- School of Physics and Astronomy, University of Exeter, Exeter, United Kingdom
Simulations from the scales of isolated galaxies to clouds have been instrumental in informing us about molecular cloud formation and evolution. Simulations are able to investigate the roles of gravity, feedback, turbulence, heating and cooling, and magnetic fields on the physics of the interstellar medium, and star formation. Compared to simulations of individual clouds, galactic and sub-galactic scale simulations can include larger galactic scale processes such as spiral arms, bars, and larger supernovae bubbles, which may influence star formation. Simulations show cloud properties and lifetimes in broad agreement with observations. Gravity and spiral arms are required to produce more massive GMCs, whilst stellar feedback, likely photoionisation, leads to relatively short cloud lifetimes. On larger scales, supernovae may be more dominant in driving the structure and dynamics, but photoionisation may still have a role. In terms of the dynamics, feedback is probably the main driver of velocity dispersions, but large scale processes such as gravity and spiral arms may also be significant. Magnetic fields are generally found to decrease star formation on galaxy or cloud scales, and simulations are ongoing to study whether clouds are sub or supercritical on different scales in galaxy scale simulations. Simulations on subgalactic scales, or zoom in simulations, allow better resolution of feedback processes, filamentary structure within clouds, and the study of stellar clusters.
1 Introduction
Simulations on the scales of isolated galaxies and on kiloparsec scales have allowed us to resolve the multiphase interstellar medium (ISM), molecular clouds, and larger scale feedback processes such as supernovae whilst also including larger scale galactic structure such as spiral arms, bars or galaxy centres. Here we present a review of the simulations in this field, what they have told us about molecular cloud formation and evolution, star formation, and the role of galactic structure. We start in the introduction with a more historical review of simulations on these scales up to around 2010. The following sections have a stronger focus on work from the last 15 years. Apart from the oldest (i.e. pre 1990) work mentioned, almost all the simulation work cited uses grid or Adaptive Mesh Refinement (AMR), Smoothed Particle Hydrodynamics (SPH), or moving mesh codes. The final section presents conclusions and suggestions for future progress. Moving forward, current progress may involve studying stellar clusters on these scales, and simulating the process of cluster dissolution from its natal gas, and perhaps on larger scales modelling galaxies in a more extragalactic context.
The earliest simulations on galaxy scales focused on understanding the development of galactic structure, and the role of cloud-cloud collisions. For example test particle, and N-body calculations showed the development of spiral or barred structure in galactic discs (e.g. Miller et al., 1970; Hohl, 1971; Toomre and Toomre, 1972; Sellwood, 1980; Combes and Sanders, 1981; Sellwood and Carlberg, 1984). Simulations of molecular or interstellar clouds adopted collisional models with assumptions about cloud growth and destruction to model the resultant mass spectra of clouds, and the influence of a spiral potential on the resultant cloud population (Roberts et al., 1984; Tomisaka, 1984; Combes and Gerin, 1985; Roberts et al., 1987).
Probably the first hydrodynamical simulations of isolated galaxies resembling modern simulations were by Wada and Norman (1999) and Wada et al. (2000) who, although they only modelled 2D rather than 3D discs, included a degree of physics surprisingly commensurate with modern day simulations. This included cooling and heating, molecular chemistry, stellar feedback in terms of both winds and supernovae, and test particles sampling the IMF such that insert winds and supernovae are added according to stellar masses and lifetimes. These simulations, and others (Dobbs, 2008) which included the cold phase of the ISM, allowed smaller clouds to form, and showed the development of larger giant molecular clouds (GMCs) through a combination of gravitational instabilities and cloud-cloud collisions. Simulations adopting an isothermal warm medium allowed easier predictions with theory. Elmegreen and Elmegreen (1983) and others predicted that GMCs form via the development of gravitational instabilities occurring at the most unstable wavelength (fastest growing mode). For a warm isothermal medium this equates to GMCs along spiral arms with characteristic masses of around 106–107 M⊙ and separations of around 1 kpc, which are reproduced in simulations (Kim and Ostriker, 2006; Dobbs, 2008).
Some of the earlier hydrodynamical simulations of galaxies also focused on the development of spurs along spiral arms (Kim and Ostriker, 2002; Chakrabarti et al., 2003; Wada and Koda, 2004; Dobbs and Bonnell, 2006; Kim and Ostriker, 2006; Shetty et al., 2007). These have been associated with instabilities which include Kelvin Helmholz (Wada and Koda, 2004; Renaud et al., 2013), feathering (Chakrabarti et al., 2003; Lee, 2014), wiggle (Kim et al., 2014), MRI (Kim and Ostriker, 2002) and gravitational (Kim and Ostriker, 2006), and cloud collisions due to orbit crowding (Dobbs and Bonnell, 2006). In most of these simulations however, the formation of the spurs is independent of how structure is produced. For example if GMCs form, the development of spurs occurs the same way, through the velocity field of the interarm region causing the GMCs to be sheared into large scale filamentary features. The exception is when the instability producing the spurs are associated with resonances in the disc and thereby occur at certain radii in the galaxy (Chakrabarti et al., 2003).
Other simulations focused on the driving of structure in the interstellar medium due to supernova feedback (Rosen and Bregman, 1995; Gazol-Patiño and Passot, 1999; Korpi et al., 1999; Wada et al., 2000; Wada and Norman, 2001; de Avillez and Breitschwerdt, 2005; Slyz et al., 2005; Joung and Mac Low, 2006). These simulations, a precursor of the more recent Simulating the lifecycle of molecular Clouds (SILCC) simulations (Walch et al., 2015), typically model a section of galaxy disc and include cooling and heating of the ISM via a cooling curve, stellar feedback and a vertical disc gravitational potential.
The ISM in these simulations with supernovae feedback is characterised by cold filamentary structures embedded in a warmer medium, and superbubbles generated by supernovae. Cold clouds are able to form even in the absence of self gravity, where supernovae shells collide, though self gravity significantly effects the density PDF (Joung and Mac Low, 2006). In the absence of any spiral structure the gas does not form larger clouds, and the clouds tend to be very filamentary. Even when self gravity is included, the ISM in these simulations shows only small scale structure (Wada and Norman, 2001; Jeffreson et al., 2021) (for Milky Way or LMC type galaxies, for gas rich low rotation systems which do form massive clumps see, e.g. Escala and Larson, 2008).
As simulations started to include most of the key physical processes relevant to molecular cloud formation and evolution, namely gravitational instabilities, thermodynamics of the ISM and stellar feedback, it started to be possible to investigate molecular cloud properties in simulations. Including such physics produces a realistic population of clouds whose properties and lifetimes can be evaluated, albeit these may change depending on the details of the feedback, and the inclusion of other processes the most important of which is likely magnetic fields.
The past decade or so has seen a greater concentration on the modelling of feedback processes. This is due to a number of factors, including the realisation that stellar feedback is required to create realistic disc galaxies, with the focus on feedback in larger scale simulations leading to interest of the effects of feedback more generally on galactic and ISM evolution. In particular, feedback has been thought to be necessary to produce a low star formation efficiency, and is also required to produce a realistic three phase medium. Including feedback is complex since there are many different processes to consider, so identifying the role of each has required a large investment of time to incorporate processes into numerical codes, and a large number of simulations, thus feedback has probably been the main focus of simulations in the last decade or so.
Separating galaxy scale simulations, subgalactic scale simulations and those of individual molecular clouds or colliding flows is difficult, since many processes are investigated across simulations covering multiple scales. Instead references are still included to smaller (molecular cloud) scale simulations and their relation to larger scales considered.
2 Molecular cloud formation
The processes by which molecular clouds are thought to form are described in detail in Dobbs et al. (2014). Here there is a stronger focus on the numerical simulations which demonstrate cloud formation by different mechanisms, and the different conditions under which these mechanisms will dominate cloud formation.
Any instability in the gas, perturbations or development of structure in the gas, will promote thermal instabilities, and lead to denser structures in the cold stable HI phase of the ISM. In the absence of gravity or magnetic fields, such instabilities are the main driver of structure. Converging or colliding flows, which could nonetheless result from spiral arms or supernova explosions into surrounding media, allow the accumulation of more significant amounts of thermally unstable gas. Simulations have modelled the thermal instability in converging flows, by modelling smooth colliding flows seeded with a small perturbation, for example a sinusoidal density perturbation (Hennebelle and Pérault, 1999; Koyama and Inutsuka, 2002; Heitsch et al., 2006). These simulations show that the thermal instability is able to drive the formation of small clouds. Inoue and Inutsuka (2009) suggest that repeated compression of gas leads to the build up of larger clouds. Alternatively turbulence readily drives the formation of cold structures in the gas (Audit and Hennebelle, 2005). With the inclusion of gravity, these clouds collapse to form stars (Vázquez-Semadeni et al., 2000). In the presence of a sufficiently strong magnetic field, magnetic pressure can suppress structure formation via thermal instability perpendicular to the field (see review by Hennebelle and Inutsuka, 2019 and references therein). Perturbations are expected to be preferentially along the field lines, although with a non uniform field there will be some component of pressure parallel to the field, and Alfvén waves can also contribute a pressure force in this direction (Martin et al., 1997; Falceta-Gonçalves et al., 2003; Pinto et al., 2012). Simulations on a galaxy scale show that gas can readily cool when converging flows occur at spiral arms (Dobbs, 2008).
A magnetic medium which is otherwise non-self gravitating, and isothermal, but subject to a vertical gravitational potential, may be subject to Parker instabilities whereby denser gas accumulates in the troughs in the magnetic field. Simulations by Kim et al. (1998) show that the density enhancement due to Parker instabilities alone is only a factor of a few, so not viable to form clouds. However Kim and Ostriker (2000) suggest that Parker instabilities could seed gravitational instabilities, and likewise Kosiński and Hanasz (2006) show that Parker instabilities can induce thermal instabilities and thus form molecular clouds. Parker instabilities combined with cooling lead to the formation of cold dense HI clouds in galaxy scale simulations by Körtgen et al. (2019).
Once structure occurs in the gas, through cold HI clouds, or molecular clouds, it is possible for cloud-cloud collisions to occur. These are much more likely to occur in spiral arms, where a greater amount of dense gas is present, and where the orbits of the clouds converge (Dobbs and Bonnell, 2006). Cloud-cloud collisions are evident in simulations of whole galaxies with cold gas (Dobbs and Bonnell, 2006; Tasker et al., 2008; Skarbinski et al., 2023). In galaxies like the Milky Way, cloud-cloud collisions may typically be fairly gentle events with low Mach numbers (e.g. Skarbinski et al., 2023), rather than violent ‘collisions’ and may not generally have much effect on the star formation rate, unless the velocities involved are quite high. However they may at least in some cases gather large volumes of cold gas together into more massive clouds.
With the inclusion of self gravity of the gas, the gas is subject to gravitational instabilities. In simulations with warm gas, Jeans instabilities in the gas are clearly evident, since the gas is smooth and cloud-cloud collisions are minimal, cooling is not present, and as mentioned in the previous section the spacing of the structures in the gas can be equated to the Jeans length (Kim and Ostriker, 2006; Dobbs, 2008). With cooling, the sound speed of the gas is variable in the ISM, allowing gravity to induce structure over the range of wavelengths which are unstable (Elmegreen, 1989).
Finally molecular cloud formation can be induced by colliding flows from supernovae or winds. As mentioned above, supernovae are often assumed to lead to the converging flow set-ups considered in those types of simulations, and are presumed to drive the formation of structure in vertical box models of the ISM. This scenario has been modelled most explicitly by Dawson et al. (2015) who performed simulations of two supernovae. The gas is compressed where the supernovae winds collide, becoming thermally unstable similar to the previous models. The morphology of the region is highly structured, ascribed to Rayleigh Taylor instabilities.
Once all the above physics is included in large scale simulations, it is difficult to cleanly distinguish which processes are dominating. It is likely that all the above are producing at least some effect on the gas distribution and the resultant properties of GMCs. However Parker instabilities seem to play a smaller role, or only operate in conjunction with other processes, whilst gravitational instabilities, and cloud-cloud collisions (particularly in spiral arms) seem to be required to produce more massive clouds.
2.1 ISM properties
The development and evolution of GMCs in a larger galactic context is interrelated with the wider scale properties of the ISM. The main properties of the ISM that we can check are the amount of gas in different phases, scale heights of phases, and the structure of the components of the ISM. Observationally there are large uncertainties, and significant variations on the amount of gas in different phases, even just considering spiral galaxies. For the Milky Way, observations suggest that molecular gas constitutes around one-third of the mass of the ISM (Kennicutt and Evans, 2012). For the atomic gas, roughly one-third lies in each of the cold, unstable and warm phases (McClure-Griffiths et al., 2023). Thus overall around half the gas may lie in the cold molecular or cold atomic phases and the other half in the unstable or warm regime (the mass of the hot ionised component is negligible). Some galaxies with higher surface densities, e.g. M51 will be mostly molecular, whilst there will also be radial variation within galaxies, for example the inner spiral arms or molecular ring in the Milky Way has a higher molecular fraction (Sanders et al., 1984; Bronfman et al., 1988).
Most simulations of isolated Milky Way like galaxies have shown fractions of gas in these phases in agreement with the observations (Harfst et al., 2006; Dobbs, 2008; Dobbs et al., 2011a; Hill et al., 2012). Modelling of dwarf galaxies shows that the cold gas is dominated by HI rather than H2 (Hu et al., 2016). The amount of gas in different phases will be set mainly by the dust content of the simulated ISM (see Section 4), the chemistry scheme (or explicit cooling curve) used in the code, as well as the resolution (see Kim and Ostriker, 2017). At solar metallicity, the chemistry, heating and cooling scheme will produce a 104 K component (warm neutral medium) which starts to transition to the cold phase of the ISM around 0.1 cm−3. Once gas reaches 100 cm−3 it should be fully in the cold phase unless directly impacted by feedback. This should then lead to realistic gas fractions in the given components, with supernovae feedback producing hotter gas, though if feedback is non-existent (in the presence of self gravity), or too strong then the cold components will be too large or too small respectively. Cosmic rays will also heat the ISM and effect the transition between warm and cold gas (Huang et al., 2022; Kim et al., 2023), though Rathjen et al. (2021) find that they only have a modest influence on gas phases and star formation in their simulations of the ISM. Simulations indicate that at large distances above the midplane cosmic rays dominate over the thermal pressure (Wiener et al., 2013; Salem and Bryan, 2014; Girichidis et al., 2018) since supernovae are absent at these scale heights (Girichidis et al., 2018).
Scale heights of the gas are typically larger than would occur solely from the thermal sound speed, rather they reflect the velocity dispersion associated with turbulence in the ISM. So, for example the scale height of the cold ISM is around 100 pc, whilst the warm neutral medium the scale height is around 400 pc, the warm or hot ionised medium several 100’s parsecs. Simulations with feedback can reproduce these scale heights (Rosen and Bregman, 1995; Dobbs et al., 2011a; Hill et al., 2012; Kim and Ostriker, 2017; Benincasa et al., 2020b). MRI can also increase the scale height of the gas. In simulations by Piontek and Ostriker (2007) the scale height of the cold gas is around 20 or 40 pc, and the unstable medium around 150 pc, so this mechanism alone is probably not sufficiently to account for the observed values. Hill et al. (2012) find that the addition of the magnetic field does not have a significant impact on the scale height of the gas in their simulation, though it will have a moderate effect on the proportion of gas in different phases due to the magnetic pressure leading to more lower density gas.
2.2 Cloud properties and lifetimes
Simulations of isolated galaxies can typically resolve clouds of 104–107 M⊙, and thus it is possible to start to look at cloud mass spectra, Larson’s scaling relations and cloud rotations. Clouds can be selected from simulations using a surface or volume density criterion (Dobbs and Bonnell, 2006; Tasker and Tan, 2009; Jeffreson et al., 2020), friends of friends algorithm (Benincasa et al., 2013; Dobbs and Pringle, 2013), or observationally orientated algorithms such as clumpfind (Grisdale et al., 2018) or cprops (Dobbs et al., 2019). Figure 1 shows a galaxy simulation by Jeffreson et al. (2020), where the gas is postprocessed to calculate molecular gas fractions and clouds identified at a given surface density contour.
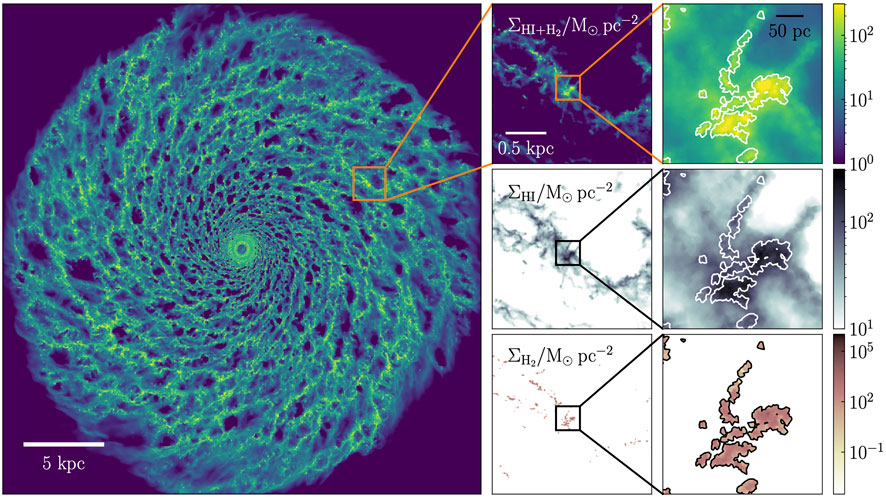
FIGURE 1. Molecular cloud identification in a galaxy simulation by Jeffreson et al. (2020) (Figure 1) at a time of 600 Myr. The left hand panel shows the total gas column density, and the right hand panels a 2kpc region seen in total, molecular and atomic column densities, with an example GMC extracted.
Simulations obtain cloud mass spectra dN/dM ∝ Mα where α is typically in the range −2.5 to −1.5 (Wada et al., 2000; Harfst et al., 2006; Tasker and Tan, 2009; Khoperskov et al., 2013; Jeffreson et al., 2020). Observations find similar results (Fukui et al., 2001; Rosolowsky et al., 2003; Roman-Duval et al., 2010; Rice et al., 2016). Wada et al. (2000) show that a steeper mass spectrum is obtained when including stellar feedback compared to without, and the mass spectrum truncates at around 106 M⊙ compared to 107 M⊙, indicating that feedback is preventing the formation of more massive clouds. Some results show that without feedback, the mass spectrum is incompatible with observations with too many massive GMCs (Dobbs et al., 2011a; Grisdale et al., 2018). This is in contradiction to Tasker and Tan (2009) and Wada et al. (2000), where clouds do not seem to require feedback to disperse (rather this is attributed to velocities induced by cloud collisions), presumably due to differences between the numerical codes used. The mass spectrum may vary slightly according to environment, e.g. spiral arms (Colombo et al., 2014) or the Outer Galaxy (Padoan et al., 2016), whilst the slope may depend on the algorithm to find the clouds (Dobbs et al., 2019), and the viewing perspective of the clouds (Khoperskov et al., 2016). Minor differences may occur according to the nature of the spiral arms (Pettitt et al., 2020).
Global disc or spiral arm scale simulations are able to reproduce the Larson’s relations (Dobbs et al., 2011a; Benincasa et al., 2013; Falceta-Gonçalves et al., 2015; Khoperskov et al., 2016), but show quite a lot of scatter compared to at least early Milky Way studies (e.g. the original Larson, 1981 results). They tend to show better correspondence with more recent observational extragalactic studies (Colombo et al., 2014; Sun et al., 2018). This could indicate that more recent results are less hampered by interdependencies between different observed cloud properties, the size of the aperture or whether clouds/apertures are used (Spilker et al., 2022), or that there is a greater variation with environment when considering whole galaxies (Rice et al., 2016). The latter is also supported by Padoan et al. (2016) who only consider the Outer Galaxy environment and find a similar degree of scatter in both observations and simulations.
Molecular clouds are considered bound if their virial prameter
(e.g. Bertoldi and McKee, 1992) is
Cloud rotations are another diagnostic by which to check whether the simulations are producing GMCs which match the properties of observed GMCs (Phillips, 1999; Imara and Blitz, 2011; Imara et al., 2011; Braine et al., 2018). AMR simulations can have a tendency to produce GMCs with excessive angular momentum, apparent as spiral shaped rotating clouds, but Seifried et al. (2017) show that with careful restraints on refinement, this issue is avoided. Simulations show that GMCs formed via gravitational instability tend to exhibit prograde rotation (i.e. their internal rotation is the same as that of the galaxy), but cloud-cloud collisions can lead to GMCs rotating in the opposite direction exhibiting retrograde rotation (Dobbs, 2008; Tasker and Tan, 2009; Aouad et al., 2020; Jeffreson et al., 2020). The distributions of prograde and retrograde clouds match well the observational results. Williamson et al. (2014) also find that stellar feedback increases the fraction of retrograde clouds, whilst in the Galactic Centre, shear may determine cloud rotation (Kruijssen et al., 2019).
Simulations indicate that cloud-cloud collisions are expected to occur reasonably frequently in spiral galaxies. For example Tasker and Tan (2009) give a time of 1/5 of an orbital time between collisions, Dobbs et al. (2015) around 8 Myr. Cloud-cloud collisions in a non-interacting galaxy simulation typically occur at velocities of a few km s−1 (Dobbs et al., 2015; Skarbinski et al., 2023) to around 10 km s−1 (Fujimoto et al., 2014), with a maximum velocity of around 20 km s−1. This is in line with Milky Way observations, whereby the highest velocity collisions are estimated to be around 15–20 km s−1 (Furukawa et al., 2009; Fukui et al., 2015; Schneider et al., 2023).
Cloud lifetimes are discussed extensively in Chevance et al. (2022), but an overview of the simulation side is presented here as well. Most simulations appear to produce GMCs with fairly short lifetimes, typically around 4–10 Myr (Dobbs and Pringle, 2013; Rogers and Pittard, 2013; Kim and Ostriker, 2018; Benincasa et al., 2020a), with cloud disruption occurring due to feedback. These lifetimes are at the lower end of most observational studies. Dobbs and Pringle (2013) find the longest lived clouds associated with the most massive, virialised clouds. Benincasa et al. (2020a) see no relation of cloud lifetime with mass, but do similarly see longer lifetimes with lower virial parameter clouds. Jeffreson et al. (2021) find longer lived clouds, with lifetimes of 10–40 Myr. They introduce a momentum feedback scheme which effects the degree of clustering of supernovae and can reduce or increase cloud lifetimes. However even without this scheme clouds would be longer lived compared to the other results, and, for example the simulations by Kim and Ostriker (2018) are already high enough resolution to presumably resolve clustering of massive stars. Possibly differences in how clouds are identified between models may contribute to different timescales, as well as any differences in feedback prescriptions.
3 Feedback
Earlier isolated galaxy studies tested whether feedback produces a realistic ISM, and GMC properties in agreement with observations. Driven at least partly by observational studies, the focus of more recent work has moved towards the localised relation between GMCs and star formation, for example the lifetimes of GMCs as discussed above, the time for clusters to move from the embedded to exposed phase, and the most important form of feedback driving these timescales.
Of earlier isolated galaxy simulations with feedback, Wada et al. (2000) include test star particles representing massive stars, which inject energy continuously to represent winds, and deposit thermal energy equivalent to 1051 ergs each time a supernova occurs. Tasker (2011) include photoelectric heating in the form of a radially dependent heating rate around star particles, which similar to Wada et al. (2000), are inserted when gas is cold and dense. Both of these works simulate disc galaxies, but with highly flocculent spiral structure only arising in the gas. Dobbs et al. (2011a) include a simplified feedback prescription, whereby energy is inserted instantaneously following star formation a snowlplough solution. Hopkins et al. (2011) include feedback as an input of momentum in clumpy regions identified as star-forming. Both Dobbs et al. (2011a) and Hopkins et al. (2011) obtain realistic star formation rates regulated by feedback (the former using a galaxy with a spiral potential, the latter using a live stellar disc), though an efficiency parameter for the feedback is required. In the simulations by Tasker (2011), the heating does not appear to have a large effect on the morphology of the galaxy, the star formation rate, or the properties of the clouds, although it does seem to affect their rotation. The supernovae schemes in Wada et al. (2000) and Dobbs et al. (2011a), in contrast have a strong impact on the disc, and the resulting clouds. As mentioned above Wada et al. (2000) see the maximum mass truncated with their supernovae plus winds scheme, whilst other simulations see a bimodal mass spectrum dominated by high mass clouds (Dobbs et al., 2011a; Grisdale et al., 2018). Hopkins et al. (2011) also find models with cooling and self gravity, but no stellar feedback problematic, seeing the formation of very massive clouds, runaway collapse to high densities, high star formation rates and unrealistic galaxy morphologies. The differences are probably two fold. First, Tasker (2011) see shorter lived clouds forming even without feedback, kept in virial equilibrium through cloud-cloud collisions. Wada et al. (2000) are also able to run simulations without feedback without seeing a build up of high mass clouds. Secondly the photoionising heating scheme is probably gentler than a large deposit of energy more characteristic of the supernovae schemes.
In more recent simulations, the emphasis has been to consider the timescale of different feedback processes, including early (e.g. photoionisation, winds) and late (e.g. supernovae) feedback. Iffrig and Hennebelle (2015) find that the location of supernovae, whether they are randomly distributed in the ISM when they occur, or distributed in dense gas, makes a large difference on the galactic structure and star formation rate. Gatto et al. (2015) also find that if supernovae occur in dense gas this leads to both too little molecular gas and too much hot gas. Many smaller scale simulations over the past decade have pointed towards ionisation being effective in dispersing clouds before supernovae occur (see Figure 2) on timescales of a few or several Myr (Dale et al., 2005; Sales et al., 2014; Geen et al., 2016; Gavagnin et al., 2017; Peters et al., 2017; Ali et al., 2018; Kim and Ostriker, 2018; Haid et al., 2019; Fukushima et al., 2020; González-Samaniego and Vazquez-Semadeni, 2020; Lucas et al., 2020; Dobbs et al., 2022a; Bending et al., 2022).
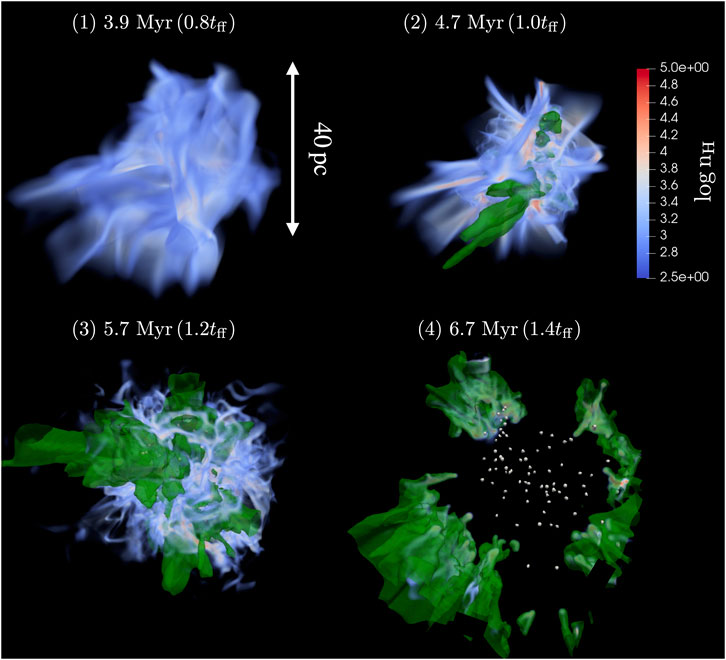
FIGURE 2. A 3D volume rendering of the density field for a 105 M⊙, solar metallicity cloud subject to ionising radiation is shown at four different times, taken from Fukushima et al. (2020) (Figure 2). The ionisation fronts are shown in green, and the white dots in the final panel represent the positions of the star cluster particles. The length scale for each panel is 40 pc.
Ionisation is more difficult to resolve on larger scales, and typically (though see Benincasa et al., 2020b) simulations heat gas to 104 K within a Stromgen radius (Baba et al., 2017; Marinacci et al., 2019; Jeffreson et al., 2021). Ionisation has been included in whole galaxy simulations but there is not a clear consensus yet on the role of ionisation on larger scales. Rosdahl et al. (2015) find that ionisation is gentler and less effective than supernovae whilst Benincasa et al. (2020b) find supernovae are required in addition to ionisation to regulate the star formation rate in their simulations. Hu et al. (2016) find supernovae are dominant in simulations of dwarf galaxies. However Jeffreson et al. (2021) highlight the importance of photoionisation at earlier times (compared to supernovae) to regulate cloud lifetimes, whilst Stinson et al. (2013) find early feedback is important for cosmological simulations. It seems likely that both play a role, e.g. photoionisation initially creates cavities and shapes the gas but this is further accentuated by supernovae (Rogers and Pittard, 2013; Grudić et al., 2022; Herrington et al., 2023). The current literature seems to find that on larger scales supernovae and ionisation are dominant compared to winds (Marinacci et al., 2019; Ali et al., 2022) and radiation pressure (Marinacci et al., 2019). Photoionisation may have a greater role for simulations whereby gravitational collapse to high densities occurs without early feedback.
The role of feedback is likely to be complex and will vary according to length and time scales, and not necessarily always to lower the star formation rate. On larger galaxy scales, and over 10s or 100 s Myr timescales, feedback is required to prevent the accumulation of most of the gas into massive long lived clouds which would turn most of their gas into stars. Instead feedback keeps clouds in a continuous state of flux, their lifetimes relatively short, retains high velocity dispersions in the ISM and returns gas to lower, warmer phases of the interstellar medium, keeping the star formation moderate. Other processes, such as gravity, spiral shocks and cloud-cloud collisions (see next section) may drive turbulence, which similarly contributes to the galaxy wide velocity dispersion but as discussed in Section 2.2, simulations are somewhat divided as to whether these are sufficient to maintain a population of turbulent, short-lived clouds. Cloud-cloud collisions are usually found to produce similar or higher star formation rates compared to clouds in isolation (Wu et al., 2017; Dobbs et al., 2020; Liow and Dobbs, 2020; Tanvir and Dale, 2020; Hunter et al., 2023)). On intermediate scale however, feedback may have more of a triggering effect. This is not always so evident in numerical simulations, since if they typically only model an isolated cloud, the feedback simply escapes into a vacuum. Observers and theorists have nevertheless long suggested that feedback triggers star formation (e.g. Elmegreen and Lada, 1977; McCray and Kafatos, 1987; Tenorio-Tagle and Palous, 1987; Elmegreen, 2002). Herrington et al. (2023) find that when including supernovae from a previous generation of stars, the star formation rate is increased compared to not having a preexisting population. This is due to the supernovae (and to a lesser extent ionisation) compressing structure in the gas to higher densities, and thereby forming stars. Comparing this to the picture by Inutsuka et al. (2015), whereby molecular clouds are envisioned to lie at the edges of expanding shells, it may be that most star formation occurs in this way. In equilibrium, the feedback simply converts a continuous steady amount of the ISM into star forming gas. On smaller, core scales, feedback again appears to reduce star formation (e.g. Guszejnov et al., 2022).
The impact of the magnetic field on the larger scale effects of feedback has been investigated by a few authors. Using both theoretical arguments and simulations, Leão et al. (2009) find that the size of supernovae remnants is reduced in the presence of a strong magnetic field, so predict that supernovae may have a limiting contribution to the effectiveness of feedback in shaping the ISM and triggering new star formation. Iffrig and Hennebelle (2015) also run simulations of supernovae occurring in a magnetic medium, and find that supernovae combined with a magnetic field are better able to drive the velocity dispersion of the gas. Similar results are seen in simulations of a vertically stratified section of disc (Iffrig and Hennebelle, 2017), and comparing the resulting density distributions (their Figure 1), the inclusion of a magnetic field actually appears to produce clearer cavities.
The role of feedback in shaping the structure of the ISM on galaxy scales is apparent in, for example the observations of holes in HI (Bagetakos et al., 2011) or more recently PHANGS maps of galaxies (Watkins et al., 2023a; Watkins et al., 2023b). The holes are widespread and a substantial component by area. Cavities can form simply from the gaps between sheared molecular clouds, however such cavities would be strongly sheared in the interarm regions (see, e.g. shells subjects to shear modelled by Tenorio-Tagle and Palous (1987)). Whereas, for example in Watkins et al. (2023a), although highly elliptical bubbles are associated with dynamical creation or older bubbles, many more circular bubbles are also present. Watkins et al. (2023b) also show that the sizes and expansions of these features is consistent with having been caused by supernovae from the underlying young stellar population. Observations show the formation of molecular clouds on the edges of superbubble regions (Tanaka et al., 2007; Dawson et al., 2011; Dawson et al. 2013; Sano et al., 2018; Bialy et al., 2021). More locally, nearby star forming regions appear to be shaped by feedback, and exhibit age spreads consistent with a sequence of star formation occurring, then triggering either new structures or collapse in preexisting dense or molecular clouds (Oey et al., 2005; Großschedl et al., 2021; Miret-Roig et al., 2022; Zucker et al., 2022).
3.1 Turbulent driving
Simulations without feedback have suggested that turbulence may be driven by gravitational instabilities (Wada and Norman, 1999; Wada et al., 2002; Tasker and Tan, 2009), MRI (Kim et al., 2003) or shear (Fleck, 1981; Meidt et al., 2018). This might be more relevant in some environments, e.g. dwarf galaxies or low star forming galaxies (Wada et al., 2002; Agertz et al., 2009), or the outer parts of galaxies, where feedback is minimal. Thermal instability has also been suggested, which can induce velocities of a few km s−1 (Koyama and Inutsuka, 2002). Spiral arms are also able to induce velocity dispersions in the spiral arms, both from the shock (Kim et al., 2006; Dobbs and Bonnell, 2007), and tidal forces acting over larger scales along the spiral arm (Falceta-Gonçalves et al., 2015). Most consensus however points towards supernovae driving velocity dispersions of several km s−1 up to 100–200 pc scales (Slyz et al., 2005; Dib et al., 2006; Joung and Mac Low, 2006; Dobbs et al., 2011a; Hill et al., 2012; Gent et al., 2013; Lu et al., 2020; Herrington et al., 2023). There is still some debate though whether feedback alone drives turbulence, and whether feedback or gravity is the source of the shape of the velocity power law spectrum. Based on modelling sections of the ISM, Seifried et al. (2018) suggest that supernovae may not be frequent enough to maintain velocity dispersions at large distances from clouds. Supernovae, or other feedback from massive stars, may also not be able to explain turbulence deeper within molecular clouds which do not contain massive stars. Ejdetjärn et al. (2022) find in disc galaxy simulations that the velocities reached are the same, due to gravity, regardless of feedback. Most simulations however find a larger velocity dispersion when supernovae are included and that the velocity dispersion increases as a function of how many supernovae occur. Colman et al. (2022) drive turbulence explicitly on given scales as may be typified by feedback processes operating on particular distance scales, but find that feedback alone is insufficient to explain the observed power spectrum particular at the largest scales, whilst Smith et al. (2022) similarly find that turbulence needs to be driven across multiple scales to produce realistic star clusters. Grisdale et al. (2017) suppose that a combination of gravity, shear and feedback drive the turbulent power spectrum. Bournaud et al. (2010) suggest that gravitational instabilities produce the velocity power law (see also Fensch et al., 2023) but feedback is essential to transform dense gas to the diffuse phase, and replenish the low density, high velocity dispersion gas covering larger scales.
On cloud scales, photoionisation has been found to produce a Kolmogorov type spectrum by Boneberg et al. (2015), but it is not clear how much photoionisation drives turbulence on galaxy scales (e.g. Vandenbroucke and Wood, 2019). Herrington et al. (2023) find that supernovae drive larger velocity dispersions compared to photoionisation. Sartorio et al. (2021) also find that if turbulence is already driven by a larger scale process, ionisation from a nearby source may have limited impact on the velocity and density fields.
4 Chemistry
In terms of chemistry, isolated galaxy studies have concentrated on including molecular hydrogen and CO formation in their models. Many of these studies have investigated the formation of molecular hydrogen in different environments, and whether such clouds could be observed in CO. But also a second reason for the inclusion of molecular hydrogen formation has been the observation that the Kennicutt Schmidt relation links better to molecular hydrogen than atomic hydrogen or total gas density, so some simulations have used molecular hydrogen density as a threshold for forming stars rather than total density.
The formation of molecular hydrogen and CO are governed by basic equations,
and
see e.g. Hollenbach et al. (1971); Nelson and Langer (1997); Bergin et al. (2004). Here, n represent number densities, N is column density, Rgr is the formation rate on grains, ζcr and ζdiss are the cosmic ray and photodissociation rates respectively, k0 is a constant, and τ the optical depth. β and Γ are functions which additionally depend on the OI abundance and the average interstellar radiation field (Habing, 1968). In terms of the properties of the gas, the formation of molecular hydrogen is dependent on density squared and temperature, so even though higher temperatures are more conducive to forming H2, the high density of cold gas means that molecules preferentially form in cold dense regions. As illustrated in Figure 14 of Dobbs (2008), molecular hydrogen formation and destruction versus time exhibits a hysteresis loop. As density increases, H2 fraction increases as molecular hydrogen forms. However once formed, gas can remain molecular at lower densities due to self shielding. Molecular hydrogen formation will also depend on the amount of dust, and the properties of dust grains (see below), which are encapsulated in the Rgr parameter. Simulations on galaxy scales have showed densities, metallicities and temperatures at which H2 forms (Dobbs and Bonnell, 2006; Pelupessy et al., 2006; Dobbs, 2008; Gnedin et al., 2009; Richings et al., 2014; Bellomi et al., 2020; Jeffreson et al., 2020) and that the compression of cold gas in spiral arms or by turbulence is sufficient to form H2. The timescale for gas to move from being atomic to obtaining modest molecular fractions is found to be of order Myr when the gas is compressed by spiral arms of turbulence (Glover and Mac Low, 2007; Dobbs, 2008).
Robertson and Kravtsov (2008) investigated the Kennicutt Schmidt relation and found better agreement with a n = 1.4 power law when using the molecular gas surface density rather than total gas surface density, which gives a cut off at low densities (see also Monaco et al., 2012). Alternatively the H2 surface density can be used when the Kennicutt Schmidt relation is used to input the star formation rate in simulations, in part naturally reducing the star formation efficiency (Lagos et al., 2011; Kuhlen et al., 2012; Sillero et al., 2021; Valentini et al., 2023). By combining with a radiative transfer code, synthetic HI, HISA, CO and also CII maps of galaxies, or sections of galaxies can be produced (Dib and Burkert, 2005; Acreman et al., 2010; Duarte-Cabral et al., 2015; Heiner et al., 2015; Seifried et al., 2020; Ebagezio et al., 2022).
Simulations with chemistry can also be used to determine some measurements which are observationally challenging, e.g. the fraction of dark molecular gas (see Figure 3), the X factor converting from CO intensities to molecular masses, and variation of the X factor with environment. Simulations indicate that there are significant amounts of dark H2 (Dobbs, 2008; Smith et al., 2014; Glover and Smith, 2016; Gong et al., 2018; Seifried et al., 2020) that would not be observed with CO. The X factor has been found to vary with densities, velocity linewidths, star formation rate at high densities, metallicity (Shetty et al., 2011; Clark and Glover, 2015) and cosmic ray ionisation rate (Peñaloza et al., 2018; Bisbas et al., 2021). Seemingly in contrast to Clark and Glover (2015), Bisbas et al. (2021) do not find a variation with FUV, though this could be because they do not consider the same high densities as Clark and Glover (2015).
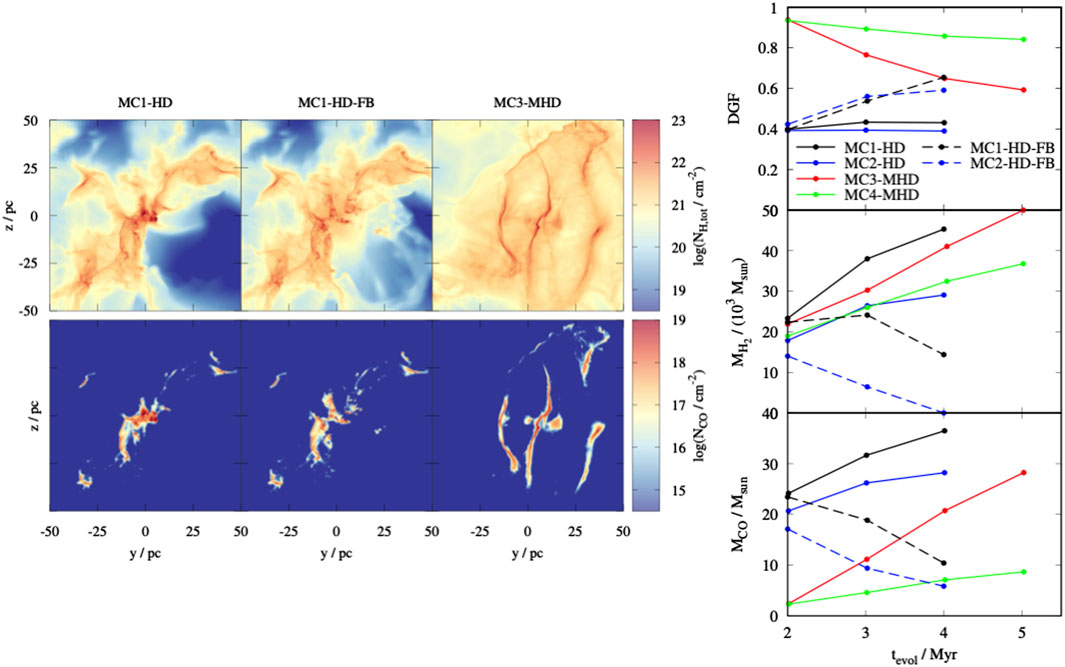
FIGURE 3. The total gas column density (upper left), and CO column density (lower left) are shown from simulations of molecular clouds from Seifried et al. (2020) (Figures 1, 2). The simulations include feedback (centre) and MHD (right). Plots showing the variation of mass of the dark molecular gas fraction, hydrogen and CO are shown on the right.
Uncertainties in determining molecular densities in simulations include the efficiency of H2 formation on grains, self shielding and the UV field, the amount of feedback and the resolution of the simulations. The efficiency of H2 formation on grains may depend on the grain type, and grain sizes which in turn depend on environment and grain growth, (Smoluchowski, 1981; Tielens and Hagen, 1982; Snow, 1983; Biham et al., 1998; Biham et al., 2001; Caselli et al., 1998; Cuppen et al., 2006; Perets et al., 2007). The efficiency will also depend on how quickly hydrogen atoms combine on the surface of grains, the rate of diffusion. This can be determined through numerical calculations and laboratory experiments (Duley, 1996; Ruffle and Herbst, 2000; Hincelin et al., 2015). However there is still some uncertainty of what this quantity will be in astrophysical environments. In particular there is found to be significant dependence on the temperature (Cazaux and Tielens, 2004; Iqbal et al., 2012; Le Bourlot et al., 2012; Grieco et al., 2023).
Self shielding of the molecular hydrogen can be calculated, for example using a typical distance to massive stars (Dobbs and Bonnell, 2006; Khoperskov et al., 2013), or the Jeans length (Glover, 2015), but ideally a ray tracing method or tree based method like treecol can be used to determine a better measure of the column density for self shielding (Clark et al., 2012; Hartwig et al., 2015). The feedback scheme will also affect the amount of molecular hydrogen. So, for example, the amount of H2 will depend both on the level of feedback, and whether feedback quickly disperses a cloud or not (Walch et al., 2015). Finally, although cosmological simulations are keen to include H2 and CO evolution, the results from galaxy and smaller scale simulations, as well as tests by Krumholz and Gnedin (2011), suggest that resolving these species accurately requires quite high resolution. Duarte-Cabral and Dobbs (2016) find that subgalaxy simulations with subparsec resolution achieve higher fractions of H2 compared to lower resolution whole galaxy simulations. Nickerson et al. (2019) perform different resolution galaxy simulations and find that the H2 content is not converged, though their 6 pc resolution simulation produces H2 fractions in agreement with observations. Gong et al. (2018) find that 2 pc resolution is required for convergence of H2, whilst CO is still not converged at 1 pc resolution. Joshi et al. (2019) suggest even higher resolution of 0.2 pc for H2. CO may require a resolution of around 0.1 pc (Joshi et al., 2019; Borchert et al., 2022). Jeffreson et al. (2020) suggest the resolution required may also be sensitive to the calculation of self shielding, but still note high resolution is required.
5 Magnetic fields
A review of the role of magnetic fields on molecular cloud scales is presented in Hennebelle and Inutsuka (2019), whilst Pattle et al. (2022) review observations and simulations again on molecular cloud scales and smaller.
Generally, aside from the possibility of inducing Parker instabilities as discussed above, magnetic fields are thought to reduce and/or slow down star formation in galaxies and molecular clouds, if indeed they have an effect. At the simplest level, magnetic fields exhibit a pressure which on galaxy scales smoothes out structure induced by other processes such as spiral arms and self gravity (Dobbs and Price, 2008; Hill et al., 2012; Schmidt et al., 2013). On cloud scales, the magnetic field may be so strong that the molecular cloud is subcritical, and the magnetic force is stronger than the gravitational force such that the cloud is stabilised against collapse. The condition for this to occur is that the ratio of the cloud mass to the critical mass Mcrit is less than 1, ie.
where ϕB = BπR2 and the first expression is for a uniform spherical cloud, and the second can be applied to line of sight Zeeman measurements (Crutcher, 2004; Krumholz, 2011). Crutcher et al. (2010) estimate that most clouds are supercritical, and find a change from the magnetic field being constant, to having a ρ2/3 dependence at n ∼ 300 cm−3, which they suggest may be where clouds start being gravitationally dominated. Recent simulations by Auddy et al. (2022) of turbulent magnetic ISM are able to reproduce a change in slope, which they find is due to transitioning from sub to supercriticality, and is also dependent on the Alfvén Mach number of the turbulence.
Star formation can commence in subcritical clouds via ambipolar diffusion and/or through accretion of mass onto the cloud which could push the mass over the critical mass. Simulations of molecular cloud formation have studied whether indeed the clouds formed in the simulations are supercritical, and if so whether they undergo a transition from super to subcritical. Körtgen and Banerjee (2015) investigate this problem in simulations of driven turbulence and find that above certain field strengths, the clouds are subcritical and it is difficult for star formation to proceed. Likewise Ostriker et al. (1999) find that subcritical clouds do not collapse. However in galaxy scale simulations, accretion onto molecular clouds readily lead them to become supercritical and thereby collapse (Körtgen et al., 2018). Interestingly Hu et al. (2023) suggest that most clouds, even HI clouds, are supercritical, and simply measurements of subcritical clouds are due to observational biases. On galaxy scales, numerous large scale simulations have shown that magnetic fields suppress the star formation rate by a factor of 2 or 3 (Vázquez-Semadeni et al., 2011; Kim et al., 2021; Wibking and Krumholz, 2023) (see Figure 4). The main exception is recent work by Whitworth et al. (2023), who model dwarf galaxies and find the magnetic field does not effect the star formation rate.
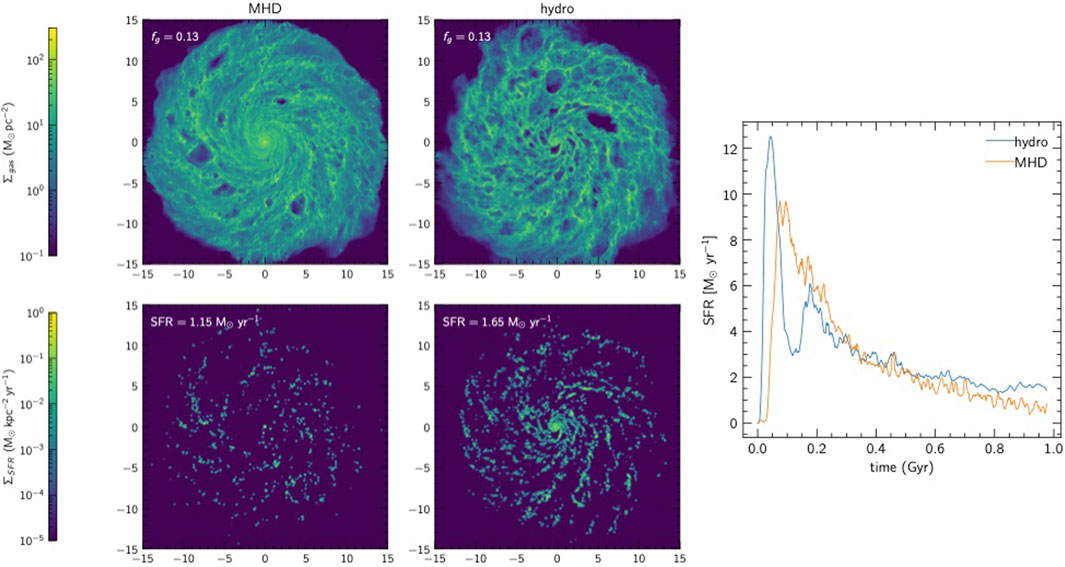
FIGURE 4. Galaxy simulations from Wibking and Krumholz (2023) (Figures 1, 2) with and without magnetic fields are shown at a time of 1 Gyr. The upper panels show the density, and the lower panels show the star formation rate per unit area. The right panel shows the star formation rate over time for the two simulations.
The effects of magnetic fields may also have a directional dependence. This tends to be the case for relatively strong fields, as for weaker fields the field has less impact on the gas. As noted in Hennebelle and Inutsuka (2019), magnetic fields will effect gas differently parallel or perpendicular to the field. Consequently, a strongly magnetised medium may allow the formation of filaments, but be non-conducive to forming more spherical clouds (Heitsch et al., 2009; Inoue et al., 2009; Hennebelle, 2013; Wareing et al., 2016). Observationally magnetic fields are found to be aligned with density structures in the ISM, but at the highest densities, fields become orientated perpendicular to dense filaments (McClure-Griffiths et al., 2006; Alves et al., 2008; Heyer and Brunt, 2012; Clark et al., 2014; Planck Collaboration et al., 2016). The change in direction has been related to the transition to gravitationally dominated structures (Soler et al., 2013; Chen et al., 2020; Barreto-Mota et al., 2021; Girichidis, 2021), though it is worth noting that such a change in orientation has been seen even in simulations without self gravity (Gazol and Villagran, 2021). The behaviour of gas at shocks will also differ according to magnetic field orientation. Again for weak fields, the orientation makes little difference. However for strong fields, the magnetic field will be amplified if the field is parallel to the shock. Consequently, if the field is parallel to the shock, when the field is amplified, the gas becomes magnetically supported and star formation is prevented. Dobbs and Wurster (2021) find that the star formation is delayed rather than prevented, but the morphology of the region is somewhat different. Another consequence is that lower density shocked regions are likely to occur when the field is parallel to the shock, and higher density regions when the field is perpendicular, similar to observations.
It was previously supposed that magnetic fields might prevent dissipation of turbulence, and hence prolong star formation (e.g. Shu et al., 1987). However simulations find that once clouds are supercritical, the timescale for collapse with magnetic fields is not significantly longer than the unmagnetised case (Mac Low et al., 1998; Ostriker et al., 1999), with the proviso that locally there may be more variation due to directional dependence on the influence of the magnetic field. As such, it may be that magnetic fields keep mass critically supported, rather than slowing down the process of star formation substantially (Vázquez-Semadeni et al., 2011).
A caveat with both simulations of turbulence and magnetic fields, is that the resolution of the simulations is insufficient to model the full range of Reynolds numbers (Elmegreen and Scalo, 2004), or the range of Prandtl numbers (Nixon and Pringle, 2019), expected for the ISM.
6 Environment
So far we have considered different physical processes that could largely be considered independently of galactic environment. However a galaxy contains multiple environments in which different processes may dominate, and which exhibit different characteristics. Isolated galaxy simulations have the advantage of being able to model different regions simultaneously.
One of the longstanding questions in star formation is whether spiral arms trigger star formation, or simply gather gas, which would anyway be forming stars, together. Spiral arms represent regions of lower shear, and the gas may shock as it traverses the minimum of the spiral potential (Roberts, 1969). If the gas is relatively uniform low density, and undergoes a shock at the spiral arms, then it may well reach densities high enough to induce molecule formation and subsequently star formation. So, for example, in simulations without self gravity, molecular gas is predominantly situated in the spiral arms, but atomic between spiral arms (Dobbs and Bonnell, 2006; Dobbs, 2008; Kim et al., 2008; Kim et al., 2010), and thus the spiral arms are the dominant mechanism driving molecular, or dense gas formation. However if the gas already lies in relatively dense clouds, or filaments, then the spiral arms may simply act to gather these clouds and filaments together. In later simulations which include self gravity, spiral arms and feedback (Dobbs et al., 2011a; Pettitt et al., 2017; Kim et al., 2020; Tress et al., 2020), there is little difference in the star formation rate with and without spiral arms, suggesting that spiral arms do not have a big impact on star formation. As shown in Duarte-Cabral and Dobbs (2017) gas entering the spiral arms already includes giant molecular filaments, sheared into their elongated shapes during the inter-arm region passage. The main impact seems to be the formation of a few more massive clouds when spiral arms are included, which though they can have a disproportionate effect on the star formation rate, do not hugely increase star formation (Dobbs and Pringle, 2013; Dobbs et al., 2017).
The outer regions of galaxies are typically associated with less star formation, including less massive star formation. Schaye (2004) predicted that the edge of the cold component of galaxies coincides with the range of star formation in the disc. Inspired by GALEX observations of extended massive star formation, beyond the readily observable galaxy discs, Bush et al. (2010) showed in simulations that spiral arms out to large radii could allow star formation to proceed at the outskirts of galaxies. Likewise Smith et al. (2023) find cold gas forming in spiral arms at the edge of galaxies. As mentioned above, other means of driving turbulence may be required at the outer galaxy rather than relying on massive stars, though Dib et al. (2006) suggest supernovae may be sufficient depending on the star formation efficiency. Also, galaxies are not in isolation, and accretion of gas may promote both turbulence and star formation at the edge of galaxy discs. A few simulations have shown that the impact of high velocity clouds can drive turbulence and possibly induce a mini-starburst (Santillán et al., 2007; Alig et al., 2018), whilst more generally, the accretion rate onto galaxies may be sufficient to drive turbulence, and allow star formation to continue at a steady rate (Klessen and Hennebelle, 2010; Burkert, 2017).
Similarly to the spiral arms, simulations of the bar region also suggest that cloud mergers are more frequent leading to the formation of more massive clouds (Renaud et al., 2013; Fujimoto et al., 2014). Renaud et al. (2013) also highlight the role of resonances in channeling gas to the ends of the bar to form massive clouds and clusters (Renaud et al., 2015; Ali et al., 2023) (Figure 5). Most work on galaxy centres has focused on our on Galactic Centre. In simulations of the Galactic Centre, the bar region also channels gas into the galactic centre (Shin et al., 2017; Sormani et al., 2018; 2019; Tress et al., 2020; Moon et al., 2021), fuelling the nuclear ring. Simulations predict that the fuelling of gas leads to collisions with very high velocities, up to ∼ 200 km s−1 (Sormani et al., 2019; Hatchfield et al., 2021). Observationally, molecular clouds at the Galactic Centre show lower star formation rates than their disc equivalents (Longmore et al., 2013) so simulations of the Galactic Centre have been used to try and understand why this is. Bertram et al. (2015) perform simulations of molecular clouds subject to conditions at the Galactic Centre, including a strong radiation field representative of the Galactic Centre, but find that star formation still occurs within a gravitational free fall time, and that the amount of star formation is higher than observed. However by including the orbit of the clouds at the Galactic Centre, Kruijssen et al. (2019) find that shear and torque are also important conditions needed to better reproduce the observed properties of the clouds, and that the tidal field at the Galactic Centre is required (Dale et al., 2019).
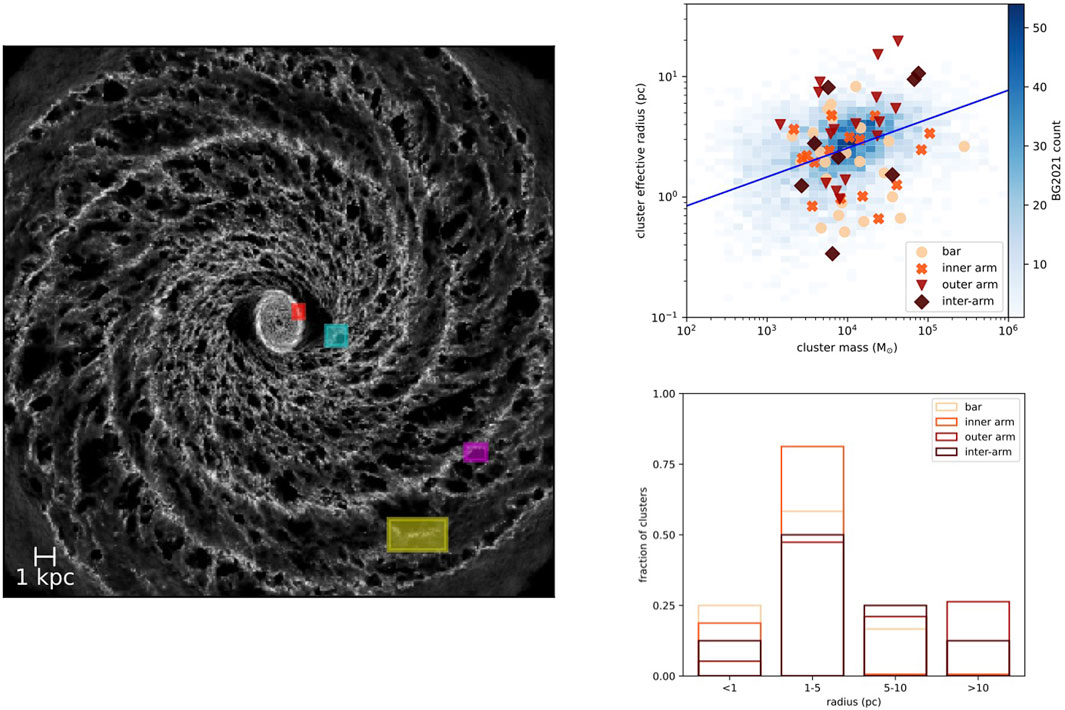
FIGURE 5. Regions from a barred galaxy simulation which are resimulated at higher resolution are shown in the left panel, from Ali et al. (2023) (Figures 1, 7). The right panels show the variation of cluster properties with environment, in particular the outer galaxy and inter-arm reigons produce larger radius clusters, whereas the bar and inner spiral arm produce more compact clusters.
The above simulations of the Galactic Centre aim to model the central part of the Milky Way, but otherwise the above calculations largely model generic Milky Way like spiral or barred spiral galaxies. An alternative approach is to try and model specific galaxies, usually by running many lower resolution, or N-body simulations to determine a good match. This has the advantage that a more robust, and absolute comparison with observational properties can be made since the properties of the simulated and observed galaxy, such as surface densities, rotation curve, spiral pattern roughly match. Quite a few studies have attempted to find a match to the Milky Way by trying to reproduce the CO map of the Galaxy (Wada et al., 1994; Englmaier and Gerhard, 1999; Fux, 1999; Bissantz et al., 2003; Rodriguez-Fernandez and Combes, 2008; Baba et al., 2009; Khoperskov et al., 2013; Pettitt et al., 2014; Pettitt et al., 2015; Li et al., 2016; Li et al., 2022). The CO map has the advantage that it traces fairly narrow features compared with stars or HI. Such simulations give insight on, for example the pattern speed and pitch angle of the spiral arms, pattern speed and length of the bar, and how well a fixed spiral pattern versus live potential match the CO observations. In some ways modelling external galaxies is easier since we have face on observations to compare with. For external galaxies, simulations have been able to successfully reproduce the large scale structure of M51 (Salo and Laurikainen, 2000a; Salo and Laurikainen, 2000b; Dobbs et al., 2010; Tress et al., 2020), M33 (Dobbs et al., 2018; Semczuk et al., 2018) and barred galaxies NGC4303 and NGC 3627 (Iles et al., 2022). Of those simulations which include molecular cloud analysis, Dobbs et al. (2019) are able to reproduce the radial variation of clouds in M33, and find that the molecular cloud properties depend primarily on gas surface density, and less on the spiral structure and stellar feedback. Other studies similarly find that the molecular cloud properties are less dependent on interactions such as that of M51, and again primarily dependent on the gas density, and level of feedback (Pettitt et al., 2020; Tress et al., 2020). An alternative approach by Sills et al. (2023) is to use observational gas densities and velocities as initial conditions, there for the Orion cloud, rather than a whole galaxy, although they had to make some assumptions about the cloud’s properties to set up full 6D positions and velocities.
7 Sub-galactic simulations or simulations resolving sub-cloud structure
Simulations which span between the scales of clouds and galaxies have some key advantages compared to larger galaxy or smaller cloud scale simulations. It is difficult to well resolve clusters, or feedback processes over entire galaxy scales. Conversely, simulations on molecular cloud scales do not typically include larger galactic scale processes such as spiral arms, bars, larger supernovae bubbles, which may influence star formation. Galaxy scale simulations in general lack the resolution to resolve the detailed structure of molecular clouds, the full cloud population down to 1,000 or 100 M⊙, or clumps or cores within molecular clouds. A number of approaches have been made to incorporate galaxy scale effects simultaneously with high resolution. These include shearing box simulations, taking a section from a galaxy scale simulation and re-resolving at higher resolution, taking a vertical section of the galactic plane, and setting a higher level of resolution within a region of the galaxy disc. Shearing box simulations have the advantage that they can include spiral arms, and the gas is periodic, so such simulations can be run for a long period of time (10’s or even 100’s Myrs). It is difficult however to model, for example a bar, or study the radial variation within a galaxy. The vertical section simulations have similar advantages and disadvantages, in that they can be run relatively long, but tend to only include variation in the z direction, so shear, spiral arms and galactic rotation are not typically included. Simulations which remodel a section of a galaxy have the advantage that they can be relatively flexible, e.g. different regions can be selected, and it can be easy to include a potential or live stellar disc to allow the gas to follow the large scale dynamics. However such simulations can only be run for limited timescales due both to the boundary conditions and the increased computational cost at higher resolution. Allocating high resolution in a small part of the disc is the most satisfactory, as most of these disadvantages are not present, though the computational costs for including high resolution are still high so it is difficult to run the refined or high resolution simulations for long periods of time. For all these methods there are still concerns about the boundaries, or transition between different resolutions (e.g. Seifried et al., 2017; Herrington et al., 2023).
The TIGRESS simulations (Kim and Ostriker, 2017; Kim et al., 2020), building on previous work started by (Kim and Ostriker, 2002), model a shearing box of ISM with photoelectric heating, supernovae and MHD. The inclusion of galactic rotation is found to be important for clouds to be sheared out and preventing large scale gravitational collapse. The simulations are run for around 700 Myr which allows some semi-equilibrium state to be reached within the region (after around 100 Myr). The star formation rate is nevertheless cyclical, which leads to the emergence of galactic winds, which then fall back down on the disc (Kim and Ostriker, 2018). Kim et al. (2020) also include a spiral potential. The SILCC simulations model a vertical section of the disc, resolving the formation of molecular clouds, concentrating on molecular chemistry and the effects of feedback (Walch et al., 2015).
Other simulations have modelled a small region taken from a galaxy simulation (Van Loo et al., 2013; Bonnell et al., 2013; Butler et al., 2015; Dobbs, 2015; Smilgys and Bonnell, 2017; Bending et al., 2020; Dobbs et al., 2022a; Dobbs et al., 2022b; Bending et al., 2020; Herrington et al., 2023; Ali et al., 2023). Some of the more recent of these papers focus more on clusters, following the evolution of clusters represented by groups of sink particles, but can also include initial conditions which have arisen from global galaxy simulations. Dobbs et al. (2022a) show that particularly massive clusters may emerge at the sites of strongly converging flows in galaxy, and that photoionisation is necessary to produced the observed radii of stellar clusters, whilst Dobbs et al. (2022b) show the emergence of OB associations in lower density regions of converging flows. Simulations on these scales also indicate that clusters, at least more massive clusters, appear to be formed hierarchically, through the mergers of smaller clusters (Smilgys and Bonnell, 2017; Dobbs et al., 2022b).
Smith et al. (2020) run a galaxy scale simulation and then modify the resolution within a 3 kpc box after the simulation reaches 150 Myr. This means they can continue modelling the whole galaxy, but simultaneously follow the formation and evolution of filamentary structures on small scales (Figure 6). They follow the evolution of filaments which are forming stars, whereby feedback breaks up the filaments, and quiescent filaments which undergo gravitational fragmentation. Renaud et al. (2013) also achieve very high resolution in their galaxy scale simulation, but in their case they re-resolve the whole galaxy simulation, again after the simulation has already run for about 240 Myr.
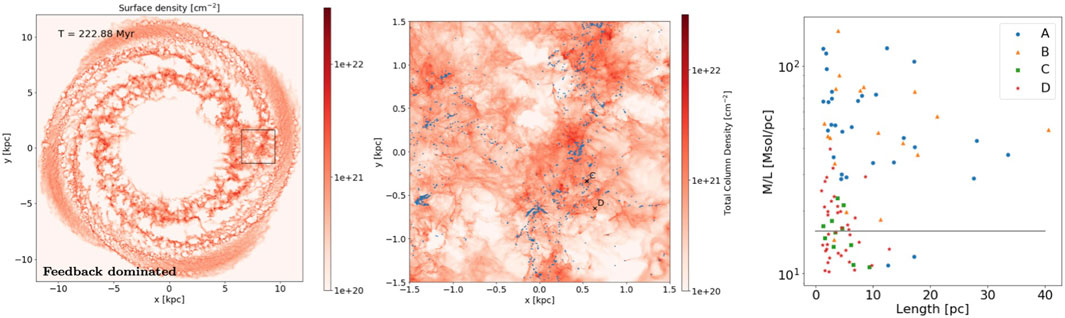
FIGURE 6. A galaxy simulation is shown from Smith et al. (2020) (Figures 1, 10) (left) and a zoom in high resolution section with higher refinement is shown in the middle panel. The right hand panel shows the distribution of filament mass to length ratios and lengths, the letters C and D represent star forming regions dominated by feedback, whilst A and B represent regions dominated by the spiral arm potential where little feedback has occurred.
8 Conclusion and looking ahead
Simulations on isolated galaxy and subgalactic scales over the last 25 years or so have played a key role in developing our understanding of molecular cloud evolution and star formation within molecular clouds. Some of the earlier numerical work, carried out in the 2000s, focused on testing theories about instabilities in the ISM and molecular cloud formation that had been put forward in the previous decades, but which were unable to be investigated numerically until computers were sufficiently powerful to enable hydrodynamic simulations on kpc or galaxy scales. The simulations also took advantage of the fact that in the 1980s and 1990s the first observational surveys of molecular clouds gave statistical properties to compare with, and that the first surveys of clouds in external galaxies were starting to be made. With regards to molecular cloud formation, gravitational instabilities coupled with cooling, and cloud-cloud collisions are likely to be the most important processes in most galactic environments. Feedback is probably the main driver of the observed velocity dispersions in molecular clouds, though gravity and convergent flows or tidal velocity fields may also be significant, particularly in regions less impacted by massive stars. Cloud mass spectra, which are reproduced in most simulations unless they are dominated by gravitational collapse into massive clouds, can be explained theoretically either through gravitational instabilities in a multiphase medium (Elmegreen, 1989), or through cloud-cloud collisions (Kwan, 1979). Again probably both are present.
As the resolution of CO surveys has improved, observations have been able to probe both a larger range of external galaxies (e.g. PHANGS Leroy et al., 2021) and environments within galaxies. At the same time, simulations have investigated the role of spiral arms, bars and galactic centres. So far spiral arms are not found to have a large role in the properties of molecular clouds or galactic star formation rates, as found in both simulations and observations. Though spiral arms do appear to be able to produce more massive, and highly star forming GMCs. Bars may be more complex, as they host low density, low star forming regions, regions with strong shear channeling gas to the centre, as well as massive highly star forming regions. The last two of these have at least been investigated in simulations of bars and the Galactic Centre. So far there has been a considerable advance of our understanding of star formation at the Galactic Centre through simulations and observations. However it is not clear how typical our Galaxy Centre is compared to galaxies generally in the local Universe.
Since most surveys focus on the inner parts of galaxies, outer regions have not been such a focus of more recent work, however they may become increasingly significant if we are to understand the longer term evolution, and the role of accretion. Zoom in models of individual galaxies from cosmological simulations may be preferable in this case since they capture the surrounding environment. The LYRA simulations (Gutcke et al., 2022) take initial conditions from the EAGLE simulations and achieve 4 M⊙ per cell resolution but focus on dwarf galaxies. The VINTERGATAN zoom in simulations of the Milky Way (Agertz et al., 2021) have a gas mass resolution of 7070 M⊙, which is a slightly higher than isolated spiral galaxy simulations but unlike isolated galaxy models, these were run for the entirety of the Milky Way’s history.
The past decade or so has seen much more interest in linking the smaller scale effects of feedback with the global evolution of clouds. Most simulations now find fairly short lifetimes, as based on the constituent gas present in the cloud, not the lifetime of H2 molecules. Most work on smaller scales indicates the role of photoionisation in immediately dispersing gas from clouds, in agreement with observations (Chevance et al., 2020) although on larger galactic scales, it is not clear whether photoionisation, supernovae, or both equally drive the structure. The role of magnetic fields has been studied considerably in simulations of turbulence in the ISM, but more simulations are now starting to look at MHD on galaxy scales.
So far most simulations have investigated the properties of the gas, but a logical next step, resources and codes provided, would be to study the formation of clusters, OB associations and if applicable, isolated star formation. Following the full formation and evolution of clusters by resolving the formation of individual stars is still restricted to relatively small cloud scales, and achieving this on large, e.g. galaxy scales seems impractical for the foreseeable future. For example to resolve individual star formation down to brown dwarfs at the opacity limit with sink particles, Bate et al. (2003) resolve densities of 10–13 g cm−3, and use particle masses of 10–5 M⊙. On a galactic scale modelling a fairly low mass of gas of 109 M⊙ with an SPH code would require 1014 SPH particles. Even if a single particle represented a solar or higher mass star this would still require 10 billion SPH particles. However there are still ways to more approximately model and follow cluster formation and evolution. One approach is to semi-evolve the clusters by adopting a subgrid model for their formation and dispersal. This approach has the advantage that it can be implemented on any scale simulation, on galaxy scales and cosmological scales (Pfeffer et al., 2018; Reina-Campos et al., 2022; Grudić et al., 2023). For example in Reina-Campos et al. (2022) each star particle formed in a star formation event then represents the fraction of star formation that ends up in a bound cluster. An alternative is simply to use sink or star particles (Hennebelle and Iffrig, 2014; Gatto et al., 2017; Geen et al., 2018; Kim and Ostriker, 2018; Smith et al., 2020; Dobbs et al., 2022a; Li et al., 2022), but each of these still represents a group of stars rather than a single star particle.
An approach which potentially allows the full modelling of stellar clusters even with low resolution in the gas is to decouple the gas resolution from that of the stars. When star formation occurs, star particles are inserted with masses according to an assumed IMF (Hirai et al., 2021; Rieder et al., 2022; Lahén et al., 2023). For example Rieder et al. (2022) model the formation of clusters resolving all stars with masses down to 0.1 M⊙ in a section of a spiral galaxy. Lahén et al. (2023) also model the full IMF of stars in simulations of dwarf galaxies. There are still drawbacks to this method, for example a decision still has to be made about the spatial and velocity distribution of the stars when they added. However reasonable estimates can be made given the spatial dimensions of the simulation (Liow et al., 2022). Hybrid N-body + hydrodynamics codes (Fujii et al., 2021; Rieder et al., 2022) enable simulations to be more tractable with very large numbers of star particles, as quickly becomes the case once spatial scales become the size of clouds or larger.
Author contributions
CD: Writing–original draft, Writing–review and editing.
Funding
The author(s) declare financial support was received for the research, authorship, and/or publication of this article. CD is funded by the European Research Council H2020-EU.1.1 ICYBOB project (Grant No. 818940).
Conflict of interest
The author declares that the research was conducted in the absence of any commercial or financial relationships that could be construed as a potential conflict of interest.
Publisher’s note
All claims expressed in this article are solely those of the authors and do not necessarily represent those of their affiliated organizations, or those of the publisher, the editors and the reviewers. Any product that may be evaluated in this article, or claim that may be made by its manufacturer, is not guaranteed or endorsed by the publisher.
References
Acreman, D. M., Douglas, K. A., Dobbs, C. L., and Brunt, C. M. (2010). Synthetic HI observations of a simulated spiral galaxy. MNRAS 406, 1460–1470. doi:10.1111/j.1365-2966.2010.16858.x
Agertz, O., Lake, G., Teyssier, R., Moore, B., Mayer, L., and Romeo, A. B. (2009). Large-scale galactic turbulence: can self-gravity drive the observed HI velocity dispersions? MNRAS 392, 294–308. doi:10.1111/j.1365-2966.2008.14043.x
Agertz, O., Renaud, F., Feltzing, S., Read, J. I., Ryde, N., Andersson, E. P., et al. (2021). VINTERGATAN - I. The origins of chemically, kinematically, and structurally distinct discs in a simulated Milky Way-mass galaxy. MNRAS 503, 5826–5845. doi:10.1093/MNRAS/stab322
Ali, A., Harries, T. J., and Douglas, T. A. (2018). Modelling massive star feedback with Monte Carlo radiation hydrodynamics: photoionization and radiation pressure in a turbulent cloud. MNRAS 477, 5422–5436. doi:10.1093/MNRAS/sty1001
Ali, A. A., Bending, T. J. R., and Dobbs, C. L. (2022). Stellar winds and photoionization in a spiral arm. MNRAS 510, 5592–5602. doi:10.1093/MNRAS/stac025
Ali, A. A., Dobbs, C. L., Bending, T. J. R., Buckner, A. S. M., and Pettitt, A. R. (2023). Star cluster formation and feedback in different environments of a Milky Way-like galaxy. MNRAS 524, 555–568. doi:10.1093/MNRAS/stad1917
Alig, C., Hammer, S., Borodatchenkova, N., Dobbs, C. L., and Burkert, A. (2018). Simulating the impact of the Smith cloud. Astrophysical J. 869, L2. doi:10.3847/2041-8213/aaf1cb
Alves, F. O., Franco, G. A. P., and Girart, J. M. (2008). Optical polarimetry toward the Pipe nebula: revealing the importance of the magnetic field. A&A 486, L13–L16. doi:10.1051/0004-6361:200810091
Aouad, C. J., James, P. A., and Chilingarian, I. V. (2020). Coupling local to global star formation in spiral galaxies: the effect of differential rotation. MNRAS 496, 5211–5226. doi:10.1093/MNRAS/staa1945
Auddy, S., Basu, S., and Kudoh, T. (2022). The magnetic field versus density relation in star-forming molecular clouds. Astrophysical J. 928, L2. doi:10.3847/2041-8213/ac5a5a
Audit, E., and Hennebelle, P. (2005). Thermal condensation in a turbulent atomic hydrogen flow. A&A 433, 1–13. doi:10.1051/0004-6361:20041474
Baba, J., Asaki, Y., Makino, J., Miyoshi, M., Saitoh, T. R., and Wada, K. (2009). The origin of large peculiar motions of star-forming regions and spiral structures of our galaxy. Astrophysical J. 706, 471–481. doi:10.1088/0004-637X/706/1/471
Baba, J., Morokuma-Matsui, K., and Saitoh, T. R. (2017). Eventful evolution of giant molecular clouds in dynamically evolving spiral arms. MNRAS 464, 246–263. doi:10.1093/MNRAS/stw2378
Bagetakos, I., Brinks, E., Walter, F., de Blok, W. J. G., Usero, A., Leroy, A. K., et al. (2011). The fine-scale structure of the neutral interstellar medium in nearby galaxies. AJ 141, 23. doi:10.1088/0004-6256/141/1/23
Barreto-Mota, L., de Gouveia Dal Pino, E. M., Burkhart, B., Melioli, C., Santos-Lima, R., and Kadowaki, L. H. S. (2021). Magnetic field orientation in self-gravitating turbulent molecular clouds. MNRAS 503, 5425–5447. doi:10.1093/MNRAS/stab798
Bate, M. R., Bonnell, I. A., and Bromm, V. (2003). The formation of a star cluster: predicting the properties of stars and brown dwarfs. MNRAS 339, 577–599. doi:10.1046/j.1365-8711.2003.06210.x
Bellomi, E., Godard, B., Hennebelle, P., Valdivia, V., Pineau des Forêts, G., Lesaffre, P., et al. (2020). 3D chemical structure of diffuse turbulent ISM. I. Statistics of the HI-to-H2 transition. A&A 643, A36. doi:10.1051/0004-6361/202038593
Bending, T. J. R., Dobbs, C. L., and Bate, M. R. (2020). Photoionizing feedback in spiral arm molecular clouds. MNRAS 495, 1672–1691. doi:10.1093/MNRAS/staa1293
Bending, T. J. R., Dobbs, C. L., and Bate, M. R. (2022). Supernovae and photoionizing feedback in spiral arm molecular clouds. MNRAS 513, 2088–2099. doi:10.1093/MNRAS/stac965
Benincasa, S. M., Loebman, S. R., Wetzel, A., Hopkins, P. F., Murray, N., Bellardini, M. A., et al. (2020a). Live fast, die young: GMC lifetimes in the FIRE cosmological simulations of Milky Way mass galaxies. MNRAS 497, 3993–3999. doi:10.1093/MNRAS/staa2116
Benincasa, S. M., Tasker, E. J., Pudritz, R. E., and Wadsley, J. (2013). Giant molecular cloud formation in disk galaxies: characterizing simulated versus observed cloud catalogs. Astrophysical J. 776, 23. doi:10.1088/0004-637X/776/1/23
Benincasa, S. M., Wadsley, J. W., Couchman, H. M. P., Pettitt, A. R., Keller, B. W., Woods, R. M., et al. (2020b). The anatomy of a star-forming galaxy II: FUV heating via dust. MNRAS 499, 2028–2041. doi:10.1093/MNRAS/staa2935
Bergin, E. A., Hartmann, L. W., Raymond, J. C., and Ballesteros-Paredes, J. (2004). Molecular cloud formation behind shock waves. Astrophysical J. 612, 921–939. doi:10.1086/422578
Bertoldi, F., and McKee, C. F. (1992). Pressure-confined clumps in magnetized molecular clouds. Astrophysical J. 395, 140. doi:10.1086/171638
Bertram, E., Glover, S. C. O., Clark, P. C., and Klessen, R. S. (2015). Star formation efficiencies of molecular clouds in a galactic centre environment. MNRAS 451, 3679–3692. doi:10.1093/MNRAS/stv1239
Bialy, S., Zucker, C., Goodman, A., Foley, M. M., Alves, J., Semenov, V. A., et al. (2021). The per-tau shell: a giant star-forming spherical shell revealed by 3D dust observations. Astrophysical J. 919, L5. doi:10.3847/2041-8213/ac1f95
Biham, O., Furman, I., Katz, N., Pirronello, V., and Vidali, G. (1998). H2 formation on interstellar grains in different physical regimes. MNRAS 296, 869–872. doi:10.1046/j.1365-8711.1998.01427.x
Biham, O., Furman, I., Pirronello, V., and Vidali, G. (2001). Master equation for hydrogen recombination on grain surfaces. Astrophysical J. 553, 595–603. doi:10.1086/320975
Bisbas, T. G., Tan, J. C., and Tanaka, K. E. I. (2021). Photodissociation region diagnostics across galactic environments. MNRAS 502, 2701–2732. doi:10.1093/MNRAS/stab121
Bissantz, N., Englmaier, P., and Gerhard, O. (2003). Gas dynamics in the Milky Way: second pattern speed and large-scale morphology. MNRAS 340, 949–968. doi:10.1046/j.1365-8711.2003.06358.x
Boneberg, D. M., Dale, J. E., Girichidis, P., and Ercolano, B. (2015). Turbulence in giant molecular clouds: the effect of photoionization feedback. MNRAS 447, 1341–1352. doi:10.1093/MNRAS/stu2498
Bonnell, I. A., Dobbs, C. L., and Smith, R. J. (2013). Shocks, cooling and the origin of star formation rates in spiral galaxies. MNRAS 430, 1790–1800. doi:10.1093/MNRAS/stt004
Borchert, E. M. A., Walch, S., Seifried, D., Clarke, S. D., Franeck, A., and Nürnberger, P. C. (2022). Synthetic CO emission and the XCO factor of young molecular clouds: a convergence study. MNRAS 510, 753–773. doi:10.1093/MNRAS/stab3354
Bournaud, F., Elmegreen, B. G., Teyssier, R., Block, D. L., and Puerari, I. (2010). ISM properties in hydrodynamic galaxy simulations: turbulence cascades, cloud formation, role of gravity and feedback. MNRAS 409, 1088–1099. doi:10.1111/j.1365-2966.2010.17370.x
Braine, J., Rosolowsky, E., Gratier, P., Corbelli, E., and Schuster, K. F. (2018). Properties and rotation of molecular clouds in M 33. A&A 612, A51. doi:10.1051/0004-6361/201732405
Bronfman, L., Cohen, R. S., Alvarez, H., May, J., and Thaddeus, P. (1988). A CO survey of the southern Milky way: the mean radial distribution of molecular clouds within the solar circle. Astrophysical J. 324, 248. doi:10.1086/165892
Burkert, A. (2017). A bathtub model for the star-forming interstellar medium. Mem. della Soc. Astron. Ital. 88, 533. doi:10.48550/arXiv.1709.02112
Bush, S. J., Cox, T. J., Hayward, C. C., Thilker, D., Hernquist, L., and Besla, G. (2010). Spiral-induced star formation in the outer disks of galaxies. Astrophysical J. 713, 780–799. doi:10.1088/0004-637X/713/2/780
Butler, M. J., Tan, J. C., and Van Loo, S. (2015). Kiloparsec-scale simulations of star formation in disk galaxies III. Structure and dynamics of filaments and clumps in giant molecular clouds. Astrophysical J. 805, 1. doi:10.1088/0004-637X/805/1/1
Caselli, P., Hasegawa, T. I., and Herbst, E. (1998). A proposed modification of the rate equations for reactions on grain surfaces. Astrophysical J. 495, 309–316. doi:10.1086/305253
Cazaux, S., and Tielens, A. G. G. M. (2004). H2 formation on grain surfaces. Astrophysical J. 604, 222–237. doi:10.1086/381775
Chakrabarti, S., Laughlin, G., and Shu, F. H. (2003). Branch, spur, and feather formation in spiral galaxies. Astrophysical J. 596, 220–239. doi:10.1086/377578
Chen, C.-Y., Mundy, L. G., Ostriker, E. C., Storm, S., and Dhabal, A. (2020). Self-gravitating filament formation from shocked flows: velocity gradients across filaments. MNRAS 494, 3675–3685. doi:10.1093/MNRAS/staa960
Chevance, M., Kruijssen, J. M. D., Hygate, A. P. S., Schruba, A., Longmore, S. N., Groves, B., et al. (2020). The lifecycle of molecular clouds in nearby star-forming disc galaxies. MNRAS 493, 2872–2909. doi:10.1093/MNRAS/stz3525
Chevance, M., Krumholz, M. R., McLeod, A. F., Ostriker, E. C., Rosolowsky, E. W., and Sternberg, A. (2022). The life and times of giant molecular clouds. arXiv e-prints, arXiv:2203.09570.
Clark, P. C., and Glover, S. C. O. (2015). Does the CO-to-H2 conversion factor depend on the star formation rate? MNRAS 452, 2057–2070. doi:10.1093/MNRAS/stv1369
Clark, P. C., Glover, S. C. O., and Klessen, R. S. (2012). TreeCol: a novel approach to estimating column densities in astrophysical simulations. MNRAS 420, 745–756. doi:10.1111/j.1365-2966.2011.20087.x
Clark, S. E., Peek, J. E. G., and Putman, M. E. (2014). Magnetically aligned H I fibers and the rolling hough transform. Astrophysical J. 789, 82. doi:10.1088/0004-637X/789/1/82
Colman, T., Robitaille, J.-F., Hennebelle, P., Miville-Deschênes, M.-A., Brucy, N., Klessen, R. S., et al. (2022). The signature of large-scale turbulence driving on the structure of the interstellar medium. MNRAS 514, 3670–3684. doi:10.1093/MNRAS/stac1543
Colombo, D., Hughes, A., Schinnerer, E., Meidt, S. E., Leroy, A. K., Pety, J., et al. (2014). The PdBI arcsecond whirlpool survey (PAWS): environmental dependence of giant molecular cloud properties in M51. Astrophysical J. 784, 3. doi:10.1088/0004-637X/784/1/3
Combes, F., and Gerin, M. (1985). Spiral structure of molecular clouds in response to bar forcing: a particle simulation. A&A 150, 327–338.
Combes, F., and Sanders, R. H. (1981). Formation and properties of persisting stellar bars. A&A 96, 164–173.
Crutcher, R. M. (2004). What drives star formation? Ap&SS 292, 225–237. doi:10.1023/B:ASTR.0000045021.42255.95
Crutcher, R. M., Wandelt, B., Heiles, C., Falgarone, E., and Troland, T. H. (2010). Magnetic fields in interstellar clouds from zeeman observations: inference of total field strengths by bayesian analysis. Astrophysical J. 725, 466–479. doi:10.1088/0004-637X/725/1/466
Cuppen, H. M., Morata, O., and Herbst, E. (2006). Monte Carlo simulations of H2 formation on stochastically heated grains. MNRAS 367, 1757–1765. doi:10.1111/j.1365-2966.2006.10079.x
Dale, J. E., Bonnell, I. A., Clarke, C. J., and Bate, M. R. (2005). Photoionizing feedback in star cluster formation. MNRAS 358, 291–304. doi:10.1111/j.1365-2966.2005.08806.x
Dale, J. E., Kruijssen, J. M. D., and Longmore, S. N. (2019). The dynamical evolution of molecular clouds near the Galactic Centre - III. Tidally induced star formation in protocluster clouds. MNRAS 486, 3307–3326. doi:10.1093/MNRAS/stz888
Dawson, J. R., McClure-Griffiths, N. M., Kawamura, A., Mizuno, N., Onishi, T., Mizuno, A., et al. (2011). Supershells as molecular cloud factories: parsec resolution observations of H I and 12CO(J = 1-0) in GSH 287+04-17 and GSH 277+00+36. Astrophysical J. 728, 127. doi:10.1088/0004-637X/728/2/127
Dawson, J. R., McClure-Griffiths, N. M., Wong, T., Dickey, J. M., Hughes, A., Fukui, Y., et al. (2013). Supergiant shells and molecular cloud formation in the large magellanic cloud. Astrophysical J. 763, 56. doi:10.1088/0004-637X/763/1/56
Dawson, J. R., Ntormousi, E., Fukui, Y., Hayakawa, T., and Fierlinger, K. (2015). A young giant molecular cloud formed at the interface of two colliding supershells: observations meet simulations. Astrophysical J. 799, 64. doi:10.1088/0004-637X/799/1/64
de Avillez, M. A., and Breitschwerdt, D. (2005). Global dynamical evolution of the ISM in star forming galaxies. I. High resolution 3D simulations: effect of the magnetic field. A&A 436, 585–600. doi:10.1051/0004-6361:20042146
Dib, S., Bell, E., and Burkert, A. (2006). The supernova rate-velocity dispersion relation in the interstellar medium. Astrophysical J. 638, 797–810. doi:10.1086/498857
Dib, S., and Burkert, A. (2005). On the origin of the H I holes in the interstellar medium of dwarf irregular galaxies. Astrophysical J. 630, 238–249. doi:10.1086/431785
Dobbs, C. L. (2008). GMC formation by agglomeration and self gravity. MNRAS 391, 844–858. doi:10.1111/j.1365-2966.2008.13939.x
Dobbs, C. L. (2015). The interstellar medium and star formation on kpc size scales. MNRAS 447, 3390–3401. doi:10.1093/MNRAS/stu2585
Dobbs, C. L., Adamo, A., Few, C. G., Calzetti, D., Dale, D. A., Elmegreen, B. G., et al. (2017). The properties, origin and evolution of stellar clusters in galaxy simulations and observations. MNRAS 464, 3580–3596. doi:10.1093/MNRAS/stw2200
Dobbs, C. L., Bending, T. J. R., Pettitt, A. R., and Bate, M. R. (2022a). The formation of massive stellar clusters in converging galactic flows with photoionization. MNRAS 509, 954–973. doi:10.1093/MNRAS/stab3036
Dobbs, C. L., Bending, T. J. R., Pettitt, A. R., Buckner, A. S. M., and Bate, M. R. (2022b). The formation of clusters and OB associations in different density spiral arm environments. MNRAS 517, 675–696. doi:10.1093/MNRAS/stac2474
Dobbs, C. L., and Bonnell, I. A. (2006). Spurs and feathering in spiral galaxies. MNRAS 367, 873–878. doi:10.1111/j.1365-2966.2006.10146.x
Dobbs, C. L., and Bonnell, I. A. (2007). Clumpy and fractal shocks, and the generation of a velocity dispersion in molecular clouds. MNRAS 374, 1115–1124. doi:10.1111/j.1365-2966.2006.11227.x
Dobbs, C. L., Burkert, A., and Pringle, J. E. (2011a). The properties of the interstellar medium in disc galaxies with stellar feedback. MNRAS 417, 1318–1334. doi:10.1111/j.1365-2966.2011.19346.x
Dobbs, C. L., Burkert, A., and Pringle, J. E. (2011b). Why are most molecular clouds not gravitationally bound? MNRAS 413, 2935–2942. doi:10.1111/j.1365-2966.2011.18371.x
Dobbs, C. L., Krumholz, M. R., Ballesteros-Paredes, J., Bolatto, A. D., Fukui, Y., Heyer, M., et al. (2014). “Formation of molecular clouds and global conditions for star Formation,” in Protostars and planets VI. Editors H. Beuther, R. S. Klessen, C. P. Dullemond, and T. Henning (United States: University of Arizona Press). doi:10.2458/azu_uapress_9780816531240-ch001
Dobbs, C. L., Liow, K. Y., and Rieder, S. (2020). The formation of young massive clusters by colliding flows. MNRAS 496, L1–L5. doi:10.1093/MNRASl/slaa072
Dobbs, C. L., Pettitt, A. R., Corbelli, E., and Pringle, J. E. (2018). Simulations of the flocculent spiral M33: what drives the spiral structure? MNRAS 478, 3793–3808. doi:10.1093/MNRAS/sty1231
Dobbs, C. L., and Price, D. J. (2008). Magnetic fields and the dynamics of spiral galaxies. MNRAS 383, 497–512. doi:10.1111/j.1365-2966.2007.12591.x
Dobbs, C. L., and Pringle, J. E. (2013). The exciting lives of giant molecular clouds. MNRAS 432, 653–667. doi:10.1093/MNRAS/stt508
Dobbs, C. L., Pringle, J. E., and Duarte-Cabral, A. (2015). The frequency and nature of ‘cloud-cloud collisions’ in galaxies. MNRAS 446, 3608–3620. doi:10.1093/MNRAS/stu2319
Dobbs, C. L., Rosolowsky, E., Pettitt, A. R., Braine, J., Corbelli, E., and Sun, J. (2019). Comparing the properties of GMCs in M33 from simulations and observations. MNRAS 485, 4997–5009. doi:10.1093/MNRAS/stz674
Dobbs, C. L., Theis, C., Pringle, J. E., and Bate, M. R. (2010). Simulations of the grand design galaxy M51: a case study for analysing tidally induced spiral structure. MNRAS 403, 625–645. doi:10.1111/j.1365-2966.2009.16161.x
Dobbs, C. L., and Wurster, J. (2021). The properties of clusters, and the orientation of magnetic fields relative to filaments, in magnetohydrodynamic simulations of colliding clouds. MNRAS 502, 2285–2295. doi:10.1093/MNRAS/stab150
Duarte-Cabral, A., Acreman, D. M., Dobbs, C. L., Mottram, J. C., Gibson, S. J., Brunt, C. M., et al. (2015). Synthetic CO, H2 and H I surveys of the second galactic quadrant, and the properties of molecular gas. MNRAS 447, 2144–2158. doi:10.1093/MNRAS/stu2586
Duarte-Cabral, A., Colombo, D., Urquhart, J. S., Ginsburg, A., Russeil, D., Schuller, F., et al. (2021). The SEDIGISM survey: molecular clouds in the inner Galaxy. MNRAS 500 (3), 3027–3049. doi:10.1093/MNRAS/staa2480
Duarte-Cabral, A., and Dobbs, C. L. (2016). What can simulated molecular clouds tell us about real molecular clouds? MNRAS 458, 3667–3683. doi:10.1093/MNRAS/stw469
Duarte-Cabral, A., and Dobbs, C. L. (2017). The evolution of giant molecular filaments. MNRAS 470, 4261–4273. doi:10.1093/MNRAS/stx1524
Duley, W. W. (1996). The formation of H2 by H-atom reaction with grain surfaces. MNRAS 279, 591–594. doi:10.1093/MNRAS/279.2.591
Ebagezio, S., Seifried, D., Walch, S., Nürnberger, P. C., Rathjen, T. E., and Naab, T. (2022). CO and [CII] line emission of molecular clouds – the impact of stellar feedback and non-equilibrium chemistry. arXiv e-prints , arXiv:2206.06393.
Ejdetjärn, T., Agertz, O., Östlin, G., Renaud, F., and Romeo, A. B. (2022). From giant clumps to clouds - III. The connection between star formation and turbulence in the ISM. MNRAS 514, 480–496. doi:10.1093/MNRAS/stac1414
Elmegreen, B. G. (1989). Molecular cloud formation by gravitational instabilities in a clumpy interstellar medium. Astrophysical J. 344, 306. doi:10.1086/167798
Elmegreen, B. G. (2002). Star Formation from galaxies to globules. Astrophysical J. 577, 206–220. doi:10.1086/342177
Elmegreen, B. G., and Elmegreen, D. M. (1983). Regular strings of HII regions and superclouds in spiral galaxies: clues to the origin of cloudy structure. MNRAS 203, 31–45. doi:10.1093/MNRAS/203.1.31
Elmegreen, B. G., and Lada, C. J. (1977). Sequential formation of subgroups in OB associations. Astrophysical J. 214, 725–741. doi:10.1086/155302
Elmegreen, B. G., and Scalo, J. (2004). Interstellar turbulence I: observations and processes. ARA&A 42, 211–273. doi:10.1146/annurev.astro.41.011802.094859
Englmaier, P., and Gerhard, O. (1999). Gas dynamics and large-scale morphology of the Milky Way galaxy. MNRAS 304, 512–534. doi:10.1046/j.1365-8711.1999.02280.x
Escala, A., and Larson, R. B. (2008). Stability of galactic gas disks and the formation of massive clusters. Astrophysical J. 685, L31–L34. doi:10.1086/592271
Evans, I., Neal, J., Heyer, M., Miville-Deschênes, M.-A., Nguyen-Luong, Q., and Merello, M. (2021). Which molecular cloud structures are bound? Astrophysical J. 920, 126. doi:10.3847/1538-4357/ac1425
Falceta-Gonçalves, D., Bonnell, I., Kowal, G., Lépine, J. R. D., and Braga, C. A. S. (2015). The onset of large-scale turbulence in the interstellar medium of spiral galaxies. MNRAS 446, 973–989. doi:10.1093/MNRAS/stu2127
Falceta-Gonçalves, D., de Juli, M. C., and Jatenco-Pereira, V. (2003). Dusty molecular cloud collapse in the presence of Alfvén waves. Astrophysical J. 597, 970–974. doi:10.1086/378584
Fensch, J., Bournaud, F., Brucy, N., Dubois, Y., Hennebelle, P., and Rosdahl, J. (2023). Universal gravity-driven isothermal turbulence cascade in disk galaxies. A&A 672, A193. doi:10.1051/0004-6361/202245491
Fleck, J. R. C. (1981). On the generation and maintenance of turbulence in the interstellar medium. Astrophysical J. 246, L151–L154. doi:10.1086/183573
Fujii, M. S., Saitoh, T. R., Wang, L., and Hirai, Y. (2021). SIRIUS project. II. A new tree-direct hybrid code for smoothed particle hydrodynamics/N-body simulations of star clusters. PASJ 73, 1057–1073. doi:10.1093/pasj/psab037
Fujimoto, Y., Tasker, E. J., and Habe, A. (2014). Environmental dependence of star formation induced by cloud collisions in a barred galaxy. MNRAS 445, L65–L69. doi:10.1093/MNRASl/slu138
Fukui, Y., Mizuno, N., Yamaguchi, R., Mizuno, A., and Onishi, T. (2001). On the mass spectrum of giant molecular clouds in the large magellanic cloud. PASJ 53, L41–L44. doi:10.1093/pasj/53.6.L41
Fukui, , Harada, R., Tokuda, K., Morioka, Y., Onishi, T., Torii, K., et al. (2015). High-mass star formation triggered by collision between CO filaments in N159 west in the large magellanic cloud. Astrophysical J. 807, L4. doi:10.1088/2041-8205/807/1/L4
Fukushima, H., Yajima, H., Sugimura, K., Hosokawa, T., Omukai, K., and Matsumoto, T. (2020). Star cluster formation and cloud dispersal by radiative feedback: dependence on metallicity and compactness. MNRAS 497, 3830–3845. doi:10.1093/MNRAS/staa2062
Furukawa, N., Dawson, J. R., Ohama, A., Kawamura, A., Mizuno, N., Onishi, T., et al. (2009). Molecular clouds toward RCW49 and westerlund 2: evidence for cluster formation triggered by cloud-cloud collision. Astrophysical J. 696, L115–L119. doi:10.1088/0004-637X/696/2/L115
Fux, R. (1999). 3D self-consistent N-body barred models of the Milky Way. II. Gas dynamics. A&A 345, 787–812. doi:10.48550/arXiv.astro-ph/9903154
Gatto, A., Walch, S., Low, M. M. M., Naab, T., Girichidis, P., Glover, S. C. O., et al. (2015). Modelling the supernova-driven ISM in different environments. MNRAS 449, 1057–1075. doi:10.1093/MNRAS/stv324
Gatto, A., Walch, S., Naab, T., Girichidis, P., Wünsch, R., Glover, S. C. O., et al. (2017). The SILCC project - III. Regulation of star formation and outflows by stellar winds and supernovae. MNRAS 466, 1903–1924. doi:10.1093/MNRAS/stw3209
Gavagnin, E., Bleuler, A., Rosdahl, J., and Teyssier, R. (2017). Star cluster formation in a turbulent molecular cloud self-regulated by photoionization feedback. MNRAS 472, 4155–4172. doi:10.1093/MNRAS/stx2222
Gazol, A., and Villagran, M. A. (2021). The physical and the geometrical properties of simulated cold H I structures. MNRAS 501, 3099–3112. doi:10.1093/MNRAS/staa3852
Gazol-Patiño, A., and Passot, T. (1999). A turbulent model for the interstellar medium. III. Stratification and supernova explosions. Astrophysical J. 518, 748–759. doi:10.1086/307306
Geen, S., Hennebelle, P., Tremblin, P., and Rosdahl, J. (2016). Feedback in Clouds II: UV photoionization and the first supernova in a massive cloud. MNRAS 463, 3129–3142. doi:10.1093/MNRAS/stw2235
Geen, S., Watson, S. K., Rosdahl, J., Bieri, R., Klessen, R. S., and Hennebelle, P. (2018). On the indeterministic nature of star formation on the cloud scale. MNRAS 481, 2548–2569. doi:10.1093/MNRAS/sty2439
Gent, F. A., Shukurov, A., Fletcher, A., Sarson, G. R., and Mantere, M. J. (2013). The supernova-regulated ISM - I. The multiphase structure. MNRAS 432, 1396–1423. doi:10.1093/MNRAS/stt560
Girichidis, P. (2021). Alignment of the magnetic field in star-forming regions and why it might be difficult to observe. MNRAS 507, 5641–5657. doi:10.1093/MNRAS/stab2157
Girichidis, P., Naab, T., Hanasz, M., and Walch, S. (2018). Cooler and smoother - the impact of cosmic rays on the phase structure of galactic outflows. MNRAS 479, 3042–3067. doi:10.1093/MNRAS/sty1653
Glover, S. C. O. (2015). Simulating the formation of massive seed black holes in the early Universe - I. An improved chemical model. MNRAS 451, 2082–2096. doi:10.1093/MNRAS/stv1059
Glover, S. C. O., and Mac Low, M.-M. (2007). Simulating the formation of molecular clouds. II. Rapid formation from turbulent initial conditions. Astrophysical J. 659, 1317–1337. doi:10.1086/512227
Glover, S. C. O., and Smith, R. J. (2016). CO-dark gas and molecular filaments in Milky Way-type galaxies - II. The temperature distribution of the gas. MNRAS 462, 3011–3025. doi:10.1093/MNRAS/stw1879
Gnedin, N. Y., Tassis, K., and Kravtsov, A. V. (2009). Modeling molecular hydrogen and star formation in cosmological simulations. Astrophysical J. 697, 55–67. doi:10.1088/0004-637X/697/1/55
Gong, M., Ostriker, E. C., and Kim, C.-G. (2018). The X CO conversion factor from galactic multiphase ISM simulations. Astrophysical J. 858, 16. doi:10.3847/1538-4357/aab9af
González-Samaniego, A., and Vazquez-Semadeni, E. (2020). The effect of photoionizing feedback on the shaping of hierarchically-forming stellar clusters. MNRAS 499, 668–680. doi:10.1093/MNRAS/staa2921
Grieco, F., Theulé, P., De Looze, I., and Dulieu, F. (2023). Enhanced star formation through the high-temperature formation of H2 on carbonaceous dust grains. Nat. Astron. 7, 541–545. doi:10.1038/s41550-023-01902-4
Grisdale, K., Agertz, O., Renaud, F., and Romeo, A. B. (2018). Physical properties and scaling relations of molecular clouds: the effect of stellar feedback. MNRAS 479, 3167–3180. doi:10.1093/MNRAS/sty1595
Grisdale, K., Agertz, O., Romeo, A. B., Renaud, F., and Read, J. I. (2017). The impact of stellar feedback on the density and velocity structure of the interstellar medium. MNRAS 466, 1093–1110. doi:10.1093/MNRAS/stw3133
Großschedl, J. E., Alves, J., Meingast, S., and Herbst-Kiss, G. (2021). 3D dynamics of the Orion cloud complex. Discovery of coherent radial gas motions at the 100-pc scale. A&A 647, A91. doi:10.1051/0004-6361/202038913
Grudić, M. Y., Guszejnov, D., Offner, S. S. R., Rosen, A. L., Raju, A. N., Faucher-Giguère, C.-A., et al. (2022). The dynamics and outcome of star formation with jets, radiation, winds, and supernovae in concert. MNRAS 512, 216–232. doi:10.1093/MNRAS/stac526
Grudić, M. Y., Hafen, Z., Rodriguez, C. L., Guszejnov, D., Lamberts, A., Wetzel, A., et al. (2023). Great balls of FIRE - I. The formation of star clusters across cosmic time in a Milky Way-mass galaxy. MNRAS 519, 1366–1380. doi:10.1093/MNRAS/stac3573
Guszejnov, D., Grudić, M. Y., Offner, S. S. R., Faucher-Giguère, C.-A., Hopkins, P. F., and Rosen, A. L. (2022). Effects of the environment and feedback physics on the initial mass function of stars in the STARFORGE simulations. MNRAS 515, 4929–4952. doi:10.1093/MNRAS/stac2060
Gutcke, T. A., Pfrommer, C., Bryan, G. L., Pakmor, R., Springel, V., and Naab, T. (2022). LYRA. III. The smallest reionization survivors. Astrophysical J. 941, 120. doi:10.3847/1538-4357/aca1b4
Habing, H. J. (1968). The interstellar radiation density between 912 A and 2400 A. Bull. Astron. Inst. Neth. 19, 421.
Haid, S., Walch, S., Seifried, D., Wünsch, R., Dinnbier, F., and Naab, T. (2019). SILCC-Zoom: the early impact of ionizing radiation on forming molecular clouds. MNRAS 482, 4062–4083. doi:10.1093/MNRAS/sty2938
Harfst, S., Theis, C., and Hensler, G. (2006). Modelling galaxies with a 3d multi-phase ISM. A&A 449, 509–518. doi:10.1051/0004-6361:20042190
Hartwig, T., Glover, S. C. O., Klessen, R. S., Latif, M. A., and Volonteri, M. (2015). How an improved implementation of H2 self-shielding influences the formation of massive stars and black holes. MNRAS 452, 1233–1244. doi:10.1093/MNRAS/stv1368
Hatchfield, H. P., Sormani, M. C., Tress, R. G., Battersby, C., Smith, R. J., Glover, S. C. O., et al. (2021). Dynamically driven inflow onto the galactic center and its effect upon molecular clouds. Astrophysical J. 922, 79. doi:10.3847/1538-4357/ac1e89
Heiner, J. S., Vázquez-Semadeni, E., and Ballesteros-Paredes, J. (2015). Molecular cloud formation as seen in synthetic H I and molecular gas observations. MNRAS 452, 1353–1374. doi:10.1093/MNRAS/stv1153
Heitsch, F., Slyz, A. D., Devriendt, J. E. G., Hartmann, L. W., and Burkert, A. (2006). The birth of molecular clouds: formation of atomic precursors in colliding flows. Astrophysical J. 648, 1052–1065. doi:10.1086/505931
Heitsch, F., Stone, J. M., and Hartmann, L. W. (2009). Effects of magnetic field strength and orientation on molecular cloud formation. Astrophysical J. 695, 248–258. doi:10.1088/0004-637X/695/1/248
Hennebelle, P. (2013). On the origin of non-self-gravitating filaments in the ISM. A&A 556, A153. doi:10.1051/0004-6361/201321292
Hennebelle, P., and Iffrig, O. (2014). Simulations of magnetized multiphase galactic disc regulated by supernovae explosions. A&A 570, A81. doi:10.1051/0004-6361/201423392
Hennebelle, P., and Inutsuka, S.-i. (2019). The role of magnetic field in molecular cloud formation and evolution. Front. Astronomy Space Sci. 6, 5. doi:10.3389/fspas.2019.00005
Hennebelle, P., and Pérault, M. (1999). Dynamical condensation in a thermally bistable flow. Application to interstellar cirrus. A&A 351, 309–322.
Herrington, N. P., Dobbs, C. L., and Bending, T. J. R. (2023). The role of previous generations of stars in triggering star formation and driving gas dynamics. MNRAS 521, 5712–5723. doi:10.1093/MNRAS/stad923
Heyer, M. H., and Brunt, C. M. (2012). Trans-Alfvénic motions in the Taurus molecular cloud. MNRAS 420, 1562–1569. doi:10.1111/j.1365-2966.2011.20142.x
Hill, A. S., Joung, M. R., Mac Low, M.-M., Benjamin, R. A., Haffner, L. M., Klingenberg, C., et al. (2012). Vertical structure of a supernova-driven turbulent, magnetized interstellar medium. Astrophysical J. 750, 104. doi:10.1088/0004-637X/750/2/104
Hincelin, U., Chang, Q., and Herbst, E. (2015). A new and simple approach to determine the abundance of hydrogen molecules on interstellar ice mantles. A&A 574, A24. doi:10.1051/0004-6361/201424807
Hirai, Y., Fujii, M. S., and Saitoh, T. R. (2021). SIRIUS project. I. Star formation models for star-by-star simulations of star clusters and galaxy formation. PASJ 73, 1036–1056. doi:10.1093/pasj/psab038
Hohl, F. (1971). Numerical experiments with a disk of stars. Astrophysical J. 168, 343. doi:10.1086/151091
Hollenbach, D. J., Werner, M. W., and Salpeter, E. E. (1971). Molecular hydrogen in H i regions. Astrophysical J. 163, 165. doi:10.1086/150755
Hopkins, P. F., Quataert, E., and Murray, N. (2011). Self-regulated star formation in galaxies via momentum input from massive stars. MNRAS 417, 950–973. doi:10.1111/j.1365-2966.2011.19306.x
Hu, C.-Y., Naab, T., Walch, S., Glover, S. C. O., and Clark, P. C. (2016). Star formation and molecular hydrogen in dwarf galaxies: a non-equilibrium view. MNRAS 458, 3528–3553. doi:10.1093/MNRAS/stw544
Hu, Z., Wibking, B. D., and Krumholz, M. R. (2023). The sub-critical illusion: synthetic Zeeman effect observations from galactic zoom-in simulations. MNRAS 521, 5604–5615. doi:10.1093/MNRAS/stad931
Huang, X., Jiang, Y.-f., and Davis, S. W. (2022). Cosmic-Ray-driven multiphase gas formed via thermal instability. Astrophysical J. 931, 140. doi:10.3847/1538-4357/ac69dc
Hunter, G. H., Clark, P. C., Glover, S. C. O., and Klessen, R. S. (2023). Towards the impact of GMC collisions on the star formation rate. MNRAS 519, 4152–4170. doi:10.1093/MNRAS/stac3751
Iffrig, O., and Hennebelle, P. (2015). Mutual influence of supernovae and molecular clouds. A&A 576, A95. doi:10.1051/0004-6361/201424556
Iffrig, O., and Hennebelle, P. (2017). Structure distribution and turbulence in self-consistently supernova-driven ISM of multiphase magnetized galactic discs. A&A 604, A70. doi:10.1051/0004-6361/201630290
Iles, E. J., Pettitt, A. R., and Okamoto, T. (2022). Differences in star formation activity between tidally triggered and isolated bars: a case study of NGC 4303 and NGC 3627. MNRAS 510, 3899–3916. doi:10.1093/MNRAS/stab3330
Imara, N., Bigiel, F., and Blitz, L. (2011). Angular momentum in giant molecular clouds. II. M33. Astrophysical J. 732, 79. doi:10.1088/0004-637X/732/2/79
Imara, N., and Blitz, L. (2011). Angular momentum in giant molecular clouds. I. The Milky way. Astrophysical J. 732, 78. doi:10.1088/0004-637X/732/2/78
Inoue, T., and Inutsuka, S.-i. (2009). Two-fluid magnetohydrodynamics simulations of converging H I flows in the interstellar medium. II. Are molecular clouds generated directly from a warm neutral medium? Astrophysical J. 704, 161–169. doi:10.1088/0004-637X/704/1/161
Inoue, T., Yamazaki, R., and Inutsuka, S.-i. (2009). Turbulence and magnetic field amplification in supernova remnants: interactions between a strong shock wave and multiphase interstellar medium. Astrophysical J. 695, 825–833. doi:10.1088/0004-637X/695/2/825
Inutsuka, S.-i., Inoue, T., Iwasaki, K., and Hosokawa, T. (2015). The formation and destruction of molecular clouds and galactic star formation. An origin for the cloud mass function and star formation efficiency. A&A 580, A49. doi:10.1051/0004-6361/201425584
Iqbal, W., Acharyya, K., and Herbst, E. (2012). Kinetic Monte Carlo studies of H2 formation on grain surfaces over a wide temperature range. Astrophysical J. 751, 58. doi:10.1088/0004-637X/751/1/58
Jeffreson, S. M. R., Kruijssen, J. M. D., Keller, B. W., Chevance, M., and Glover, S. C. O. (2020). The role of galactic dynamics in shaping the physical properties of giant molecular clouds in Milky Way-like galaxies. MNRAS 498, 385–429. doi:10.1093/MNRAS/staa2127
Jeffreson, S. M. R., Krumholz, M. R., Fujimoto, Y., Armillotta, L., Keller, B. W., Chevance, M., et al. (2021). Momentum feedback from marginally resolved H II regions in isolated disc galaxies. MNRAS 505, 3470–3491. doi:10.1093/MNRAS/stab1536
Joshi, P. R., Walch, S., Seifried, D., Glover, S. C. O., Clarke, S. D., and Weis, M. (2019). On the resolution requirements for modelling molecular gas formation in solar neighbourhood conditions. MNRAS 484, 1735–1755. doi:10.1093/MNRAS/stz052
Joung, M. K. R., and Mac Low, M.-M. (2006). Turbulent structure of a stratified supernova-driven interstellar medium. Astrophysical J. 653, 1266–1279. doi:10.1086/508795
Kennicutt, R. C., and Evans, N. J. (2012). Star Formation in the Milky way and nearby galaxies. ARA&A 50, 531–608. doi:10.1146/annurev-astro-081811-125610
Khoperskov, S. A., Vasiliev, E. O., Ladeyschikov, D. A., Sobolev, A. M., and Khoperskov, A. V. (2016). Giant molecular cloud scaling relations: the role of the cloud definition. MNRAS 455, 1782–1795. doi:10.1093/MNRAS/stv2366
Khoperskov, S. A., Vasiliev, E. O., Sobolev, A. M., and Khoperskov, A. V. (2013). The simulation of molecular clouds formation in the Milky Way. MNRAS 428, 2311–2320. doi:10.1093/MNRAS/sts195
Kim, C.-G., Kim, W.-T., and Ostriker, E. C. (2006). Interstellar turbulence driving by galactic spiral shocks. Astrophysical J. 649, L13–L16. doi:10.1086/508160
Kim, C.-G., Kim, W.-T., and Ostriker, E. C. (2008). Galactic spiral shocks with thermal instability. Astrophysical J. 681, 1148–1162. doi:10.1086/588752
Kim, C.-G., Kim, W.-T., and Ostriker, E. C. (2010). Galactic spiral shocks with thermal instability in vertically stratified galactic disks. Astrophysical J. 720, 1454–1471. doi:10.1088/0004-637X/720/2/1454
Kim, C.-G., and Ostriker, E. C. (2017). Three-phase interstellar medium in galaxies resolving evolution with star formation and supernova feedback (TIGRESS): algorithms, fiducial model, and convergence. Astrophysical J. 846, 133. doi:10.3847/1538-4357/aa8599
Kim, C.-G., and Ostriker, E. C. (2018). Numerical simulations of multiphase winds and fountains from star-forming galactic disks. I. Solar neighborhood TIGRESS model. Astrophysical J. 853, 173. doi:10.3847/1538-4357/aaa5ff
Kim, J., Hong, S. S., Ryu, D., and Jones, T. W. (1998). Three-dimensional evolution of the parker instability under a uniform gravity. Astrophysical J. 506, L139–L142. doi:10.1086/311649
Kim, J.-G., Gong, M., Kim, C.-G., and Ostriker, E. C. (2023). Photochemistry and heating/cooling of the multiphase interstellar medium with UV radiative transfer for magnetohydrodynamic simulations. Astrophysical JournalS 264, 10. doi:10.3847/1538-4365/ac9b1d
Kim, J.-G., Ostriker, E. C., and Filippova, N. (2021). Star Formation efficiency and dispersal of giant molecular clouds with UV radiation feedback: dependence on gravitational boundedness and magnetic fields. Astrophysical J. 911, 128. doi:10.3847/1538-4357/abe934
Kim, W.-T., Kim, C.-G., and Ostriker, E. C. (2020). Local simulations of spiral galaxies with the TIGRESS framework. I. Star formation and arm spurs/feathers. Astrophysical J. 898, 35. doi:10.3847/1538-4357/ab9b87
Kim, W.-T., Kim, Y., and Kim, J.-G. (2014). Nature of the wiggle instability of galactic spiral shocks. Astrophysical J. 789, 68. doi:10.1088/0004-637X/789/1/68
Kim, W.-T., and Ostriker, E. C. (2000). Magnetohydrodynamic instabilities in shearing, rotating, stratified winds and disks. Astrophysical J. 540, 372–403. doi:10.1086/309293
Kim, W.-T., and Ostriker, E. C. (2002). Formation and fragmentation of gaseous spurs in spiral galaxies. Astrophysical J. 570, 132–151. doi:10.1086/339352
Kim, W.-T., and Ostriker, E. C. (2006). Formation of spiral-arm spurs and bound clouds in vertically stratified galactic gas disks. Astrophysical J. 646, 213–231. doi:10.1086/504677
Kim, W.-T., Ostriker, E. C., and Stone, J. M. (2003). Magnetorotationally driven galactic turbulence and the formation of giant molecular clouds. Astrophysical J. 599, 1157–1172. doi:10.1086/379367
Klessen, R. S., and Hennebelle, P. (2010). Accretion-driven turbulence as universal process: galaxies, molecular clouds, and protostellar disks. A&A 520, A17. doi:10.1051/0004-6361/200913780
Korpi, M. J., Brandenburg, A., Shukurov, A., Tuominen, I., and Nordlund, Å. (1999). A supernova-regulated interstellar medium: simulations of the turbulent multiphase medium. Astrophysical J. 514, L99–L102. doi:10.1086/311954
Körtgen, B., and Banerjee, R. (2015). Impact of magnetic fields on molecular cloud formation and evolution. MNRAS 451, 3340–3353. doi:10.1093/MNRAS/stv1200
Körtgen, B., Banerjee, R., Pudritz, R. E., and Schmidt, W. (2018). The origin of filamentary star forming clouds in magnetised galaxies. MNRAS 479, L40–L44. doi:10.1093/MNRASl/sly094
Körtgen, B., Banerjee, R., Pudritz, R. E., and Schmidt, W. (2019). Global dynamics of the interstellar medium in magnetized disc galaxies. MNRAS 489, 5004–5021. doi:10.1093/MNRAS/stz2491
Kosiński, R., and Hanasz, M. (2006). On the influence of cooling and heating processes on Parker instability. MNRAS 368, 759–768. doi:10.1111/j.1365-2966.2006.10142.x
Koyama, H., and Inutsuka, S.-i. (2002). An origin of supersonic motions in interstellar clouds. Astrophysical J. 564, L97–L100. –L100. doi:10.1086/338978
Kruijssen, J. M. D., Dale, J. E., Longmore, S. N., Walker, D. L., Henshaw, J. D., Jeffreson, S. M. R., et al. (2019). The dynamical evolution of molecular clouds near the Galactic Centre - II. Spatial structure and kinematics of simulated clouds. MNRAS 484, 5734–5754. doi:10.1093/MNRAS/stz381
Krumholz, M. R. (2011). “Star Formation in molecular clouds,” in XV special courses at the national observatory of rio de Janeiro. Editors E. Telles, R. Dupke, and D. Lazzaro (Denmark: American Institute of Physics Conference Series). 9–57. doi:10.1063/1.3636038
Krumholz, M. R., and Gnedin, N. Y. (2011). A comparison of methods for determining the molecular content of model galaxies. Astrophysical J. 729, 36. doi:10.1088/0004-637X/729/1/36
Kuhlen, M., Krumholz, M. R., Madau, P., Smith, B. D., and Wise, J. (2012). Dwarf galaxy formation with H2-regulated star formation. Astrophysical J. 749, 36. doi:10.1088/0004-637X/749/1/36
Kwan, J. (1979). The mass spectrum of interstellar clouds. Astrophysical J. 229, 567–577. doi:10.1086/156990
Lagos, C. D. P., Baugh, C. M., Lacey, C. G., Benson, A. J., Kim, H.-S., and Power, C. (2011). Cosmic evolution of the atomic and molecular gas contents of galaxies. MNRAS 418, 1649–1667. doi:10.1111/j.1365-2966.2011.19583.x
Lahén, N., Naab, T., Kauffmann, G., Szécsi, D., Hislop, J. M., Rantala, A., et al. (2023). Formation of star clusters and enrichment by massive stars in simulations of low-metallicity galaxies with a fully sampled initial stellar mass function. MNRAS 522, 3092–3116. doi:10.1093/MNRAS/stad1147
Larson, R. B. (1981). Turbulence and star formation in molecular clouds. MNRAS 194, 809–826. doi:10.1093/MNRAS/194.4.809
Leão, M. R. M., de Gouveia Dal Pino, E. M., Falceta-Gonçalves, D., Melioli, C., and Geraissate, F. G. (2009). Local star formation triggered by supernova shocks in magnetized diffuse neutral clouds. MNRAS 394, 157–173. doi:10.1111/j.1365-2966.2008.14337.x
Le Bourlot, J., Le Petit, F., Pinto, C., Roueff, E., and Roy, F. (2012). Surface chemistry in the interstellar medium. I. H2 formation by Langmuir-Hinshelwood and Eley-Rideal mechanisms. A&A 541, A76. doi:10.1051/0004-6361/201118126
Lee, W.-K. (2014). Feathering instability of spiral arms. II. Parameter study. Astrophysical J. 792, 122. doi:10.1088/0004-637X/792/2/122
Leroy, A. K., Schinnerer, E., Hughes, A., Rosolowsky, E., Pety, J., Schruba, A., et al. (2021). PHANGS-ALMA: arcsecond CO(2-1) imaging of nearby star-forming galaxies. Astrophysical JournalS 257, 43. doi:10.3847/1538-4365/ac17f3
Li, H., Vogelsberger, M., Bryan, G. L., Marinacci, F., Sales, L. V., and Torrey, P. (2022). Formation and evolution of young massive clusters in galaxy mergers: the SMUGGLE view. MNRAS 514, 265–279. doi:10.1093/MNRAS/stac1136
Li, Z., Gerhard, O., Shen, J., Portail, M., and Wegg, C. (2016). Gas dynamics in the Milky way: a low pattern speed model. Astrophysical J. 824, 13. doi:10.3847/0004-637X/824/1/13
Liow, K. Y., and Dobbs, C. L. (2020). The role of collision speed, cloud density, and turbulence in the formation of young massive clusters via cloud-cloud collisions. MNRAS 499, 1099–1115. doi:10.1093/MNRAS/staa2857
Liow, K. Y., Rieder, S., Dobbs, C. L., and Jaffa, S. E. (2022). Grouped star formation: converting sink particles to stars in hydrodynamical simulations. MNRAS 510, 2657–2670. doi:10.1093/MNRAS/stab3617
Longmore, S. N., Kruijssen, J. M. D., Bally, J., Ott, J., Testi, L., Rathborne, J., et al. (2013). Candidate super star cluster progenitor gas clouds possibly triggered by close passage to Sgr a. MNRAS 433, L15–L19. doi:10.1093/MNRASl/slt048
Lu, Z.-J., Pelkonen, V.-M., Padoan, P., Pan, L., Haugbølle, T., and Nordlund, Å. (2020). The effect of supernovae on the turbulence and dispersal of molecular clouds. Astrophysical J. 904, 58. doi:10.3847/1538-4357/abbd8f
Lucas, W. E., Bonnell, I. A., and Dale, J. E. (2020). Supernova feedback and the energy deposition in molecular clouds. MNRAS 493, 4700–4710. doi:10.1093/MNRAS/staa451
Mac Low, M.-M., Klessen, R. S., Burkert, A., and Smith, M. D. (1998). Kinetic energy decay rates of supersonic and super-alfvénic turbulence in star-forming clouds. Phys. Rev. Lett. 80, 2754–2757. doi:10.1103/PhysRevLett.80.2754
Marinacci, F., Sales, L. V., Vogelsberger, M., Torrey, P., and Springel, V. (2019). Simulating the interstellar medium and stellar feedback on a moving mesh: implementation and isolated galaxies. MNRAS 489, 4233–4260. doi:10.1093/MNRAS/stz2391
Martin, C. E., Heyvaerts, J., and Priest, E. R. (1997). Alfven wave support of a dwarf molecular cloud. I. an isothermal model. A&A 326, 1176–1186.
McClure-Griffiths, N. M., Dickey, J. M., Gaensler, B. M., Green, A. J., and Haverkorn, M. (2006). Magnetically dominated strands of cold hydrogen in the riegel-crutcher cloud. Astrophysical J. 652, 1339–1347. doi:10.1086/508706
McClure-Griffiths, N. M., Stanimirovic, S., and Rybarczyk, D. R. (2023). Atomic hydrogen in the Milky way: a stepping stone in the evolution of galaxies. arXiv e-prints, arXiv:2307.08464.
McCray, R., and Kafatos, M. (1987). Supershells and propagating star formation. Astrophysical J. 317, 190. doi:10.1086/165267
Meidt, S. E., Leroy, A. K., Rosolowsky, E., Kruijssen, J. M. D., Schinnerer, E., Schruba, A., et al. (2018). A model for the onset of self-gravitation and star formation in molecular gas governed by galactic forces. I. Cloud-Scale gas motions. Astrophysical J. 854, 100. doi:10.3847/1538-4357/aaa290
Miller, R. H., Prendergast, K. H., and Quirk, W. J. (1970). Numerical experiments on spiral structure. Astrophysical J. 161, 903. doi:10.1086/150593
Miret-Roig, N., Galli, P. A. B., Olivares, J., Bouy, H., Alves, J., and Barrado, D. (2022). The star formation history of Upper Scorpius and Ophiuchus. A 7D picture: positions, kinematics, and dynamical traceback ages. A&A 667, A163. doi:10.1051/0004-6361/202244709
Monaco, P., Murante, G., Borgani, S., and Dolag, K. (2012). Schmidt-Kennicutt relations in SPH simulations of disc galaxies with effective thermal feedback from supernovae. MNRAS 421, 2485–2497. doi:10.1111/j.1365-2966.2012.20482.x
Moon, S., Kim, W.-T., Kim, C.-G., and Ostriker, E. C. (2021). Star Formation in nuclear rings with the TIGRESS framework. Astrophysical J. 914, 9. doi:10.3847/1538-4357/abfa93
Nelson, R. P., and Langer, W. D. (1997). The dynamics of low-mass molecular clouds in external radiation fields. Astrophysical J. 482, 796–826. doi:10.1086/304167
Nickerson, S., Teyssier, R., and Rosdahl, J. (2019). Towards the complete census of molecular hydrogen in a simulated disc galaxy. MNRAS 484, 1238–1256. doi:10.1093/MNRAS/stz048
Nixon, C. J., and Pringle, J. E. (2019). On the role of magnetic fields in star formation. New Astron 67, 89–96. doi:10.1016/j.newast.2018.09.007
Oey, M. S., Watson, A. M., Kern, K., and Walth, G. L. (2005). Hierarchical triggering of star formation by superbubbles in W3/W4. AJ 129, 393–401. doi:10.1086/426333
Ostriker, E. C., Gammie, C. F., and Stone, J. M. (1999). Kinetic and structural evolution of self-gravitating, magnetized clouds: 2.5-dimensional simulations of decaying turbulence. Astrophysical J. 513, 259–274. doi:10.1086/306842
Padoan, P., Juvela, M., Pan, L., Haugbølle, T., and Nordlund, Å. (2016). Supernova driving. III. Synthetic molecular cloud observations. Astrophysical J. 826, 140. doi:10.3847/0004-637X/826/2/140
Pan, H.-A., Fujimoto, Y., Tasker, E. J., Rosolowsky, E., Colombo, D., Benincasa, S. M., et al. (2016). Effects of galactic disc inclination and resolution on observed GMC properties and Larson’s scaling relations. MNRAS 458, 2443–2453. doi:10.1093/MNRAS/stw478
Pattle, K., Fissel, L., Tahani, M., Liu, T., and Ntormousi, E. (2022). Magnetic fields in star formation: from clouds to cores. arXiv e-prints, arXiv:2203.
Pelupessy, F. I., Papadopoulos, P. P., and van der Werf, P. (2006). Incorporating the molecular gas phase in galaxy-sized numerical simulations: first applications in dwarf galaxies. Astrophysical J. 645, 1024–1042. doi:10.1086/504366
Peñaloza, C. H., Clark, P. C., Glover, S. C. O., and Klessen, R. S. (2018). CO line ratios in molecular clouds: the impact of environment. MNRAS 475, 1508–1520. doi:10.1093/MNRAS/stx3263
Perets, H. B., Lederhendler, A., Biham, O., Vidali, G., Li, L., Swords, S., et al. (2007). Molecular hydrogen formation on amorphous silicates under interstellar conditions. Astrophysical J. 661, L163–L166. doi:10.1086/518862
Peters, T., Naab, T., Walch, S., Glover, S. C. O., Girichidis, P., Pellegrini, E., et al. (2017). The SILCC project - IV. Impact of dissociating and ionizing radiation on the interstellar medium and Hα emission as a tracer of the star formation rate. MNRAS 466, 3293–3308. doi:10.1093/MNRAS/stw3216
Pettitt, A. R., Dobbs, C. L., Acreman, D. M., and Bate, M. R. (2015). The morphology of the Milky Way - II. Reconstructing CO maps from disc galaxies with live stellar distributions. MNRAS 449, 3911–3926. doi:10.1093/MNRAS/stv600
Pettitt, A. R., Dobbs, C. L., Acreman, D. M., and Price, D. J. (2014). The morphology of the Milky Way - I. Reconstructing CO maps from simulations in fixed potentials. MNRAS 444, 919–941. doi:10.1093/MNRAS/stu1075
Pettitt, A. R., Dobbs, C. L., Baba, J., Colombo, D., Duarte-Cabral, A., Egusa, F., et al. (2020). How do different spiral arm models impact the ISM and GMC population? MNRAS 498, 1159–1174. doi:10.1093/MNRAS/staa2242
Pettitt, A. R., Tasker, E. J., Wadsley, J. W., Keller, B. W., and Benincasa, S. M. (2017). Star formation and ISM morphology in tidally induced spiral structures. MNRAS 468, 4189–4204. doi:10.1093/MNRAS/stx736
Pfeffer, J., Kruijssen, J. M. D., Crain, R. A., and Bastian, N. (2018). The E-MOSAICS project: simulating the formation and co-evolution of galaxies and their star cluster populations. MNRAS 475, 4309–4346. doi:10.1093/MNRAS/stx3124
Phillips, J. P. (1999). Rotation in molecular clouds. Astrophysics Space Sci. 134, 241–254. doi:10.1051/aas:1999137
Pinto, C., Verdini, A., Galli, D., and Velli, M. (2012). Reflection and dissipation of Alfvén waves in interstellar clouds. A&A 544, A66. doi:10.1051/0004-6361/201219019
Piontek, R. A., and Ostriker, E. C. (2007). Models of vertically stratified two-phase ISM disks with MRI-driven turbulence. Astrophysical J. 663, 183–203. doi:10.1086/518103
Planck Collaboration Adam, R., Ade, P. A. R., Aghanim, N., Alves, M. I. R., Arnaud, M., et al. (2016). Planck intermediate results. XXXII. The relative orientation between the magnetic field and structures traced by interstellar dust. A&A 586, A135. doi:10.1051/0004-6361/201425044
Rathjen, T.-E., Naab, T., Girichidis, P., Walch, S., Wünsch, R., Dinnbier, F., et al. (2021). SILCC VI - multiphase ISM structure, stellar clustering, and outflows with supernovae, stellar winds, ionizing radiation, and cosmic rays. MNRAS 504, 1039–1061. doi:10.1093/MNRAS/stab900
Reina-Campos, M., Keller, B. W., Kruijssen, J. M. D., Gensior, J., Trujillo-Gomez, S., Jeffreson, S. M. R., et al. (2022). Introducing EMP-Pathfinder: modelling the simultaneous formation and evolution of stellar clusters in their host galaxies. MNRAS 517, 3144–3180. doi:10.1093/MNRAS/stac1934
Renaud, F., Bournaud, F., Emsellem, E., Agertz, O., Athanassoula, E., Combes, F., et al. (2015). Environmental regulation of cloud and star formation in galactic bars. MNRAS 454, 3299–3310. doi:10.1093/MNRAS/stv2223
Renaud, F., Bournaud, F., Emsellem, E., Elmegreen, B., Teyssier, R., Alves, J., et al. (2013). A sub-parsec resolution simulation of the Milky Way: global structure of the interstellar medium and properties of molecular clouds. MNRAS 436, 1836–1851. doi:10.1093/MNRAS/stt1698
Rice, T. S., Goodman, A. A., Bergin, E. A., Beaumont, C., and Dame, T. M. (2016). A uniform catalog of molecular clouds in the Milky way. Astrophysical J. 822, 52. doi:10.3847/0004-637X/822/1/52
Richings, A. J., Schaye, J., and Oppenheimer, B. D. (2014). Non-equilibrium chemistry and cooling in the diffuse interstellar medium - II. Shielded gas. MNRAS 442, 2780–2796. doi:10.1093/MNRAS/stu1046
Rieder, S., Dobbs, C., Bending, T., Liow, K. Y., and Wurster, J. (2022). The formation and early evolution of embedded star clusters in spiral galaxies. MNRAS 509, 6155–6168. doi:10.1093/MNRAS/stab3425
Roberts, J., William, W., and Hausman, M. A. (1984). Spiral structure and star formation. I - formation mechanisms and mean free paths. Astrophysical J. 277, 744–767. doi:10.1086/161746
Roberts, J., William, W., and Stewart, G. R. (1987). The role of orbital dynamics and cloud-cloud collisions in the formation of giant molecular clouds in global spiral structures. Astrophysical J. 314, 10. doi:10.1086/165035
Roberts, W. W. (1969). Large-scale shock formation in spiral galaxies and its implications on star formation. Astrophysical J. 158, 123. doi:10.1086/150177
Robertson, B. E., and Kravtsov, A. V. (2008). Molecular hydrogen and global star formation relations in galaxies. Astrophysical J. 680, 1083–1111. doi:10.1086/587796
Rodriguez-Fernandez, N. J., and Combes, F. (2008). Gas flow models in the Milky Way embedded bars. A&A 489, 115–133. doi:10.1051/0004-6361:200809644
Rogers, H., and Pittard, J. M. (2013). Feedback from winds and supernovae in massive stellar clusters - I. Hydrodynamics. MNRAS 431, 1337–1351. doi:10.1093/MNRAS/stt255
Roman-Duval, J., Jackson, J. M., Heyer, M., Rathborne, J., and Simon, R. (2010). Physical properties and galactic distribution of molecular clouds identified in the galactic ring survey. Astrophysical J. 723, 492–507. doi:10.1088/0004-637X/723/1/492
Rosdahl, J., Schaye, J., Teyssier, R., and Agertz, O. (2015). Galaxies that shine: radiation-hydrodynamical simulations of disc galaxies. MNRAS 451, 34–58. doi:10.1093/MNRAS/stv937
Rosen, A., and Bregman, J. N. (1995). Global models of the interstellar medium in disk galaxies. Astrophysical J. 440, 634. doi:10.1086/175303
Rosolowsky, E. (2007). Giant molecular clouds in M31. I. Molecular cloud properties. Astrophysical J. 654, 240–251. doi:10.1086/509249
Rosolowsky, E., Engargiola, G., Plambeck, R., and Blitz, L. (2003). Giant molecular clouds in M33. II. High-resolution observations. Astrophysical J. 599, 258–274. doi:10.1086/379166
Ruffle, D. P., and Herbst, E. (2000). New models of interstellar gas-grain chemistry - I. Surface diffusion rates. MNRAS 319, 837–850. doi:10.1046/j.1365-8711.2000.03911.x
Salem, M., and Bryan, G. L. (2014). Cosmic ray driven outflows in global galaxy disc models. MNRAS 437, 3312–3330. doi:10.1093/MNRAS/stt2121
Sales, L. V., Marinacci, F., Springel, V., and Petkova, M. (2014). Stellar feedback by radiation pressure and photoionization. MNRAS 439, 2990–3006. doi:10.1093/MNRAS/stu155
Salo, H., and Laurikainen, E. (2000a). N-body model for M51 - I. Multiple encounter versus single passage? MNRAS 319, 377–392. doi:10.1046/j.1365-8711.2000.03650.x
Salo, H., and Laurikainen, E. (2000b). N-body model for M51 - II. Inner structure. MNRAS 319, 393–413. doi:10.1046/j.1365-8711.2000.03651.x
Sanders, D. B., Solomon, P. M., and Scoville, N. Z. (1984). Giant molecular clouds in the Galaxy. I - the axisymmetric distribution of H2. Astrophysical J. 276, 182–203. doi:10.1086/161602
Sano, H., Yamane, Y., Tokuda, K., Fujii, K., Tsuge, K., Nagaya, T., et al. (2018). Molecular clouds associated with the type ia SNR N103B in the large magellanic cloud. Astrophysical J. 867, 7. doi:10.3847/1538-4357/aae07c
Santillán, A., Sánchez-Salcedo, F. J., and Franco, J. (2007). Exploring cloudy gas accretion as a source of interstellar turbulence in the outskirts of disks. Astrophysical J. 662, L19–L22. doi:10.1086/519247
Sartorio, N. S., Vandenbroucke, B., Falceta-Goncalves, D., and Wood, K. (2021). Photoionization feedback in turbulent molecular clouds. MNRAS 500, 1833–1843. doi:10.1093/MNRAS/staa3380
Schaye, J. (2004). Star Formation thresholds and galaxy edges: why and where. Astrophysical J. 609, 667–682. doi:10.1086/421232
Schmidt, W., Collins, D. C., and Kritsuk, A. G. (2013). Local support against gravity in magnetoturbulent fluids. MNRAS 431, 3196–3215. doi:10.1093/MNRAS/stt399
Schneider, N., Bonne, L., Bontemps, S., Kabanovic, S., Simon, R., Ossenkopf-Okada, V., et al. (2023). Ionized carbon as a tracer of the assembly of interstellar clouds. Nat. Astron. 7, 546–556. doi:10.1038/s41550-023-01901-5
Seifried, D., Haid, S., Walch, S., Borchert, E. M. A., and Bisbas, T. G. (2020). SILCC-Zoom: H2 and CO-dark gas in molecular clouds - the impact of feedback and magnetic fields. MNRAS 492, 1465–1483. doi:10.1093/MNRAS/stz3563
Seifried, D., Walch, S., Girichidis, P., Naab, T., Wünsch, R., Klessen, R. S., et al. (2017). SILCC-Zoom: the dynamic and chemical evolution of molecular clouds. MNRAS 472, 4797–4818. doi:10.1093/MNRAS/stx2343
Seifried, D., Walch, S., Haid, S., Girichidis, P., and Naab, T. (2018). Is molecular cloud turbulence driven by external supernova explosions? Astrophysical J. 855, 81. doi:10.3847/1538-4357/aaacff
Sellwood, J. A., and Carlberg, R. G. (1984). Spiral instabilities provoked by accretion and star formation. Astrophysical J. 282, 61–74. doi:10.1086/162176
Semczuk, M., Łokas, E. L., Salomon, J.-B., Athanassoula, E., and D’Onghia, E. (2018). Tidally induced morphology of M33 in hydrodynamical simulations of its recent interaction with M31. Astrophysical J. 864, 34. doi:10.3847/1538-4357/aad4ae
Shetty, R., Collins, D. C., Kauffmann, J., Goodman, A. A., Rosolowsky, E. W., and Norman, M. L. (2010). The effect of projection on derived mass-size and linewidth-size relationships. Astrophysical J. 712, 1049–1056. doi:10.1088/0004-637X/712/2/1049
Shetty, R., Glover, S. C., Dullemond, C. P., Ostriker, E. C., Harris, A. I., and Klessen, R. S. (2011). Modelling CO emission - II. The physical characteristics that determine the X factor in Galactic molecular clouds. MNRAS 415, 3253–3274. doi:10.1111/j.1365-2966.2011.18937.x
Shetty, R., Vogel, S. N., Ostriker, E. C., and Teuben, P. J. (2007). Kinematics of spiral-arm streaming in M51. Astrophysical J. 665, 1138–1158. doi:10.1086/520037
Shin, J., Kim, S. S., Baba, J., Saitoh, T. R., Hwang, J.-S., Chun, K., et al. (2017). Hydrodynamic simulations of the central molecular zone with a realistic galactic potential. Astrophysical J. 841, 74. doi:10.3847/1538-4357/aa7061
Shu, F. H., Adams, F. C., and Lizano, S. (1987). Star formation in molecular clouds: observation and theory. ARA&A 25, 23–81. doi:10.1146/annurev.aa.25.090187.000323
Sillero, E., Tissera, P. B., Lambas, D. G., Bovino, S., Schleicher, D. R., Grassi, T., et al. (2021). Modelling H2 and its effects on star formation using a joint implementation of GADGET-3 and KROME. MNRAS 504, 2325–2345. doi:10.1093/MNRAS/stab1015
Sills, A., Rieder, S., Buckner, A. S. M., Hacar, A., Portegies Zwart, S., and Teixeira, P. S. (2023). Using molecular gas observations to guide initial conditions for star cluster simulations. MNRAS 519, 4142–4151. doi:10.1093/MNRAS/stac3745
Skarbinski, M., Jeffreson, S. M. R., and Goodman, A. A. (2023). Building the molecular cloud population: the role of cloud mergers. MNRAS 519, 1887–1898. doi:10.1093/MNRAS/stac3627
Slyz, A. D., Devriendt, J. E. G., Bryan, G., and Silk, J. (2005). Towards simulating star formation in the interstellar medium. MNRAS 356, 737–752. doi:10.1111/j.1365-2966.2004.08494.x
Smilgys, R., and Bonnell, I. A. (2017). Formation of stellar clusters. MNRAS 472, 4982–4991. doi:10.1093/MNRAS/stx2396
Smith, J. D., Dale, J. E., Jaffa, S. E., and Krause, M. G. H. (2022). Star cluster formation in clouds with externally driven turbulence. MNRAS 516, 4212–4219. doi:10.1093/MNRAS/stac2295
Smith, R. J., Glover, S. C. O., Clark, P. C., Klessen, R. S., and Springel, V. (2014). CO-dark gas and molecular filaments in Milky Way-type galaxies. MNRAS 441, 1628–1645. doi:10.1093/MNRAS/stu616
Smith, R. J., Tress, R., Soler, J. D., Klessen, R. S., Glover, S. C. O., Hennebelle, P., et al. (2023). On the distribution of the cold neutral medium in galaxy discs. MNRAS 524, 873–885. doi:10.1093/MNRAS/stad1537
Smith, R. J., Treß, R. G., Sormani, M. C., Glover, S. C. O., Klessen, R. S., Clark, P. C., et al. (2020). The Cloud Factory I: generating resolved filamentary molecular clouds from galactic-scale forces. MNRAS 492, 1594–1613. doi:10.1093/MNRAS/stz3328
Smoluchowski, R. (1981). Rate of H2 formation on amorphous grains. Ap&SS 75, 353–363. doi:10.1007/BF00648648
Snow, T. P. (1983). On the possibly low H2 formation rate in dense clouds. Astrophysical J. 269, L57. doi:10.1086/184055
Soler, J. D., Hennebelle, P., Martin, P. G., Miville-Deschênes, M. A., Netterfield, C. B., and Fissel, L. M. (2013). An imprint of molecular cloud magnetization in the morphology of the dust polarized emission. Astrophysical J. 774, 128. doi:10.1088/0004-637X/774/2/128
Sormani, M. C., Sobacchi, E., Fragkoudi, F., Ridley, M., Treß, R. G., Glover, S. C. O., et al. (2018). A dynamical mechanism for the origin of nuclear rings. MNRAS 481, 2–19. doi:10.1093/MNRAS/sty2246
Sormani, M. C., Treß, R. G., Glover, S. C. O., Klessen, R. S., Barnes, A. T., Battersby, C. D., et al. (2019). The geometry of the gas surrounding the Central Molecular Zone: on the origin of localized molecular clouds with extreme velocity dispersions. MNRAS 488, 4663–4673. doi:10.1093/MNRAS/stz2054
Spilker, A., Kainulainen, J., and Orkisz, J. (2022). Bird’s eye view of molecular clouds in the Milky Way. II. Cloud kinematics from subparsec to kiloparsec scales. A&A 667, A110. doi:10.1051/0004-6361/202244392
Stinson, G. S., Brook, C., Macciò, A. V., Wadsley, J., Quinn, T. R., and Couchman, H. M. P. (2013). Making Galaxies in a Cosmological Context: the need for early stellar feedback. MNRAS 428, 129–140. doi:10.1093/MNRAS/sts028
Sun, J., Leroy, A. K., Schinnerer, E., Hughes, A., Rosolowsky, E., Querejeta, M., et al. (2020). Molecular gas properties on cloud scales across the local star-forming galaxy population. Astrophysical J. 901, L8. doi:10.3847/2041-8213/abb3be
Sun, J., Leroy, A. K., Schruba, A., Rosolowsky, E., Hughes, A., Kruijssen, J. M. D., et al. (2018). Cloud-scale molecular gas properties in 15 nearby galaxies. Astrophysical J. 860, 172. doi:10.3847/1538-4357/aac326
Tanaka, K., Kamegai, K., Nagai, M., and Oka, T. (2007). High-resolution mappings of the l=1°3 complex in molecular lines: discovery of a proto-superbubble. PASJ 59, 323–333. doi:10.1093/pasj/59.2.323
Tanvir, T. S., and Dale, J. E. (2020). Collision between molecular clouds - I. The effect of the cloud virial ratio in head-on collisions. MNRAS 494, 246–258. doi:10.1093/MNRAS/staa665
Tasker, E. J. (2011). Star Formation in disk galaxies. II. The effect of star formation and photoelectric heating on the formation and evolution of giant molecular clouds. Astrophysical J. 730, 11. –+. doi:10.1088/0004-637X/730/1/11
Tasker, E. J., Bryan, G. L., and Tan, J. C. (2008). Simulating the ISM in global disk galaxies. ArXiv:0802.3944.
Tasker, E. J., and Tan, J. C. (2009). Star Formation in disk galaxies. I. Formation and evolution of giant molecular clouds via gravitational instability and cloud collisions. Astrophysical J. 700, 358–375. doi:10.1088/0004-637X/700/1/358
Tenorio-Tagle, G., and Palous, J. (1987). Giant-scale supernova remnants - the role of differential galactic rotation and the formation of molecular clouds. A&A 186, 287–294.
Tielens, A. G. G. M., and Hagen, W. (1982). Model calculations of the molecular composition of interstellar grain mantles. A&A 114, 245–260.
Tomisaka, K. (1984). Coagulation of interstellar clouds in spiral gravitational potential and formation of giant molecular clouds. PASJ 36, 457–475.
Toomre, A., and Toomre, J. (1972). Galactic bridges and tails. Astrophysical J. 178, 623–666. doi:10.1086/151823
Tress, R. G., Smith, R. J., Sormani, M. C., Glover, S. C. O., Klessen, R. S., Mac Low, M.-M., et al. (2020). Simulations of the star-forming molecular gas in an interacting M51-like galaxy. MNRAS 492, 2973–2995. doi:10.1093/MNRAS/stz3600
Valentini, M., Dolag, K., Borgani, S., Murante, G., Maio, U., Tornatore, L., et al. (2023). Impact of H2-driven star formation and stellar feedback from low-enrichment environments on the formation of spiral galaxies. MNRAS 518, 1128–1147. doi:10.1093/MNRAS/stac2110
Vandenbroucke, B., and Wood, K. (2019). Radiation hydrodynamics simulations of the evolution of the diffuse ionized gas in disc galaxies. MNRAS 488, 1977–1986. doi:10.1093/MNRAS/stz1841
Van Loo, S., Butler, M. J., and Tan, J. C. (2013). Kiloparsec-scale simulations of star formation in disk galaxies. I. The unmagnetized and zero-feedback limit. Astrophysical J. 764, 36. doi:10.1088/0004-637X/764/1/36
Vázquez-Semadeni, E., Banerjee, R., Gómez, G. C., Hennebelle, P., Duffin, D., and Klessen, R. S. (2011). Molecular cloud evolution - IV. Magnetic fields, ambipolar diffusion and the star formation efficiency. MNRAS 414, 2511–2527. doi:10.1111/j.1365-2966.2011.18569.x
Vázquez-Semadeni, E., Gazol, A., and Scalo, J. (2000). Is thermal instability significant in turbulent galactic gas? Astrophysical J. 540, 271–285. doi:10.1086/309318
Wada, K., and Koda, J. (2004). Instabilities of spiral shocks - I. Onset of wiggle instability and its mechanism. MNRAS 349, 270–280. doi:10.1111/j.1365-2966.2004.07484.x
Wada, K., Meurer, G., and Norman, C. A. (2002). Gravity-driven turbulence in galactic disks. Astrophysical J. 577, 197–205. doi:10.1086/342151
Wada, K., and Norman, C. A. (1999). The global structure and evolution of a self-gravitating multiphase interstellar medium in a galactic disk. Astrophysical J. 516, L13–L16. doi:10.1086/311987
Wada, K., and Norman, C. A. (2001). Numerical models of the multiphase interstellar matter with stellar energy feedback on a galactic scale. Astrophysical J. 547, 172–186. doi:10.1086/318344
Wada, K., Spaans, M., and Kim, S. (2000). Formation of cavities, filaments, and clumps by the nonlinear development of thermal and gravitational instabilities in the interstellar medium under stellar feedback. Astrophysical J. 540, 797–807. doi:10.1086/309347
Wada, K., Taniguchi, Y., Habe, A., and Hasegawa, T. (1994). Gasdynamical approach to a face-on view of the Milky way. Astrophysical J. 437, L123. doi:10.1086/187698
Walch, S., Girichidis, P., Naab, T., Gatto, A., Glover, S. C. O., Wünsch, R., et al. (2015). The SILCC (SImulating the LifeCycle of molecular Clouds) project - I. Chemical evolution of the supernova-driven ISM. MNRAS 454, 246–276. doi:10.1093/MNRAS/stv1975
Wareing, C. J., Pittard, J. M., Falle, S. A. E. G., and Van Loo, S. (2016). Magnetohydrodynamical simulation of the formation of clumps and filaments in quiescent diffuse medium by thermal instability. MNRAS 459, 1803–1818. doi:10.1093/MNRAS/stw581
Watkins, E. J., Barnes, A. T., Henny, K., Kim, H., Kreckel, K., Meidt, S. E., et al. (2023a). PHANGS-JWST first results: a statistical view on bubble evolution in NGC 628. Astrophysical J. 944, L24. doi:10.3847/2041-8213/aca6e4
Watkins, E. J., Kreckel, K., Groves, B., Glover, S. C. O., Whitmore, B. C., Leroy, A. K., et al. (2023b). Quantifying the energetics of molecular superbubbles in PHANGS galaxies. A&A 676, A67. doi:10.1051/0004-6361/202346075
Whitworth, D. J., Smith, R. J., Klessen, R. S., Mac Low, M.-M., Glover, S. C. O., Tress, R., et al. (2023). Magnetic fields do not suppress global star formation in low metallicity dwarf galaxies. MNRAS 520, 89–106. doi:10.1093/MNRAS/stad105
Wibking, B. D., and Krumholz, M. R. (2023). The global structure of magnetic fields and gas in simulated Milky Way-analogue galaxies. MNRAS 521, 5972–5990. doi:10.1093/MNRAS/stac2648
Wiener, J., Zweibel, E. G., and Oh, S. P. (2013). Cosmic ray heating of the warm ionized medium. Astrophysical J. 767, 87. doi:10.1088/0004-637X/767/1/87
Williamson, D. J., Thacker, R. J., Wurster, J., and Gibson, B. K. (2014). Cloud angular momentum and effective viscosity in global SPH simulations with feedback. MNRAS 442, 3674–3685. doi:10.1093/MNRAS/stu1121
Wu, B., Tan, J. C., Christie, D., Nakamura, F., Van Loo, S., and Collins, D. (2017). GMC collisions as triggers of star formation. III. Density and magnetically regulated star formation. Astrophysical J. 841, 88. doi:10.3847/1538-4357/aa6ffa
Keywords: interstellar medium, galaxies, star formation-galaxies, star formation-HII regions-molecular clouds, numerical simulations
Citation: Dobbs C (2023) 2a Results: galaxy to cloud scales. Front. Astron. Space Sci. 10:1272771. doi: 10.3389/fspas.2023.1272771
Received: 04 August 2023; Accepted: 20 October 2023;
Published: 09 November 2023.
Edited by:
Vincenzo Ripepi, Astronomical Observatory of Capodimonte (INAF), ItalyReviewed by:
Diego Falceta-Gonçalves, University of São Paulo, BrazilKeping Qiu, Nanjing University, China
Copyright © 2023 Dobbs. This is an open-access article distributed under the terms of the Creative Commons Attribution License (CC BY). The use, distribution or reproduction in other forums is permitted, provided the original author(s) and the copyright owner(s) are credited and that the original publication in this journal is cited, in accordance with accepted academic practice. No use, distribution or reproduction is permitted which does not comply with these terms.
*Correspondence: Clare Dobbs, Yy5sLmRvYmJzQGV4ZXRlci5hYy51aw==