- Physics Division, Oak Ridge National Laboratory, Oak Ridge, TN, United States
Research into the cosmic synthesis of the elements, the evolution and explosion of stars, the nature of the early Universe, and other important topics in nuclear astrophysics are at the forefront of nuclear science. These studies are motivating laboratory measurements and theoretical calculations that, after significant investments, are pushing the boundaries of what is possible. The latest nuclear results, however, must be specially prepared before they can be used to advance our knowledge of the cosmos. This processing requires a set of resources unique to nuclear astrophysics, and an impressive collection of nuclear reaction and nuclear structure datasets, processing codes, thermonuclear reaction rate libraries, and simulation codes and services have been developed for the field. There are, however, some serious challenges to these efforts that will only worsen in the future, making it important to develop strategies and act now to ensure a sustainable future for this work. After detailing the specific data types needed for nuclear astrophysics and the available data resources, the major challenges in this work and their implications are discussed. A set of initiatives are proposed to meet those challenges along with suggested implementations and possible ways that they may advance our understanding of the Universe and strengthen the field of nuclear astrophysics.
1 Introduction
1.1 Importance of nuclear astrophysics
Nuclear astrophysics addresses many fascinating unsolved puzzles in the cosmos. Some of these are broad questions, such as the origin of the elements heavier than Fe (National Research Council, 2003). Others are more specific, such as: the cosmic origins of the rare 180Ta (de Laeter and Bukilic, 2005), 92−94Mo and 96−98Ru (Bliss et al., 2018); the origins of the very abundant (and fragile) 19F (Sieverding et al., 2018); and the formation of 7Li 3 min after the Big Bang (Cyburt et al., 2008). Some mysteries are tied to specific astrophysical environments, such as: the heaviest elements created in nova explosions (Bode and Evans, 2012; Liang et al., 2020); the nucleosynthesis in neutron star mergers as driven by neutron captures on n-rich unstable nuclei (Wanajo et al., 2021); the formation of heavy elements in core-collapse supernovae (Yamazaki et al., 2022); and the possible ejection of p-nuclides from X-ray bursts (Petrovici et al., 2019). Some puzzles impact the Universe as a whole, such as a constraint on the total amount of baryonic matter via the formation of light elements in the early Universe (Walker et al., 1991; Smith et al., 1993). Others focus on the dynamics of particular events, such as the mechanism of thermonuclear supernova explosions (Poludnenko et al., 2019), or broader questions such as the overall impact of the 12C(α, γ)16O reaction rate on stellar evolution (Pepper et al., 2022). The popularity of the field of nuclear astrophysics is growing due to new astrophysical observations [e.g., neutron star mergers (Wanajo et al., 2021)], new observatories [e.g., the James Webb Telescope (Natarajan et al., 2017)], and new accelerator facilities [e.g., FRIB (Wei et al., 2019), RIBF (Motobayashi and Sakurai, 2012), FAIR (Scheidenberge, 2017), RAON (Hong, 2023)]. For these (and many other) reasons, nuclear astrophysics has become firmly established as a major component of low-energy nuclear physics research, as reflected in long range plans for nuclear science in the US (Nuclear Science Advisory Committee, 2015) and Europe (NUPECC, 2017).
1.2 Importance of nuclear data for nuclear astrophysics
Nuclear data is essential to study the mysteries mentioned above. One reason: nuclear interactions drive the evolution of stars and their synthesis of elements, so data on these provide an empirical foundation for nuclear astrophysics studies. Another reason is that nuclear data is needed to plan new laboratory measurements of cross sections and level properties that are critical for nuclear astrophysics. Furthermore, nuclear data provides valuable benchmarks for reaction models that provide thousands of cross sections that are inaccessible to measurement. Finally, processed nuclear data in the form of thermonuclear reaction rates (discussed below) are critical input for the astrophysical simulations that advance our field. Some specific examples are simulations that determine the sensitivity of billion-dollar satellites to detect exploding stars, identify high priority measurements at radioactive beam facilities, determine the astrophysical impact of recent measurements, and assess the uncertainties of astrophysical model predictions to enable quantitative comparisons with observations.
The experimental and theoretical efforts in nuclear physics needed for astrophysics studies are well documented in numerous review articles [e.g., Smith and Rehm (2001); Adelberger et al. (2011); Käppeler et al. (2011)], community white papers (e.g., Schatz et al., 2022), and nuclear science long range plans (e.g., Nuclear Science Advisory Committee, 2015; NUPECC, 2017). However, the efforts to compile, evaluate, process, and disseminate this information for use by the nuclear astrophysics research community—essential steps to ensure the best nuclear data is used to advance the field—are generally not included in such review and planning documents. These steps form the “nuclear data pipeline” (Schnabel et al., 2021), and they ensure that the most up-to-date, precise, and accurate results from measurements and theoretical calculations are utilized in astrophysics simulations to advance the field.
This document addresses these essential nuclear data steps and their associated resources. First, the data types specifically needed for nuclear astrophysics research are briefly described in Section 1.3. In Section 2, details are given for the available resources in nuclear reaction data, nuclear structure data, thermonuclear reaction rates, simulation codes, processing codes, software services, and more. The goal of Section 2 is to inspire researchers to fully utilize this impressive breadth of resources to facilitate their work. Unfortunately, some of these datasets are missing the latest experimental and theoretical results, some of the services are based on decades-old technology or outdated data, and most of the resources lack a path forward for updates and upgrades. These and related problems will be discussed in Section 3, followed by a prioritization of the most critical data needs going forward in Section 4—along with possible solutions in the form of initiatives. Finally, the challenges of implementing these initiatives is discussed in Section 5.
1.3 Specialized nuclear data needs
The overall nuclear data needs for nuclear astrophysics studies are quite specialized [see, e.g., Smith (2003); Smith et al. (2008); Smith MS. (2011)], spanning nuclear reaction data, nuclear structure data, and processed data (i.e., thermonuclear reaction rates); these data types are described briefly below. Because of the specific nature of these reaction and structure data needs (e.g., which isotopes, which reactions, which properties, which energies), specialized efforts are required to obtain the data through measurements and theory. However, specialized efforts are also required to subsequently compile, evaluate, process, and disseminate these important data so that they can be used in astrophysical studies.
1.3.1 Nuclear reaction data
Reaction data is essential in nuclear astrophysics to track the changes in composition and the generation of energy inside stellar systems. Reactions are divided into two classes, strong (e.g., captures) and weak (e.g., decays). For strong reactions, interaction probabilities are characterized by cross sections. Nuclear astrophysics studies do not, however, require cross sections of all reaction types at all energies; Figure 1 shows the set of reaction types most predominantly utilized in this field. Noted exceptions to this are the important 12C + 12C, 12C + 16O, 16O + 16O, and similar reactions occurring in carbon and oxygen burning stages of massive stars where the low abundance of light nuclei causes heavy ion reactions to dominate (Nagorcka et al., 1971; Rolfs and Rodney, 1988). Furthermore, cross sections for different reaction types are needed for nuclei in different locations of the nuclear chart (Figure 1). For example, neutron captures are important for stable nuclei and those that have a neutron excess, while proton and alpha captures are needed for stable nuclei and those with a proton excess. Similarly, beta decays are critical for nuclei with excess neutrons, while positron decays are needed for nuclides on the p-rich side of stability. Finally, cross sections are needed at the low relative energies (typically ≤1 MeV/u) characteristic of the interactions of nuclides inside stars. Such low interaction energies are both a help—by placing only modest requirements on accelerators and detection systems—and a hindrance—by causing much lower yields due to the Coulomb barrier—to direct measurements of astrophysical reactions.
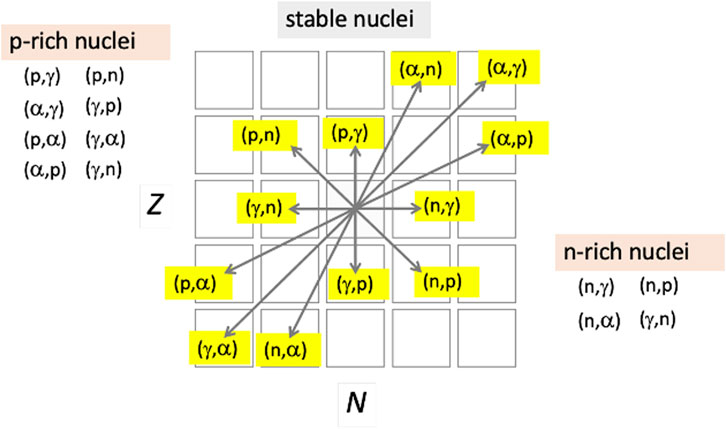
FIGURE 1. Reactions of importance for nuclear astrophysics shown on the N-Z plane for stable nuclei (center), and as lists for proton-rich nuclei (right), and neutron-rich nuclei (left).
1.3.2 Nuclear structure data
Extensive data on the structure of nuclei are also needed for astrophysical studies. The properties of single-particle levels that are within ∼1 MeV of a particle threshold are of particular importance: these levels have the correct energy and configuration to be readily populated by particle capture and other reactions that drive astrophysical processes. In contrast, such reactions do not favor populating levels at higher excitation energy or with collective configurations (e.g., rotational or vibrational excitations). By measuring single-particle level properties—or calculating them with theoretical models—the resonant cross sections (and thermonuclear reaction rates, discussed below) can be determined (Rolfs and Rodney, 1988). This “indirect” approach at estimating thermonuclear rates is especially valuable for nuclei far from stability (Smith and Rehm, 2001) where capture reactions cannot be measured directly, forcing a reliance on other reaction measurement approaches (e.g., transfer reactions, surrogate reactions, decay studies) or on theoretical models. Because resonant contributions to reaction rates depend exponentially on resonance energies, precision energy values are needed. Other important level properties include the partial and total widths, spectroscopic factors, spin-parities, and separation energies (e.g., reaction Q-values or nuclear masses).
Significant experimental and theoretical efforts have been directed at nuclear mass determinations, leading to high precision measurements [e.g., with particle traps (Kankainen et al., 2020)] and sophisticated global models with RMS differences from measurements as low as 600 keV (Goriely, 2023a). Machine learning has been utilized in numerous recent studies of nuclear masses—as well as in other areas of nuclear physics (Boehnlein et al., 2022)—with some studies achieving ∼200 keV RMS deviation from evaluated masses (Shelley and Pastore, 2021). Because there is so much activity in nuclear mass research, a comprehensive review of progress would greatly benefit the field; many advances have been made in the 20 years since the last review (Lunney et al., 2003).
For stable nuclei, many of the relevant properties have been measured, with the exception of a fair number of partial widths and spectroscopic factors for near-threshold single-particle levels. For studies of individual nuclei, shell model codes [e.g., Brown (2004)] and ab initio approaches [e.g., Hergert (2020)] are commonly used. Calculations over the entire nuclear chart are absolutely essential to provide all the necessary data for certain astrophysical simulations. Such “global” calculations are, for example, critical for the neutron-rich, far-from-stability nuclei that are the epicenter of the rapid neutron capture process (r-process) (Cowan et al., 2021), because few of the relevant level properties have been measured and most are experimentally inaccessible. A recent review article (Goriely, 2023a) provides an in-depth discussion of theoretical approaches to model structure information, including level densities, optical model potentials, gamma-ray strength functions, fission product distributions, beta decays, and nuclear masses.
There are other structure data needs in addition to those mentioned above. For example, the properties of bound levels are necessary to calculate the direct reaction cross section component (Brune and Davids, 2015). Also, spontaneous and neutron-induced fission yield distributions are important to treat the synthesis of the heaviest elements in the r-process (Côté et al., 2018); details of this are discussed in Section 2.7. Finally, for weak reactions, beta- and positron-decay lifetimes are needed, as well as delayed single- and multiple-particle emission probabilities after decay; weak reactions are described in more detail in Section 2.3.
1.3.3 Thermonuclear reaction rates
Cross sections can be converted to thermonuclear reaction rates through a convolution with the Maxwell-Boltzmann temperature-dependent energy distribution of interacting particles in a star (Rolfs and Rodney, 1988). This holds for cross sections obtained from laboratory measurements, as well as for those calculated from a reaction model or from the properties of relevant levels. The rates of charged particle reactions depend exponentially on temperature, with some rates varying by 30 orders of magnitude or more over the 107 K–1010K temperature range relevant for astrophysical environments. Figure 2 shows a typical example, the rate for the 17F(p,γ)18Ne reaction that plays a critical role in the synthesis of 17O in nova explosions (Bardayan et al., 1999). Collections of hundreds to thousands of individual reaction rates—rate “libraries”—are the core nuclear physics input for simulations of astrophysical environments.
2 Available resources
2.1 Nuclear reaction data and codes
2.1.1 The evaluated nuclear data file (ENDF)
The Evaluated Nuclear Data File ENDF (Brown et al., 2018; National Nuclear Data Center, 2023a) is a reaction cross section database that is managed and hosted at the National Nuclear Data Center (NNDC) (Brookhaven National Laboratory, 2023b) in the US. ENDF has an overwhelming focus on neutron-induced reactions because of their importance in nuclear energy and nuclear science applications. ENDF only has entries for reactions that have been measured in laboratories—that is, it contains no cross sections calculated entirely from theoretical models. ENDF entries are evaluated, meaning that nuclear reaction models (discussed below) are used by experts to combine multiple measurements to quantitatively determine the “best” value, to extrapolate to higher (∼20 MeV/u) or lower energies, to calculate all reaction channels at all angles, and to determine robust uncertainty information in the form of covariances (Smith DL., 2011; NNDC, 2023a).
Data from individual experiments are not stored in ENDF; these are stored in the EXFOR database (Zerkin and Pritychenko, 2018; IAEA Nuclear Data Services, 2023a). Members of the nuclear data community work with researchers to compile and import experimental results into EXFOR for subsequent dissemination to the community. ENDF evaluations, which are coordinated by the Cross Section Evaluation Working Group (CSEWG) (NNDC, 2023b), are significantly expedited by importing data into the standardized EXFOR format.
ENDF is not the only evaluated library of its kind: other similar libraries include the Joint European Fusion File (JEFF) (Nuclear Energy Agency, 2023), the Japanese Evaluated Nuclear Data Library (JENDL) (Japan Atomic Energy Agency, 2023), the Russian Nuclear Data Library (ROSFOND) (Inst Phys Power Engr, 2023), and the Chinese Evaluated Nuclear Data Library (CENDL) (Chinese Institute of Atomic Energy, 2023). The ENDF database format, unchanged since the 1960s, will soon be replaced by the Generalised Nuclear Data Structure (GNDS) format (NEA OECD, 2023). This follows more than a decade of work by international data experts to bring this critical database into the modern era.
2.1.2 ENDF and nuclear astrophysics
Because ENDF contains neutron capture reaction cross sections on stable isotopes, it can be used in simulations of the slow neutron capture process (s-process) (Käppeler et al., 2011) in AGB stars (Busso et al., 1999). In Section 2.3 below, the generation of reaction rates from ENDF cross sections will be discussed. It should be noted that, for s-process studies, there is a need to supplement ENDF cross sections with cross sections of neutron captures on long-lived radioactive “branch-point” isotopes (Bisterzo et al., 2015) that are a few mass units from stability.
During the evaluation process, the cross sections in ENDF are adjusted to agree with benchmarks from nuclear criticality safety and nuclear reactors. While this is ideal for nuclear science applications, it does mean that the cross sections are optimized at energies above those typically found in astrophysical environments. Additionally, since the ENDF evaluation methodology (Brown et al., 2018; National Nuclear Data Center, 2023a) includes all reaction channels and angles over a broad energy range, it is rarely followed by researchers in nuclear astrophysics. Rather, more streamlined reaction evaluations focused on the total cross section (and uncertainty) at astrophysical (i.e., lower) energies are often favored in the nuclear astrophysics community. Such reaction “assessments” (using this term to distinguish from full ENDF evaluations) that are streamlined and optimized for nuclear astrophysics can, in some cases, generate significantly different cross sections than those in ENDF—and which can subsequently produce different element synthesis and energy generation in astrophysical simulations [e.g., Zhang et al. (2022)].
Because ENDF does not contain cross sections for the thousands of neutron capture reactions on short-lived, neutron-rich unstable nuclei that are critical for the rapid neutron capture process (r-process) in neutron star mergers (Wanajo et al., 2021) and core collapse supernovae (Yamazaki et al., 2022), this database is not suitable for r-process studies.
2.1.3 Nuclear reaction codes
Nuclear reaction codes play an invaluable role in data analysis and in data evaluations. Examples widely used for charged-particle induced reactions include FRESCO (Thompson and Nunes, 2009) for analyzing angular distributions using coupled channels, DWUCK (Kunz, 1996) and TWOFNR (Igarashi, 1977) which use a Distorted Wave Born Approximation (DWBA) approach, and AZURE (Azuma et al., 2010) for multi-level R-matrix fits. For neutron-induced reactions, the SAMMY (Larson, 2008) code is widely used for multilevel R-matrix fits to neutron data with a Bayesian approach. SAMMY has long been an integral part of the ENDF evaluation methodology.
Nuclear reaction codes are also critical for estimating cross sections that have not yet been (or cannot practically be) measured. For capture reactions in astrophysical environments, the total cross section is the sum of three different components: direct capture into bound states (Brune and Davids, 2015), resonant capture into low-lying (non-overlapping) single-particle levels, and capture into overlapping levels. Given the bound level properties, the direct capture component can be treated with a code like RADCAP (Bertulani, 2003); given properties of the individual resonances, a standard Breit-Wigner formulation can be used for the resonant capture component. These level properties are usually obtained from experimental results (e.g., ENSDF or XUNDL) or from theoretical models as discussed in Section 2.2. The third component, capture into overlapping levels, often dominates the direct and resonant capture contributions to cross sections. The third component is usually treated with a statistical (or Hauser-Feshbach) reaction mechanism model (Hauser and Feshbach, 1952).
2.1.4 Statistical model reaction codes
Statistical model codes, in fact, provide the overwhelming majority of reaction cross sections that (after being processed into reaction rates) are used in astrophysical simulations. Popular modern statistical model codes from the nuclear data community include CoH (Kawano et al., 2010; Kawano et al., 2016), EMPIRE (Herman et al., 2007), and TALYS (Goriely et al., 2008; Koning, 2023a); older codes include GNASH (Young et al., 1992) and ALICE (Dityuk et al., 1998). Of these, TALYS has obtained wide acceptance for many studies in basic and applied nuclear science—including nuclear astrophysics. This code performs advanced global nuclear reaction modeling with robust uncertainties, and contains multiple reaction components including pre-equilibrium reaction effects, multi-particle emissions, width fluctuations, coupled channels, nuclear deformation, fission products, and many choices for nuclear level densities.
TALYS is used to generate TENDL, the TALYS Evaluated Nuclear Data Library (Koning et al., 2019; Koning, 2023b), which combines reaction evaluations with TALYS calculations to obtain reaction cross sections with nearly complete coverage of the nuclide chart for certain reaction types. This coverage makes TENDL very useful for certain astrophysics studies—specifically, of s-process and r-process nucleosynthesis driven by neutron-induced reactions. It should be noted that TENDL cross sections are optimized at energies above those needed for astrophysics and are provided, for many reactions, on a ∼1 MeV energy grid—which does not have sufficient fidelity for the low energies needed for studies of most astrophysical systems.
The nuclear astrophysics community has also developed their own statistical model codes. Early efforts, such as those by Woosley et al. (1978), set the approach followed by later efforts: calculate all binary reactions where an intermediate-mass nucleus (in that case, oxygen to krypton) reacts with a proton, neutron, alpha particle, or gamma ray over a broad energy (temperature) range characteristic of astrophysical environments. The codes also generally use theoretical expressions for nuclear partition functions and the nuclear level density, unless experimentally determined excited states are available. F. Thielemann developed the SMOKER code (Thielemann et al., 1987) that was later advanced by T. Rauscher into NON-SMOKER (Rauscher and Thielemann, 2000; Rauscher, 2023a). This Hauser-Feshbach reaction model code is fine-tuned for astrophysical applications and has been used to calculate rates for (n, γ), (n, p), (n, α), (p, γ), (p, α), (α, γ), and their inverse reactions on nuclides from Ne to Bi and for isotopes ranging from the neutron to proton driplines. Reaction rates generated from NON-SMOKER are the foundation of the JINA REACLIB (Joint Institute for Nuclear Astrophysics, 2023a) reaction rate database described below. Nucleosynthesis simulations were used to benchmark NON-SMOKER rates (Hoffman et al., 1999) and demonstrate their advantages over some earlier statistical model rate collections [including Woosley et al. (1978)].
2.2 Nuclear structure data and codes
2.2.1 The evaluated nuclear structure data file (ENSDF)
The Evaluated Nuclear Structure Data File (ENSDF) (National Nuclear Data Center, 2023b) hosted at the NNDC (Brookhaven National Laboratory, 2023b) is the international standard database for evaluated structure properties of nuclei. Some of the information contained in ENSDF includes level energies, spin-parities, total and partial widths, spectroscopic factors, reaction Q values, 1- and 2-particle separation energies, BE (2) values, beta decay lifetimes, binding energies, pairing gaps, decay schemes and branching ratios, bibliographic tags for measurements of these properties (that link to Nuclear Science References (NSR) (Pritychenko et al., 2011)), and much more. ENSDF contains evaluated data on many low-lying, single-particle resonances that are critical for thermonuclear reactions, as well as on bound levels needed for direct capture cross section calculations.
Evaluators from around the world (International Atomic Energy Agency, 2023) contribute to ENSDF evaluations. A companion database, the eXperimental Unevaluated Nuclear Data Library (XUNDL) (Brookhaven National Laboratory, 2023a), holds data from individual experiments that are used by evaluators for their ENSDF evaluations. The formats for ENSDF and XUNDL are being modernized along with the database structure, and the visualization by NuDat (National Nuclear Data Center, 2023c) is being upgraded with new capabilities.
2.2.2 Other evaluated nuclear structure data
There are other sources of level information on nuclei. One is NUBASE2020 (Kondev et al., 2021), which is a companion to the 2020 Atomic Mass Evaluation (AME) (Huang et al., 2021; Wang et al., 2021) (discussed below). NUBASE contains properties of the ground state, and any metastable states, in over 3,100 nuclides; in contrast, ENSDF contains the properties of all levels in over 3,400 nuclides. The NUBASE evaluations are consistent with the masses in the AME, but are evaluated independently from ENSDF. NUBASE is online at the Atomic Mass Data Center (AMDC) (Atomic Mass Data Center, 2023), hosted at the IAEA Nuclear Data Service (International Atomic Energy Agency, 2023a).
For neutron resonances, the Atlas of Neutron Resonances (Mughabghab, 2023) is a valuable source of experimental information. This work contains an extensive list of the individual resonance parameters for each nucleus as determined from analyzing available captures, fissions, and total neutron cross sections. Thermal cross sections are also contained in this work, along with average resonance parameters. For charged particle reactions, there are numerous targeted studies in the nuclear astrophysics community that contain resonance properties to calculate capture reactions. Some of these just contain information on one or a few nuclei [see, e.g., Smith et al. (1993); Bardayan and Smith (1997); Nesaraja et al. (2007)] while others are more expansive in scope [e.g., Iliadis et al. (2001)].
2.2.3 Structure information from theoretical models
There are numerous resources for theoretical structure information. Predominant among these is the Reference Input Parameter Library (RIPL) (Capote et al., 2009; Capote, 2023). The product of an international effort, RIPL is a valuable collection of model calculations of nuclear information needed for reaction calculations and nuclear data evaluations. RIPL includes eight sub-libraries: masses, nuclear levels, resonance spacing, optical model, level densities, giant dipole resonances, fission barriers, and computer codes. RIPL has seen wide utilization through the nuclear data community and contains valuable information for nuclear astrophysics research.
A series of global theoretical model predictions have been published by Möller et al. (1995), Möller et al. (1997), Möller et al. (2016), Möller et al. (2019) and are available online at Möller (1997). These collections contain ground-state masses, deformations, alpha-decay Q values, half-lives, beta-decay Q-values for beta-minus and electron capture decays, beta-delayed neutron-emission probabilities, potential-energy surfaces, fission-potential-energy surfaces, and (for ground states) odd-proton and odd-neutron spins, proton and neutron pairing gaps, and proton and neutron separation energies. These quantities are calculated for nearly 9,000 nuclides starting at 16O and ranging from the proton-to neutron-drip line up to Z = 136. The 2012 version of the Finite Range Drop Model (FRDM) (Möller, 1997) is a central component of these models. The site also has single-particle level diagrams and other graphical information.
A separate, smaller collection of global structure model predictions were produced together with cross section predictions from the NON-SMOKER code (Rauscher, 2023a); these are posted online at Rauscher (2023b). The available structure quantities include nuclear partition functions, nuclear levels, and ground state contributions to nuclear rates. In similar fashion, the BRUSLIB (Goriely, 2023b) library, discussed below in Section 2.3, supplements its collection of thermonuclear reaction rates with theoretical structure data, including ground state and single-particle level properties, densities and potentials, nuclear level densities, partition functions, E1 strength functions, and fission properties. The importance of the properties of bound levels for direct capture on neutron-rich unstable nuclei was pointed out in Goriely (1998a) and remains a challenge to this day.
Finally, a number of structure datasets are posted the Nuclear Computational Low Energy Initiative (NUCLEI) site (NUCLEI Collaboration, 2023). This collaboration is using high performance computing and advanced theoretical techniques to model the properties of nuclei. The data posted at their site includes mass tables, global mass model predictions, tables of 2+ level energies, fission barriers, giant dipole resonance parameters, and more. They also have a repository of nuclear codes available for download.
2.2.4 Nuclear masses
The latest evaluated nuclear mass datasets from the AME effort, as well as their NUBASE structure properties dataset, are available online at the Atomic Mass Data Center (AMDC) (Atomic Mass Data Center, 2023), hosted at the IAEA Nuclear Data Service (International Atomic Energy Agency, 2023a); the masses are also published online in Huang et al. (2021) and Wang et al. (2021). The evaluated masses in the AME are critical for determining the energy release of thermonuclear reactions and for calculating cross sections of unmeasured reactions. There is also a longstanding (2008–2022) independent effort by B. Singh to compile nuclear mass measurements. Those compilations are posted online at the nuclearmasses.org service (Smith MS. et al., 2023), which enables customized visualization and rapid comparisons (e.g., RMS differences) between theoretical, experimental, and evaluated nuclear masses (Smith MS., 2011).
2.3 Thermonuclear reaction rates
Research in nuclear astrophysics has always relied heavily on libraries of thermonuclear reaction rates. Collections of rates of charged-particle induced reactions, neutron-induced reactions, weak nuclear reactions, and other collections are briefly described below. Descriptions of processing tools required to produce these libraries from reaction and structure data files are given in Section 2.4.
The rate libraries described below contain rates from assessments of individual reactions, from large-scale reaction model calculations, from other rate libraries, or from a combination of these sources. Most libraries are general purpose, valid over the temperature range of 107K–1010K that covers most astrophysical phenomenon. However, some individual assessments are made over truncated temperature ranges corresponding to the energies measured in particular laboratory experiments, and care must be taken to extrapolate such rates for extended temperature ranges to avoid any problematic behavior. An example of this can be found in Smith et al. (1993) wherein a solar energy-based 3H (α, γ)7Li rate is modified to be valid at Big Bang temperatures.
Three different formats are used for reaction rate libraries. In one format, rate values are provided on a temperature grid. Once the tabulated (temperature, rate) ordered pairs are read into an astrophysical simulation code for each reaction, these “pointwise” rates must be interpolated at each time step because the simulations require rates on a dynamically-adjusted set of temperatures. In another format, a single functional form is chosen as an analytical expression (of rate versus temperature) for all rates, with each reaction having a different set of parameters for that function; the parameters are generated by a fit to the rate values. Once the fit parameters are read in for all reactions, the value of any rate at any temperature (i.e., at any timestep) is calculated with that single functional form and its respective parameters; no interpolation is needed. The third format utilizes a different analytical functional form for each reaction rate. In that scheme, these functions, resulting from fits, are incorporated in the simulation code and each rate is calculated at any temperature with its respective function, again with no interpolation. There are advantages and disadvantages of each of these formats, based on the convenience to prepare the library, the accuracy of the resulting rate values, the speed of execution, and the complexity of the required coding. The choice of rate library formats, however, does not limit the simulation execution speed; rather, the execution is limited by the time to invert a large matrix at each time step. This matrix is used to solve a linearized system of abundance changes over small time steps (Hix and Meyer, 2006).
2.3.1 Charged particle-induced reactions
Early efforts to generate charged particle reaction rates for studies of stellar burning were carried out by W. Fowler and his collaborators, resulting in five published collections from 1967 to 1988 (Fowler et al., 1967; Fowler et al., 1975; Harris et al., 1983; Caughlan et al., 1985; Caughlan and Fowler, 1988). The last of these libraries (Caughlan and Fowler, 1988; Smith M. et al., 2023) contains 159 rates and served as a standard for well over a decade. That collection provides a different analytical function of temperature for each rate, as well as pointwise rate values. Other smaller charged-particle reaction rate collections were subsequently created, specialized for solar burning [e.g., Adelberger et al. (1998); Adelberger et al. (2011)], for nuclei in the mass range 20–40 (Iliadis et al., 2001), for nova nucleosynthesis [e.g., Politano et al. (1995); Starrfield et al. (1998); Iliadis et al. (2001); Starrfield et al. (2001)], for X-ray bursts [e.g., van Wormer et al. (1994); Schatz et al. (1998)], and for Big Bang nucleosynthesis [e.g., Wagoner et al. (1967); Wagoner (1969); Smith et al. (1993); Descouvemont et al. (2004)].
The NACRE (Angulo et al., 1999) rate collection was assembled by the first large, international, multi-institution effort to assess charged-particle induced rates for stellar burning. This library, published in 1999, contained 86 rates on mostly stable nuclei, many of them updated from rates in the CF88 collection (Caughlan and Fowler, 1988). The effort utilized a methodology of streamlined assessments directed at determining the total cross section [or astrophysical S-factor (Rolfs and Rodney, 1988)] at low energies, with a focus on the resonant contributions from single-particle levels near a particle threshold. NACRE contains analytical expressions for S-factors, while the rates are given pointwise on a temperature grid. NACRE-II (Xu et al., 2013a; Goriely, 2013), a 2013 update, featured new rates for 34 reactions and the use of potential models for extrapolating measured cross sections to lower energies.
Rates from the NACRE collections form the core of the Brussels nuclear reaction rate library (BRUSLIB) (Goriely, 2004; Aikawa et al., 2005; Xu et al., 2013b; Goriely, 2023b). The early versions of BRUSLIB also included theoretical rates calculated from the MOST statistical reaction code (Goriely, 1998b), while later BRUSLIB versions (Goriely, 2023b) use statistical model rates calculated from TALYS (Koning, 2023a) cross sections. As mentioned above, BRUSLIB includes a large collection of theoretical structure properties, and (as discussed below) it also includes rate of neutron-induced reactions. The Nuclear Network Generator NETGEN (Aikawa et al., 2005; Jorissen, 2023) is a companion software tool that generates pointwise reaction rates from BRUSLIB for subsequent use in nucleosynthesis calculations.
The JINA REACLIB library (Cyburt et al., 2010; Joint Institute for Nuclear Astrophysics, 2023a) is one of the most widely utilized rate libraries in astrophysical simulations. It is the default library for many of the nucleosynthesis codes discussed in Section 2.4. REACLIB had its origin in 1987 (Thielemann et al., 1987) as a set of statistical model calculations using the SMOKER Hauser-Feshbach reaction model code, the precursor code to NON-SMOKER (Rauscher and Thielemann, 2000). REACLIB features one functional form [described in Cyburt et al. (2010)] to fit (parameterize) all reaction rates, and the library itself is a table of the parameter values for each rate. REACLIB has since had many upgrades and expansions, including the addition of approximately 200 rates based on assessments performed by the nuclear astrophysics community [e.g., Schatz et al. (1998); Angulo et al. (1999); Iliadis et al. (2001); Xu et al. (2013a) and many more]. Approximately 48,000 of the total 55,000 rates in JINA REACLIB are based on statistical model calculations, specifically the 2008 version of NON-SMOKER code (Rauscher and Thielemann, 2000; Rauscher, 2023a). It should be noted that JINA REACLIB also contains rates for neutron-induced reactions, as discussed below.
An important advance in the methodology of reaction rate determinations was made with the STARLIB reaction rate library (Iliadis et al., 2010a; Iliadis et al., 2010b; Iliadis et al., 2010c; Longland et al., 2010; Sallaska et al., 2013; Iliadis, 2023). STARLIB features rate determinations, upper and lower limits, and probability distribution functions based on Monte Carlo propagations of nuclear level uncertainties through the reaction rate calculation. STARLIB contains 62 charged particle-induced reactions on nuclei in the mass range of 14–40 that are treated with this method. This library provides pointwise rates (no analytical formulae), and adds a large number (over 47,000) of rates determined from TALYS (Koning, 2023a) cross section calculations and additional rates from other sources (e.g., NACRE, decays from ENSDF, and more) (Sallaska et al., 2013) to produce a rate library useful for astrophysical simulations of proton-rich environments.
2.3.2 Neutron-induced reactions
For neutron-induced reactions, many collections do not provide thermonuclear reaction rates; rather, they feature Maxwellian-averaged Cross Sections (MACS) [see, for example, Käppeler (2012)] calculated at an average temperature characteristic of s-process nucleosynthesis. A MACS is obtained by folding the energy-dependent differential cross section with the thermal velocity distribution of neutrons in the stellar plasma, and is proportional to the thermonuclear rate divided by the mean thermal velocity. Many collections of MACS provided values only at kT = 25 keV [e.g., Kaeppeler et al. (1982); Käppeler (2012)], in part because many neutron-induced reaction measurements are made using the 7Li (p,n) reaction as a source of neutrons; this reaction produces a Maxwellian-like distribution of neutrons peaked at that energy (Beer et al., 1980). It should be noted that some authors prefer to provide MACS at kT = 30 keV [e.g., Bao et al. (2000)]. The approach of providing MACS at one average temperature is, however, problematic for simulations that feature thermonuclear burning at other temperatures. Examples of other important temperatures for stellar neutron-induced reactions include 8 keV for the 13C pocket formation after the third dredge up in AGB stars (Bisterzo et al., 2015), 90 keV for shell carbon burning in AGB stars (Käppeler, 2012), and up to 1 MeV for r-process nucleosynthesis (Horowitz et al., 2019).
Early efforts to generate collections of MACS for neutron-induced reactions—primarily for neutron captures—were made in the 1960s and 1970s by Macklin and Gibbons (1965) and Allen et al. (1971). These libraries formed the basis for early quantitative studies of s-process nucleosynthesis by W. Fowler and others. It should be noted that these collections included MACS on a grid of kT values from 5 to 90 keV, making them suitable for a wider range of studies than some later collections.
As experimental measurements improved, rate collections were revised and expanded. The collections of Kaeppeler et al. (1982), Bao and Kaeppeler (1987), and Bao et al. (2000) significantly advanced the quality of neutron capture data processed for s-process studies. A 2005 update of Bao et al. (2000) forms the core of the Karlsruhe Astrophysical Database of Nucleosynthesis in Stars (KADONIS) (Dillmann et al., 2006; Dillman, 2023), which is supplemented from rates from other sources as well. This is a web-based collection of experimental (n, γ) MACS relevant for studies of s-process nucleosynthesis. KADONIS was subsequently extended in 2013 to contain neutron capture rates on 32 p-rich unstable nuclei for studies of the p-process (Szucs et al., 2014).
In the nuclear data community, MACS have been calculated (Pritychenko et al., 2010; Pritychenko and Mughaghab, 2012; Pritychenko, 2020; National Nuclear Data Center, 2023d) for neutron capture reactions from cross sections in the ENDF (National Nuclear Data Center, 2023a), JEFF (Nuclear Energy Agency, 2023), JENDL (Japan Atomic Energy Agency, 2023), ROSFOND (Inst Phys Power Engr, 2023), and CENDL (Chinese Institute of Atomic Energy, 2023) libraries. Comparisons [e.g., Zhang et al. (2022)] between these MACS and those generated from experiments [e.g., (Dillman (2023)] or from nuclear models [e.g., (Rauscher (2023a)] originating from nuclear astrophysics studies may improve the overall quality of all MACS collections.
For studies of the astrophysical r-process, it is critical to have reaction rates rather than MACS because of the wide range of temperatures present in neutron star mergers and core-collapse supernovae. It is also necessary to have neutron capture rates on thousands of neutron-rich unstable nuclei, which necessitates the use of nuclear models. NON-SMOKER (Rauscher, 2023a) was used to generate such rates for the JINA REACLIB library (Joint Institute for Nuclear Astrophysics, 2023a); TALYS (Koning, 2023a) was similarly used to generate rates for BRUSLIB (Goriely, 2023b). Recently, TALYS was used to generate the TENDL-Astrophysics database (Koning, 2023c), which contains neutron capture reaction rates on 8,892 isotopes with uncertainties generated from 288 models that combine different choices of input parameters—gamma strength functions, level densities, optical models, collective enhancements, width fluctuations, and mass models. The incorporation of uncertainties and the complete coverage of neutron-induced reactions on stable and neutron-rich isotopes make this library useful for quantitative investigations of s-process and r-process nucleosynthesis.
2.3.3 Weak nuclear reactions
Weak nuclear reactions such as beta decays are very important for thermonuclear burning processes. For the r-process, these decays are critical input as they set the timescale of the burning and the final abundances [see, e.g., Langanke and Martínez-Pinedo (2003); Cowan et al. (2021)]. Beta-delayed single and multiple neutron emissions are also important as they alter the mass distribution of r-process abundances. Electron (positron) capture reactions are also needed for neutron-rich (proton-rich) nuclei. Where measurements of the beta- and positron-decay lifetimes of nuclei near stability exist, they are included in ENSDF (National Nuclear Data Center, 2023b); these values are often incorporated into thermonuclear rate libraries. For r-process simulations, however, thousands of weak rates on very neutron-rich nuclei are needed, and theoretical models are required to provide the needed data.
Important early theoretical studies of weak reactions in the nuclear astrophysics community were made by Fuller et al. (1980); Fuller et al. (1982a); Fuller et al. (1982b); Fuller et al. (1985). These collections included stellar weak interaction rates for intermediate-mass nuclei (up to mass 60) and are still used by some astrophysical simulation codes today (Timmes, 2023). Temperature dependences were soon added to tables of weak rates (Takahashi and Yokoi, 1987). Many other advances have followed, using a wide variety of theoretical approaches including microscopic models, shell-model Monte-Carlo, random phase approximation (RPA), quasiparticle RPA, relativistic RPA, finite-temperature relativistic nuclear field theory, and many more [see, e.g., Ferreira et al. (2014); Litvinova et al. (2020); Suzuki (2022); Goriely (2023a); Suzuki et al. (2023)]. While some tables of weak rates have been published in the literature [see, e.g., Takahashi and Yokoi (1987); Möller et al. (1997)], others have been folded into the major thermonuclear reaction rate libraries (described above) including JINA REACLIB (Joint Institute for Nuclear Astrophysics, 2023a), BRUSLIB (Goriely, 2023b), and others. The most recent global calculation, in Ney et al. (2020), employs a finite-amplitude method and quasiparticle RPA to calculate beta decays of nearly 4,000 neutron-rich nuclei; the beta decays and strength functions are available as a Supporting Material to the journal article (Ney et al., 2023).
2.4 Astrophysics simulation codes, processing tools, and software systems
2.4.1 Simulation codes
Astrophysics simulations play a pivotal role in enabling studies of the cosmic creation of elements and the evolution and explosion of stellar systems. The two major aspects of these simulations, the hydrodynamics and the thermonuclear burning, are closely coupled: energy generated by thermonuclear burning alters the hydrodynamics (i.e., temperature and density), and updated hydrodynamics alters the reaction rates and subsequent thermonuclear burning. While the ideal simulation would fully couple these two aspects—a high resolution three-dimensional (3D) numerical hydrodynamical treatment coupled to a complete thermonuclear reaction network—this is not yet possible (even in 2D) due to the extreme computational resources required. For this reason, simulations emphasizing the hydrodynamic aspects utilize a truncated reaction network (e.g., an alpha-nuclei network); this provides, in a computationally possible manner, an approximation to the nuclear energy generation that dynamically modifies the hydrodynamic conditions at each time step. The current state-of-the-art in core-collapse supernova modeling has, notably, now reached high resolution 3D numerical hydrodynamics simulations with a 160 nuclide network (Sandoval et al., 2021). With computing power now reaching the exaflop (1018 floating point operations per second) scale (ORNL, 2023), it is exciting to imagine that coupling 3D hydrodynamics and full thermonuclear burning will perhaps be achieved for some systems within a decade.
In contrast, there are two categories of simulations with modest computational requirements: those that combine an approximate 1D analytical hydrodynamics calculation with a full thermonuclear burn simulation, and “post-processing” codes that calculate a full thermonuclear burn over an static (imported) hydrodynamics profile. These simulations can routinely track compositional changes over thousands of nuclear species.
For each of these three categories of simulations, thermonuclear reaction rate libraries provide critical nuclear physics input. Most codes having a preferred rate library format of either pointwise rates or analytical rate formulae. Additionally, some codes have a default rate library that is hardwired, others enable rate library substitutions after a fair amount of effort, and still others make it easy to rapidly change rate libraries. Below, we describe a variety of astrophysical simulation codes that are available to the community.
The Modules for Experiments in Stellar Astrophysics (MESA) (Paxton et al., 2011; MESA Collaboration, 2023) is a widely used set of open source libraries for computational stellar astrophysics. It features a 1D stellar evolution module that couples hydrodynamics and nucleosynthesis using adaptive mesh refinement, and has separate modules for nuclear reaction rates, equations of state, and more. The default thermonuclear reaction rate library for MESA is a custom combination of 300 rates from CF88, NACRE, and others; there is an option to use rates from the JINA REACLIB collection as well.
The NUGRID post-processing nucleosynthesis code (Denissenkov et al., 2014; NUGRID Collaboration, 2023) has the capability of processing the multi-zone output of 1D stellar evolution codes such as MESA (Paxton et al., 2011; MESA Collaboration, 2023), and enables a dynamical adjustment of the size of the network depending on the composition. NUGRID has a reaction library that combines pointwise reaction rates from numerous sources including NACRE (Angulo et al., 1999), CF88 (Caughlan and Fowler, 1988), BRUSLIB (Goriely, 2023b), an early version of JINA REACLIB (Joint Institute for Nuclear Astrophysics, 2023a), and other sources. NUGRID also has an associated jupyter-based platform and python scripts.
XNet (Hix, 2023) is a fully-implicit post-processing nucleosynthesis code. It can be run in standalone mode or coupled to a hydrodynamics package. For the latter, XNet has been coupled to FLASH (Fryxell et al., 2000; Dubey et al., 2009) and CHIMERA (Bruenn et al., 2020) for studies of core collapse supernova simulations [see, e.g., Sandoval et al. (2021) mentioned above], as well as to other codes to study other phenomena. XNet uses thermonuclear rates from JINA REACLIB as a default, and can track ∼5,000 nuclide abundances for r-process studies. As described below, XNet has also been integrated into the Computational Infrastructure for Nuclear Astrophysics (CINA) (Smith, 2023) in a manner that enables rapid customization of input reaction rate libraries.
The Portable Routines for Integrated nucleoSynthesis Modeling (PRISM) nucleosynthesis code (Sprouse et al., 2020; Sprouse et al., 2021) is a modern code used for a novel “nucleosynthesis tracing” technique. PRISM relies primarily on theoretical reaction rates of r-process nuclei calculated with the statistical Hauser-Feshbach code CoH (Kawano et al., 2016).
SkyNet (Lippuner and Roberts, 2017) is another new nuclear reaction network code. It is written in a modular fashion and features significant attention to neutrino-induced nucleosynthesis. This code uses as default reactions from JINA REACLIB (Joint Institute for Nuclear Astrophysics, 2023a) and from other sources.
Torch (Timmes, 1999; Timmes, 2023) is an older general reaction network that is set for a 513 isotope network by default; this network can be expanded to include more species as needed. The default nuclear reactions are from a combination of an early version of REACLIB (Joint Institute for Nuclear Astrophysics, 2023a), NON-SMOKER (Rauscher, 2023a), and other sources.
The WinNet (Winteler, 2013) code is an updated version of the BasNet (Thielemann et al., 2011) reaction network; neither of these two codes are publicly distributed. WinNet can track over 5,800 nuclides, contains reactions from NON-SMOKER and many other sources, and has been used for studies of the r-process in core-collapse supernovae and in neutron star mergers [e.g., Korobkin et al. (2012)].
The Webnucleo nuclear reaction network (Meyer, 2012; Meyer, 2023) is a modular system for nucleosynthesis calculations available both in jupyter notebooks and in downloadable source code. This system accepts user-specified pointwise reaction rates as default inputs. The pynucastro library (Smith AI. et al., 2023) is a new open-source python library that enables interactive creation and exploration of nuclear reaction networks. The networks built with this system can be exported for use in large-scale simulation codes.
2.4.2 Processing Tools and software systems
Significant effort is required to prepare the thermonuclear reaction libraries that are the key nuclear input for all the simulations discussed above. Of all the necessary steps, the most critical are the calculation of resonant cross sections from the properties of near-threshold levels and the numerical integration of energy-dependent cross sections to form temperature-dependent thermonuclear reaction rates. These processing steps are well known and are discussed in books [e.g., Clayton (1984); Rolfs and Rodney (1988); Iliadis (2015)] as well as in many journal articles. For this reason, many nuclear astrophysics research groups have built, but rarely distribute, their own codes for such calculations. It should be noted, however, that some details (e.g., numerical integration routines) can produce differences in outputs from different codes.
Another important processing step is the fitting of temperature-dependent rates to analytical formulae. Such fits are challenging because reaction rates can vary by up to 30 orders of magnitude or more over astrophysical temperature ranges (see, e.g., Figure 2). Fits of rates to the REACLIB analytical formulation are, for example, discussed in Cyburt et al. (2010). Other important processing steps include creating inverse reaction rates from forward rates and checking that they obey the principle of detailed balance, converting cross sections to S-factors, numerically interpolating codes to generate thermonuclear rates at any temperature from pointwise rate tables, downloading and extracting data from standard databases [e.g., ENDF (National Nuclear Data Center, 2023a), ENSDF (National Nuclear Data Center, 2023b)], and more.
A number of these processing steps are contained within CINA (Nesaraja et al., 2005a; Nesaraja et al., 2005b; Smith, 2005; Smith et al., 2006; Smith, 2023). This software system is a unique online cloud-computing data “pipeline” that provides a simple graphical user interface (GUI) to guide users step-by-step in processing and using nuclear data in astrophysical simulations. Operating since 2004, CINA enables users to work with nuclear data, with thermonuclear reaction rates, and with nucleosynthesis simulations. For nuclear data, users can upload, manipulate, and save reaction cross sections. For thermonuclear rates, users can: convert cross sections or S-factors into thermonuclear reaction rates via numerical integration; modify, parameterize (i.e., fit to the REACLIB analytic formula), and save rates into rate libraries; and combine libraries to form custom collections that can run in nucleosynthesis simulations. For simulations, users can: upload custom hydrodynamic profiles; set up and execute post-processing nucleosynthesis simulations with the XNet code (Hix, 2023); run multi-zone simulations and compute zone-weighted final abundances; set up batch modes of runs over multiple rate libraries and hydrodynamic profiles; rapidly change nuclear physics inputs (i.e., reaction rates) and determine astrophysical impacts; run automated sensitivity studies for determining input (rate) vs output (abundance) correlations; save and visualize simulations with customizable plots and animations; analyze and annotate simulations; quantify uncertainties in predicted final abundances; and compare multiple simulation results. Information in CINA, including large custom rate libraries and simulation outputs, can easily be shared between users within the system as well as exported. CINA’s GUI is platform independent and runs on any machine with Java installed; the system is free to use and all users receive free disk space to store their runs. Since it is a remotely-executed cloud computing service, CINA requires users to have an active internet connection, but does not require any compilation, libraries, licenses, special environments, or user updates in order to operate. CINA utilizes the JINA REACLIB (Joint Institute for Nuclear Astrophysics, 2023a) database as the default rate library, but enables users to quickly create, save, share, and use customized rate libraries from within the GUI.
2.5 Bibliographic data
Bibliographic data is also critical for progress in nuclear astrophysics research. The three most valuable resources are described below, created by the astrophysics, nuclear data, and nuclear astrophysics communities, respectively. First, the NASA Astrophysical Data System ADS (NASA, 2023) is a widely used service that contains references from hundreds of journals in astrophysics and related fields, including those not accessed in other services like Monthly Notices of the Royal Astronomical Society (MNRAS) (Oxford Academic, 2023) and Publications of the Astronomical Society of the Pacific (PASP) (IOP Science, 2023). ADS also, for example, contains direct links to many articles (in HTML or PDF formats) published in the Astrophysical Journal (American Astronomical Society, 2023).
In the nuclear data community, the Nuclear Science References (NSR) (Pritychenko et al., 2011) bibliographic database is the standard source for reference material. NSR, which contains references to over 250,000 papers in nuclear physics going back over 100 years, includes a DOI number and a unique accession number for each entry; ENSDF nuclear structure evaluations list these accession numbers directly in their bibliographic database field. NSR entries also contain keywords which facilitates evaluation work as well as general searches. Finally, from the nuclear astrophysics community, the JINA Virtual Journal of Nuclear Astrophysics (Joint Institute of Nuclear Astrophysics, 2023b) is a valuable resource for research. The editors of this source scan 42 nuclear science and astrophysics journals for articles in nuclear astrophysics.
2.6 Dissemination services
Data dissemination refers to the distribution of datasets via downloads, access to datasets and their data by browsing/searching/filtering, and visualization. The two major dissemination services in the nuclear data community are the National Nuclear Data Center (NNDC) (Brookhaven National Laboratory, 2023) in the US and the Nuclear Data Services (International Atomic Energy Agency, 2023a) at the International Atomic Energy Agency (International Atomic Energy Agency, 2023b) in Vienna. These provide access to the unevaluated databases XUNDL (Brookhaven National Lab, 2023) and EXFOR (IAEA Nuclear Data Services, 2023a), the evaluated databases ENSDF (National Nuclear Data Center, 2023b) and ENDF (National Nuclear Data Center, 2023a), and many more datasets and services for basic and applied nuclear science. For any given dataset, there is no single dissemination method that satisfies the needs of the entire community. It is therefore common that different approaches are used at different nuclear data centers to access certain critical databases. For example, NuDAT (National Nuclear Data Center, 2023c) is used to access XUNDL and ENSDF at the NNDC, while LiveChart (IAEA Nuclear Data Services, 2023b) is used for similar purposes at the IAEA NDS. Because new dissemination tools are routinely added to the NNDC and IAEA NDS sites, it is recommended to regularly browse their websites for newresources.
In the nuclear astrophysics community, databases are usually disseminated via a website at the institution where the database is hosted. Many of these websites were referenced in the sections above. For example, rate libraries including JINA REACLIB (Joint Institute for Nuclear Astrophysics, 2023a), STARLIB (Iliadis, 2023), TENDL (Koning, 2023b), NACRE-II (Goriely, 2013), and KADONIS (Dillman, 2023) all have their own webpage for dissemination. In some cases, libraries can also be accessed through services that incorporate or manipulate them. For example, the JINA REACLIB library can be accessed through CINA (Smith, 2023), and NACRE and NACRE-II can be accessed through BRUSLIB (Goriely, 2023b). It is not uncommon for website addresses to change, however, as well as for web hosting to be discontinued. For example, the original web posting of the NACRE collection is no longer online. There are also examples of certain libraries being manually entered into websites, such as the CF88 library (Smith M. et al., 2023), while other datasets are posted as individual journal articles [e.g., Rauscher and Thielemann (2001)] or as the Supporting Material of an article [e.g., Ney et al. (2023)].
2.7 Other data
2.7.1 Nuclear equation of state
The nuclear equation of state (EOS) describes the characteristics (e.g., compressibility) and the dynamics of nuclear matter in ultra-dense systems such as neutron stars, core-collapse supernovae, and neutron star mergers (Oertel et al., 2017). In Sumiyoshi et al. (2020), considerations of the influence of neutron-rich matter, hyperons and quark matter, and hadron-to-quark transitions in EOS studies are reviewed. Because the EOS is needed, especially for supernova simulations, at finite temperature and various lepton fractions, the results are usually presented in a tabular fashion, and many studies [e.g., Shen (2014); Shen et al. (2020)] have produced, analyzed, and compared the impact of different EOS tables on neutron stars and related systems. While not all EOS reported in the literature are publicly distributed, an online archive of many EOS tables may be found at Shen (2023a). The EOS tables for some particular studies are also posted online [e.g., Shen (2023b); Lattimer (2023)].
2.7.2 Fission yields
In the synthesis of the heaviest elements in core-collapse supernovae and neutron star mergers, the series of neutron captures on n-rich unstable nuclei, the r-process, leads to the formation of nuclei that undergo fission [see, e.g., Côté et al. (2018)]. When fission causes these heavy nuclei break apart, this effectively prevents even more massive nuclei from being formed; it therefore helps determine the “endpoint” of the r-process. From as early as the 1930s [e.g., Bohr and Wheeler (1939)], the fission process has been a focus of a segment of the nuclear physics community. Despite much effort, the complex mechanism of fission has made it very challenging to model (Schunck and Robledo, 2016). For this reason, fission has rarely been incorporated in astrophysical simulations even though it plays a critical role in the decay of heavy nuclei. In Goriely (2015), the effects of fission recycling (where fission products undergo subsequent neutron captures leading to fission), fission fragment distributions, and the capture of prompt fission neutrons were investigated. The results of some fission models relevant for nuclear astrophysics are posted online: BRUSLIB (Goriely, 2023b) provides fission information for 1,000 isotopes with Z ranging from 90 to 110, and Goriely (2009) provides similar information. More recently, the Fission In R-process Elements (FIRE) collaboration (Côté et al., 2018; FIRE Collaboration, 2023) was formed to focus on integrating advanced models of spontaneous, neutron-induced, and β-delayed fission into rapid neutron capture (r-process) nucleosynthesis codes and examining the subsequent astrophysical impacts. A set of their fission yields are distributed at FIRE Collaboration (2022).
3 Challenges
The section above details both the specialized steps prepare nuclear data for use in astrophysical simulations, and the wide variety of data resources that help can help with this important work. While some of these resources were specifically developed for nuclear astrophysics data, others are more general purpose databases and tools developed for basic and applied nuclear science that nonetheless have significant utility for astrophysical simulations.
Despite the impressive list of available resources, the current status of nuclear data for nuclear astrophysics is problematic. Below we discuss some major challenges that constrain the scientific impact of current work and may impede future efforts.
3.1 Outdated reaction rate libraries
The most critical challenge is that the major reaction rate libraries discussed in Section 2 above do not contain the latest measurements and theoretical calculations. Because of the time required to compile, assess, and process datasets, some lag (perhaps ∼3 years) is expected between the publication of new results and their incorporation into databases. The current delays are, however, much longer. The JINA REACLIB database (Joint Institute for Nuclear Astrophysics, 2023b), for example, added no new rates for nearly a decade (2012–2021), then none since the addition of 46 new rates in 2021. Many measurements made in that decade are missing from this critical library. Also troubling is the 48,000 rates in JINA REACLIB based on NON-SMOKER (Rauscher, 2023a) cross sections have not been updated since 2009, and those particular cross sections utilized nuclear masses from the 2003 Atomic Mass Evaluation (Audi et al., 2003; Wapstra et al., 2003), which are nearly two decades out of step with the latest AME2020 values (Huang et al., 2021; Wang et al., 2021). Furthermore, the NON-SMOKER rates themselves have not been updated since 2011 (Rauscher, 2023b).
Regarding other major libraries, BRUSLIB (Goriely, 2023b) and NETGEN (Jorissen, 2023) were last updated in 2015, while STARLIB (Iliadis, 2023) has only added a few rates since 2015. Additionally, both BRUSLIB and STARLIB use rates from TALYS (Koning, 2023a) statistical model cross sections generated before 2015, and therefore do not take advantage of the significant progress in TALYS since that time (Koning et al., 2019). The NACRE effort (Angulo et al., 1999) ended in 1999, while NACRE-II (Xu et al., 2013a; Goriely, 2013) ended in 2013. KADONIS (Dillman, 2023) was completed in 2005 (with some updates in 2013), while the RIPL effort (Capote, 2023) ended in 2013.
The lack of assessment efforts and resulting time delay from measurements and theory calculations to incorporation into databases have a significant scientific impact: many astrophysics modelers are utilizing nuclear information that is many years out of date, which calls into question the reliability and accuracy of their model predictions, as well as the consistency of these predictions with other studies that have used more updated data. Actions that may reduce this time lag are discussed in Sections 4 and 5.
3.2 Growing demands, stagnant productivity
As mentioned in Section 1, this is an incredibly exciting time in the field of nuclear astrophysics. The number of new nuclear datasets will jump dramatically as new accelerator facilities [e.g., FRIB (Wei et al., 2019), RIBF (Motobayashi and Sakurai, 2012), FAIR (Scheidenberge, 2017), RAON (Hong, 2023)] begin measurements with unstable beams. Rapid progress in astrophysical simulations, including more realistic treatments of thermonuclear burning in multi-dimensional simulations and going beyond Sandoval et al. (2021) and into the exascale computing regime, will place more stringent demands on nuclear data than ever before. Additionally, a new generation of space observatories [e.g., the Origins Space Telescope (Origins Space Telescope Study Team, 2023a; Origins Space Telescope Study Team, 2023b) and the Lynx X-ray Observatory (Lynx X-ray Observatory Team, 2023)] promise decades of new discoveries that will likely push all aspects of astrophysical modeling—including the nuclear data input—to new directions and new extremes.
To ensure that this growing demand for nuclear data can be met, the productivity of efforts to compile, assess, and process data must increase. This increase could come from a combination of a larger workforce, a workforce with new capabilities, and advances in methodology. Presently, however, there is stagnation in all three areas. First, the workforce that has long pursued nuclear data work for nuclear astrophysics is subcritical and is not being readily replaced by new recruits, based on the number of publications and the authorship in the field as well as on anecdotal information. Secondly, the adoption of the latest advances in this field is lagging behind that in nuclear theory and other areas of nuclear science. Examples of this can be seen in, for example, Boehnlein et al. (2022) for progress in machine learning in nuclear physics, and Kolos et al. (2022) for nuclear applications. Third, with a number of important and valuable exceptions [e.g., Angulo et al. (1999); Sprouse et al. (2021); Iliadis et al. (2022); Mumpower et al. (2022); Smith AI. et al. (2023); Iliadis (2023); Smith (2023)], there have been limited methodology innovations in this field. As an example, no new methods of accessing thermonuclear reaction rates have been developed in nearly 40 years: the innovation of a single analytical functional form for all reaction rates (Thielemann et al., 1987) was in the mid-1980s, at about the same time that pointwise rates became popular (Caughlan et al., 1985), and this was 20 years after the introduction of using different formulae for each rate (Fowler et al., 1967).
By recognizing this coming “perfect storm” of growing demand and excitement in nuclear astrophysics coupled to stagnant productivity in the associated data effort, it may be possible to lessen the impact with prudent planning and new initiatives. Some possibilities are listed below in Sections 4 and 5.
4 Data needs and initiatives
By recasting the challenges discussed above in Section 3 into prioritized nuclear data needs, initiatives can be devised that may help ensure the longevity of efforts to best prepare nuclear data for use in astrophysical simulations. First, for the crisis of outdated reaction rate libraries, it is clear that [1] assessments of low-energy cross sections and near-threshold level properties are needed, along with [2] enhanced efforts in global nuclear structure and reaction calculations. To address the crisis of stagnant productivity in an era of growing demand, a solution may be found by [3] advancing the methodology in nuclear astrophysics data. These are all high priority needs for the nuclear astrophysics community. Below we discuss initiatives to meet these needs, then give suggestions for implementation in Section 5 below.
4.1 Enhanced cross section and level property assessments
Assessments of cross sections and level properties are the most time-consuming step in bringing lab results into astrophysics models. Unfortunately, the community is currently lacks a steady source of these assessments. In the U.S., for example, only 1% of the effort of the national nuclear data program (NNDC, 2023c) is directed at nuclear astrophysics topics, while at the same time the previous efforts in assessments (see Section 3) have been concluded. The few ongoing efforts in this area are primarily small projects in individual research groups that tend to be under- and haphazardly-funded, driven by specific short-term research goals, and pursued independently of others. As a result, these lack continuity, completeness, and a coordinated vision for the future. Establishing an effort in cross section and level property assessments for nuclear astrophysics is the critical step to initiate updates of the outdated thermonuclear rate libraries discussed in Section 3. While the particular choice of reactions and nuclear levels to assess may depend on many factors—sensitivity studies [e.g., Smith MS. (2011); Zhu et al. (2021)], recent experimental activities, the quality of existing datasets, requests by astrophysical modelers, the complexity of the evaluation, the interests of the evaluators, and the connections to astrophysical observations—it is clear that the problem of outdated thermonuclear rate libraries is most directly addressed by establishing a new assessment effort.
4.2 Enhanced global nuclear model calculations
Despite significant progress of recent efforts to provide theoretical cross sections and level properties across the nuclear chart (discussed in Sections 2.1 and 2.2), enhanced efforts in this work are needed—for nuclear astrophysics as well as for many related research areas. For cross sections, efforts with TALYS are advancing the field, but more work is needed with that code to calculate charged-particle induced reactions—and then to include the results in TENDL-Astro (Koning, 2023c). It is also important to increase the energy fidelity of low-energy TALYS cross sections on low-mass target nuclei. The use of other reactions codes like CoH (Kawano et al., 2016) and EMPIRE (Herman et al., 2007) for global calculations is also essential, to ensure that TALYS is not the sole source for this valuable information. Regarding work with global structure models, efforts are needed for comprehensive calculations of bound and near-threshold level properties, because of the non-negligible contributions of direct and resonant capture to the total capture cross section (Goriely, 1998a). It is possible that combining high performance computing techniques [e.g., NUCLEI Collaboration (2023)] with a variety of theoretical approaches [e.g., those described in Goriely (2023a)] could significantly improve structure property estimates that are essential for global reaction rate calculations. Overall, boosting effort in global model calculations of cross sections and level properties will be of tremendous benefit in nuclear astrophysics, as well as in basic and applied nuclear science.
4.3 Improved methodologies
As mentioned above in Section 3, expanding and evolving the workforce would certainly be an effective way to address the stagnant productivity of this work in an era of growing demand. In this review, however, we will focus on technical solutions to help productivity, specifically on [3] advancing the methodology in nuclear astrophysics data. Because some current codes in this community have their roots in outdated techniques (e.g., numerical approaches and approximations, poor documentation, lack of benchmarks), there may be opportunities for very cost-effective methodology improvements that “do more with less”. Changes in methodology can be inspired by advances in the underlying physics and by advances in other fields (e.g., machine learning, data science, high performance computing, and (see below) high energy physics), as well as by unexpected and innovative [“disruptive” (Williams, 2011)] ideas. In addition to boosting efficiency, new methodologies could result in improved quality, reproducibility, reliability, longevity, and provenance (i.e., tracing of data sources). Finally, given the recent emphasis on uncertainty quantification (UQ) efforts [e.g., Barnes et al. (2021); Kolos et al. (2022)], adding UQ where it is missing would be a significant methodology improvement.
One way to improve methodologies is to enhance development efforts of the software tools described in Section 2.4. As mentioned therein, many research groups have their own basic or specialized tools, and there is an established online data pipeline found in CINA (Smith, 2023) that processes cross sections into astrophysical simulations with a point and click GUI. Investments in expanding current capabilities, including folding in more advanced UQ and machine learning approaches, could significantly boost overall productivity in a very cost-effective manner. Some new features to develop include: batch processing of complete cross section libraries into rate libraries; quick transformation of rate libraries between formats used by leading simulation codes; toolkits (with templates, guides, Frequently Asked Questions, smart agents, and more) to speed reaction and level assessments; automated comparisons of rate libraries [e.g., Hoffman et al. (1999); Zhang et al. (2022)] downloading and extracting data from standard databases [e.g., ENDF (National Nuclear Data Center, 2023a), ENSDF (National Nuclear Data Center, 2023b)]; and benchmarks and validation/verification tools for datasets, processing codes, and simulation codes. Because different researchers have different needs, it is unlikely that any single processing service can meet the needs of the entire community, so such tools need not necessarily be centralized.
Inspiration for a new generation of software tools in nuclear astrophysics, however, could possibly be found by adopting approaches followed by large physics collaborations with vetted analysis software stacks [e.g., at RHIC (Potekhin, 1467 2023)] and roadmaps for future developments [e.g., the HEP Software Foundation (HEP Software Foundation, 2018)]. Aspects favored in such approaches include: developing modular, shared, community resources; ensuring resources can be scaled up, swapped, and customized; developing for longevity and sustainability; including robust documentation, debugging, and benchmarking; managing developments with software experts; minimizing duplication of effort; encouraging collaborations in data science and machine learning; and exploring new paradigms. Ultimately, whether incremental or next-generation in nature, methodology improvements have tremendous potential to improve the productivity—and many other aspects—of nuclear astrophysics data efforts.
5 Discussion
The priorities discussed above in Section 4 are to establish efforts to assess cross sections and level properties, to boost efforts in global model calculations, and to improve the methodologies used in nuclear astrophysics data work. Together, these initiatives would bring thermonuclear rate libraries more up-to-date and prepare the community to handle an onslaught of new measurements, theory calculations, and observations that may push the field in new directions. If executed well, additional benefits from these efforts may include improving their overall quality and longevity, forging stronger ties within the community and to related fields, attracting new recruits, and enabling more of the full scientific potential of measurements and theories to be explored.
While the science case for these initiatives was discussed above, in this section we briefly describe issues with their launching and implementation. First, it is critical to note that these are not risky concepts. More specifically, success of these proposed initiatives for nuclear astrophysics data do not first require an effort to demonstrate proof of principle, as there are successful exemplars of similar efforts from the past. Therefore, the success of these recommended efforts will hinge upon the well known challenges of obtaining funding, successful recruiting, and finding principle investigators with the needed technical background, motivation, and resolve.
Of these three initiatives, the first is undoubtedly the most challenging to implement: in contrast to global calculations that calculate thousands of rates at once, for example, each individual reaction must be assessed one at a time. To discuss the launch an assessment effort, it is critical to first specify the desired output and duration. A reasonable effort may have a goal of assessing approximately 100 reactions—enough to make a sizable impact on the field—in a time frame (∼5 years) typical of long topical collaborations. Assuming part-time collaborators (pursuing both research and assessments) who complete 2 assessments each per year, a group of 15 such collaborators could likely complete 100 assessments in 5 years, if year-long periods for initial training and coordination for final writing are included. These numbers are roughly consistent with the NACRE (Angulo et al., 1999) collaboration led by C. Angulo from 1993–1999, which had 28 collaborators from 8 countries who assessed 86 reactions. NACRE has, to date, been the only large, coordinated, international, multi-institutional collaboration for nuclear astrophysics assessments. It featured a standardized methodology, a thorough documentation, and internal peer reviews resulting in a high quality of work. For these reasons, NACRE is the successful exemplar of the proposed new assessment effort.
Of course, the output of any effort depends on the commitment and experience of the collaborators, the complexity of the assessments, whether the assessments are updates of previous work, and many other factors. This can be seen from the wide range of metrics of other past efforts: in 1991, 12 reactions were assessed in 1 year (Smith et al., 1993); the effort led by W. Fowler from 1967–1988 (Fowler et al., 1967; Fowler et al., 1975; Harris et al., 1983; Caughlan et al., 1985; Caughlan and Fowler, 1988) had 2 to 4 collaborators who assessed and updated between 36 and 159 reactions; and the effort led by Iliadis et al. (2001) had 5 collaborators who assessed 55 reactions. It should be noted that while there are a number of obstacles—funding, recruiting, sociological—that could hamper the launch of such a large initiative, there are also a number of advantages—extensive experience with virtual meetings, more previous efforts to learn from—that a modern effort has over the past. Most importantly, it has been 30 years since NACRE was formed, and it seems long overdue that a segment of the community came together to assess critical reactions and level properties and drive the field forward.
The challenges facing the launch of boosted efforts in global model calculations are more modest by comparison. For example, there are far fewer global reaction codes to develop than individual reactions to assess, and there are multiple potential funding sources and workforce pools to draw from because of overlaps with basic and applied nuclear science. Since this initiative is a boost (hopefully significant) to ongoing efforts, a key challenge would be to distinguish it from “business as usual”. By delineating and completing a strong set of deliverables, such as generating three global thermonuclear rate libraries along with a study comparing both the results and the codes, this challenge could be readily handled. In fact, there are many instances of theoretical collaborations that have successfully overcome this issue [e.g., for the theory of jets in relativistic heavy ion collisions (Gyulassy et al., 2015)], as well as successful theory comparison efforts [e.g., with statistical model codes (Hoffman et al., 1999) and R-matrix codes (Leeb et al., 2023)].
Finally, there are many different ways to configure an initiative to improve nuclear astrophysics data methodologies; this mirrors the range of possible activities and approaches mentioned above in Section 4. A low-cost approach would be to form a working group that regularly met to propose and discuss collaborative projects, new concepts, coding obstacles, connections with other fields, and short- and long-term planning. It would be advantageous if such a working group would strongly advocate a common conceptual and software framework—one that included defining the elements of a data pipeline and their characteristics with shared base code templates. In that way, disparate projects run independently by collaborators could work together in a virtual data pipeline that could be used by all.
Alternatively, a large scale, centrally managed initiative modeled after those in other fields (e.g., high energy physics (HEP Software Foundation, 2018)) could combine large, medium, and small scale projects to form a robust, benchmarked, vetted, cohesive software ecosystem for nuclear astrophysics research. The challenge of launching and sustaining such an effort would be offset by its technical advantages (described in Section 4) as well as by the achievement of building a system that could set the standard—for not just nuclear astrophysics, but also for the low energy nuclear science and astrophysics theory communities. Such an effort would require a combination of researchers, professional coders, project integration management experts, and students. It should be noted that an effort of this scale would be immensely valuable for recruiting a new generation into the field, and even more so if ties to machine learning and data science communities—and a commitment to community wide ideals [e.g., OPEN Data (Center for Open Science, 2023), FAIR (Wilkinson et al., 2016)]—were established as core components of the system.
6 Summary
The study of the cosmic synthesis of elements, the evolution and explosion of stars, the nature of the early Universe, and other important topics in nuclear astrophysics are now central to nuclear science. Studies of these puzzles motivate a wide range of laboratory measurements and theoretical calculations, but the latest results must be processed before they can be used in astrophysical simulations to advance the field. Highly specialized resources are required, and have been developed, for this processing, including nuclear reaction and nuclear structure data sets, thermonuclear reaction rate libraries, processing codes, and simulation codes and services. Unfortunately, many of these resources are out of date, lack an update plan, and do not utilize advanced approaches in data science. By establishing an effort to assess cross sections and level properties, boosting efforts in global model calculations, and improving nuclear astrophysics data methodologies, the latest nuclear data could be more rapidly incorporated into the astrophysical simulations that improve our understanding of the cosmos. These initiatives also have the potential to strengthen and expand the nuclear astrophysics community.
Author contributions
The author confirms being the sole contributor of this work and has approved it for publication.
Funding
This work was supported by the U.S. Department of Energy Office of Science, Office of Nuclear Physics, under Contract Number DE-AC05-00OR22725 at ORNL.
Acknowledgments
The author wishes to thank Caroline Nesaraja and Larry Zhang for helpful discussions, and the late Richard Meyer for encouraging the author to collaborate with the nuclear data community.
Conflict of interest
The author declares that the research was conducted in the absence of any commercial or financial relationships that could be construed as a potential conflict of interest.
Publisher’s note
All claims expressed in this article are solely those of the authors and do not necessarily represent those of their affiliated organizations, or those of the publisher, the editors and the reviewers. Any product that may be evaluated in this article, or claim that may be made by its manufacturer, is not guaranteed or endorsed by the publisher.
References
Adelberger, E. G., Austin, S. M., Bahcall, J. N., Balantekin, A. B., Bogaert, G., Brown, L. S., et al. (1998). Solar fusion cross sections. Rev. Mod. Phys. 70, 1265–1291. doi:10.1103/RevModPhys.70.1265
Adelberger, E. G., García, A., Robertson, R. G. H., Snover, K. A., Balantekin, A. B., Heeger, K., et al. (2011). Solar fusion cross sections. II. The pp chain and CNO cycles. Rev. Mod. Phys. 83, 195–245. doi:10.1103/RevModPhys.83.195
Aikawa, M., Arnould, M., Goriely, S., Jorissen, A., and Takahashi, K. (2005). BRUSLIB and NETGEN: The Brussels nuclear reaction rate library and nuclear network generator for astrophysics. Astron. Astrophys. 441, 1195–1203. doi:10.1051/0004-6361:20052944
Allen, B. J., Gibbons, J. H., and Macklin, R. L. (1971). Nucleosynthesis and neutron-capture cross sections. Adv. Nucl. Phys. 4, 205–259.
American Astronomical SocietyThe Astrophysical Journal (2023). The astrophysical journal. Available at: https://iopscience.iop.org/journal/0004-637X.
Angulo, C., Arnould, M., Rayet, M., Descouvemont, P., Baye, D., Leclercq-Willain, C., et al. (1999). A compilation of charged-particle induced thermonuclear reaction rates. Nucl. Phys. A 656, 3–183. doi:10.1016/s0375-9474(99)00030-5
Atomic mass data center. atomic mass evaluation - AME2020. Available at: https://www-nds.iaea.org/amdc/(2023).
International Atomic Energy Agencyenter (2023c). International network of nuclear structure decay data evaluators (NSDD). Available at: https://www-nds.iaea.org/nsdd/.
Audi, G., Wapstra, A. H., and Thibault, C. (2003). The AME2003 atomic mass evaluation: (II). Tables, graphs and references. Nucl. Phys. A 729, 337–676. doi:10.1016/j.nuclphysa.2003.11.003
Azuma, R. E., Uberseder, E., Simpson, E. C., Brune, C. R., Costantini, H., de Boer, R. J., et al. (2010). Azure: An R-matrix code for nuclear astrophysics. Phys. Rev. C 81, 045805. doi:10.1103/PhysRevC.81.045805
Bao, Z., Beer, H., Käppeler, F., Voss, F., Wisshak, K., and Rauscher, T. (2000). Neutron cross sections for nucleosynthesis studies. At. Data Nucl. Data Tables 76, 70–154. doi:10.1006/adnd.2000.0838
Bao, Z., and Kaeppeler, F. (1987). Neutron capture cross sections for s-process studies. At. Data Nucl. Data Tables 36, 411–451. doi:10.1016/0092-640x(87)90011-8
Bardayan, D. W., Blackmon, J. C., Brune, C. R., Champagne, A. E., Chen, A. A., Cox, J. M., et al. (1999). Observation of the astrophysically important 3+ state in 18Ne via elastic scattering of a radioactive 17F beam from 1H. Phys. Rev. Lett. 83, 45–48. doi:10.1103/PhysRevLett.83.45
Bardayan, D. W., and Smith, M. S. (1997). Expressions for the 14O(α,p)17F and 17F(p, γ)18Ne astrophysical reaction rates. Phys. Rev. C 56, 1647–1650. doi:10.1103/physrevc.56.1647
Barnes, J., Zhu, Y., Lund, K., Sprouse, T. M., Vassh, N., McLaughlin, G. C., et al. (2021). Kilonovae across the nuclear physics landscape: The impact of nuclear physics uncertainties on r-process-powered emission. Astrophys. J. 918, 44. doi:10.3847/1538-4357/ac0aec
Beer, H., and Käppeler, F. (1980). “The measurement of Maxwellian averaged capture cross sections for 138Ba, 140Ce, 175Lu and 176Lu with a special activation technique,” in Nuclear cross sections for technology. Editors J. L. Fowler, C. H. Johnson, and C. D. Bowman, 348.
Bertulani, C. A. (2003). Radcap: A potential model tool for direct capture reactions. Available at: https://people.nscl.msu.edu/∼brown/reaction-codes/radcap/radcap.pdf.
Bisterzo, S., Gallino, R., Käppeler, F., Wiescher, M., Imbriani, G., Straniero, O., et al. (2015). The branchings of the main s-process: Their sensitivity to α-induced reactions on 13C and 22Ne and to the uncertainties of the nuclear network. Mon. Not. Roy. Astron. Soc. 449, 506–527. doi:10.1093/mnras/stv271
Bliss, J., Arcones, A., and Qian, Y. Z. (2018). Production of Mo and Ru isotopes in neutrino-driven winds: Implications for solar abundances and presolar grains. Astrophys. J. 866, 105. doi:10.3847/1538-4357/aade8d
Bode, M. F., and Evans, A. (2012). Cambridge astrophysics series #43: classical novae (Cambridge: Cambridge Univ. Press).
Boehnlein, A., Diefenthaler, M., Sato, N., Schram, M., Ziegler, V., Fanelli, C., et al. (2022). Colloquium: Machine learning in nuclear physics. Rev. Mod. Phys. 94, 031003. doi:10.1103/RevModPhys.94.031003
Bohr, N., and Wheeler, J. A. (1939). The mechanism of nuclear fission. Phys. Rev. 56, 426–450. doi:10.1103/PhysRev.56.426
Brookhaven National Laboratory (2023a). eXperimental unevaluated nuclear data library (XUNDL). Available at: https://www.nndc.bnl.gov/ensdf/xundl/.
Brookhaven National Laboratory (2023b). National nuclear data center. Available at: https://www.nndc.bnl.gov/.
Brown, B. A. (2004). Oxbash for windows PC. Available at: https://people.nscl.msu.edu/∼brown/resources/MSU-NSCL-Report-No-1289-2004.pdf.
Brown, D. A., Chadwick, M. B., Capote, R., Kahler, A. C., Trkov, A., Herman, M. W., et al. (2018). ENDF/B-VIII.0: The 8th major release of the nuclear reaction data library with CIELO-project cross sections, new standards and thermal scattering data. Nucl. Data Sheets 148, 1–142. doi:10.1016/j.nds.2018.02.001
Bruenn, S. W., Blondin, J. M., Hix, W. R., Lentz, E. J., Messer, O. E. B., Mezzacappa, A., et al. (2020). Chimera: A massively parallel code for core-collapse supernova simulations. Astrophys. J. Suppl. Ser. 248, 11. doi:10.3847/1538-4365/ab7aff
Brune, C. R., and Davids, B. (2015). Radiative capture reactions in astrophysics. Ann. Rev. Nucl. Part. Sci. 65, 87–112. doi:10.1146/annurev-nucl-102014-022027
Busso, M., Gallino, R., and Wasserburg, G. J. (1999). Nucleosynthesis in asymptotic giant branch stars: Relevance for galactic enrichment and solar system formation. Ann. Rev. Astron. Astrophys. 37, 239–309. doi:10.1146/annurev.astro.37.1.239
Capote, R., Herman, M., Oblozinsky, P., Young, P. G., Goriely, S., Belgya, T., et al. (2009). RIPL – reference input parameter library for calculation of nuclear reactions and nuclear data evaluations. Nucl. Data Sheets 110, 3107–3214. doi:10.1016/j.nds.2009.10.004
Capote, R. (2023). Reference parameter input library - RIPL3. Available at: https://www-nds.iaea.org/RIPL-3/.
Caughlan, G. R., Fowler, W. A., Harris, M. J., and Zimmerman, B. A. (1985). Tables of thermonuclear reaction rates for low-mass nuclei (1 ⩽ Z ⩽ 14). At. Data Nucl. Data Tables 32, 197–233. doi:10.1016/0092-640X(85)90006-3
Caughlan, G. R., and Fowler, W. A. (1988). Thermonuclear reaction rates V. At. Data Nucl. Data Tables 40, 283–334. doi:10.1016/0092-640X(88)90009-5
Center for Open Science (2023). Mission statement. https://www.cos.io/about/mission.
Chinese Institute of Atomic Energy (2023). Chinese evaluated nuclear data library (CENDL). Available at: https://www.ciae.ac.cn.
Clayton, D. D. (1984). Principles of stellar evolution and nucleosynthesis. Chicago: Univ. of Chicago Press.
Côté, B., Fryer, C. L., Belczynski, K., Korobkin, O., Chruślińska, M., Vassh, N., et al. (2018). The origin of r-process elements in the milky way. Astrophys. J. 855, 99. doi:10.3847/1538-4357/aaad67
Cowan, J. J., Sneden, C., Lawler, J. E., Aprahamian, A., Wiescher, M., Langanke, K., et al. (2021). Origin of the heaviest elements: The rapid neutron-capture process. Rev. Mod. Phys. 93, 015002. doi:10.1103/RevModPhys.93.015002
Cyburt, R. H., Amthor, A. M., Ferguson, R., Meisel, Z., Smith, K., Warren, S., et al. (2010). The JINA REACLIB database: Its recent updates and impact on type-I X-ray bursts. Astrophys. J. Suppl. Ser. 189, 240–252. doi:10.1088/0067-0049/189/1/240
Cyburt, R. H., Fields, B. D., and Olive, K. A. (2008). An update on the big bang nucleosynthesis prediction for 7Li. J. Cosmol. Astropart. Phys. 11, 012. doi:10.1088/1475-7516/2008/11/012
de Laeter, J. R., and Bukilic, N. (2005). Isotope abundance of 180Tam and p-process nucleosynthesis. Phys. Rev. C 72, 025801. doi:10.1103/physrevc.72.025801
Denissenkov, P. A., Truman, J. W., Pignatari, M., Trappitsch, R., Ritter, C., Herwig, F., et al. (2014). MESA and NuGrid simulations of classical novae: CO and ONe nova nucleosynthesis. Mon. Not. Roy. Astron. Soc. 442, 2058–2074. doi:10.1093/mnras/stu1000
Descouvemont, P., Adahchoura, A., Angulo, C., Coc, A., and Vangioni-Flam, E. (2004). Compilation and R-matrix analysis of Big Bang nuclear reaction rates. At. Data Nucl. Data Tables 88, 203–236. doi:10.1016/j.adt.2004.08.001
Dillman, I. (2023). Karlsruhe astrophysical database of nucleosynthesis in stars (KADoNIS). Available at: https://exp-astro.de/kadonis.
Dillmann, I., Heil, M., Käppeler, F., Plag, R., Rauscher, T., and Thielemann, F. (2006). KADoNIS - the Karlsruhe astrophysical database of nucleosynthesis in stars. AIP Conf. Proc. 819, 123. doi:10.1063/1.2187846
Dityuk, A., Konobeyev, A. Y., Lunev, V. P., and Shubin, Y. N. (1998). New advanced version of computer code ALICE - eppe. Available at: https://inis.iaea.org/collection/NCLCollectionStore/_Public/29/024/29024357.pdf.
Dubey, A., Reid, L. B., Weide, K., Antypas, K., Ganapathy, M. K., Riley, K., et al. (2009). Extensible component based architecture for FLASH, A massively parallel, multiphysics simulation code. arXiv:0903.4875. doi:10.48550/arXiv.0903.4875
Ferreira, R. C., Dimarco, A. J., Samana, A. R., and Barbero, C. A. (2014). Weak decay processes in pre-supernova core evolution within the gross theory. Astrophys. J. 784, 24. doi:10.1088/0004-637x/784/1/24
FIRE Collaboration (2022). Fission in R-process elements. Available at: https://www.nndc.bnl.gov/fire/.
FIRE Collaboration (2023). Fission in R-process elements (FIRE). Available at: https://www.osti.gov/servlets/purl/1668514; https://www.osti.gov/biblio/1776653-fission-process-elements.
Fowler, W. A., Caughlan, G. R., and Zimmerman, B. A. (1967). Thermonuclear reaction rates. Ann. Rev. Astron. Astrophys. 5, 525–570. doi:10.1146/annurev.aa.05.090167.002521
Fowler, W. A., Caughlan, G. R., and Zimmerman, B. A. (1975). Thermonuclear reaction rates, II. Ann. Rev. Astron. Astrophys. 13, 69–112. doi:10.1146/annurev.aa.13.090175.000441
Fryxell, B., Olson, K., Ricker, P., Timmes, F. X., Zingale, M., Lamb, D. Q., et al. (2000). Flash: An adaptive mesh hydrodynamics code for modeling astrophysical thermonuclear flashes. Astrophys. J. Suppl. Ser. 131, 273–334. doi:10.1086/317361
Fuller, G. M., Fowler, W. A., and Newman, M. J. (1982b). Stellar weak interaction rates for intermediate mass nuclei. III - rate tables for the free nucleons and nuclei with A = 21 to A = 60. Astrophys. J. Suppl. Ser. 48, 279–319. doi:10.1086/190779
Fuller, G. M., Fowler, W. A., and Newman, M. J. (1982a). Stellar weak interaction rates for intermediate-mass nuclei. II - A = 21 to A = 60. Astrophys. J. 252, 715–740. doi:10.1086/159597
Fuller, G. M., Fowler, W. A., and Newman, M. J. (1985). Stellar weak interaction rates for intermediate-mass nuclei. IV - interpolation procedures for rapidly varying lepton capture rates using effective log (ft)-values. Astrophys. J. 293, 1–16. doi:10.1086/163208
Fuller, G. M., Fowler, W. A., and Newman, M. J. (1980). Stellar weak-interaction rates for sd-shell nuclei. I - nuclear matrix element systematics with application to Al-26 and selected nuclei of importance to the supernova problem. Astrophys. J. Suppl. Ser. 42, 447–473. doi:10.1086/190657
Goriely, S. (2013). NACRE-II. Available at: http://www.astro.ulb.ac.be/nacreii/.
Goriely, S. (2004). Bruslib: The Brussels nuclear library for astrophysics applications. AIP Conf. Proc. 704, 375. doi:10.1063/1.1737131
Goriely, S. (2023b). Brussels nuclear library for astrophysics applications (BRUSLIB). Available at: http://www.astro.ulb.ac.be/bruslib.
Goriely, S. (2009). HFB fission properties. Available at: http://www.astro.ulb.ac.be/pmwiki/Brusslib/FissionHfb.
Goriely, S., Hilaire, S., and Koning, A. J. (2008). Improved predictions of nuclear reaction rates with the TALYS reaction code for astrophysical applications. Astron. Astrophys. 487, 767–774. doi:10.1051/0004-6361:20078825
Goriely, S. (2023a). Nuclear properties for nuclear astrophysics studies. Eur. Phys. J. A 59, 16. doi:10.1140/epja/s10050-023-00931-x
Goriely, S. (1998a). Radiative neutron captures by neutron-rich nuclei and the r-process nucleosynthesis. Phys. Lett. B 436, 10–18. doi:10.1016/S0370-2693(98)00907-1
Goriely, S. (1998b). Radiative neutron captures by neutron-rich nuclei and the r-process nucleosynthesis. Phys. Lett. B 436, 10–18. doi:10.1016/s0370-2693(98)00907-1
Goriely, S. (2015). The fundamental role of fission during r-process nucleosynthesis in neutron star mergers. Eur. Phys. J. A 51, 22. doi:10.1140/epja/i2015-15022-3
Gyulassy, M., Paul, R., Bass, S., Müller, B., Strickland, M., Wang, X-N., et al. (2015). Final report (2010-2015) for the topical collaboration on quantitative jet and electromagnetic tomography (JET) of extreme phases of matter in heavy-ion collisions. https://www.osti.gov/servlets/purl/1242882.
Harris, M. J., Fowler, W. A., Caughlan, G. R., and Zimmerman, B. A. (1983). Thermonuclear reaction rates, III. Ann. Rev. Astron. Astrophys. 21, 165–176. doi:10.1146/annurev.aa.21.090183.001121
Hauser, W., and Feshbach, H. (1952). The inelastic scattering of neutrons. Phys. Rev. 87, 366–373. doi:10.1103/physrev.87.366
HEP Software Foundation (2018). A roadmap for HEP software and computing R&D for the 2020s. Available at: https://arxiv.org/pdf/1712.06982.pdf.
Hergert, H. (2020). A guided tour of ab initio nuclear many-body theory. Front. Phys. 8. doi:10.3389/fphy.2020.00379
Herman, M., Capote, R., Carlson, B. V., Obložinský, P., Sin, M., Trkov, A., et al. (2007). Empire: Nuclear reaction model code system for data evaluation. Nucl. Data Sheets 108, 2655–2715. doi:10.1016/j.nds.2007.11.003
Hix, W. R., and Meyer, B. S. (2006). Thermonuclear kinetics in astrophysics. Nucl. Phys. A 777, 188–207. doi:10.1016/j.nuclphysa.2004.10.009
Hix, W. R. (2023). XNet. Available at: https://eagle.phys.utk.edu/xnet/trac/;https://github.com/starkiller-astro/XNet.
Hoffman, R. D., Woosley, S. E., Weaver, T. A., Rauscher, T., and Thielemann, F. K. (1999). The reaction rate sensitivity of nucleosynthesis in type II supernovae. Astrophys. J. 521, 735–752. doi:10.1086/307568
Hong, B. (2023). Status of the RAON project in Korea. AAPPS Bull. 33, 3. doi:10.1007/s43673-022-00074-z
Horowitz, C. J., Arcones, A., Côté, B., Dillmann, I., Nazarewicz, W., Roederer, I. U., et al. (2019). r-process nucleosynthesis: connecting rare-isotope beam facilities with the cosmos. J. Phys. G. 46, 083001. doi:10.1088/1361-6471/ab0849
Huang, W. J., Wang, M., Kondev, F. G., Audi, G., and Naimi, S. (2021). The AME 2020 atomic mass evaluation (I). Evaluation of input data, and adjustment procedures. Chin. Phys. C 45, 030002. doi:10.1088/1674-1137/abddb0
IAEA Nuclear Data Services (2023a). Experimental nuclear reaction data (EXFOR). Available at: https://www-nds.iaea.org/exfor/.
IAEA Nuclear Data Services (2023b). Live chart of the nuclides. Available at: https://www-nds.iaea.org/relnsd/vcharthtml/VChartHTML.html.
Igarashi, M. (1977). Twofnr. Available at: https://people.nscl.msu.edu/∼brown/reaction-codes/twofnr/twofnr.pdf.
Iliadis, C., D’Auria, J. M., Starrfield, S., Thompson, W. J., and Wiescher, M. (2001). Proton-induced thermonuclear reaction rates for A = 20 - 40 nuclei. Astrophys. J. Suppl. Ser. 134, 151–171. doi:10.1086/320364
Iliadis, C., Longland, R., Champagne, A. E., and Coc, A. (2010b). Charged-particle thermonuclear reaction rates: III. Nuclear physics input. Nuc. Phys. A 841, 251–322. doi:10.1016/j.nuclphysa.2010.04.010
Iliadis, C., Longland, R., Champagne, A. E., and Coc, A. (2010c). Charged-particle thermonuclear reaction rates: IV. Comparison to previous work. Nuc. Phys. A 841, 323–388. doi:10.1016/j.nuclphysa.2010.04.012
Iliadis, C., Longland, R., Champagne, A. E., Coc, A., and Fitzgerald, R. (2010a). Charged-particle thermonuclear reaction rates: II. Tables and graphs of reaction rates and probability density functions. Nuc. Phys. A 841, 31–250. doi:10.1016/j.nuclphysa.2010.04.009
Iliadis, C., Palanivelrajan, V., and de Souza, R. S. (2022). Bayesian estimation of the s factor and thermonuclear reaction rate for 16O(p,γ)17F. Phys. Rev. C 106, 055802. doi:10.1103/PhysRevC.106.055802
Iliadis, C. (2023). Starlib. Available at: https://starlib.github.io/Rate-Library/.
Inst Phys Power Engr (2023). Rosfond. Available at: https://www.ippe.obninsk.ru/podr/abbn/libr/rosfond.php.
International Atomic Energy Agency (2023b). International atomic energy agency. Available at: https://www.iaea.org/.
International Atomic Energy Agency (2023a). Nuclear data services. Available at: https://www-nds.iaea.org/.
IOP Science (2023). Publications of the astronomical society of the pacific (PASP). Available at: https://iopscience.iop.org/journal/1538-3873.
Japan Atomic Energy Agency (2023). Japan evaluated nuclear data library (JENDL). Available at: https://wwwndc.jaea.go.jp/index.html.
Joint Institute for Nuclear Astrophysics (2023a). REACLIB. Available at: https://reaclib.jinaweb.org.
Joint Institute of Nuclear Astrophysics (2023b). JINA virtual journal of nuclear astrophysics. Available at: https://journals.jinaweb.org/jinavj/.
Jorissen, A. (2023). Netgen. Available at: http://www.astro/ulb.ac.be/Netgen/form.html.
Kaeppeler, F., Beer, H., Wisshak, K., Clayton, D. D., Macklin, R. L., and Ward, R. A. (1982). S-process studies in the light of new experimental cross sections - distribution of neutron fluences and r-process residuals. Astrophys. J. 257, 821–846. doi:10.1086/160033
Kankainen, A., Eronen, T., Nesterenko, D., de Roubin, A., and Vilen, M. (2020). Recent experiments at the JYFLTRAP Penning trap. Hyperfine Interact. 241, 43. doi:10.1007/s10751-020-01711-5
Käppeler, F., Gallino, R., Bisterzo, S., and Aoki, W. (2011). The s process: Nuclear physics, stellar models, and observations. Rev. Mod. Phys. 83, 157–193. doi:10.1103/RevModPhys.83.157
Käppeler, F. (2012). Stellar neutron capture rates and the s process. EPJ Web Conf. 21, 03006. doi:10.1051/epjconf/20122103006
Kawano, T., Capote, R., Hilaire, S., and Huu-Tai, P. C. (2016). Statistical Hauser-Feshbach theory with width-fluctuation correction including direct reaction channels for neutron-induced reactions at low energies. Phys. Rev. C 94, 014612. doi:10.1103/physrevc.94.014612
Kawano, T., Talou, P., Chadwick, M. B., and Watanabe, T. (2010). Monte Carlo simulation for particle and γ-ray emissions in statistical Hauser-Feshbach model. J. Nucl. Sci. Technol. 47, 462–469. doi:10.1080/18811248.2010.9711637
Kolos, K., Sobes, V., Vogt, R., Romano, C. E., Smith, M. S., Bernstein, L. A., et al. (2022). Current nuclear data needs for applications. Phys. Rev. Res. 4, 021001. doi:10.1103/PhysRevResearch.4.021001
Kondev, F., Wang, M., Huang, W., Naimi, S., and Audi, G. (2021). The NUBASE2020 evaluation of nuclear physics properties. Chin. Phys. C 45, 030001. doi:10.1088/1674-1137/abddae
Koning, A. (2023a). TALYS. Available at: https://www-nds.iaea.org/talys/.
Koning, A. (2023b). TALYS evaluated nuclear data library (TENDL). Available at: https://tendl.web.psi.ch/tendl_2021/tendl2021.html.
Koning, A. (2023c). TENDL-Astrophysics. Available at: https://tendl.web.psi.ch/tendl_2021/tar_files/astro/astro.html.
Koning, A. J., Rochman, D., Sublet, J. C., Dzysiuk, N., Fleming, M., and van der Mark, S. (2019). TENDL: Complete nuclear data library for innovative nuclear science and technology. Nucl. Data Sheets 155, 1–55. doi:10.1016/j.nds.2019.01.002
Korobkin, O., Rosswog, S., Arcones, A., and Winteler, C. (2012). On the astrophysical robustness of the neutron star merger r-process. Mon. Not. Roy. Astron. Soc. 426, 1940–1949. doi:10.1111/j.1365-2966.2012.21859.x
Kunz, P. D. (1996). DWUCK-4/5, scattering cross-sections of spin 0 and 1/2 and 1 particles by DWBA. Available at: https://inis.iaea.org/search/search.aspx?orig_q=RN.
Langanke, K., and Martínez-Pinedo, G. (2003). Nuclear weak-interaction processes in stars. Rev. Mod. Phys. 75, 819–862. doi:10.1103/RevModPhys.75.819
Larson, N. M. (2008). Updated users’ guide for SAMMY: Multilevel R-matrix fits to neutron data using Bayes’ equations. Available at: https://info.ornl.gov/sites/publications/files/Pub13056.pdf.
Lattimer, J. (2023). Lattimer-Swesty EOS web site. Available at: http://www.astro.sunysb.edu/dswesty/lseos.html.
Leeb, H., Dimitriou, P., and Thompson, I. (2023). R-matrix Codes for Charged-particle Reactions in the Resolved Resonance Region (4) https://www-nds.iaea.org/publications/indc/indc-nds-0767/.
Liang, J., Chen, A. A., Anger, M., Bishop, S., Faestermann, T., Fry, C., et al. (2020). Spectroscopic study of 39Ca for endpoint nucleosynthesis in classical novae. J. Phys. Conf. Ser. 1668, 012025. doi:10.1088/1742-6596/1668/1/012025
Lippuner, J., and Roberts, L. F. (2017). SkyNet: A modular nuclear reaction network library. Astrophys. J. Suppl. Ser. 233, 18. doi:10.3847/1538-4365/aa94cb
Litvinova, E., Robin, C., and Wibowo, H. (2020). Temperature dependence of nuclear spin-isospin response and beta decay in hot astrophysical environments. Phys. Lett. B 800, 135134. doi:10.1016/j.physletb.2019.135134
Longland, R., Iliadis, C., Champagne, A. E., Newton, J. R., Ugalde, C., Coc, A., et al. (2010). Charged-particle thermonuclear reaction rates: I. Monte Carlo method and statistical distributions. Nuc. Phys. A 841, 1–30. doi:10.1016/j.nuclphysa.2010.04.008
Lunney, D., Pearson, J. M., and Thibault, C. (2003). Recent trends in the determination of nuclear masses. Rev. Mod. Phys. 75, 1021–1082. doi:10.1103/RevModPhys.75.1021
Lynx X-ray Observatory Team (2023). Lynx X-ray observatory. Available at: https://www.lynxobservatory.com/.
Macklin, R. L., and Gibbons, J. H. (1965). Neutron capture data at stellar temperatures. Rev. Mod. Phys. 37, 166–176. doi:10.1103/revmodphys.37.166
MESA Collaboration (2023). Modules for experiments in stellar astrophysics (MESA). Available at: https://docs.mesastar.org/en/release-r22.05.1/,http://mesa.sourceforge.net/.
Meyer, B. S. (2023). Webnucleo. Available at: https://sourceforge.net/p/libnucnet/home/Home/.
Meyer, B. S. (2012). Webnucleo.org. Proc. XII international symposium on nuclei in the cosmos (Australia: Proceedings of Science), 096.
Möller, P. (1997). Astrophysical data for charged-particle reactions. Available at: https://t2.lanl.gov/nis/data/astro/molnix96/molnix.html.
Möller, P., Mumpower, M. R., Kawano, T., and Myers, W. D. (2019). Nuclear properties for astrophysical and radioactive-ion-beam applications (II). At. Data Nucl. Data Tables 125, 1–192. doi:10.1016/j.adt.2018.03.003
Möller, P., Nix, J., and Kratz, K. L. (1997). Nuclear properties for astrophysical and radioactive-ion-beam applications. At. Data Nucl. Data Tables 66, 131–343. doi:10.1006/adnd.1997.0746
Möller, P., Nix, J. R., Myers, W. D., and Swiatecki, W. J. (1995). Nuclear ground-state masses and deformations. At. Data Nucl. Data Tables 59, 185–381. doi:10.1006/adnd.1995.1002
Möller, P., Sierk, A. J., Ichikawa, T., and Sagawa, H. (2016). Nuclear ground-state masses and deformations: FRDM. At. Data Nucl. Data Tables 109-110, 1–204. doi:10.1016/j.adt.2015.10.002
Motobayashi, T., and Sakurai, H. (2012). Research with fast radioactive isotope beams at RIKEN. Prog. Theor. Exp. Phys. 2012. doi:10.1093/ptep/pts059
Mughabghab, S. F. (2023). Atlas of neutron resonances. Available at: https://www.nndc.bnl.gov/atlas/.
Mumpower, M. R., Sprouse, T. M., Kawano, T., Herman, M. W., Lovell, A. E., Misch, G. W., et al. (2022). Nuclear data activities for medium mass and heavy nuclei at Los Alamos. Available at: https://arxiv.org/abs/2210.12136 (ArXiv:2210.12136 (nucl-th).
Nagorcka, B., Symons, G., Zuk, W., and Patterson, J. (1971). Extrapolation of the 12C+12C and 12C+16O reaction cross sections to astrophysical energies. Nature 231, 17–18. doi:10.1038/physci231017a0
NASA. NASA (2023). Astrophysical data system. Available at: https://ui.adsabs.harvard.edu/.
Natarajan, P., Pacucci, F., Ferrara, A., Agarwal, B., Ricarte, A., Zackrisson, E., et al. (2017). Unveiling the first black holes with JWST: Multi-wavelength spectral predictions. Astrophys. J. 838, 117. doi:10.3847/1538-4357/aa6330
National Nuclear Data Center (2023a). Evaluated nuclear data file (ENDF). Available at: https://www.nndc.bnl.gov/endf/.
National Nuclear Data Center (2023b). Evaluated nuclear structure data file (ENSDF). Available at: https://www.nndc.bnl.gov/ensdf/.
National Nuclear Data Center (2023d). Maxwellian-averaged cross sections. Available at: https://www.nndc.bnl.gov/astro/calcmacs.jsp.
National Nuclear Data Center (2023c). Nudat (ENSDF). Available at: https://www.nndc.bnl.gov/nudat3/.
National Research Council (2003). Connecting quarks with the cosmos – eleven science questions for the new century. Available at: https://nap.nationalacademies.org/catalog/10079/connecting-quarks-with-the-cosmos-eleven-science-questions-for-the.
NEA OECD (2023). The generalised nuclear database structure: Establishing an international nuclear data standard. Available at: https://www.oecd-nea.org/jcms/pl_37782/the-generalised-nuclear-database-structure-establishing-an-international-nuclear-data-standard.
Nesaraja, C., Lingerfelt, E., Scott, J., Smith, M., Hix, W., Bardayan, D., et al. (2005a). A new computational infrastructure for nuclear astrophysics. Nucl. Phys. A 758, 174C–177C. doi:10.1016/j.nuclphysa.2005.05.173
Nesaraja, C., Smith, M., Bardayan, D., Blackmon, J., Chae, K., Guidry, M., et al. (2005b). New evaluations and computational infrastructure for management and visualization of nuclear astrophysics data. Int. Conf. Nucl. Data Sci. Tech. 769, 1378. doi:10.1016/j.nuclphysa.2005.05.173
Nesaraja, C. D., Shu, N., Bardayan, D. W., Blackmon, J. C., Chen, Y. S., Kozub, R. L., et al. (2007). Nuclear structure properties of astrophysical importance for 19Ne above the proton threshold energy. Phys. Rev. C 75, 055809. doi:10.1103/physrevc.75.055809
Ney, E. M., Engel, J., Li, T., and Schunck, N. (2023). Supplemental Material: Global description of β− decay with the axially deformed Skyrme finite-amplitude method: Extension to odd-mass and odd-odd nuclei. Available at: http://link.aps.org/supplemental/10.1103/PhysRevC.102.034326.
Ney, E. M., Engel, J., Li, T., and Schunck, N. (2020). Global description of β− decay with the axially deformed Skyrme finite-amplitude method: Extension to odd-mass and odd-odd nuclei. Phys. Rev. C 102, 034326. doi:10.1103/PhysRevC.102.034326
NNDC (2023a). Covariances in the ENDF/B-VII.1 evaluated nuclear data library. Available at: https://www.nndc.bnl.gov/endf-b7.1/covariances.html.
NNDC (2023b). The cross section evaluation working group. Available at: https://www.nndc.bnl.gov/csewg/.
NNDC (2023c). US nuclear data program. Available at: https://www.nndc.bnl.gov/usndp/.
Nuclear Energy Agency (2023). Joint evaluated fission and fusion (JEFF) file. Available at: https://www.nea.fr.html/.
Nuclear Science Advisory Committee (2015). Reaching for the horizon: The 2015 long range plan for nuclear science. Available at: https://science.osti.gov/-/media/np/nsac/pdf/2015LRP/2015_LRPNS_091815.pdf.
NUCLEI Collaboration (2023). Nuclei: Nuclear computational low energy initiative. Available at: https://nuclei.mps.ohio-state.edu/.
NUGRID Collaboration (2023). Nugrid. Available at: https://nugrid.github.io/content/codes_collab.html.
NUPECC (2017). NuPECC long range plan 2017 perspectives in nuclear physics. Available at: https://www.esf.org/fileadmin/user_upload/esf/Nupecc-LRP2017.pdf.
Oertel, M., Hempel, M., Klähn, T., and Typel, S. (2017). Equations of state for supernovae and compact stars. Rev. Mod. Phys. 89, 015007. doi:10.1103/RevModPhys.89.015007
Origins Space Telescope Study Team (2023a). ORIGINS space telescope. Available at: https://origins.ipac.caltech.edu/.
Origins Space Telescope Study Team (2023b). ORIGINS space telescope mission concept study report. Available at: https://origins.ipac.caltech.edu/download/MediaFile/174/original.
ORNL (2023). Exascale astrophysics: simulating a more detailed universe with frontier supercomputer. Available at: https://scitechdaily.com/exascale-astrophysics-simulating-a-more-detailed-universe-with-frontier-supercomputer/.
Oxford Academic (2023). Monthly Notices of the royal astronomical society (MNRAS). Available at: https://academic.oup.com/mnras.
Paxton, B., Bildsten, L., Dotter, A., Herwig, F., Lesaffre, P., and Timmes, F. (2011). Modules for experiments in stellar astrophysics (MESA). Astrophys. J. Suppl. Ser. 192, 3. doi:10.1088/0067-0049/192/1/3
Pepper, B. T., Istrate, A. G., Romero, A. D., and Kepler, S. O. (2022). The impact of the uncertainties in the 12C(α, γ)16O reaction rate on the evolution of low-to intermediate-mass stars. Mon. Not. Roy. Astron. Soc. 513, 1499–1512. doi:10.1093/mnras/stac1016
Petrovici, A., Mare, A. S., Andrei, O., and Meyer, B. S. (2019). Impact of 68Se and 72Kr stellar weak interaction rates on rp-process nucleosynthesis and energetics. Phys. Rev. C 100, 015810. doi:10.1103/physrevc.100.015810
Politano, M., Starrfield, S., Truran, J. W., Weiss, A., and Sparks, W. M. (1995). Hydrodynamic studies of accretion onto massive white dwarfs: ONeMg-Enriched nova outbursts. I. Dependence on white dwarf mass. Astrophys. J. 448, 807. doi:10.1086/176009
Poludnenko, A. Y., Chambers, J., Ahmed, K., Gamezo, V. N., and Taylor, B. D. (2019). A unified mechanism for unconfined deflagration-to-detonation transition in terrestrial chemical systems and type Ia supernovae. Science 366, eaau7365. doi:10.1126/science.aau7365
Potekhin, M. (2023). Data and analysis preservation in the PHENIX experiment at RHIC. J. Phys. Conf. Ser. 2438 012020. doi:10.1088/1742-6596/2438/1/012020
Pritychenko, B., Běták, E., Kellett, M., Singh, B., and Totans, J. (2011). The nuclear science references (NSR) database and web retrieval system. Nucl. Inst. Meth. A 640, 213–218. doi:10.1016/j.nima.2011.03.018
Pritychenko, B., and Mughaghab, S. F. (2012). Neutron thermal cross sections, westcott factors, resonance integrals, Maxwellian averaged cross sections and astrophysical reaction rates calculated from the ENDF/B-VII.1, JEFF-3.1.2, JENDL-4.0, ROSFOND-2010, CENDL-3.1 and EAF-2010 evaluated data libraries. At. Data Nucl. Data Tables 113, 3120–3144. doi:10.1016/j.nds.2012.11.007
Pritychenko, B., Mughaghab, S. F., and Sonzogni, A. A. (2010). Calculations of Maxwellian-averaged cross sections and astrophysical reaction rates using the ENDF/B-VII.0, JEFF-3.1, JENDL-3.3, and ENDF/B-VI.8 evaluated nuclear reaction data libraries. At. Data Nucl. Data Tables 96, 645–748. doi:10.1016/j.adt.2010.05.002
Pritychenko, B. (2020). The use of the ENDF library for nucleosynthesis studies. EPJ Web Conf 239, 07002. doi:10.1051/epjconf/202023907002
Rauscher, T. (2023a). Nonsmoker. Available at: https://nucastro.org/nonsmoker.html.
Rauscher, T. (2023b). Nuclear astrophysics results provided by Thomas Rauscher. Available at: https://nucastro.org/.
Rauscher, T., and Thielemann, F. K. (2000). Astrophysical reaction rates from statistical model calculations. At. Data Nucl. Data Tables 75, 1–351. doi:10.1006/adnd.2000.0834
Rauscher, T., and Thielemann, F. K. (2001). Tables of nuclear cross sections and reaction rates: An addendum to the paper “astrophysical reaction rates from statistical model calculations”. At. Data Nucl. Data Tables 79, 47–64. doi:10.1006/adnd.2001.0863
Sallaska, A. L., Iliadis, C., Champagne, A. E., Goriely, S., Starrfield, S., and Timmes, F. X. (2013). Starlib: A next-generation reaction-rate library for nuclear astrophysics. Astrophys. J. Suppl. Ser. 207, 18. doi:10.1088/0067-0049/207/1/18
Sandoval, M. A., Hix, W. R., Messer, O. E. B., Lentz, E. J., and Harris, J. A. (2021). Three-dimensional core-collapse supernova simulations with 160 isotopic species evolved to shock breakout. Astrophys. J. 921, 113. doi:10.3847/1538-4357/ac1d49
Schatz, H., Aprahamian, A., Goerres, J., Wiescher, M., Rauscher, T., Rembges, J. F., et al. (1998). Rp-process nucleosynthesis at extreme temperature and density conditions. Phys. Rep. 294, 167–263. doi:10.1016/s0370-1573(97)00048-3
Schatz, H., Reyes, A. D. B., Best, A., Brown, E. F., Chatziioannou, K., Chipps, K. A., et al. (2022). Horizons: Nuclear astrophysics in the 2020s and beyond. J. Phys. G. 49, 110502. doi:10.1088/1361-6471/ac8890
Scheidenberge, C. (2017). NUSTAR experiments on the way from GSI to FAIR. Eurasian J. Phys. Funct. Matl. 1, 19. doi:10.29317/ejpfm.2017010103
Schnabel, G., Sjöstrand, H., Hansson, J., Rochman, D., Koning, A., and Capote, R. (2021). Conception and software implementation of a nuclear data evaluation pipeline. Nucl. Data Sheets 173, 239–284. doi:10.1016/j.nds.2021.04.007
Schunck, N., and Robledo, L. M. (2016). Microscopic theory of nuclear fission: A review. Rep. Prog. Phys. 79, 116301. doi:10.1088/0034-4885/79/11/116301
Shelley, M., and Pastore, A. (2021). A new mass model for nuclear astrophysics: Crossing 200 keV accuracy. Universe 7, 131. doi:10.3390/universe7050131
Shen, H. (2023b). Equation of state. Available at: http://www.rcnp.osaka-u.ac.jp/∼shen/.
Shen, H. (2023a). Home page of relativistic EOS table for supernovae. Available at: http://user.numazu-ct.ac.jp/∼sumi/eos/index.html.
Shen, H., Ji, F., Hu, J., and Sumiyoshi, K. (2020). Effects of symmetry energy on the equation of state for simulations of core-collapse supernovae and neutron-star mergers. Astrophys. J. 891, 148. doi:10.3847/1538-4357/ab72fd
Shen, H. (2014). Relativistic EOS for supernova simulations. Eur. Phys. J. Web Conf. 66, 07023. doi:10.1051/epjconf/20146607023
Sieverding, A., Martínez-Pinedo, G., Huther, L., Langanke, K., and Heger, A. (2018). The ν-process in the light of an improved understanding of supernova neutrino spectra. Astrophys. J. 865, 143. doi:10.3847/1538-4357/aadd48
Smith, A. I., Johnson, E. T., Chen, Z., Eiden, K., Willcox, D. E., Boyd, B., et al. (2023c). Pynucastro: A Python library for nuclear astrophysics. Astrophys. J. 947, 65. doi:10.3847/1538-4357/acbaff
Smith, D. L. (2011b). Evaluated nuclear data covariances: The journey from ENDF/B-VII.0 to ENDF/B-VII.1. Nucl. Data Sheets 112, 3037–3053. doi:10.1016/j.nds.2011.11.004
Smith, M., Ma, Z., Cain, B. H., and Gupta, M. (2023b). Caughlan and Fowler 1988 thermonuclear reaction rates. Available at: http://www.nuclear.csdb.cn/data/CF88/.
Smith, M. (2003). Nuclear data for astrophysics. Nucl. Phys. A 718, 339C–346C. doi:10.1016/s0375-9474(03)00736-x
Smith, M. S., Lingerfelt, E. J., Kim, B., Nesaraja, C. D., Kondev, F. G., and Koura, H. (2023a). nuclearmasses.org. Available at: http://nuclearmasses.org.
Smith, Michael S. (2023). Computational infrastructure for nuclear astrophysics (CINA). Available at: https://nucastrodata.org/infrastructure/.
Smith, M. S. (2005). Future of nuclear data for nuclear astrophysics. Int. Conf. Nucl. Data Sci. Tech. 769, 1331. doi:10.1063/1.1945251
Smith, M. S., Kawano, L. H., and Malaney, R. A. (1993). Experimental, computational, and observational analysis of primordial nucleosynthesis. Astrophys. J. Suppl. Ser. 85, 219. doi:10.1086/191763
Smith, M. S., Lingerfelt, E. J., Nesaraja, C. D., Hix, W. R., Roberts, L. F., Koura, H., et al. (2008). Nuclear data for astrophysics: Resources, challenges, strategies, and software solutions. Proc. Int. Conf. Nucl. Data Sci. Tech. 2, 1319. doi:10.1051/ndata:07770
Smith, M. S., Lingerfelt, E. J., Scott, J. P., Nesaraja, C. D., Hix, W. R., Chae, K., et al. (2006). Computational infrastructure for nuclear astrophysics. Proc. Orig. Matter Evol. Galaxies AIP Conf. Proc. 847, 470.
Smith, M. S. (2011a). Nuclear data for astrophysics research: A new online paradigm. J. Korean Phys. Soc. 59, 761–766. doi:10.3938/jkps.59.761
Smith, M. S., and Rehm, K. E. (2001). Nuclear astrophysics measurements with radioactive beams. Ann. Rev. Nucl. Part. Sci. 51, 91–130. doi:10.1146/annurev.nucl.51.101701.132430
Sprouse, T. M., Mumpower, M. R., and Surman, R. (2020). Following fission products in explosive astrophysical environments. EPJ Web Conf 242, 04001. doi:10.1051/epjconf/202024204001
Sprouse, T. M., Mumpower, M. R., and Surman, R. (2021). Following nuclei through nucleosynthesis: A novel tracing technique. Phys. Rev. C 104, 015803. doi:10.1103/physrevc.104.015803
Starrfield, S., Iliadis, C., Truran, J. W., Wiescher, M., and Sparks, W. M. (2001). Nuclear reaction rates and the nova outburst. Nuc. Phys. A 688, 110–117. doi:10.1016/s0375-9474(01)00679-0
Starrfield, S., Truran, J. W., Wiescher, M. C., and Sparks, W. M. (1998). Evolutionary sequences for Nova V1974 Cygni using new nuclear reaction rates and opacities. Mon. Not. Roy. Astron. Soc. 296, 502–522. doi:10.1046/j.1365-8711.1998.01312.x
Sumiyoshi, K., Kojo, T., and Furusawa, S. (2020). Equation of state in neutron stars and supernovae. Springer Nature Singapore, 1–51. doi:10.1007/978-981-15-8818-1_104-1
Suzuki, I., Shimizu, N., Tsunoda, Y., Otsuka, T., and Nomoto, K. (2023). Shell-model study of nuclear weak rates relevant to astrophysical processes in stars. J. Phys. Conf. Ser. 2453, 012003. doi:10.1088/1742-6596/2453/1/012003
Suzuki, T. (2022). Nuclear weak rates and nuclear weak processes in stars. Prog. Part. Nucl. Phys. 126, 103974. doi:10.1016/j.ppnp.2022.103974
Szucs, T., Dillman, I., Plan, R., and Fulop, Z. (2014). KADoNIS-p: The astrophysical p-process databas. Nucl. Data Sheets 120, 191–193. doi:10.1016/j.nds.2014.07.043
Takahashi, K., and Yokoi, K. (1987). Beta-decay rates of highly ionized heavy atoms in stellar interiors. At. Data Nucl. Data Tables 36, 375–409. doi:10.1016/0092-640X(87)90010-6
Thielemann, F. K., Arcones, A., Käppeli, R., Liebendörfer, M., Rauscher, T., Winteler, C., et al. (2011). What are the astrophysical sites for the r-process and the production of heavy elements? Prog. Part. Nucl. Phys. 66, 346–353. doi:10.1016/j.ppnp.2011.01.032
Thielemann, F. K., Arnould, M., and Truran, J. (1987). Thermonuclear reactions at high temperatures and densities. Adv. Nucl. Astrophys. 688, 525.
Thompson, I., and Nunes, F. (2009). Nuclear reactions for astrophysics. Cambridge, UK: Cambridge Univ. Press.
Timmes, F. X. (2023). Softwired reaction network. Available at: https://cococubed.com/code_pages/net_torch.shtml.
Timmes, F. X. (1999). Integration of nuclear reaction networks for stellar hydrodynamics. Astrophys. J. Suppl. Ser. 124, 241–263. doi:10.1086/313257
van Wormer, L., Görres, J., Iliadis, C., Wiescher, M., and Thielemann, F. K. (1994). Reaction rates and reaction sequences in the rp-process. Astrophys. J. 432, 326. doi:10.1086/174572
Wagoner, R. V., Fowler, W. A., and Hoyle, F. (1967). On the synthesis of elements at very high temperatures. Astrophys. J. 148, 3. doi:10.1086/149126
Wagoner, R. V. (1969). Synthesis of the elements within objects exploding from very high temperatures. Astrophys. J. Suppl. Ser. 18, 247. doi:10.1086/190191
Walker, T. P., Steigman, G., Schramm, D. N., Olive, K. A., and Kang, H. S. (1991). Primordial nucleosynthesis redux. Astrophys. J. 376, 51. doi:10.1086/170255
Wanajo, S., Hirai, Y., and Prantzos, N. (2021). Neutron star mergers as the astrophysical site of the r-process in the Milky Way and its satellite galaxies. Mon. Not. Roy. Astron. Soc. 505, 5862–5883. doi:10.1093/mnras/stab1655
Wang, M., Huang, W. J., Kondev, F. G., Audi, G., and Naimi, S. (2021). The AME 2020 atomic mass evaluation (II). Tables, graphs and references. Chin. Phys. C 45, 030003. doi:10.1088/1674-1137/abddaf
Wapstra, A. H., Audi, G., and Thibault, C. (2003). The AME2003 atomic mass evaluation: (I). Evaluation of input data, adjustment procedures. Nucl. Phys. A 729, 129–336. doi:10.1016/j.nuclphysa.2003.11.002
Wei, J., Ao, H., Beher, S., Bultman, N., Casagrande, F., Cogan, S., et al. (2019). Advances of the FRIB project. Int. J. Mod. Phys. E 28, 1930003. doi:10.1142/s0218301319300030
Wilkinson, M. D., Dumontier, M., Aalbersberg, I. J., Appleton, G., Axton, M., Baak, A., et al. (2016). The FAIR Guiding Principles for scientific data management and stewardship. Sci. Data 3, 160018. doi:10.1038/sdata.2016.18
Williams, L. (2011). Disrupt: Think the unthinkable to spark transformation in your business. Chicago: Pearson.
Winteler, C. (2013). Light element production in the Big Bang and the synthesis of heavy elements in 3D MHD jets from core-collapse supernovae. Basel. Ph.D. Thesis, Univ. Available at: https://edoc.unibas.ch/29895/1/thesis_winteler.pdf.
Woosley, S., Fowler, W. A., Holmes, J., and Zimmerman, B. (1978). Semiempirical thermonuclear reaction-rate data for intermediate-mass nuclei. At. Data Nucl. Data Tables 22, 371–441. doi:10.1016/0092-640X(78)90018-9
Xu, Y., Goriely, S., Jorissen, A., Chen, G., and Arnould, M. (2013b). Databases and tools for nuclear astrophysics applications. Astron. Astrophys. 549, A106. doi:10.1051/0004-6361/201220537
Xu, Y., Takahashi, K., Goriely, S., Arnould, M., Ohta, M., and Utsunomiya, H. (2013a). NACRE II: An update of the NACRE compilation of charged-particle-induced thermonuclear reaction rates for nuclei with mass number A≤ 16. Nuc. Phys. A 918, 61–169. doi:10.1016/j.nuclphysa.2013.09.007
Yamazaki, Y., He, Z., Kajino, T., Mathews, G. J., Famiano, M. A., Tang, X., et al. (2022). Possibility to identify the contributions from collapsars, supernovae, and neutron star mergers from the evolution of the r-process mass abundance distribution. Astrophys. J. 933, 112. doi:10.3847/1538-4357/ac721c
Young, P. G., Arthur, E. D., and Chadwick, M. B. (1992). Comprehensive nuclear model calculations: Introduction to the theory and use of the GNASH code. Available at: https://inis.iaea.org/collection/NCLCollectionStore/_Public/24/013/24013108.pdf.
Zerkin, V. V., and Pritychenko, B. (2018). The experimental nuclear reaction data (EXFOR): Extended computer database and Web retrieval system. Nucl. Inst. Meth. A 888, 31–43. doi:10.1016/j.nima.2018.01.045
Zhang, L. Y., He, J. J., Kusakabe, M., He, Z. Y., and Kajino, T. (2022). Thermonuclear 17O(n,γ)18O reaction rate and its astrophysical implications. Astrophys. J. 927, 92. doi:10.3847/1538-4357/ac4697
Keywords: nuclear astrophysics, nuclear data, nuclear reactions, nuclear structure, evaluations, cross section, software, simulations
Citation: Smith MS (2023) Nuclear data resources and initiatives for nuclear astrophysics. Front. Astron. Space Sci. 10:1243615. doi: 10.3389/fspas.2023.1243615
Received: 21 June 2023; Accepted: 17 July 2023;
Published: 10 November 2023.
Edited by:
Yi Xu, Horia Hulubei National Institute for Research and Development in Physics and Nuclear Engineering (IFIN-HH), RomaniaReviewed by:
Zilong Chang, Indiana University, United StatesAntonio Caciolli, University of Padua, Italy
Copyright © 2023 Smith. This is an open-access article distributed under the terms of the Creative Commons Attribution License (CC BY). The use, distribution or reproduction in other forums is permitted, provided the original author(s) and the copyright owner(s) are credited and that the original publication in this journal is cited, in accordance with accepted academic practice. No use, distribution or reproduction is permitted which does not comply with these terms.
*Correspondence: Michael S. Smith, c21pdGhtc0Bvcm5sLmdvdg==