- 1Geomicrobiology, Center for Applied Geoscience, University of Tübingen, Tübingen, Germany
- 2Centro de Biología Molecular Severo Ochoa (CSIC-UAM), Universidad Autónoma de Madrid, Torrejón de Ardoz, Spain
- 3Planetology and Habitability Department, Centro de Astrobiología (CAB), INTA-CSIC, Torrejón de Ardoz, Spain
The discovery that most of the prokaryotic diversity and biomass on Earth resides in the deep subsurface, calls for an improved definition of habitability, which should consider the existence of dark biospheres in other planets and moons of the Solar System and beyond. The discovery of “interior liquid water worlds” on some ice moons with waterless surfaces has piqued wide astrobiological interest, but the sporadic mentions of the possibility of life in the deep subsurface of rocky planets in recent habitability reviews calls for a methodical effort to develop sufficient knowledge, both scientific and technological, to include the dark biospheres in our habitability assessments. In this review we analyze recent developments and the methodologies employed to characterize Earth’s continental hard rock deep subsurface to both prepare the future exploration of the putative dark biosphere of Mars and to highlight its importance when evaluating planetary habitability.
Habitability
Habitability is defined as the potential of a given planetary environment to sustain life (Cockell et al., 2016 and references therein). Obviously, this concept depends on the conditions required for life to develop, among them energy, liquid solvent to facilitate mass transfer reactions and temperatures compatible with cell functions and the liquid state of the solvent (Kasting and Catling, 2003; Nisbet et al., 2007; Lammer et al., 2009; Cockell, 2014; Cockell, 2020; Lignam and Loeb, 2020; Lignam and Loeb, 2021). In addition, access to the elements required for the synthesis of cellular components is required. In the only reference system that we have, these are: carbon (C), hydrogen (H), oxygen (O), nitrogen (N), phosphorous (P) and sulfur (S). These conditions are determined by astronomical factors such as star type, planetary rotational and orbital characteristics, presence of moons, impact events and planetary factors such as mass, atmospheric and surface characteristics, plate tectonics and magnetic fields (Cockell et al., 2016; Cockell, 2020; Lingam and Loeb, 2021). Astrophysicists long ago defined the concept of planetary habitability zone (HZ) as the annular area around a main sequence star in which rocky planets with an Earth like mass can harbor liquid water in its surface (Maunder, 1913; Huang, 1959; Dole, 1964; Hart, 1979; Kasting et al., 1993). Among the high numbers of planets detected outside the Solar System (exoplanets) up to now, there is a significant proportion that meets these habitability conditions (https://exoplanets.nasa.gov/what-is-an-exoplanet/planet-types/terrestrial/). The discovery of extremophilic organisms able to thrive in extreme environmental conditions of temperature, pH, ionic concentration, water activity, pressure, radiation, etc… has increased the probability of finding life in conditions that 50 years ago were unimaginable (Merino et al., 2019).
The astrobiological interest of habitability has been recently reviewed by several researchers (Cockell et al., 2016; Méndez et al., 2021). Cockell and collaborators constrained their habitability concept to known life to avoid the problem of defining life and because our ignorance of whether terrestrial life is universal. They distinguish between surface liquid water worlds and interior liquid water worlds, such as icy moons and terrestrial-type rocky planets where liquid water is only in their subsurface. This is the first mention on a habitability review of a possible internal source of energy generating liquid water in the interior of a planetary body. The focus of Méndez and collaborators is on Habitability Models for Astrobiology. In their review they recognize that “identifying water in the atmosphere of planets (in addition to other biosignature-relevant gases) is the only way to narrow down potential life-hosting targets, since subsurface life deep in the interior may not be able to modify the atmospheres of planets enough to be detectable remotely”, pointing out the limitations of habitability exploration of rocky exoplanets (Méndez et al., 2021). Furthermore, the sentence quoted above is the only reference to deep subsurface life in the entire review. Interestingly, due to the experience of some of the authors in deep subsurface research, McMahon and collaborators elaborated the term “subsurface-habitability zone” (SSHZ) in 2013, acknowledging the proven existence of life in the deep subsurface of Earth as well as the possibility of interior liquid water on other planets depending on distance from the star and internal planetary heat (McMahon et al., 2013; MacMahon and Parnell, 2014). Despite its importance, this concept has not received the attention it deserved, most likely because research on hard rock deep subsurface is still very limited, and an in-depth discussion of the concepts required to analyze the habitability of a planet remains to be seen.
Although it is recognized the importance of the very prolific deep subsurface ocean research in the development of the dark biosphere concept, in this work only results from the deep oceanic crust are considered, because most of the work performed in the deep subsurface ocean has been done in sediments, requiring the presence of liquid water on the surface of the rocky planet, which was not the aim of the review. Furthermore, even if it is recognized the interest of the subsurface oceans detected in some ice moons from the Solar System, they are not considered also because do not fit in the hard rock deep subsurface concept, subject of the work. In this review we analyze the recent developments in characterizing the Earth continental hard rock deep subsurface, the methodologies used for its study as preparation for the exploration of the deep subsurface of Mars and to emphasize the need to include the dark biospheres in our habitability assessments.
The Earth hard rock dark biosphere
It took almost two hundred years to demonstrate Darwin’s prediction of existence of life in the deep subsurface (Darwin, 1839). Although some pioneering observations were made at the beginning of the last century by taking advantage of samples obtained from oil drilling operations (Bastin et al., 1926), the consensus was that the detected microorganisms were contaminants resulting from the drilling operation. This is one of the biggest problems in this area of research (Lipman, 1931). The situation changed radically after the discovery of life associated to submarine hydrothermal vents (Corliss et al., 1979), which opened a very fruitful era of oceanic drilling projects searching for life in marine sediments and in the deep oceanic crust (Whelan et al., 1986; D´Hondt et al., 2007; Edwards et al., 2012a; Edwards et al., 2012b; Kallmeyer et al., 2012; Inagaki et al., 2015; Sueoka et al., 2019; Li et al., 2020), although the pioneering work of Morita and Zobell many years before should be underlined (Morita and Zobell, 1955). The methodologies developed to control the different sources of contamination during drilling was a crucial advance and dissipated skepticism around the study of the dark biosphere (Kieft, 2010). In 1992 Tomas Gold speculated, in his seminal paper, on the existence of life in the subsurface independent of radiation and the possibility of its presence in other planets (Gold, 1992). Since then, several studies have proved the existence of a great microbial diversity in the continental deep subsurface (also known as terrestrial deep subsurface) (Stevens and McKinley, 1995; Chapelle et al., 2002; Zhang et al., 2005; Suzuki et al., 2013; Wu et al., 2016; Momper et al., 2017b; Purkamo et al., 2018; Sherwood Lollar et al., 2019; Nuppunen-Puputti et al., 2022; Soares et al., 2023). “Life Underground” funded by the NASA Astrobiology Institute (NAI) and the “Center for Dark Energy Biosphere” funded by the National Science Foundation have been two interesting initiatives to foster deep subsurface research. Recent evaluations assert that most of the prokaryotic biomass and diversity on this planet is, in fact, within the deep subsurface (Colman et al., 2017; Bar-On et al., 2018; Magnabosco et al., 2018). Thus, incorporating dark biospheres into our habitability assessments should be a high priority.
Despite the important advances made in the methodologies to recover and analyze samples from the continental deep subsurface, the number of studies using pristine core samples from devoted drilling operations is still limited, mainly due to economic and technical limitations (Zhang et al., 2005; Fernández-Remolar et al., 2008; Gronstal et al., 2009; Wu et al., 2016; Amils et al., 2023; Templeton and Caro, 2023). For this reason, many researchers have taken advantage of samples obtained from different “subsurface windows”, such as artesian wells, springs, radioactive waste disposal and underground research facilities, or deep mining operations. Most of them rely on groundwater, as it is much easier to collect and analyze, generating useful microbial diversity information. Nevertheless, these studies lack information on the relationship of microorganisms with the mineral components of the complex subsurface solid matrix in which they develop and on the interconnectivity required to understand the critical operation of deep subsurface biogeochemical cycles, essential to their successful performance (Stevens and McKinley, 1995; Pedersen, 1999; Chapelle et al., 2002; Murakami et al., 2002; Onstott et al., 2003; Sahl et al., 2008; Suzuki et al., 2013; Magnabosco et al., 2014; Momper et al., 2017a; Purkamo et al., 2018; Sherwood Lollar et al., 2019; Nuppunen-Puputti et al., 2022).
Unfortunately, the exploration of the deep subsurface biosphere in igneous ocean crust is very recent, mainly due to technical difficulties, thus very little microbiological information has been generated from the few studied areas (Edwards et al., 2012a; Li et al., 2020), although preliminary results showed some general trends already observed in the hard rock continental deep subsurface (Li et al., 2020; Quemener et al., 2020).
Microbial cell density and diversity
The microbial cell number for the dark biosphere detected using groundwater samples from continental subsurface varies between 102 and 107 cells/mL (Pedersen, 2000; Basso et al., 2009; Itävaara et al., 2011). These values are much lower, up to 105 cells/gr, if solid, low porosity rocks with variable mineralogical content are considered (Onstott et al., 2003; Zhang et al., 2005; Fry et al., 2009; Breuker et al., 2011; Cockell et al., 2012; Dutta et al., 2018; Cockell et al., 2021; Amils et al., 2023). In the case of the oceanic crust the cellular density seems to be even lower, up to 103 cells/mL (Li et al., 2020). Considering these numbers and the suggestion that life can develop to a depth of 5–10 km, being temperature the most important limiting factor (Gold, 1992), we must conclude that the percentage of microbial life in the dark biosphere is considerable (Whitman et al., 1998; Kallmeyer et al., 2012; Bar-On et al., 2018; Magnabosco et al., 2018). Consequently, an important challenge is to identify the sources of energy that maintain this biomass.
Even though there is great variability of microbial populations identified in the continental deep subsurface, mainly due to the geological characteristics of the drilling locations and the methodologies used for its analysis, some general conclusions can be extracted from what we know so far. 1) The number of microorganisms decreases with depth (Moser et al., 2005; Itävaara et al., 2011; Cockell et al., 2012; McMahon and Parnell, 2014), although in some drilling operations this decrease is not observed (Amils et al., 2023). 2) Microbial diversity, in general, tends to decrease also with depth (Zhang et al., 2005; Lin et al., 2006; Chivian et al., 2008), although some exceptions have been reported (Itävaara et al., 2011; Amils et al., 2023). 3) Abundance and diversity of Bacteria is much higher than Archaea (Takai et al., 2001; Cockell et al., 2012; Ino et al., 2016; Lau et al., 2016; Rempfert et al., 2017; Amils et al., 2023). 4) Pseudomonadota, Actinomycetota and Bacillota have been described as the most common phyla in the continental deep subsurface (Zhang et al., 2005; Fry et al., 2009; Onstott et al., 2009; Magnabosco et al., 2016; Wu et al., 2016; Nuppunen-Puputti et al., 2022; Amils et al., 2023; Soares et al., 2023), although members of other phyla (Nitrospirota; Chloroflexota, Acidobacteriota and Deinococcota) and candidate phyla have been also reported. 5) New groups of unknown microorganisms have also been discovered (Takai et al., 2001; Gihring et al., 2006; Sahl et al., 2008).
Viruses have also been detected (Kyle et al., 2008; Eydal et al., 2009; Lau et al., 2014; Nyyssönen et al., 2014; Labonté et al., 2015; Rahlf et al., 2021), but regrettably, the information on the subsurface viral community is still very scarce impeding a clear vision of the role they play in the ecosystem. Fungi and nematodes are the only eukaryotes identified as members of the continental subsurface community, although the latter has only been identified in water samples from a subterranean mine (Borgonie et al., 2011). The fungal community, on the other hand, has been detected on multiple occasions in hard rock samples from different locations (Pedersen, 1997; Purkamo et al., 2013; Sohlberg et al., 2015; Ivarson et al., 2018) and recently in deep ocean crust (Quemener et al., 2020). Despite representing a very small fraction of the subsurface community and the little information currently available, they could play a very important role in the dissolution of recalcitrant organic matter and minerals, thus actively contributing to the energy input into the ecosystem, as have been suggested in marine sediments and in the deep ocean crust (Quemener et al., 2020; Reese et al., 2021).
Sources of energy in the continental dark biosphere
Four are the basic requirements for a habitable continental deep subsurface environment: energy, liquid water, adequate temperature and bioessential elements (Hoehler, 2004). The hard rock deep subsurface is characterized by the lack of solar radiation, in general, absence of O2, and an increase in temperature and pressure with depth (Kieft, 2016). Obviously, the geological characteristics of the substrate: mineral composition, porosity, flow of water, presence of faults, will have an important influence in the development of microorganisms in the deep subsurface (Fredrickson et al., 1997; Pedersen, 2000; Amend et al., 2011; Li et al., 2020; Templeton and Caro, 2023).
Some authors maintain that most microorganisms from the continental deep subsurface are in the state of anabiosis, which means that they are not metabolically active but in a state of dormancy, with minimal energy consumption, which agrees with the observation of fast development of metabolically active microorganisms in enrichment cultures (D’Hondt et al., 2002; Rajala et al., 2015; Leandro et al., 2018). Others, using methodologies like Fluorescence in situ Hybridization (FISH), which can detect microorganisms with a significant number of ribosomes (Hoshino et al., 2008), suggest that the deep subsurface microorganisms are metabolically active (Escudero et al., 2018). Obviously, these observations are dependent on the characteristics of the drilled substrate, the type of samples, and the methodologies used, which makes generalizing extremely difficult.
To the best of our knowledge, no one has been able to measure the activity of microorganisms in situ in the rock continental subsurface. Viable microorganisms have been detected after long periods of time isolated in subsurface (Vreeland et al., 1998; Orsi et al., 2021) and it has been theorized that they could only survive under these conditions if they used some energy to avoid DNA and protein damage (Morita, 1999). Some researchers have used amino acid racemization to evaluate microbial deep subsurface doubling times (Steen et al., 2013). Indeed, some studies have corroborated that the strategy of repairing damaged cellular structures seems to be favored over dormancy in subsurface environments (Johnson et al., 2007) and that carbon turnover, at least in ocean sediments and continental groundwater samples, can vary from 1 year to several decades (Jørgensen, 2011; Onstott et al., 2014; Becraft et al., 2021). Thus, the possibility of extremely low levels of growth, in other words, geological duplication times, must also be considered in hard rock continental environments (Phelps et al., 1994; Steen et al., 2013).
An extremely critical and debated issue in the study of continental deep subsurface ecology is whether the energy sources should be endogenous or compatible with the existence of products generated in the surface. Purists maintain that only microorganisms that develop in the absence of any photosynthetic product should be considered members of the continental deep subsurface (Stevens and McKinley, 1995; Nealson et al., 2005; Orcutt et al., 2011; Momper et al., 2017b). These environments have been named Subsurface Lithoautotrophic Microbial Ecosystems (SLiMEs). But the fact that chemoheterotrophic microorganisms, i.e., microorganisms that use organic carbon as an energy source, are detected in abundance in the subsurface and the biomass must be recycled to keep geobiological cycles operative, makes this a difficult conundrum. Knowing that O2 is rapidly consumed by aerobic and facultative microorganisms, we should consider the continental hard rock deep subsurface an anoxic environment, although exceptions may occur (Ruff et al., 2023), in which only anaerobic metabolisms prevail. Thus, anaerobic respiration is the main energy generation system, and due to the shortage of organic matter, minerals are the main source of electron donors and acceptors (Jones and Bennett, 2017; Rempfert et al., 2017; Amils et al., 2023; Templeton and Caro, 2023), although fermentation should also be considered an important recycling system (Sanz et al., 2021), as it facilitates the operation of the biogeochemical cycles (Amils et al., 2023). It is well established that different microorganisms can directly use minerals as electron donors and acceptors (Shock, 2009; El- Naggar et al., 2010) or dissolve minerals to release compounds to be used as energy source in chemolithotrophic metabolisms (Rogers et al., 1998; Edwards et al., 2000; Shelobolina et al., 2012; Vera et al., 2013; Dong et al., 2014; Osburn et al., 2014; Templeton and Caro, 2023).
Because H2 is one of the most abundant gases in the continental deep subsurface it has been considered an important source of energy for chemolithoautotrophic microorganisms (Stevens and McKinley, 1995; Pedersen, 1997). According to the model advanced by these authors H2 is the main driver of a system in which hydrogenotrophic methanogenesis and acetogenesis constitute the basis of the ecosystem (Figure 1), releasing methane (CH4) and acetate and generating the biomass to be used by heterotrophic microorganisms that, in turn, contribute to the maintenance of an active C cycle, which has been recently shown to be operating in the deep subsurface (Amils et al., 2023). Hydrogen could be produced abiotically including serpentinization and water radiolysis (Apps and van de Kamp, 1993; Stevens and McKinley, 1995; Lin et al., 2004; Sherwood-Lollar et al., 2007; Klein et al., 2020; Coskun et al., 2021; Leong et al., 2021; Templeton and Caro, 2023), but recently it has been shown that an important amount of H2 in the subsurface of the Iberian Pyrite Belt is biologically produced (Sanz et al., 2021). This factor should be included in the analysis of other subsurface ecosystems to increase our understanding of the H cycle in the deep subsurface.
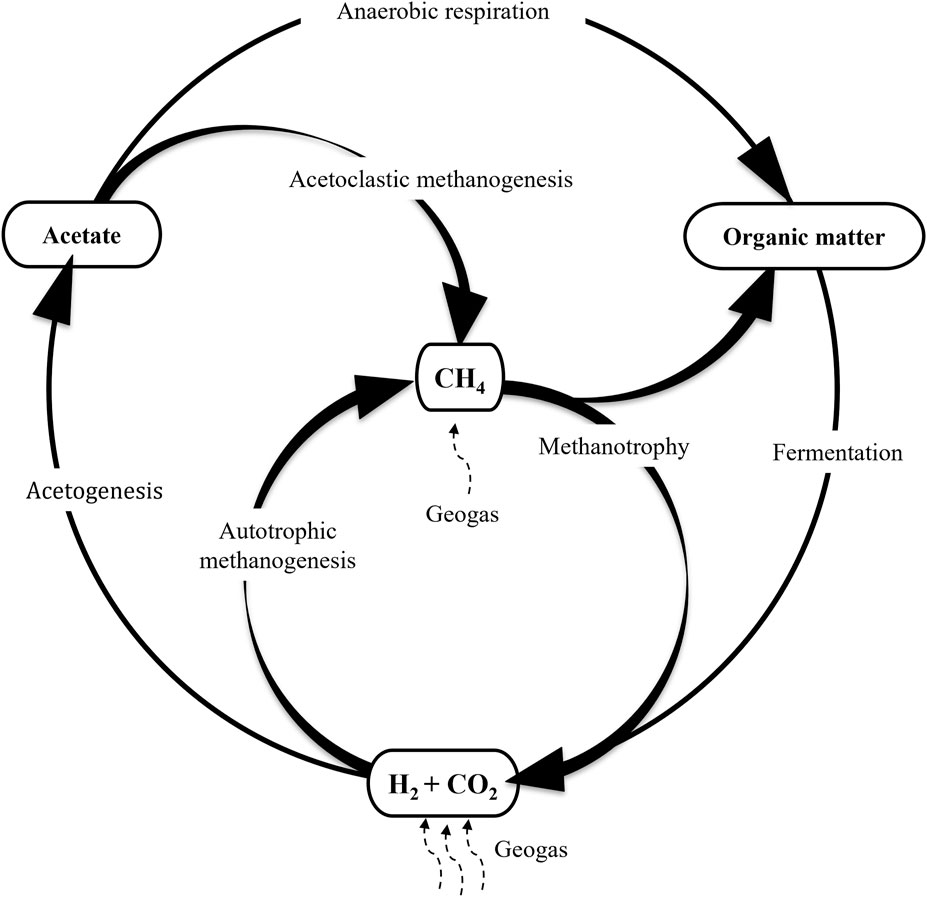
FIGURE 1. Proposed model in which H2 is the main energy source for primary productivity in the deep subsurface. Figure modified from Pedersen (1997).
To some researchers H2 is the most abundant source of energy in the deep subsurface which explains the continental dark biosphere’s independence from the surface (Chapelle et al., 2002; Nealson et al., 2005; Brazelton et al., 2012; Lau et al., 2016). H2, carbon dioxide (CO2) and CH4 have been reported in most of the drilling operations in which these gases have been measured, together with the identification of microorganisms able to use or produce them, which is a good back up for the suggested hypothesis (Pedersen, 2000; Moser et al., 2005; Itävaara et al., 2011; Wu et al., 2017; Amils et al., 2023). Nevertheless, other authors disagree with this view, claiming that in the deep subsurface, at least in part, there is a source of organic matter from the surface, such as petroleum deposits and sedimentary rocks (Fredrickson and Balkwill, 2006), in addition to the possibility of percolating water containing organic matter through pores and fractures of the rock. As mentioned earlier, the debate between defendants of each view is difficult to solve with the information available at the moment and the huge diversity of ecosystems studied. Obviously, this will not be a problem in the evaluation of habitability in other planetary systems.
Hydrogenotrophic methanogenesis was the first archaeal metabolic activity detected in the continental deep subsurface (Pedersen and Albinsson, 1992) and has been repeatedly identified in most drilling operations, directly, by detecting its metabolic product (CH4) and/or the gases involved in its synthesis (H2 and CO2) or indirectly, by identifying the microorganisms responsible (Moser et al., 2005; Probst et al., 2014; Puente-Sánchez et al., 2014; Purkamo et al., 2015; Rempfert et al., 2017; Amils et al., 2023). Interestingly, in many drilling operations Cyanobacteria have been frequently identified (Onstott et al., 2003; Zhang et al., 2005; Bomberg et al., 2014; Purkamo et al., 2015; Ino et al., 2017; Rempfert et al., 2017; Puente-Sánchez et al., 2018; Amils et al., 2023), so today it is well established that members of this phyla, which obviously cannot make use of solar radiation as a source of energy, can develop in the deep subsurface using alternative sources of energy, such as H2 (Puente-Sánchez et al., 2018).
In addition to H2 and reduced organic matter in the continental deep subsurface, chemolithoautotrophic microorganisms can use CO2 as carbon source and other energy sources such as reduced sulfur compounds (Amend and Teske, 2005; Gihring et al., 2006; Amend et al., 2011; Lau et al., 2016; Amils et al., 2023), iron (Sahl et al., 2008; Swanner et al., 2011; Shelobolina et al., 2012; Amils et al., 2023), nitrogen (Swanner et al., 2011; Nyyssönen et al., 2014; Lau et al., 2016; Amils et al., 2023), arsenic (Zhang et al., 2005; Sahl et al., 2008), manganese (Moser et al., 2005), or methane (Nyyssönen et al., 2012; Lau et al., 2016; Ino et al., 2017; Amils et al., 2023).
Methodologies currently used in the hard rock continental subsurface characterization
Several excellent reviews have been published covering the methodologies recommended for controlling the unavoidable contamination during drilling (Kieft, 2010; Wilkins et al., 2014). In this section we will concentrate on the methodologies that we consider fundamental for a full characterization of the operation of the hard rock continental deep subsurface. As mentioned, the deep subsurface is anoxic, thus it is extremely important to avoid any exposure of the drilled cores to atmospheric oxygen during the generation of the samples (Kieft, 2010). For this reason, after extraction, cores should be immediately placed in plastic bags and O2 displaced with N2. It is advisable to have access to an anaerobic glove box near the drilling site, so that powdered samples from the interior of the cores, using a rotary hammer drill, under sterile and temperature-controlled conditions can be generated and processed as soon as possible (Puente-Sánchez et al., 2018). This requirement will not be necessary for the characterization of Mars subsurface.
Most geomicrobiological subsurface information can be generated using different conventional analytical methodologies (for a recent description of most of these methodologies in hard rock deep subsurface drilling see Amils et al., 2023): Inductively Coupled Plasma Mass Spectrometry (ICP-MS) and Total Reflection X-Ray Fluorescence (TXRF) for elemental analysis of the solid substrate and the bioavailable elements; X-Ray Diffraction (XRD) for the mineral composition of the substrate; Scanning Electron Microscopy (SEM) for the visualization of mineral-microbe interactions; Confocal Raman Spectroscopy to evaluate mineral-microbe interaction (Escudero et al., 2020); Isotope Ratio Mass Spectrometry for identification of the involved metabolisms (Cabugao et al., 2022); ionic and gas chromatography for the analysis of substrates and products from different metabolisms; 16S rRNA gene cloning, 16S rRNA gene amplicon and metagenomic shotgun sequencing (Puente-Sánchez et al., 2018), immunological detection, FISH, Catalysed Reported Deposition Fluorescence in situ Hybridization (CARD-FISH), and lipid biomarker analysis (Li et al., 2020) for complementary evaluation of microbial diversity, each one with its own limitations; enrichment cultures, metatranscriptomics (Li et al., 2020), quantitative PCR (Jesser et al., 2015) metaproteomics (Bagnoud et al., 2016), single cell genomics (Becraft et al., 2021) and Time-of-flight Secondary Ionization Mass Spectrometry (Tof-SIMS) (Viang and Dong, 2012) for identification of specific metabolisms; isolation of microorganisms for phenotypic characterization; genome sequencing and gene annotation for the identification of potential metabolisms. The use of several complementary methodologies is strongly recommended to identify convergent results avoiding the bias introduced by each one (Amils et al., 2023).
Suitability of fluorescence in situ hybridization for hard rock deep subsurface characterization
Of the different methodologies used to identify the microbial diversity, the adaptation of rRNA-targeted fluorescence in situ hybridization (rRNA-FISH) (Amand et al., 2005) to the analysis of microorganisms associated to semisolid and solid substrates was an important advance in the study of the hard rock deep subsurface (Hoshino et al., 2008). FISH technique allows the identification of microorganisms of interest, their quantification, and the analysis of their distribution in the sample (Figure 2). In fact, thanks to the possibility of performing multiple hybridizations on a single sample, this technique has been key to the identification of microbial associations and complementary metabolisms in both the oceanic (Schippers et al., 2005) and continental subsurface (Amils et al., 2023) An example is shown in Figure 2B, in which members of the genus Acidovorax, capable of oxidizing Fe(II) (Kappler et al., 2005), and the genus Sulfobacillus, which can couple the oxidation of reduced sulfur compounds to the reduction of Fe(III) (Justice et al., 2014), coexist together in the same microniche. Because FISH methodology requires fixation and denaturation of samples immediately after their extraction from within the cores, contamination is very unlikely. In addition, an important advantage over the rest of the methodologies is that it only requires a very small amount of sample, generating information on the presence of different microorganisms at microscopy size resolution, which cannot be obtained with the rest of the methodologies, as they require larger samples for their analysis (up to 100 g for DNA sequencing). Furthermore, FISH is a non-destructive methodology allowing the study of subsurface microorganisms in their natural environment: rock. Other used molecular biology methodologies such as “omics” yield significant bulk diversity results, but, unlike FISH, cannot provide information on the interconnection between the identified microorganisms and the mineral features of the complex matrix in which they inhabit.
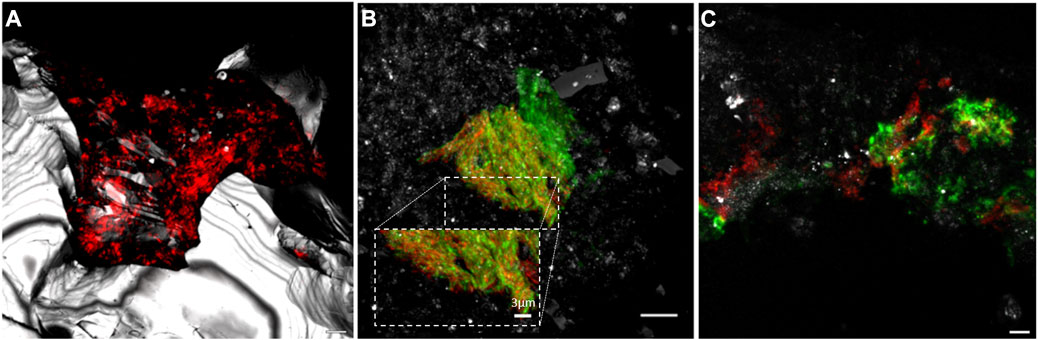
FIGURE 2. Microbial communities on mineral substrates of the Iberian Pyrite Belt subsurface visualized by Catalysed Reported Deposition Fluorescence in situ Hybridization (CARD-FISH) and Confocal Laser Scanning Microscopy (CLSM). (A) Bacteria domain at 139.4. (B) Interaction of Acidovorax (red) and Sulfobacillus (green) at 249.8 mbs. (C) Acidiphillium spp. biofilm at 228.4 mbs, in red, probe signal; in green, exopolysaccharides; in grey, reflection. Scale bars, 5 μm.
Because, in general, the fluorescence probes for FISH are designed to hybridize with the ribosomal RNA, positive signals strongly suggest the presence of metabolically active microorganisms in the deep subsurface, a fundamental question in subsurface geomicrobiology (Schippers et al., 2005; Puente-Sánchez et al., 2018). The use of CARD-FISH allows amplification of the hybridization signal facilitating the distinction between real hybridizations and artifacts, such as the presence of autofluorescence minerals or the unspecific binding of the probe or dye to the diverse mineral content present in each sample (Escudero et al., 2018; Templeton and Caro, 2023). In addition, the combination of FISH with fluorescence lectin binding assay (FLBA) allowed the existence of biofilms made of exopolysaccharides in the oligotrophic deep subsurface to be demonstrated (Figure 2C), contrary to the generally accepted idea that, in these extreme oligotrophic conditions, microorganisms are unable to use their limited source of energy in the generation of these metabolically expensive structures (Escudero et al., 2018; Amils et al., 2023). If needed, microorganisms use their limited resources to produce biofilms, taking advantage of their useful properties such as protection against desiccation, diffusion control of metabolic products and substrates, facilitating the operation of complementary metabolisms and interconnecting microniches, among others (Flemming et al., 2016).
Sequence information is required for the design of specific fluorescence probes. An ideal situation would be to carry out the metagenomic analysis of the 16S rRNA genes present to facilitate the design of FISH probes. But in the absence of this information, a huge collection of published FISH probes of different specificity can facilitate the analysis (Amils et al., 2023). Due to its outstanding properties new FISH procedures have been developed targeting not only rRNA but also mRNA or single genes (Moraru et al., 2010).
The use of confocal laser scanning microscopy (CLSM) has been an important improvement in the detection of microorganisms occupying different focal planes in the mineral substrates. Correlative fluorescence and Raman microscopy (Raman-FISH) can be used to analyze the mineral-microorganism interactions, a fundamental relationship in the deep subsurface (Escudero et al., 2020). The introduction of super-resolution microscopy has been an important advance to overcome the limitations of using fluorescence methodologies for the analysis of complex subsurface samples (Moraru and Amann, 2012). The Nanoscale Secondary Ion Mass Spectrometry (NanoSIMS) ability to measure stable isotopes and radioisotopes with appropriate half-lives has been used to image metabolically active microorganisms in complex communities such as those existing in the deep subsurface, and its coupling with Halogen in situ Hybridization (HISH) allow the simultaneous identification of microorganisms and the substrate uptake, providing important basic information on the operation of the deep subsurface (Musat et al., 2008).
Mars dark biosphere exploration
As mentioned and important limitation to estimate the subsurface habitability zone for rocky exoplanets is that deep subsurface life may not be able to modify the atmospheres of planets to be detectable remotely. This limitation does not exist in our Solar System, where exploration missions could be designed to collect information on the subsurface, or even better, to bring samples to Earth for analysis with more powerful and updated methodologies. This is the case for Mars, the planet that lost its surface water, and for which life at the surface is unlikely due to the extreme conditions such as lack of water, intense radiation, low temperatures, and strong oxidizing conditions (Margulis et al., 1979; Schofield et al., 1997; Rafkin et al., 2016; Martínez et al., 2017). The demonstration of Darwin´s prediction of life in hard rock deep subsurface of Earth changed this pessimistic point of view (Zhang et al., 2005; Fry et al., 2009; Breuker et al., 2011; Momper et al., 2017a; Dutta et al., 2018; Cockell et al., 2021) especially after the detection of liquid water in Mars subsurface (Orsei et al., 2018). There are an important number of studies suggesting the existence of life in the deep subsurface of Mars extrapolating results obtained on planet Earth (Boston et al., 1992; Jakosky and Shock, 1998; Cockell and Barlow, 2002; Michalski et al., 2018; Tarnas et al., 2018; Onstott et al., 2019; Sueoka et al., 2019; Stamenkovic et al., 2021; Sauterey et al., 2022). The demonstration of microbial life supporting the operation of the most important biogeochemical cycles (C, H, N, S, and Fe) at different depths in the hard rock deep subsurface of the Iberian Pyrite Belt (Amils et al., 2023), considered a good geological and mineralogical terrestrial Mars analogue (Fernández-Remolar et al., 2005), allows us to assert that the possible existence of past or even extant life in the subsurface of Mars must be considered (Price et al., 2018; 2022; Stamenkovic et al., 2019; Koike et al., 2020; Purkamo et al., 2020; Tarnas et al., 2021) and searched in future exploration missions. This will be the first space mission to explore the importance of the dark biosphere in the concept of planetary habitability.
Final considerations
The astrobiological interest of deep subsurface life is mainly concerned with broadening our concept of habitability which is fundamental to the search for life in the Universe. Our current concept of habitability does not take into consideration the possible existence of hard rock dark biospheres in rocky planets. Obviously, the presence of life in the hard rock continental deep subsurface has expanded our awareness of habitability significantly, although yet it is difficult to evaluate. McMahon and collaborators’ introduction of the concept of a subsurface habitability zone was a pivotal advance in the field (McMahon et al., 2013). However, their calculations need more input based on an increase in our knowledge of the limits of life in the continental deep subsurface, which to date, are still only speculative. These include sources of energy available and the way in which basic elemental biogeochemical cycles, fundamental for successful dark biospheres, operate. An important limitation to our ability to estimate the subsurface habitability zone for an exoplanet is that currently we can only evaluate its mass, density, surface, and atmosphere as well as its distance from a star and the star’s type, (Méndez et al., 2021). Future advances in the characterization of rocky exoplanets might allow solving this limitation.
We need to improve our still meager knowledge on the Earth igneous ocean crust and the continental dark biosphere to prepare the exploration of its possible existence on Mars or in the interior liquid water worlds in our Solar system, while we progress in the characterization of rocky exoplanets to incorporate them in the evaluation of their habitability, not only considering the possible existence of liquid water in its surface but the existence of conditions that could facilitate the habitability of its subsurface.
Author contributions
All authors listed have made a substantial, direct, and intellectual contribution to the work and approved it for publication.
Funding
This work has been financed by grants PID 2019-104812GB-I00 and TED 2021-129563B-I00 from the Spanish Ministerio de Ciencia e Innovación.
Acknowledgments
Authors want to thank R. Samalot for the English correction of the manuscript.
Conflict of interest
The authors declare that the research was conducted in the absence of any commercial or financial relationships that could be construed as a potential conflict of interest.
The handling editor AGF declared a past co-authorship with the author RA.
Publisher’s note
All claims expressed in this article are solely those of the authors and do not necessarily represent those of their affiliated organizations, or those of the publisher, the editors and the reviewers. Any product that may be evaluated in this article, or claim that may be made by its manufacturer, is not guaranteed or endorsed by the publisher.
References
Amand, A. L. S., Frank, D. N., De Groote, M. A., Basaraba, R. J., Orme, I. M., and Pace, N. R. (2005). Use of specific rRNA oligonucleotide probes for microscopic detection of Mycobacterium tuberculosis in cultures and tissue specimens. J. Clin. Microbiol. 43, 5369–5371. doi:10.1128/JCM.43.10.5369-5371.2005
Amend, J. P., McCollon, T. M., Hentscher, M., and Bach, W. (2011). Catabolic and anabolic energy for chemolithoautotrophs in deep-sea hydrothermal systems hosted in different rock types. Geochim. Cosmochim. Acta 75, 5736–5748. doi:10.1016/j.gca.2011.07.041
Amend, J. P., and Teske, A. (2005). Expanding frontiers in deep subsurface microbiology. Palaeogeogr. Palaeoclimatol. Palaeoecol. 219, 131–155. doi:10.1016/j.palaeo.2004.10.018
Amils, R., Escudero, C., Oggerin, M., Puente-Sánchez, F., Arce-Rodríguez, A., Fernández-Remolar, D., et al. (2023). Coupled C, H, N, S and Fe biogeochemical cycles operating in the continental deep subsurface of the Iberian Pyrite Belt. Environ. Microbiol. 25, 428–453. doi:10.1111/1462-2920.16291
Apps, J. A., and van de Kamp, P. C. (1993). Energy gases of abiogenic origin in the Earth’s crust. U. S. Geol. Surv. Prof. Pap. 1570, 81–132.
Bagnoud, A., Chourey, K., Hettich, R. L., Bruijn, I., Andersson, A. F., Leupin, O. X., et al. (2016). Reconstructing a hydrogen-driven microbial metabolic network in Opalinus Clay rock. Nat. Commun. 7, 12770. doi:10.1038/ncomms12770
Bar-On, Y., Phillips, R., and Milo, R. (2018). The biomass distribution on Earth. Proc. Nat. Acad. Sci. U. S. A. 115, 6506–6511. doi:10.1073/pnas.1711842115
Basso, O., Lascourreges, J. F., Le Borgne, F., Le Goff, C., and Magot, M. (2009). Characterization by culture and molecular analysis of the microbial.diversity of a deep subsurface gas storage aquifer. Res. Microbiol. 160, 107–116. doi:10.1016/j.resmic.2008.10.010
Bastin, E. S., Greer, F. E., Merritt, C., and Moulton, G. (1926). The presence of sulphate reducing bacteria in oil field waters. Science 63, 21–24. doi:10.1126/science.63.1618.21
Becraft, E. D., Lau Vetter, M. C., Bezuidt, O. K., Brown, J. M., Labonté, J. M., Kauneckaite-Griguole, K., et al. (2021). Evolutionary stasis of a deep subsurface microbial lineage. ISME J. 15 (10), 2830–2842. doi:10.1038/s41396-021-00965-3
Bomberg, M., Nyyssönen, M., Nousiainen, A., Hultman, J., Paulin, L., Auvinen, P., et al. (2014). Evaluation of molecular techniques in characterization of deep terrestrial biosphere. Open J. Ecol. 4, 468–487. doi:10.4236/oje.2014.48040
Borgonie, G., García-Moyano, A., Litthauer, D., Bert, W., Bester, A., van Heerden, E., et al. (2011). Nematoda from the terrestrial deep subsurface of South Africa. Nature 474, 79–82. doi:10.1038/nature09974
Boston, J. P., Ivanov, M. V., and McKay, C. P. (1992). On the possibility of chemosynthetic ecosystems in subsurface habitats on Mars. Icarus 95, 300–308. doi:10.1016/0019-1035(92)90045-9
Brazelton, W. J., Nelson, B., and Schrenk, M. O. (2012). Metagenomic evidence for H2 oxidation and H2 production by serpentinite-hosted subsurface microbial communities. Front. Microbiol. 2 (268), 268. doi:10.3389/fmicb.2011.00268
Breuker, A., Köweker, G., Blazejak, A., and Schippers, A. (2011). The deep biosphere in terrestrial sediments in the Chesapeake Bay area, Virginia, USA. Front. Microbiol. 2, 156. doi:10.3389/fmicb.2011.00156
Cabugao, K. G., Gushgari-Doyle, S., Chacon, S. S., Wu, X., Bhattacharyya, A., Bouskill, N., et al. (2022). Characterizing natural organic matter transformations by microbial communities in terrestrial subsurface ecosystems: A critical review of analytical techniques and challenges. Front. Microbiol. 13, 864895. doi:10.3389/fmicb.2022.864895
Chapelle, F. H., O'neill, K., Bradley, P. M., Methé, B. A., Ciufo, S. A., Knobel, L. L., et al. (2002). A hydrogen-based subsurface microbial community dominated by methanogens. Nature 415, 312–315. doi:10.1038/415312a
Chivian, D., Brodie, E. L., Alm, E. J., Culley, D. E., Dehal, P. S., DeSantis, T. Z., et al. (2008). Environmental genomics reveals a single-species ecosystem deep within Earth. Science 322, 275–278. doi:10.1126/science.1155495
Cockell, C. S. (2020). Astrobiology: Understanding life in the universe. 2nd edition, Hoboken: Wiley.
Cockell, C. S., and Barlow, N. G. (2002). Impact excavation and the search for subsurface life on Mars. Icarus 155, 340–349. doi:10.1006/icar.2001.6725
Cockell, C. S., Bush, T., Bryce, C., Direito, S., Fox-Powell, M., Harrison, J. P., et al. (2016). Habitability: A review. Astrobiol 16, 89–117. doi:10.1089/ast.2015.1295
Cockell, C. S., Schaefer, B., Wuchter, C., Coolen, M. J. L., Grice, K., Schnieders, L., et al. (2021). Shaping of the present-day deep biosphere at Chicxulub by the impact catastrophe that ended the cretaceous. Front. Microbiol. 12, 668240. doi:10.3389/fmicb,2021.668240
Cockell, C. S. (2014). Trajectories of martian habitability. Astrobiol 14, 182–203. doi:10.1089/ast.2013.1106
Cockell, C. S., Voytek, M. A., Gronstal, A. L., Finster, K., Kirshtein, J. D., Howard, K., et al. (2012). Impact disruption and recovery of the deep subsurface biosphere. Astrobiol 12, 231–246. doi:10.1089/ast.2011.0722
Colman, D., Poudel, S., Stamps, B. W., Boyd, E. S., and Spear, J. R. (2017). Microbial competition in porous environments can select against rapid biofilm growth. Proc. Natl. Acad. Sci. 114 (2), E161–E170. doi:10.1073/pnas.1525228113
Corliss, J. B., Dymond, J., Gordon, L. I., Edmond, J. M., von Herzen, R. P., Ballard, R. D., et al. (1979). Submarine thermal springs on the galapagos rift. Science 203, 1073–1083. doi:10.1126/science.203.4385.1073
Coskun, Ö. K., Vuillemin, A., Schubotz, F., Klein, F., Sichel, S. E., Eisenreich, W., et al. (2021). Quantifying the effects of hydrogen on carbon assimilation in a seafloor microbial community associated with ultramafic rocks. ISME J 16, 257–271. doi:10.1038/s41396-021-01066-x
Darwin, C. (1839). Voyages of the adventure and Beagle, volume III–journal and remarks. London: Henry Colburn, 1832–1836.
D’Hondt, S., Rutherford, S., and Spivack, A. J. (2002). Metabolic activity of subsurface life in deep-sea sediments. Science 295, 2067–2070. doi:10.1126/science.1064878
Dong, Y., Sanford, R. A., Locke, R. A., Cann, I. K., Mackie, R. I., and Fouke, B. W. (2014). Fe-oxide grain coatings support bacterial Fe-reducing metabolisms in 1.7-2.0 km-deep subsurface quartz arenite sandstone reservoirs of the Illinois Basin (USA). Front. Microbiol. 5 (511), 511. doi:10.3389/fmicb.2014.00511
Dutta, A., Dutta Gupta, S., Gupta, A., Sarkar, J., Roy, S., Mukherjee, A., et al. (2018). Exploration of deep terrestrial subsurface microbiome in Late Cretaceous Deccan traps and underlying Archean basement, India. India Sci. Rep. 8, 17459. doi:10.1038/s41598-018-35940-0
D´Hondt, S., Inagaki, F., Ferdelman, T., Jorgensen, B. B., Kato, K., Kemp, P., et al. (2007). Exploring subseafloor life with the integrated ocean drilling program. Sci. Drill. 5, 26–37. doi:10.5194/sd-5-26-2007
Edwards, K. J., Becker, K., and Colwell, F. (2012a). The deep, dark energy biosphere: Intraterrestrial life on Earth. Annu.Rev.Earth Planet. Sci. 40, 551–568. doi:10.1146/annurev-earth-042711-105500
Edwards, K. J., Bond, P. L., Gihring, T. M., and Banfield, J. F. (2000). An archaeal iron oxidizing extreme acidophile important in acid mine drainage. Science 287, 1796–1799. doi:10.1126/science.287.5459.1796
Edwards, K. J., Fisher, A. T., and Wheat, C. G. (2012b). The deep subsurface biosphere in igneous ocean crust: Frontier habitats for microbiological exploration. Front. Microbiol. 3, 8. doi:10.3389/fmicb.2012.00008
El-Naggar, M. Y., Wanger, G., Leung, K. M., Yuzvinsky, T. D., Southam, G., Yang, J., et al. (2010). Electrical transport along bacterial nanowires from Shewanella oneidensis MR-1. Proc.Natl. Acad. Sci. U. S. A. 107, 18127–18131. doi:10.1073/pnas.1004880107
Escudero, C., del Campo, A., Ares, J. R., Sánchez, C., Martínex, J. M., Gómez, F., et al. (2020). Visualizing microorganisms-mineral interactions in the iberian pyrite Belt subsurface: The Acidovorax case. Front. Microbiol. 11, 572104. doi:10.3389/fmicb.2020.572104
Escudero, C., Vera, M., Oggerin, M., and Amils, R. (2018). Active microbial biofilms in deep poor porous continental subsurface rocks. Sci. Rep. 8 (1), 1538. doi:10.1038/s41598-018-19903-z
Eydal, H. S., Jägevall, S., Hermansson, M., and Pedersen, K. (2009). Bacteriophage lytic to Desulfovibrio aespoeensis isolated from deep groundwater. ISME J. 3, 1139–1147. doi:10.1038/ismej.2009.66
Fernández-Remolar, D. C., Prieto-Ballesteros, O., Rodríguez, N., Gómez, F., Amils, R., Gómez- Elvira, J., et al. (2008). Underground habitats in the río tinto basin: A model for subsurface life habitats on Mars. Astrobiol 8, 1023–1047. doi:10.1089/ast.2006.0104
Fernández-Remolar, D., Morris, R. V., Gruener, J. E., Amils, R., and Knoll, A. (2005). The Río Tinto Basin, Spain: Mineralogy, sedimentary geobiology, and implications for interpretation of outcrop rocks at Meridiani Planum, Mars. Earth Planet. Sci. Lett. 240, 149–167. doi:10.1016/j.epsl.2005.09.043
Flemming, H.-C., Wingender, T., Szewzyk, P., Steinberg, P., Rice, S. A., and Kjelleberg, S. (2016). Biofilms: An emergent form of bacterial life. Nat. Rev. Microbiol. 14, 563–575. doi:10.1038/nrmicro.2016.94
Fredrickson, J. K., and Balkwill, D. L. (2006). Geomicrobial processes and biodiversity in the deep terrestrial subsurface. Geomicrobiol. J. 23, 345–356. doi:10.1080/01490450600875571
Fredrickson, J. K., McKinley, J. P., Bjornstad, B. N., Long, P. E., Ringelberg, D. B., White, D. C., et al. (1997). Pore-size constraints on the activity and survival of subsurface bacteria in a late cretaceous shale-sandstone sequence, northwestern New Mexico. Geomicrobiol. J. 14, 183–202. doi:10.1080/01490459709378043
Fry, N. K., Fredrickson, J. K., Fishbain, S., Wagner, M., and Stahl, D. A. (2009). Population structure of microbial communities associated with two deep, anaerobic, alkaline aquifers. Appl. Environ. Microbiol. 63, 1498–1504. doi:10.1128/AEM.63.4.1498-1504.1997
Gihring, T., Moser, D., Lin, L. H., Davidson, M., Onstott, T., Morgan, L., et al. (2006). The distribution of microbial taxa in the subsurface water of the Kalahari Shield, South Africa. Geomicrobiol. J. 23, 415–430. doi:10.1080/01490450600875696
Gold, T. (1992). The deep, hot biosphere. Proc. Natl. Acad. Sci. 89, 6045–6049. doi:10.1073/pnas.89.13.6045
Gronstal, A. L., Voytek, M. A., Kirshtein, J. D., Nicole, M., Lowit, M. D., and Cockell, C. S. (2009). Contamination assessment in microbiological sampling of the Eyreville core, Chesapeake Bay impact structure. Geol. Soc. Am. Spec. Pap. 458, 951–964. doi:10.1130/2009.2458(41)
Hart, M. H. (1979). Habitable zones about main sequence stars. Icarus 37, 351–357. doi:10.1016/0019-1035(79)90141-6
Hoehler, T. M. (2004). Biological energy requirements as quantitative boundary conditions for life in the subsurface. Geobiol 2, 205–215. doi:10.1111/j.1472-4677.2004.00033.x
Hoshino, T., Yilmaz, L. S., Noguera, D. R., Daims, H., and Wagner, M. (2008). Quantification of target molecules needed to detect microorganisms by fluorescence in situ hybridization (FISH) and catalyzed reporter deposition-FISH. Appl. Environ. Microbiol. 74, 5068–5077. doi:10.1128/AEM.00208-08
Inagaki, F., Hinrichs, K.-U., Kubo, Y., Bowles, M. W., Heuer, V. B., Hong, W.-L., et al. (2015). Exploring deep microbial life in coal-bearing sediment down to ∼2.5 km below the ocean floor. Science 349, 420–424. doi:10.1126/science.aaa6882
Ino, K., Hernsdorf, A. W., Konno, U., Kouduka, M., Yanagawa, K., Kato, S., et al. (2017). Ecological and genomic profiling of anaerobic methane-oxidizing archaea in a deep granitic environment. ISME J. 12, 31–47. doi:10.1038/ismej.2017.140
Ino, K., Konno, U., Kouduka, M., Hirota, A., Togo, Y. S., Fukuda, A., et al. (2016). Deep microbial life in high-quality granitic groundwater from geochemically and geographically distinct underground boreholes. Environ. Microbiol. Rep. 8, 285–294. doi:10.1111/1758-2229.12379
Itävaara, M., Nyyssönen, M., Kapanen, A., Nousiainen, A., Ahonen, L., and Kukkonen, I. (2011). Characterization of bacterial diversity to a depth of 1500 m in the Outokumpu deep borehole, Fennoscandian Shield. FEMS Microbio.l Ecol. 77, 295–309. doi:10.1111/j.1574-6941.2011.01111.x
Ivarson, M., Bengtson, S., Drake, H., and Francis, W. (2018). Fungi in deep subsurface environments. Adv. Appl. Microbiol. 102, 83–116. doi:10.1016/bs.aambs.2017.11.001
Jakosky, B. M., and Shock, E. L. (1998). The biological potential of Mars, the early Earth, and Europa. J. Geophys. Res. 103, 19359–19364. doi:10.1029/98je01892
Jesser, K. J., Fullerton, H., Hager, K. W., and Moyer, C. L. (2015). Quantitative PCR analysis of functional genes in iron-rich microbial mats at an active hydrothermal vent system (Lō'ihi Seamount, Hawai'i). Appl. Environ. Microbiol. 81 (9), 2976–2984. doi:10.1128/AEM.03608-14
Johnson, S. S., Hebsgaard, M. B., Christensen, T. R., Mastepanov, M., Nielsen, R., Munch, K., et al. (2007) Ancient bacteria show evidence of DNA repair. Proc. Natl. Acad. Sci. U. S. A. 4, 104(36):14401–14405. doi:10.1073/pnas.0706787104
Jones, A. A., and Bennett, P. C. (2017). Mineral ecology: surface specific colonization and geochemical drivers of biofilm accumulation, composition, and phylogeny. Front. Microbiol. 8, 491. doi:10.3389/fmicb.2017.00491
Jørgensen, B. B. (2011). Deep subseafloor microbial cells on physiological standby. Proc. Natl. Acad. Sci. 108 (45), 18193–18194. doi:10.1073/pnas.1115421108
Justice, N. B., Norman, A., Brown, C. T., Singh, A., Thomas, B. C., and Banfield, J. F. (2014). Comparison of environmental and isolate Sulfobacillus genomes reveals diverse carbon, sulfur, nitrogen, and hydrogen metabolisms. BMC genomics 15, 1107. doi:10.1186/1471-2164-15-1107
Kallmeyer, J., Pockalny, R., Adhikari, R. R., Smith, D. C., and D´Hont, S. (2012). Global distribution of microbial abundance and biomass in subseafloor sediment. Proc. Nat. Acad. Sci. U. S. A. 109, 16213–16216. doi:10.1073/pnas.1203849109
Kappler, A., Schink, B., and Newman, D. K. (2005). Fe(III) mineral formation and cell encrustation by the nitrate-dependent Fe(II)-oxidizer strain BoFeN1. Geobiol 3, 235–245. doi:10.1111/j.1472-4669.2006.00056.x
Kasting, J. F., and Catling, D. (2003). Evolution of a Habitable Planet. Ann. Rev. Astron. Astrophys. 41, 429–463. doi:10.1146/annurev.astro.41.071601.170049
Kasting, J. F., Whitmire, D. P., and Reynolds, R. T. (1993). Habitable zones around main sequence stars. Icarus 101, 108–128. doi:10.1006/icar.1993.1010
Kieft, T. L. (2016). “Microbiology of the deep continental biosphere. Their world: A diversity of microbial environments. 1, 225–249. doi:10.1007/978-3-319-28071-4_6
Kieft, T. (2010). “Sampling the deep sub-surface using drilling and coring techniques,” in Handbook of hydrocarbon and lipid microbiology (Springer), 3427–3441.
Klein, F., Tarnas, J. D., and Bach, W. (2020). Abiotic sources of molecular hydrogen on Earth. Elements 16 (1), 19–24. doi:10.2138/gselements.16.1.19
Koike, M., Nakada, R., Kajitani, I., Usui, T., Tamenori, Y., Sugahara, H., et al. (2020). In-situ preservation of nitrogen-bearing organics in Noachian Martian carbonates. Nat. Comm. 11 (1), 1988. doi:10.1038/s41467-020-15931-4
Kyle, J. E., Eydal, H. S., Ferris, F. G., and Pedersen, K. (2008). Viruses in granitic groundwater from 69 to 450 m depth of the Äspö hard rock laboratory, Sweden. ISME J. 2, 571–574. doi:10.1038/ismej.2008.18
Labonté, J. M., Field, E. K., Lau, M., Chivian, D., Van Heerden, E., Wommack, K. E., et al. (2015). Single cell genomics indicates horizontal gene transfer and viral infections in a deep subsurface Firmicutes population. Front. Microbio,l 6, 349. doi:10.3389/fmicb.2015.00349
Lammer, H., Bredehoft, J. H., Coustenis, A., Khodachenko, M. L., Kaltenegger, L., Grasset, O., et al. (2009). What makes a planet habitable? Astron. Astrophys. Rev. 17, 181–249. doi:10.1007/s00159-009-0019-z
Lau, M. C., Cameron, C., Magnabosco, C., Brown, C. T., Schilkey, F., Grim, S., et al. (2014). Phylogeny and phylogeography of functional genes shared among seven terrestrial subsurface metagenomes reveal N-cycling and microbial evolutionary relationships. Front. Microbiol. 5, 531. doi:10.3389/fmicb.2014.00531
Lau, M. C., Kieft, T. L., Kuloyo, O., Linage-Alvarez, B., Van Heerden, E., Lindsay, M. R., et al. (2016). An oligotrophic deep-subsurface community dependent on syntrophy is dominated by sulfur-driven autotrophic denitrifiers. Proc. Nat. Acad. Sci. 113, E7927–E7936. doi:10.1073/pnas.1612244113
Leandro, T., Rodrígez, N., Sanz, J. L., da Costa, M. S., and Amils, R. (2018). Study of methanogenic enrichment cultures of rock cores from the deep subsurface of the Iberian Pyritic Belt. Heliyon 4, e00605. doi:10.1016/j.heliyon.2018.e00605
Leong, J. A. M., Howells, A. E., Robinson, K. J., Cox, A., Debes, R. V., Fecteau, K., et al. (2021). Theoretical prediction versus environmental observations on serpentinization fluids: lessons from the Smail Ophiolite in Oman. J. Geophys. Res. Solid Earth 126, e2020JBO20756. doi:10.1029/2020JBO20756
Li, J., Mara, P., Schubotz, F., Sylvan, J. B., Burgaud, G., Klein, F., et al. (2020). Recycling and metabolic flexibility dictate life in the lower oceanic crust. Nature 579, 250–255. doi:10.1038/s41586-020-2075-5
Lin, L. H., Slater, G. F., Sherwood Lollar, B., Lacrampe-Couloume, G., and Onstott, T. C. (2004). The yield and isotopic composition of radiolytic H2, a potential energy source for the deep subsurface biosphere. Geochim. Cosmochim. Acta 69 (4), 893–903. doi:10.1016/j.gca.2004.07.032
Lin, L. H., Wang, P. L., Rumble, D., Lippmann-Pipke, J., Boice, E., Pratt, L. M., et al. (2006). Long-term sustainability of a high-energy, low-diversity crustal biome. Science 314, 479–482. doi:10.1126/science.1127376
Lingam, M., and Loeb, A. (2021). Life in the Cosmos. From biosignatures to thechnosignatures. Boston: Harvard University Press.
Lingam, M., and Loeb, A. (2020). Potential for liquid water biochemistry deep under the surfaces of the Moon, Mars and beyond. Astrophys. J. Lett- 90, L11. doi:10.3847/2041-8213/abb608
Lipman, C. B. (1931). Living microorganisms in ancient rocks. J. Bacteriol. 22, 183–198. doi:10.1128/jb.22.3.183-198.1931
Magnabosco, C., Lin, L. H., Dong, H., Bomberg, M., Ghiorse, W., Stan-Lotter, H., et al. (2018). The biomass and biodiversity of the continental subsurface. Nat. Geosci. 11, 707–717. doi:10.1038/s41561-018-0221-6
Magnabosco, C., Ryan, K., Lau, M. C., Kuloyo, O., Lollar, B. S., Kieft, T. L., et al. (2016). A metagenomic window into carbon metabolism at 3 km depth in Precambrian continental crust. ISME J. 10, 730–741. doi:10.1038/ismej.2015.150
Magnabosco, C., Tekere, M., Lau, M. C., Linage, B., Kuloyo, O., Erasmus, M., et al. (2014). Comparisons of the composition and biogeographic distribution of the bacterial communities occupying South African thermal springs with those inhabiting deep subsurface fracture water. Front. Microbiol. 5, 679. doi:10.3389/fmicb.2014.00679
Margulis, L., Mazur, P., Barghoom, E. S., Halvorson, H. O., Jukes, T. H. J., and Kaplan, I. R. (1979). TheViking Mission: implications for life in Vallis Marineris area. Science 305, 78–81. doi:10.1126/science.1097549
Martínez, G. M., Newman, C. N., deVicente-Retortillo, A., Fischer, E., Rennó, N. O., Richardson, M. I., et al. (2017). Erratum to: The Modern Near-Surface Martian Climate: A Review of in-situ Meteorological Data from Viking to Curiosity. Space Sci. Rev. 212, 339–340. doi:10.1007/s11214-017-0368-2
McMahon, S., O´Malley-James, J., and Parnell, J. (2013). Circumstellar habitable zones for Deep terrestrial biospheres. Planet. Space Sci. 85, 312–318. doi:10.1016/j.pss.2013.07.002
McMahon, S., and Parnell, J. (2014). Weighing the deep continental biosphere. FEMS Microbiol. Ecol. 87, 113–120. doi:10.1111/1574-6941.12196
Méndez, A., Rivera-Valentín, E. G., Schulze-Makuch, D., Filberto, J., Ramírez, R. M., Wood, T. E., et al. (2021). Habitability models for Astrobiology. Astrobiol 21 (8), 1017–1027. doi:10.1089/ast.2020.2342
Merino, N., Aronson, H. S., Bojanova, D. P., Feyhl-Busca, J., Wong, M. L., Zhang, S., et al. (2019). Living at the extremes: Extremophiles and the limits of life in a planetary context. Front. Microbiol. 10, 780. doi:10.3389/fmicb.2019.00780
Michalski, J. R., Onstott, T. C., Mojzsis, S. J., Mustard, J., Chan, Q. H. S., Niles, P. B., et al. (2018). The Martian subsurface as a potential window into the origin of life. Nat. Geosc. 11, 21–26. doi:10.1038/s41561-017-0015-2
Momper, L., Jungbluth, S. P., Lee, M. D., and Amend, J. P. (2017a). Energy and carbon metabolisms in a deep terrestrial subsurface fluid microbial community. ISME J. 11, 2319–2333. doi:10.1038/ismej.2017.94
Momper, L., Reese, B. K., Zinke, L., Wanger, G., Osburn, M. R., Moser, D., et al. (2017b). Major phylum-level differences between pore fluid and host rock bacterial communities in the terrestrial deep subsurface. Environ. Microbiol. Rep. 9, 501–511. doi:10.1111/1758-2229.12563
Moraru, C., Amann, R., Cremer, M., and Schermelleh, L. (2012). Crystal ball: fluorescence in situ hybridization in the age of super-resolution microscopy. Syst. Appl. Microbiol. 35, 43–64. doi:10.1007/978-1-62703-137-0_4
Moraru, C., Lam, P., Fuchs, B. M., Kuypers, M. M., and Amann, R. (2010). GeneFISH - an in situ technique for linking gene presence and cell identity in environmental microorganisms. Environ. Microbiol. 12, 3057–3073. doi:10.1111/j.1462-2920.2010.02281.x
Morita, R. Y. (1999). Is H2 the universal energy source for long-term survival? Microb. Ecol. 38, 307–320. doi:10.1007/s002489901002
Morita, R., and Zobell, C. (1955). Occurrence of bacteria in pelagic sediments collected during the Mid- Pacific Expedition. Deep-Sea Res. 3, 66–73. doi:10.1016/0146-6313(55)90036-8
Moser, D. P., Gihring, T. M., Brockman, F. J., Fredrickson, J. K., Balkwill, D. L., Dollhopf, M. E., et al. (2005). Desulfotomaculum and Methanobacterium spp. Dominate a 4- to 5-Kilometer-Deep Fault. Appl. Environ. Microbiol. 71, 8773–8783. doi:10.1128/AEM.71.12.8773-8783.2005
Murakami, Y., Fujita, Y., Naganuma, T., and Iwatsuki, T. (2002). Abundance and viability of the groundwater microbial communities from a borehole in the Tono uranium deposit area, central Japan. Microb. Environ. 17, 63–74. doi:10.1264/jsme2.2002.63
Musat, N., Halm, H., Winterholler, B., Hoppe, P., Peduzi, S., Hillion, F., et al. (2008). A single-cell view on the ecophysiology of anaerobic phototrophic bacteria. Proc. Natl. Acad. Sci. 105, 17861–17866. doi:10.1073/pnas0809329105
Nealson, K. H., Inagaki, F., and Takai, K. (2005). Hydrogen-driven subsurface lithoautotrophic microbial ecosystems (SLiMEs): do they exist and why should we care? Trends Microbiol. 13, 405–410. doi:10.1016/j.tim.2005.07.010
Nisbet, E., Zahnle, K., Gerasimov, M. V., Helbert, J., Jaumann, R., Hofman, B. A., et al. (2007). Creating habitable zones. At all scales, from planets to mud-micro-habitats, on Earth and on Mars. Space aci. Rev. 129, 79–121. doi:10.1007/978-0-387-74288-5_4
Nuppunen-Puputti, M., Kietäväinen, R., Raulio, M., Soro, A., Purkamo, L., Kukkonen, I., et al. (2022). Epilithic microbial community functionality in deep oligotrophic continental bedrock. Front. Microbiol. 13, 826048. doi:10.3389/fmicb.2022.826048
Nyyssönen, M., Bomberg, M., Kapanen, A., Nousiainen, A., Pitkänen, P., and Itävaara, M. (2012). Methanogenic and sulphate-reducing microbial communities in deep groundwater of crystalline rock fractures in Olkiluoto, Finland. Geomicrobiol. J. 29, 863–878. doi:10.1080/01490451.2011.635759
Nyyssönen, M., Hultman, J., Ahonen, L., Kukkonen, I., Paulin, L., Laine, P., et al. (2014). Taxonomically and functionally diverse microbial communities in deep crystalline rocks of the Fennoscandian shield. ISME J. 8, 126–138. doi:10.1038/ismej.2013.125
Onstott, T. C., Ehlmann, B. L., Sapers, H., Coleman, M., Ivarsonn, M., Marlow, J. J., et al. (2019). Paleo-rock-hosted life on Earth and the search on Mars: a review and strategy for exploration. Astrobiol 19 (10), 1230–1262. doi:10.1089/ast.2018.1960
Onstott, T., Magnabosco, C., Aubrey, A. D., Burton, A. S., Dworkin, J. P., Elsila, J. E., et al. (2014). Does aspartic acid racemization constrain the depth limit of the subsurface biosphere? Geobiology 12 (1), 1–19. doi:10.1111/gbi.12069
Onstott, T., McGown, D. J., Bakemans, C., Ruskeeniemi, T., Ahonen, L., Telling, J., et al. (2009). Microbial Communities in Subpermafrost Saline Fracture Water at the Lupin Au Mine, Nunavut, Canada. Microb. Ecol. 58, 786–807. doi:10.1007/s00248-009-9553-5
Onstott, T., Moser, D. P., Pfiffner, S. M., Fredrickson, J. K., Brockman, F. J., Phelps, T., et al. (2003). Indigenous and contaminant microbes in ultradeep mines. Environ. Microbiol. 5, 1168–1191. doi:10.1046/j.1462-2920.2003.00512.x
Orcutt, B. N., Sylvan, J. B., Knab, N. J., and Edwards, K. J. (2011). Microbial ecology of the dark ocean above, at, and below the seafloor. Microbiol. Mol. Biol. Rev. 75, 361–422. doi:10.1128/MMBR.00039-10
Orsei, R., Lauro, S. E., Pettinelli, E., Cicchetti, A., Coradini, M., Di Paolo, U., et al. (2018). Radar evidence of subglacial liquid water on Mars. Science 361, 490–493. doi:10.1126/science.aar7268
Orsi, W. D., Magritsch, T., Vargas, S., Coskun, O. K., Vuillemin, A., Höhna, S., et al. (2021). Genome Evolution in Bacteria Isolated from Million-Year-Old Subseafloor Sediment. mBio 12, e0115021–21. doi:10.1128/mBio01150-21
Osburn, M. R., Larowe, D. E., Momper, L. M., and Amend, J. P. (2014). Chemolithotrophy in the continental deep subsurface: Sanford Underground Research Facility (SURF), USA. Microbiol 5, 610. doi:10.3389/fmicb.2014.00610
Pedersen, K., and Albinsson, Y. (1992). Possible Effects of Bacteria on Trace Element Migration in Crystalline Bed-Rock. Radiochim. Acta 58 (59), 365–370. doi:10.1524/ract.1992.5859.2.365
Pedersen, K. (2000). Exploration of deep intraterrestrial microbial life: current perspectives. FEMS Microbiol. Lett. 185, 9–16. doi:10.1111/j.1574-6968.2000.tb09033.x
Pedersen, K. (1997). Microbial life in deep granitic rock. FEMS Microbiol. Rev. 20, 399–414. doi:10.1111/j.1574-6976.1997.tb00325.x
Pedersen, K. (1999). Subterranean microorganisms and radioactive waste disposal in Sweden. Eng. Geol. 52, 163–176. doi:10.1016/s0013-7952(99)00004-6
Phelps, T., Murphy, E., Pfiffner, S., and White, D. (1994). Comparison between geochemical and biological estimates of subsurface microbial activities. Microb. Ecol. 28, 335–349. doi:10.1007/BF00662027
Price, A., Macey, M. C., Pearson, V. K., Schwenzer, S. P., Ramkissoon, N. K., and Olsson-Francis, S. P. (2022). Oligotrophic growth of nitrate-dependent Fe2+-oxidizing microorganisms under simulated early martian conditions. Front. Microbiol. 13, 800219. doi:10.3389/fmicb.2022.800219
Price, A., Pearson, V. K., Schwenzer, S. P., Miot, J., and Olsson-Francis, K. (2018). Nitrate-dependent iron oxidation: a potential Mars metabolism. Front. Microbiol. 9, 513. doi:10.3389/fmicb.2018.00513
Probst, A. J., Birarda, G., Holman, H. Y. N., DeSantis, T. Z., Wanner, G., Andersen, G. L., et al. (2014). Coupling Genetic and Chemical Microbiome Profiling Reveals Heterogeneity of Archaeome and Bacteriome in Subsurface Biofilms That Are Dominated by the Same Archaeal Species. PLoS One 9 (6), e99801. doi:10.1371/journal.pone.0099801
Puente-Sánchez, F., Arce-Rodríguez, A., Oggerin, M., García-Villadangos, M., Moreno-Paz, M., Blanco, Y., et al. (2018). Viable cyanobacteria in the deep continental subsurface. Proc. Natl. Acad. Sci. 115, 10702–10707. doi:10.1073/pnas.1808176115
Puente-Sánchez, F., Moreno-Paz, M., Rivas, L., Cruz-Gil, P., García-Villadangos, M., Gómez, M., et al. (2014). Deep subsurface sulfate reduction and methanogenesis in the Iberian Pyrite Belt revealed through geochemistry and molecular biomarkers. Geobiol 12, 34–47. doi:10.1111/gbi.12065
Purkamo, L., Bomberg, M., Nyyssönen, M., Kukkonen, I., Ahonen, L., and Itävaara, M. (2015). Heterotrophic communities supplied by ancient organic carbon predominate in deep Fennoscandian bedrock fluids. Microb. Eco.l 69, 319–332. doi:10.1007/s00248-014-0490-6
Purkamo, L., Bomberg, M., Nyyssönen, M., Kukkonen, I., Ahonen, L., Kietäväinen, R., et al. (2013). Dissecting the deep biosphere: retrieving authentic microbial communities from packer-isolated deep crystalline bedrock fracture zones. FEMS Microbiol. Ecol. 85, 324–337. doi:10.1111/15746941.12126
Purkamo, L., Kietäväinen, R., Miettinen, H., Sohlberg, E., Kukkonen, I., Itävaara, M., et al. (2018). Diversity and functionality of archaeal, bacterial and fungal communities in deep Archaean bedrock groundwater. FEMS Microbiol. Ecol. 94, 8. doi:10.1093/femsec/fiy116
Purkamo, L., Kietäväinen, R., Nuppumen-Puputto, M., Bomberg, M., and Cousins, C. (2020). Ultradeep Microbial Communities at 4.4 km within Crystalline Bedrock: Implications for Habitability in a Planetary Context. Life 10, 2. doi:10.3390/life10010002
Quemener, M., Mara, P., Schubotz, F., Beaudoin, D., Li, W., Pachiadaki, M., et al. (2020). Meta-omics highlights the diversity, activity and adaptations of fungi in deep oceanic crust. Environ. Microbiol. 22 (9), 3950–3967. doi:10.1111/1462-2920.15181
Rafkin, S. C. R., Pla-García, J., Kahre, M. A., Gómez-Elvira, J., Hamilton, V. E., Marín, M., et al. (2016). The meteorology of Gale Crater as determined from Rover Environmental Monitoring Station observations and numerical modeling. Part II: Interpretation. Icarus 280, 114–138. doi:10.1016/j.icarus.2016.01.031
Rahlf, J., Turzynski, V., Esser, S. P., Monsees, I., Bornemann, T. L. V., Figueroa-Gonzalez, P. A., et al. (2021). Lytic archaeal viruses infect abundant primary producers in Earth´s crust. Nat. Commun. 12, 4642. doi:10.1038/s41467-021-24803-4
Rajala, P., Bomberg, M., Kietäväinen, R., Kukkonen, I., Ahonen, L., Nyyssönen, M., et al. (2015). Rapid reactivation of deep subsurface microbes in the presence of C-1 compounds. Microorg 3, 17–33. doi:10.3390/microorganisms3010017
Reese, B. K., Sobol, M. S., Bowles, M. W., and Hinrichs, K. U. (2021). Redefining the subsurface biosphere: characterization of fungi isolated from energy-limited marine deep subsurface sediment. Front. Fungal Biol. 2, 727543. doi:10.3389/ffunb.2021.727543
Rempfert, K. R., Miller, H. M., Bompard, N., Nothaft, D., Matter, J. M., Kelemen, ., et al. (2017). Geological and geochemical controls on subsurface microbial life in the Samail Ophiolite, Oman. Front. Microbiol. 8, 56. doi:10.3389/fmicb.2017.00056
Rogers, J., Bennett, P., and Choi, W. (1998). Feldspars as a source of nutrients for microorganisms. Am. Mineral. 83, 1532–1540. doi:10.2138/am-1998-11-1241
Ruff, S. E., Humez, P., Hrabe de Angelis, I., Diao, M., Nightingale, M., Chao, S., et al. (2023) Hydrogen and dark oxygen drive microbial productivity in diverse groundwater ecosystems. Nat. Commun. 14(1), 3194. doi:10.1038/s41467-023-38523-4
Sahl, J. W., Schmidt, R., Swanner, E. D., Mandernack, K. W., Templeton, A. S., Kieft, T. L., et al. (2008). Subsurface microbial diversity in deep-granitic-fracture water in Colorado. Appl. Environ. Microbiol. 74, 143–152. doi:10.1128/AEM.01133-07
Sanz, J. L., Rodríguez, N., Escudero, C., and CarrizoAmils, D. R. (2021). Biological production of H2, CH4 and CO2 in the Deep subsurface of the Iberian Pyrite Belt. Environ. Microbiol. 23 (7), 3913–3922. doi:10.1111/1462-2920.15561
Sauterey, B., Charnay, B., Affholder, A., Mazevet, S., and Ferriére, R. (2022). Early Mars habitability and global cooling by H2-based methanogens. Nat. Astron. 6, 1263–1271. doi:10.1038/s41550-022-01786-w
Schippers, A., Neretin, L. N., Kallmeyer, J., Ferdelman, T. G., Cragg, B. A., Parkes, J., et al. (2005). Prokaryotic cells of the deep sub-seafloor biosphere identified as living bacteria. Nature 433, 861–864. doi:10.1038/nature03302
Schofield, J. T., Barnes, J. R., Crisp, D., Haberle, R. M., Larsen, S., Magalhäes, J. A., et al. (1997). The Mars Pathfinder Atmospheric Structure Investigation/Meteorology (ASI/MET) Experiment. Science 278, 1752–1758. doi:10.1126/science.278.5344.1752
Shelobolina, E., Xu, H., Konishi, H., Kukkadapu, R., Wu, T., Blöthe, M., et al. (2012). Microbial lithotrophic oxidation of structural Fe (II) in biotite. Appl. Environ. Microbiol. 78, 5746–5752. doi:10.1128/AEM.01034-12
Sherwood Lollar, G. S., Warr, O., Telling, J., Osburn, M. R., and Sherwood Lollar, B. (2019). ‘Follow the Water’: Hydrogeochemical Constraints on Microbial Investigations 2.4 km Below Surface at the Kidd Creek Deep Fluid and Deep Life Observatory. Hydrogeochem. constrains Microb. investigations 2.4 km bellow Surf. A. T. Kidd Creek Deep Fluid Deep Life Observatory. Geomicrobiol. J. 36, 859–872. doi:10.1080/01490451.2019.1641770
Sherwood-Lollar, B., Voglesonger, K., Lin, L. H., Lacrampe-Couloume, G., Abrajano, T. A., Onstott, T. C., et al. (2007). Hydrogeologic Controls on Episodic H2 Release from Precambrian Fractured Rocks—Energy for Deep Subsurface Life on Earth and Mars. Astrobiol 7 (6), 971–986. doi:10.1089/ast.2006.0096
Shock, E. L. (2009). Minerals as energy sources for microorganisms. Econ. Geol. 104, 1235–1248. doi:10.2113/gsecongeo.104.8.1235
Soares, A., Edwards, A., An, D., Bagnoud, A., Bradley, J., Barnhart, E., et al. (2023). A global perspective on bacterial diversity in the terrestrial deep subsurface. Microbiol 169, 001172. doi:10.1099/mic.0.001172
Sohlberg, E., Bomberg, M., Miettinen, H., Nyyssönen, M., Salavirta, H., Vikman, M., et al. (2015). Revealing the unexplored fungal communities in deep groundwater of crystalline bedrock fracture zones in Olkiluoto, Finland. Front. Microbiol. 6, 573. doi:10.3389/fmicb.2015.00573
Stamenkovic, V., Beegle, L. W., Zacny, K., Arumugam, D. D., Baglioni, P., Barba, W., et al. (2019). The next frontier for planetary and human exploration. Nat. Astron. 3, 116–120. doi:10.1038/s41550-018-0676-9
Stamenkovic, V., Lynch, K., Boston, P., Tarnas, J., Edwards, C. D., Sherwood-Lollar, B., et al. (2021). Deep Trek: Science of Subsurface Habitability and Life on Mars. Bull. Am. Astronomical Soc. 53 (4). doi:10.3847/25c2cfeb.dc18f731
Steen, A. D., Jorgensen, B. B., and Lomstein, B. (2013). Abiotic racemization kinetics of amino acids in marine sediments. PLoS ONE 8 (8), e71648. doi:10.1371/journal.pone.0071648
Stevens, T. O., and McKinley, J. P. (1995). Lithoautotrophic microbial ecosystems in deep basalt aquifers. Science 270, 450–455. doi:10.1126/science.270.5235.450
Sueoka, Y., Yamashita, S., Kouduka, M., and Suzuki, Y. (2019). Deep microbial colonization in saponite-bearing fractures in aged basaltic crust: implications for subsurface life on Mars. Front. Microbiol. 10, 2793. doi:10.3389/fmicb.2019.02793
Suzuki, S., Si, I., Wu, A., Cheung, A., Tenney, A., Wanger, G., et al. (2013). Microbial diversity in The Cedars, an ultrabasic, ultrareducing, and low salinity serpentinizing ecosystem. Proc. Natl. Acad. Sci. 110, 15336–15341. doi:10.1073/pnas.1302426110
Swanner, E. D., Nell, R. M., and Templeton, A. S. (2011). Ralstonia species mediate Fe-oxidation in circumneutral, metal-rich subsurface fluids of Henderson mine, CO. Co. Chem. Geol. 284, 339–350. doi:10.1016/j.chemgeo.2011.03.015
Takai, K., Moser, D. P., DeFlaun, M., Onstott, T. C., and Fredrickson, J. K. (2001). Archaeal diversity in waters from deep South African gold mines. Appl. Environ. Microbiol. 67, 5750–5760. doi:10.1128/AEM.67.21.5750-5760.2001
Tarnas, J. D., Mustard, J. F., Sherwood-Lollar, B., Bramble, M. S., Cannon, K. M., Palumbo, T. C., et al. (2018). Radiolytic H2 production on Noachian Mars: Implications for habitability and atmospheric warming. Earth Planet. Sci. Lett. 502, 133–145. doi:10.1016/j.epsl.2018.09.001
Tarnas, J. D., Mustard, J. F., Sherwood-Lollar, B., Cannon, K. M., Lorand, J-P., Onstott, T. C., et al. (2021). Earth-like habitable environments in the subsurface of Mars. Astrobiol 21 (6), 741–756. doi:10.1089/ast.2020.2386
Templeton, A. S., and Caro, T. A. (2023). The rock-hosted biosphere. Annu. Rev. Earth Planet. Sci. 51, 493–519. doi:10.1146/annurev-earth-031920-081957
Vera, M., Schippers, A., and Sand, W. (2013). Progress in bioleaching: fundamentals and mechanisms of bacterial metal sulfide oxidation—part A. Appl. Microbiol. Biotechnol. 97, 7529–7541. doi:10.1007/s00253-013-4954-2
Viang, H., and Dong, H. (2012). Biogeochemistry and geomicrobiology in extreme environments: Preface. Geosci. Front. 3 (3), 269–271. doi:10.1016/j.gsf.2012.03.001
Vreeland, R. H., Piselli, A. F., McDonnough, S., and Meyers, S. (1998). Distribution and diversity of halophilic bacteria in a subsurface salt formation. Extremophiles 2, 321–331. doi:10.1007/s007920050075
Whelan, J., Oremland, R., Tarafa, M., Smith, R., Howarth, R., and Lee, C. (1986). Evidence for sulfate-reducing and methane-producing microorganisms in sediments from sites 618, 619, and 622. Initial Rep. deep sea Drill. Proj. 96, 767–775. doi:10.2973/dsdp.proc.96.147.1986
Whitman, W. B., Coleman, D. C., and Wiebe, W. J. (1998). Prokaryotes: the unseen majority. Proc. Nat. Acad. Sci. U. S. A. 95 (12), 6578–6583. doi:10.1073/pnas.95.12.6578
Wilkins, M., Daly, R., Mouser, P., Trexler, R., Wrighton, K., Sharma, S., et al. (2014). Trends and future challenges in sampling the deep terrestrial biosphere. Front. Microbiol. 5, 481. doi:10.3389/fmicb.2014.00481
Wu, X., Holmfeldt, K., Hubalek, V., Lundin, D., Åström, M., Bertilsson, S., et al. (2016). Microbial metagenomes from three aquifers in the Fennoscandian shield terrestrial deep biosphere reveal metabolic partitioning among populations. ISME J. 10, 1192–1203. doi:10.1038/ismej.2015.185
Wu, X., Pedersen, K., Edlund, J., Eriksson, L., Åström, M., Andersson, A. F., et al. (2017). Potential for hydrogen-oxidizing chemolithoautotrophic and diazotrophic populations to initiate biofilm formation in oligotrophic, deep terrestrial subsurface waters. Microbiome 5, 37. doi:10.1186/s40168-017-0253-y
Keywords: hard rock deep subsurface, dark biosphere, habitability, Mars, liquid water, energy
Citation: Escudero C and Amils R (2023) Hard rock dark biosphere and habitability. Front. Astron. Space Sci. 10:1203845. doi: 10.3389/fspas.2023.1203845
Received: 11 April 2023; Accepted: 25 July 2023;
Published: 04 August 2023.
Edited by:
Alberto Fairén, Spanish National Research Council (CSIC), SpainReviewed by:
Manasvi Lingam, Florida Institute of Technology, United StatesWilliam D. Orsi, Ludwig Maximilian University of Munich, Germany
Copyright © 2023 Escudero and Amils. This is an open-access article distributed under the terms of the Creative Commons Attribution License (CC BY). The use, distribution or reproduction in other forums is permitted, provided the original author(s) and the copyright owner(s) are credited and that the original publication in this journal is cited, in accordance with accepted academic practice. No use, distribution or reproduction is permitted which does not comply with these terms.
*Correspondence:Ricardo Amils, cmFtaWxzQGNibS5jc2ljLmVz