postberg, F. (2024). A computational chemistry approach to analysing mass spectra of organic-bearing ice grains from icy ocean worlds. Berlin, Germany: AbGradEPEC 2024.
Olbers, D., Willebrand, J., and Eden, C. (2012). Ocean dynamics. Springer Science and Business Media, 703.
Pappalardo, R. T., Buratti, B. J., Korth, H., Senske, D. A., Blaney, D. L., Blankenship, D. D., et al. (2024). Science overview of the Europa clipper mission. Space Sci. Rev. 220, 40. doi:10.1007/s11214-024-01070-5
Pavlov, S. G., Jessberger, E. K., Hübers, H. W., Schröder, S., Rauschenbach, I., Florek, S., et al. (2011). Miniaturized laser-induced plasma spectrometry for planetary in situ analysis–The case for Jupiter’s moon Europa. Adv. Space Res. 48, 764–778. doi:10.1016/j.asr.2010.06.034
Plesa, A.-C., Rückriemen-Bez, T., and Wünnemann, K. (2024). The role of impacts on ice shell dynamics and surface-to-ocean exchange on Europa. Eur. Sci. Congr. 17, EPSC2024–701. doi:10.5194/epsc2024-701
Porazinska, D. L., Fountain, A. G., Nylen, T. H., Tranter, M., Virginia, R. A., and Wall, D. H. (2004). The biodiversity and biogeochemistry of cryoconite holes from McMurdo Dry Valley glaciers, Antarctica. Arct. Antarct. Alp. Res. 36, 84–91. doi:10.1657/1523-0430(2004)036[0084:tbaboc]2.0.co;2
Porco, C. C., Helfenstein, P., Thomas, P. C., Ingersoll, A. P., Wisdom, J., West, R., et al. (2006). Cassini observes the active south Pole of Enceladus. Science 311, 1393–1401. doi:10.1126/science.1123013
Postberg, F., Kempf, S., Schmidt, J., Brilliantov, N., Beinsen, A., Abel, B., et al. (2009). Sodium salts in E-ring ice grains from an ocean below the surface of Enceladus. Nature 459, 1098–1101. doi:10.1038/nature08046
Postberg, F., Khawaja, N., Abel, B., Choblet, G., Glein, C. R., Gudipati, M. S., et al. (2018). Macromolecular organic compounds from the depths of Enceladus. Nature 558, 564–568. doi:10.1038/s41586-018-0246-4
Postberg, F., Sekine, Y., Klenner, F., Glein, C. R., Zou, Z., Abel, B., et al. (2023). Detection of phosphates originating from Enceladus’s ocean. Nature 618, 489–493. doi:10.1038/s41586-023-05987-9
Purser, A., Hehemann, L., Boehringer, L., Werner, E., Pineda-Metz, S. E. A., Vignes, L., et al. (2022). The COSMUS expedition: seafloor images and acoustic bathymetric data from the PS124 expedition to the southern Weddell Sea, Antarctica. Earth Syst. Sci. Data 14, 3635–3648. doi:10.5194/essd-14-3635-2022
Rabbow, E., Rettberg, P., Parpart, A., Panitz, C., Schulte, W., Molter, F., et al. (2017). EXPOSE-R2: the astrobiological ESA mission on board of the International Space Station. Front. Microbiol. 8, 1533. doi:10.3389/fmicb.2017.01533
Ramirez-Llodra, E., Argentino, C., Baker, M., Boetius, A., Costa, C., Dahle, H., et al. (2023). Hot vents beneath an icy ocean: the aurora vent field, Gakkel Ridge, revealed. Oceanography 36, 6–17. doi:10.5670/oceanog.2023.103
Rapin, W., Maurice, S., Ollila, A., et al. (2023). μLIBS: a micro-scale elemenal analyser for lightweight in situ exploration. LPI Contrib. No., 2806. Abstract #1942.
Rapley, C., Aghanim, N., Anand, M., et al. (2022). Report and recommendations to the European space agency ministerial Council.
Rettberg, P., Antunes, A., Brucato, J., Cabezas, P., Collins, G., Haddaji, A., et al. (2019). Biological contamination prevention for outer solar system moons of astrobiological interest: what do we need to know? Astrobiology 19, 951–974. doi:10.1089/ast.2018.1996
Rinaldi, R. E., Koci, B. R., and Sonderup, J. M. (1990). Evaluation of deep ice core drilling systems, 90-01. PICO TR, 1–34.
Rovira-Navarro, M., Rieutord, M., Gerkema, R., Maas, L. R., van der Wal, W., and Vermeersen, B. (2019). Do tidally-generated inertial waves heat the subsurface oceans of Europa and Enceladus? Icarus 321, 126–140. doi:10.1016/j.icarus.2018.11.010
Rückriemen-Bez, T., Plesa, A.-C., Kowalski, J., and Terschanski, B. (2022). Large-scale dynamics and the fate of salts in Europa's icy shell. Eur. Sci. Congr. 16, EPSC2022–689. doi:10.5194/epsc2022-689
Rückriemen-Bez, T., Terschanski, B., Plesa, A. C., et al. (2023). Coupling ice-ocean interface models with global-scale ice shell evolution models applied to Jovian moon Europa. Eur. Geosci. Union General Assem. EGU23–6803. doi:10.5194/egusphere-egu23-6803
Rudolph, M. L., Manga, M., Walker, M., and Rhoden, A. R. (2022). Cooling crusts create concomitant cryovolcanic cracks. Geophys. Res. Lett. 49, e2021GL094421. doi:10.1029/2021gl094421
Rummel, J. D., and Pugel, D. E. (2019). Planetary protection technologies for planetary science instruments, spacecraft, and missions: report of the NASA Planetary Protection Technology Definition Team (PPTDT). Life Sci. Space Res. 23, 60–68. doi:10.1016/j.lssr.2019.06.003
Russel, M. J., Murray, A. E., and Hand, K. P. (2017). The possible emergence of life and differentiation of a shallow biosphere on irradiated icy worlds: the example of Europa. Astrobiology 17, 1265–1273. doi:10.1089/ast.2016.1600
Sanderink, A., Klenner, F., Zymak, I., Žabka, J., Postberg, F., Lebreton, J. P., et al. (2023). OLYMPIA-LILBID: a new laboratory setup to calibrate spaceborne hypervelocity ice grain detectors using high-resolution mass spectrometry. Anal. Chem. 95, 3621–3628. doi:10.1021/acs.analchem.2c04429
Saur, J., Duling, S., Roth, L., Jia, X., Strobel, D. F., Feldman, P. D., et al. (2015). The search for a subsurface ocean in Ganymede with Hubble Space Telescope observations of its auroral ovals. J. Geophys. Res. Space Phys. 120, 1715–1737. doi:10.1002/2014ja020778
Schmidt, B. E., Blankenship, D. D., Patterson, G. W., and Schenk, P. M. (2011). Active formation of “chaos terrain” over shallow subsurface water on Europa. Nature 479, 502–505. doi:10.1038/nature10608
Schmidt, J., Brilliantov, N., Spahn, F., and Kempf, S. (2008). Slow dust in Enceladus' plume from condensation and wall collisions in tiger stripe fractures. Nature 451, 685–688. doi:10.1038/nature06491
Schmidt, J., Ershova, A., Postberg, F., et al. (2024). The Enceladus dust plume from the Cassini cosmic dust analyzer. Eur. Sci. Congr. 17, EPSC2024–510. doi:10.5194/epsc2024-510
Schröder, S., Pavlov, S. G., Rauschenbach, I., Jessberger, E., and Hübers, H. W. (2013). Detection and identification of salts and frozen salt solutions combining laser-induced breakdown spectroscopy and multivariate analysis methods: a study for future martian exploration. Icarus 223, 61–73. doi:10.1016/j.icarus.2012.11.011
Schüller, K., and Kowalski, J. (2019). Melting probe technology for subsurface exploration of extraterrestrial ice – critical refreezing length and the role of gravity. Icarus 317, 1–9. doi:10.1016/j.icarus.2018.05.022
Schulze-Makuch, D., and Irwin, L. N. (2002). Energy cycling and hypothetical organisms in Europa's ocean. Astrobiology 2, 105–121. doi:10.1089/153110702753621385
Schulze-Makuch, D., and Irwin, L. N. (2018). Life in the universe: expectations and constraints. 3rd edition. Springer, 343.
Shibley, N. C., and Goodman, J. (2024). Europa’s coupled ice–ocean system: temporal evolution of a pure ice shell. Icarus 410, 115872. doi:10.1016/j.icarus.2023.115872
Siegert, M. J., and Kennicutt, M. C. (2018). Governance of the exploration of subglacial Antarctica. Front. Environ. Sci. 6, 103. doi:10.3389/fenvs.2018.00103
Smith, H. J., Dieser, M., McKnight, D. M., SanClements, M., and Foreman, C. (2018). Relationship between dissolved organic matter quality and microbial community composition across polar glacial environments. FEMS Microbiol. Ecol. 94, fiy090. doi:10.1093/femsec/fiy090
Smith, I. E. M., and Price, R. C. (2006). The Tonga–Kermadec arc and Havre–Lau back-arc system: their role in the development of tectonic and magmatic models for the western Pacific. J. Volcanol. Geotherm. Res. 156, 315–331. doi:10.1016/j.jvolgeores.2006.03.006
Soderlund, K. M. (2019). Ocean dynamics of outer solar system satellites. Geophys. Res. Lett. 46, 8700–8710. doi:10.1029/2018gl081880
Soderlund, K. M., Kalousová, K., Buffo, J. J., Glein, C. R., Goodman, J. C., Mitri, G., et al. (2020). Ice-ocean exchange processes in the Jovian and Saturnian satellites. Space Sci. Rev. 216, 80–57. doi:10.1007/s11214-020-00706-6
Sohl, F., Spohn, T., Breuer, D., and Nagel, K. (2002). Implications from Galileo observations on the interior structure and chemistry of the Galilean satellites. Icarus 157, 104–119. doi:10.1006/icar.2002.6828
Sotin, C., Tobie, G., Wahr, J., et al. (2009). “Tides and tidal heating on Europa,” in Europa Pappalardo RT, McKinnon WB. Editor K. K. Khurana (The University of Arizona Press), 85–118.
Sparks, W. B., Schmidt, B. E., McGrath, M. A., Hand, K. P., Spencer, J. R., Cracraft, M., et al. (2017). Active cryovolcanism on Europa? Astrophys. J. Lett. 839, L18. doi:10.3847/2041-8213/aa67f8
Spencer, J. R., and Nimmo, F. (2013). Enceladus: an active ice world in the Saturn system. Annu. Rev. Earth Planet. Sci. 41, 693–717. doi:10.1146/annurev-earth-050212-124025
Srama, R., Ahrens, T. J., Altobelli, N., Auer, S., Bradley, J. G., Burton, M., et al. (2004). The Cassini cosmic dust analyzer. Space Sci. Rev. 114, 465–518. doi:10.1007/s11214-004-1435-z
Storrie-Lombardi, M. C., and Sattler, B. (2009). Laser-induced fluorescence emission (L.I.F.E.): in situ nondestructive detection of microbial life in the ice covers of antarctic lakes. Astrobiology 9, 659–672. doi:10.1089/ast.2009.0351
Stratmann, T., Simon-Lledó, E., Morganti, T. M., de Kluijver, A., Vedenin, A., and Purser, A. (2022). Habitat types and megabenthos composition from three sponge-dominated high-Arctic seamounts. Sci. Rep. 12, 20610. doi:10.1038/s41598-022-25240-z
Tacconi, L. J., Arridge, C. S., Buonanno, A., et al. (2021). Voyage 2050 final recommendations from the voyage 2050 senior committee.
Terra-Nova, F., Amit, H., Choblet, G., Tobie, G., Bouffard, M., and Čadek, O. (2023). The influence of heterogeneous seafloor heat flux on the cooling patterns of Ganymede’s and Titan’s subsurface oceans. Icarus 389, 115232. doi:10.1016/j.icarus.2022.115232
Thombre, R. C., Vaishampayan, P. A., and Gomez, F. (2020). “Applications of extremophiles in astrobiology,” in Physiological and biotechnological aspects of extremophiles. Editors R. Salwan, and V. Sharma (Academic Press London), 89–104.
Toner, J. D., and Catling, D. C. (2020). A carbonate-rich lake solution to the phosphate problem of the origin of life. Proc. Natl. Acad. Sci. U.S.A. 117, 883–888. doi:10.1073/pnas.1916109117
Trumbo, S. K., and Brown, M. E. (2023). The distribution of CO2 on Europa indicates an internal source of carbon. Science 381, 1308–1311. doi:10.1126/science.adg4155
Tufts, B. R., Greenberg, R., Hoppa, G., and Geissler, P. (1991). Astypalaea Linea: a large-scale strike-slip fault on Europa. Icarus 141, 53–64. doi:10.1006/icar.1999.6168
Vance, S. D., Bouffard, M., Choukroun, M., and Sorin, C. (2014). Ganymede׳s internal structure including thermodynamics of magnesium sulfate oceans in contact with ice. Planet Space Sci. 96, 62–70. doi:10.1016/j.pss.2014.03.011
Vance, S. D., Craft, K. L., Shock, E., Schmidt, B. E., Lunine, J., Hand, K. P., et al. (2023). Investigating europa's habitability with the Europa clipper. Space Sci. Rev. 219, 81. doi:10.1007/s11214-023-01025-2
Villanueva, G. L., Hammel, H. B., Milam, S. M., Faggi, S., Kofman, V., Roth, L., et al. (2023a). Endogenous CO2 ice mixture on the surface of Europa and no detection of plume activity. Science 381, 1305–1308. doi:10.1126/science.adg4270
Villanueva, G. L., Hammel, H. B., Milam, S. M., Kofman, V., Faggi, S., Glein, C. R., et al. (2023b). JWST molecular mapping and characterization of Enceladus’ water plume feeding its torus. Nat. Astron. 7, 1056–1062. doi:10.1038/s41550-023-02009-6
Waite, J. H., Glein, C. R., Perryman, R. S., Teolis, B. D., Magee, B. A., Miller, G., et al. (2017). Cassini finds molecular hydrogen in the Enceladus plume: evidence for hydrothermal processes. Science 356, 155–159. doi:10.1126/science.aai8703
Waldmann, C., and Funke, O. (2020). The TRIPLE/nanoAUV initiative a technology development initiative to support astrobiological exploration of ocean worlds. CEAS Space J. 12, 115–122. doi:10.1007/s12567-019-00275-7
Weber, J. M., Marlin, T. C., Prakash, M., Teece, B. L., Dzurilla, K., and Barge, L. M. (2023). A review on hypothesized metabolic pathways on Europa and Enceladus: space-flight detection considerations. Life 13, 1726. doi:10.3390/life13081726
Weinstock, L. S., Zierke, S., Eliseev, D., Linder, P., Vollbrecht, C., Heinen, D., et al. (2021). The autonomous pinger unit of the acoustic navigation network in EnEx-RANGE: an autonomous in-ice melting probe with acoustic instrumentation. Ann. Glaciol. 62, 89–98. doi:10.1017/aog.2020.67
Weissbrodt, P., Raupach, L., and Hacker, E. J. (1994). Improved method for contamination control during fabrication of space equipment. Proc. Space Opt. 1994 Space Instrum. Spacecr. Opt. 2210, 672–680. doi:10.1117/12.188127
Wesche, C., and Dierking, W. (2016). Estimating iceberg paths using a wind-driven drift model. Cold Regions Sci. Technol. 125, 31–39. doi:10.1016/j.coldregions.2016.01.008
Wierzchos, J., Casero, M. C., Artieda, O., and Ascaso, C. (2018). Endolithic microbial habitats as refuges for life in polyextreme environment of the Atacama Desert. Curr. Opin. Microbiol. 43, 124–131. doi:10.1016/j.mib.2018.01.003
Wolfenbarger, N. S., Fox-Powell, M. G., Buffo, J. J., Soderlund, K. M., and Blankenship, D. D. (2022). Brine volume fraction as a habitability metric for europa's ice shell. Geophys. Res. Lett. 49, e2022GL100586. doi:10.1029/2022gl100586
Wong, T., Hansen, U., Wiesehöfer, T., and McKinnon, W. B. (2022). Layering by double-diffusive convection in the Subsurface Oceans of Europa and Enceladus. J. Geophys. Res.:Planets 127, e2022JE007316. doi:10.1029/2022je007316
Zolotov, M. Y., and Kargel, J. S. (2009). “On the chemical composition of Europa’s icy shell, ocean, and underlying rocks,” in Europa Pappalardo RT, McKinnon WB. Editor K. K. Khurana (The University of Arizona Press), 431–458.
Keywords: subsurface oceans, space missions, habitability, icy moons, solar system exploration, Deutsche Astrobiologische Gesellschaft (DAbG), ocean worlds, German Aerospace Center (DLR)
Citation: Klenner F, Baqué M, Beblo-Vranesevic K, Bönigk J, Boxberg MS, Dachwald B, Digel I, Elsaesser A, Espe C, Funke O, Hauber E, Heinen D, Hofmann F, Hortal Sánchez L, Khawaja N, Napoleoni M, Plesa A-C, Postberg F, Purser A, Rückriemen-Bez T, Schröder S, Schulze-Makuch D, Ulamec S and de Vera J-PP (2024) Icy ocean worlds - astrobiology research in Germany. Front. Astron. Space Sci. 11:1422898. doi: 10.3389/fspas.2024.1422898
Received: 24 April 2024; Accepted: 22 July 2024;
Published: 14 August 2024.
Edited by:
Josep M. Trigo-Rodríguez, Spanish National Research Council (CSIC), SpainReviewed by:
Akos Kereszturi, Hungarian Academy of Sciences (MTA), HungaryAlfonso F. Davila, National Aeronautics and Space Administration, United States
Copyright © 2024 Klenner, Baqué, Beblo-Vranesevic, Bönigk, Boxberg, Dachwald, Digel, Elsaesser, Espe, Funke, Hauber, Heinen, Hofmann, Hortal Sánchez, Khawaja, Napoleoni, Plesa, Postberg, Purser, Rückriemen-Bez, Schröder, Schulze-Makuch, Ulamec and de Vera. This is an open-access article distributed under the terms of the Creative Commons Attribution License (CC BY). The use, distribution or reproduction in other forums is permitted, provided the original author(s) and the copyright owner(s) are credited and that the original publication in this journal is cited, in accordance with accepted academic practice. No use, distribution or reproduction is permitted which does not comply with these terms.
*Correspondence: Fabian Klenner, ZmtsZW5uZXJAdXcuZWR1; Jean-Pierre Paul de Vera, amVhbi1waWVycmUuZGV2ZXJhQGRsci5kZQ==