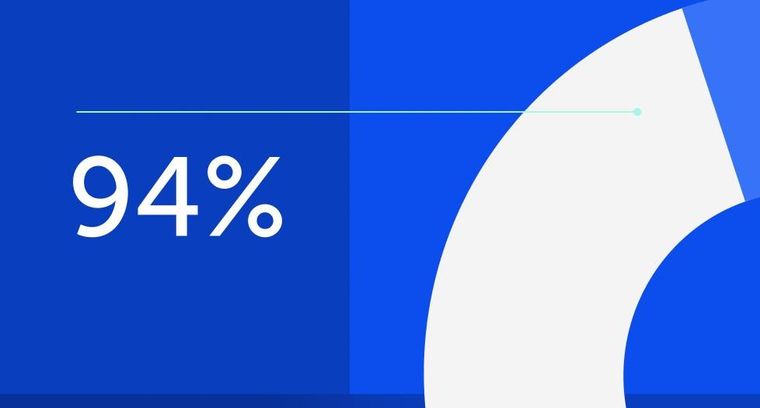
94% of researchers rate our articles as excellent or good
Learn more about the work of our research integrity team to safeguard the quality of each article we publish.
Find out more
ORIGINAL RESEARCH article
Front. Astron. Space Sci., 16 August 2023
Sec. Astrobiology
Volume 10 - 2023 | https://doi.org/10.3389/fspas.2023.1198689
This article is part of the Research TopicPresentations at the 2022 MELiSSA Conference – Current and Future Ways to Closed Life Support SystemsView all 11 articles
Bioregenerative life support systems (BLSS) are vital for long-duration and remote space missions to increase mission sustainability. These systems break down human waste materials into nutrients and CO2 for plants and other edible organisms, which in turn provide food, fresh water, and oxygen for astronauts. The central idea is to create a materially closed loop, which can significantly reduce mission mass and volume by cutting down or even eliminating disposable waste. In most BLSS studies only a fraction of the resources, such as food, are provided by the system itself, with the rest taken on board at departure or provided through resupply missions. However, for autonomous long-duration space missions without any possibility of resupply, a BLSS that generates all resources with minimal or no material loss, is essential. The goal of this study is to develop a stoichiometric model of a conceptually fully closed BLSS that provides all the metabolic needs of the crew and organisms. The MELiSSA concept of the European Space Agency is used as reference system, consisting of five interconnected compartments, each inhabited by different types of organisms. A detailed review of publicly available MELiSSA literature from 1989 to 2022 revealed that no existing stoichiometric model met the study’s requirements. Therefore, a new stoichiometric model was developed to describe the cycling of the elements C, H, O, and N through all five MELiSSA compartments and one auxiliary compartment. A compact set of chemical equations with fixed coefficients was established for this purpose. A spreadsheet model simulates the flow of all relevant compounds for a crew of six. By balancing the dimensions of the different compartments, a high degree of closure is attained at steady state, with 12 out of 14 compounds exhibiting zero loss, and oxygen and CO2 displaying only minor losses between iterations. This is the first stoichiometric model of a MELiSSA-inspired BLSS that describes a continuous provision of 100% of the food and oxygen needs of the crew. The stoichiometry serves as the foundation of an agent-based model of the MELiSSA loop, as part of the Evolving Asteroid Starships (E|A|S) research project.
Bioregenerative life support systems (BLSS) will be a key component of future long-duration space exploration, as they can reduce mission mass and volume (Imhof et al, 2017; Audas et al, 2022). A BLSS recycles human waste by feeding it to an artificial ecosystem consisting of a range of different organisms, such as higher plants, microalgae, and bacteria. These organisms gradually break down the waste and in the process provide fresh food, generate oxygen, and clean water for the crew (Nelson et al, 2010; Escobar and Nabity, 2017; Pannico et al, 2022). As a result, there is less reliance on constant resupply from Earth, which greatly reduces the material footprint needed for extended missions in space (Macelroy and Averner, 1978; Häder, 2020; Audas et al, 2022). Because of its constant fresh food provision, a BLSS also solves the problem that essential nutrients in processed and prepackaged food are deficient and degrade over time (Cooper et al, 2017; Carillo et al, 2020). Biological systems are also capable of self-repair, unlike mechanical systems that need separate systems to be repaired. Surviving organisms and tissues in a damaged BLSS can potentially recover to their original size on their own, even after significant damage (Bartsev et al, 1996).
MELiSSA is a BLSS concept developed by ESA with a consortium of 15 international partners and the involvement of approximately 50 organizations (MELiSSA Foundation, 2023). It is an artificial ecosystem consisting of five interconnected compartments inhabited by higher plants, microalgae, microorganisms, and humans (abbreviated as C1 to C5, Figure 1; Table 1). Each of the compartments has its own specific metabolic function within the entire MELiSSA loop. First, all human waste is broken down through a sequence of three bioreactor types: a thermophilic anaerobic compartment (C1), photoheterotrophic compartment (C2) and nitrifying compartment (C3). This results in nutrients and CO2 for microalgae and plants (C4a and C4b) that, in turn, provide food, oxygen, and fresh water for the crew (C5), thus closing the loop (Hendrickx and Mergeay, 2007; Clauwaert et al, 2017; Vermeulen et al, 2020a). The operational pilot plant at Universitat Autònoma de Barcelona consists of a number of connected MELiSSA compartments and demonstrates a part of the system’s entire metabolic loop (Gòdia et al, 2004; Garcia-Gragera et al, 2021). Mathematical models of the MELiSSA loop have been developed and improved since the beginning of the MELiSSA project in 1989 (MELiSSA, 1989; Dussap and Gros, 1991). Some of these models are used for predictive control, to be run alongside the actual physical system. The MELiSSA models help in understanding the dynamics of mass flow fluctuations and achieving long-term reliability of the system (Cornet et al, 2001; Ciurans Molist et al, 2020; Poulet et al, 2020). Such ecosystem models can also be used to simulate the impact of unforeseen perturbations, deliberate interventions, or design changes in the system (Volk and Rummel, 1987; Pilo Teniente, 2015; Vermeulen et al, 2019).
FIGURE 1. Overview of the MELiSSA loop with its five main compartments and a schematic overview of the different material flows. Some of these material flows have been adjusted in the stoichiometric model. See Section 3 Stoichiometric model assumptions for more details. Image by the MELiSSA Foundation.
TABLE 1. Systematic review of the stoichiometric descriptions of mass flows in the various compartments of the MELiSSA loop, as documented in publicly available literature. Only modeling studies containing at least one chemical equation are listed. “Full closure” indicates that all material outputs are utilized as inputs without the need for any external supplies. “Chemical equations” indicates the number of involved chemical equations that are factually listed in the publication. “Stoichiometrically balanced” indicates that the publication includes the stoichiometric coefficients for all fixed equations. Stoichiometric coefficients are described as dynamic when coefficients within at least one chemical equation fluctuate throughout a simulation run. The macromolecule composition is described as dynamic when the proportion of CHON elements in at least one chemical formula varies over time. C, compartment; N/A, not applicable.
To describe the material flows in an ecosystem model, the stoichiometric relations governing these flows need to be developed (Volk and Rummel, 1987; Begon and Townsend, 2021). Several authors have described mass flow models of biological life support systems (Volk and Rummel, 1987; Garland, 1989; Loader et al, 1997; Finn, 1998), but not all studies contain the underlying chemical stoichiometric equations. The stoichiometric equations describing mass flows in the MELiSSA BLSS have been published in publicly-available MELiSSA literature, both in peer-reviewed papers and research reports disseminated by the MELiSSA Foundation (Table 1). In less than half of the listed studies, all five MELiSSA compartments were modeled. The other studies describe either a smaller number of compartments, or just one. The loop is never fully closed in any of the listed studies. There are a number of reasons for this. First of all, it may indicate that certain compounds are not part of the regenerative logic of the system and are not generated within the loop, and therefore need to be fully supplied from the outside (Dussap et al, 1993; Poughon et al, 2009). Secondly, it may indicate an output that does not get regenerated, such as a residual indigestible material (Fulget et al, 1999; Poughon et al, 2000). Because of the resulting accumulation of that material, external supply is required to keep the system running. And thirdly, it may be a deliberate system design choice that only a fraction of a particular compound or resource is generated by the BLSS. Several MELiSSA studies, for example, explicitly specify that only a part of the needed food is generated by the loop (Poughon et al, 2000; Guirado and Podhajsky, 2008; Thiron, 2020). The rest is supplied externally. One of the main motivations for such a choice is the limited space that is available in common spacecraft and habitat designs to integrate bioreactors and plant growth chambers (Johnson et al, 2021; Gorce et al, 2022).
Almost three-quarters of the researched MELiSSA studies list all the stoichiometric equations of their models. The other studies limit themselves to a few selected equations as examples. The stoichiometric coefficients in the chemical equations can either be fixed beforehand, or dynamically calculated on the fly, during the simulation run. For example, in simulating the production of Limnospira indica in C4a, the elementary composition of its biomass is determined by the variable light irradiation inside the photobioreactor (Guirado and Podhajsky, 2008; Poughon et al, 2009). This then necessitates the recalculation of all subsequent stoichiometric equations that contain this dynamic empirical biomass formula.
Whenever creating a stoichiometry to describe mass flows in an ecosystem, choices need to be made about which compounds will be considered, and which level of detail will be used to describe the ecosystem’s biochemical processes (Dubitzky et al, 2011; Begon and Townsend, 2021). This choice is influenced by the level of knowledge about the individual metabolism of the different species in the ecosystem, but ultimately depends on the objective and scope of the study. In light of this, the reviewed MELiSSA studies contain varying assumptions concerning the role of different compounds such as lipids, VFAs, and CO2. This is partially due to the fact that over time the MELiSSA models became more detailed, incorporating more processes. Early MELiSSA papers assume that all lipids consumed by the astronauts are oxidized and no traces of lipids are found in the feces (Dussap and Gros, 1991; Dussap et al, 1993). This is later adjusted, and lipids become an explicit part of the feces input in C1 (Poughon et al, 2000). The mix of VFAs that is generated as output of C1 varies quite significantly between the different studies. In the earliest studies only acetic acid and butyric acid are considered (Dussap and Gros, 1991; Dussap et al, 1993), but in later studies, a broader range of VFAs is included in the stoichiometry (Poughon, 2007b).
The stoichiometric model presented in this paper provides the basis for an agent-based model (ABM) of the MELiSSA loop to study the interactions and emergent behavior of the MELiSSA system from a theoretical perspective. MELiSSA is fundamentally a complex system, and properties such as nonlinearity, stochasticity and chaos may play an important role in the overall behavior of the system, just like in any ecosystem (Bjørnstad, 2015). Because all functions in the MELiSSA loop are tightly coupled and since there are no large buffers in space (e.g., no large atmosphere or water bodies), slight variations in any of the loop’s pathways can have dramatic consequences for the entire loop (Macelroy and Averner, 1978; Poulet et al, 2018). It is precisely such tightly coupled systems that are potentially susceptible to irregular fluctuations (Bjørnstad, 2015). ABMs offer a modeling approach that helps to gain insight in such dynamics and helps to explore different design strategies for improved system robustness. ABMs are used to understand those aspects of the behavior of a system, that are not easily represented in population-level differential equations, such as variation among individuals or variation of individuals during their life cycle (Deangelis and Grimm, 2014). Such exploratory models of complex systems lead to insights about essential mechanisms and principles, a use of modeling that is different from modeling aimed at making accurate predictions (Forrest and Mitchell, 2016). MELiSSA modeling studies to date focus on predictive modeling because this is a critical step to develop a durable and reliable BLSS. The MELiSSA modelling approach is mechanistic, allowing for better understanding and improved predictions. With its exploratory approach, this study can be considered as being complementary to the existing MELiSSA modeling efforts. In order to build an ABM of MELiSSA, a stoichiometric model is needed that describes essential mass flows between all involved agents (bacteria, microalgae, higher plants and humans). The key metabolic processes in all MELiSSA compartments need to be described with enough detail to enable studying the interactions. At the same time, they need to remain abstracted enough to tlead to an efficient model and retain relevance for the objectives of the ABM which are centered around investigating the impact of heterogeneity in the system and capturing nonlinear dynamics.
The research objective of this paper is to develop a stoichiometric model of a hypothetical BLSS that provides a crew with fresh food and oxygen during long-duration space missions, without the need for any resupply. The envisioned stoichiometric model should conform to the following requirements:
• The system provides 100% of all the food and oxygen needed by the crew, and 100% of the resources needed by every other organism in the loop.
• The stoichiometric model is fully closed. Every single output is used as input, and no additional external supplies are needed during steady state.
• No kinetic limitations of the stoichiometric reactions are used. Macromolecules do not have a dynamic composition (e.g., biomass composition does not depend on light), and all stoichiometric coefficients are fixed. Such a static approach is sufficient for the current goals of the ABM of MELiSSA that is developed on top of this stoichiometry.
The current literature (listed in Table 1) has, to the authors’ knowledge, not included such a stoichiometric model of the MELiSSA loop. As described above, the loop is always considered to be partially open, primarily for practical reasons. The research reported in this paper is more theoretical in nature, focusing on the internal dynamics of hypothetically fully closed systems.
This section describes the empirical formulas, stoichiometric equations, and the setup of a static spreadsheet model.
Table 2 provides an overview of the empirical formulas of the large biomolecules. More detailed descriptions of the origin of each formula are then discussed. The empirical formulas of biomass and feces are provided for comparative purposes only, since both biomass and feces are represented as proportions of carbohydrates, proteins and lipids.
The formula for carbohydrates is the general formula for polysaccharides, used in many of the MELiSSA modeling studies (e.g., in (Dussap et al, 1993) and (Thiron, 2020)). There are, however, a range of different general formulas used for proteins in the MELiSSA literature (Fulget, 1996; Duatis et al, 2008; Thiron, 2020). Our approach was to first calculate the average protein composition of the different organisms generating biomass in our model, and then compare this result to general protein formulas published in multiple BLSS studies. In our stoichiometric model, the higher plants are represented by a generalized “ideal plant”, in line with previous MELiSSA studies (Poughon, 2007b; Guirado and Podhajsky, 2008). It is conceived as an average of the nutritional properties of bread wheat, durum wheat, potato and soybean. The CHON composition of the protein of this ideal plant is then averaged with that of Limnospira and Rhodobacter, all using values listed in MELiSSA literature (Dussap et al, 1993; Duatis et al, 2008). This results in CH1.5799O0.3134N0.2558. This composition comes very close to CH1.5900O0.3100N0.2500 used as general protein formula in studies of the Lunar Palace BLSS (Hu et al, 2010; Fu et al, 2016), and hence this latter formula was selected. Palmitic acid is often used as lipid in stoichiometries of ecosystems (Volk and Rummel, 1987; Guirado and Podhajsky, 2008). However, vegetable oils mainly consist of more complex triacylglycerols (Zambelli et al, 2015). Therefore in this stoichiometric model, lipids are represented by tripalmitin, consisting of one glycerol molecule bonded to three palmitic acid molecules. Just like with palmitic acid, the fermentation of glycerol also leads to VFAs, further contributing to the overall VFA output of C1.
Throughout the MELiSSA literature the empirical formulas of the biomass of specific organisms differ to varying degrees. The empirical formula that describes the biomass of the purple sulphur bacteria Rhodobacter varies, for example, between (Dussap et al, 1993), (Hendrickx et al, 2006), and (Thiron, 2020). This is to be expected since empirical formulas are always approximations. Moreover, in several MELiSSA modeling studies, biomass composition is dependent on environmental parameters such as light input, and consequently the biomass composition varies throughout the course of a simulation (Table 1). In the current stoichiometric model, biomass is consistently represented as a combination of carbohydrates, proteins, and lipids, and not as one single molecule. This makes it possible to trace the levels of each individual compound throughout the loop. To establish the composition of the different types of biomass, in this study an estimate was made based on averaged values from literature. As validation, the corresponding empirical formula was then compared with formulas published in MELiSSA literature.
In correspondence with the MELiSSA study of (Hendrickx et al, 2006), the same biomass composition was chosen for both Rhodospirillum rubrum and Limnospira sp. Based on literature (e.g., Dussap et al, 1993; Kobayashi and Kobayashi, 1995; Teuling et al, 2017), the following biomass composition was established (dry weight, or DW): 18% carbohydrates, 72% proteins, and 10% lipids. Both organisms contain high amounts of protein, hence their interesting potential as food sources (Vrati, 1984; Clauwaert et al, 2017; Muys et al, 2019). Using the previously established CHON compositions of carbohydrates, proteins and lipids, the overall biomass composition corresponds to the empirical formula CH1.6472O0.3598N0.1788. This is in the same range as the biomass formulas used in the MELiSSA modeling studies of (Hendrickx et al, 2006) and (Thiron, 2020).
Carbohydrates are typically the most abundant compound in plants, encompassing both structural (e.g., cellulose) and non-structural (e.g., starch and glucose) carbohydrates (Moore and Hatfield, 1994). As explained before, an ‘ideal plant’ is used in the current model. Four energy- and/or protein-rich crops were selected and combined based on (Paradiso et al, 2013): bread and durum wheat, potato and soybean. Averaging the carbohydrate, protein and lipid fractions of these four crops (Molders et al, 2012; Stasiak et al, 2012; Page and Feller, 2013; Paradiso et al, 2013), leads to the following edible plant biomass composition (DW): 70% carbohydrates, 20% proteins, and 10% lipids. Taking into account the above CHON compositions of carbohydrates, proteins and lipids, this corresponds to the empirical macroformula CH1.6889O0.6090N0.05487. This is in line with the “food” descriptions in other MELiSSA studies (Fulget, 1996; Thiron, 2020). The inedible part of the plant is considered to be 100% carbohydrates, assuming that it is only composed of cellulose.
Just like with biomass, feces are expressed in the model as a combination of carbohydrates, proteins and lipids. The composition of feces was calculated by stoichiometrically balancing the equation for human metabolism (see 5.5). The proportions of carbohydrates, proteins and lipids as reported in literature [review in (Rose et al, 2015)] were used as a guideline to solve the stoichiometry. The resulting empirical formula is CH1.6676O0.6028N0.07715.
As a general method to balance the equations that represent the reactions occurring in each compartment, each one is stated as a linear programming problem–or non-linear when the constraints of the particular reaction so require–where the goal is to minimize the total amount of matter used. The variables are defined as the number of moles of each compound on either side of the equation. The objective function is subject to the linear constraint of keeping the number of atoms of a given element equal on both sides of the equation. Additionally for each problem ad hoc constraints are described e.g., to keep proportions of VFA’s produced similar to experimental data on C2. The solving engine for these problems is MS Excel’s built-in optimization tool. For linear problems Simplex is used and for non-linear problems GRN non-linear, both on their default configurations settings. In reporting throughout this paper 4 significant decimals are used, but during calculations in Excel 14 decimals were used.
This stoichiometry is a full revision of a version of that was concisely published in two IAC conference papers (Vermeulen et al, 2018b; 2019) and presented at two MELiSSA conferences (Vermeulen et al, 2018a; 2020b). The revisions were based on a detailed validation session and on further literature study. The main changes were the following:
• All biomass compositions were re-evaluated (Limnospira, Rhodospirillum and higher plants) to more accurately represent their carbohydrate, protein and lipid content.
• No more distinction between fecal and bacterial protein, in line with the singular use of carbohydrates and lipids in the stoichiometry.
• The VFA spectrum was extended beyond acetic acid and butyric acid.
• Instead of representing urine as NH3, it is now represented as urea, in order to bring carbon into the urine cycling. Hence, a new subsystem with its own stoichiometry representing the breakdown of urea was added.
To calculate the steady state flow values for each stream, each compartment’s equation is linked, thus building an equation based model that represents the whole cycle. To solve it, the one a priori known value is used: the consumption rate of the crew compartment, which has been set at 3,000 kcal/day/person. Knowing these requirements for C5, the required food outputs of C4a and C4b are calculated. By continuing to work backwards through the entire stoichiometry, the flow rates through the entire loop could be established over a period of 1 day for a crew of six.
Since there is a subcycle between C1 and C2 the exchange flows between them need to be calculated (Figure 2). Having the required steady state flows into C1, and out of C2 (except the subcycle flows into each other), the process of exchange between C1 and C2 can be examined. This requires an iterative approach in which, given an initial output of VFAs from C1 to C2, a subsequent C2 to C1 flow of biomass is calculated. This, in turn, updates the flow from C1 to C2 again. This eventually converges into a stable exchange between C1 and C2, with complete processing of all VFAs. This then results in cycle-wide steady state flow rates.
FIGURE 2. Diagram representing the subcycle between C1 and C2, and the process of converging to a stable exchange of VFAs and biomass between the two compartments.
This section describes the general assumptions behind the model and those per compartment.
This stoichiometry is based on the four CHON elements; sulfur and phosphor are not yet included. Throughout the entire loop, food and feces are described as a proportion of carbohydrates, proteins and lipids. The water in this stoichiometric model is what is used in the chemical reactions. The actual water needs of a human crew (drinking and hygiene water) have not been included. Net biomass production only occurs in C2, C4a, and C4b because this biomass is a material input for other compartments. For the other compartments it is assumed there is no growth, and hence no net biomass production occurs. This has an impact on the stoichiometric approach for each compartment, which differs when describing a growth metabolism vs. a maintenance metabolism without growth (Cruvellier et al, 2016). It is further assumed that all waste materials are fully digested in C1, and no undigestible residues are left. And there is no limitation in terms of available space and hence the number of bioreactors and growth chambers that can be included.
Several adjustments have been made to the official concept of the MELiSSA loop, as illustrated in Figure 1. They can be summarized as follows (more details further down in this section):
• The Rhodospirillum biomass that is produced in C2 is not used for human consumption, but is sent to C1 where it is anaerobically digested, together with the other organic waste.
• C2 does not produce any net CO2.
• Urea is included as an individual waste product and is processed by C3.
• C3 consists of two subcompartments, C3a and C3b, to respectively process ammonia and urea.
• An auxiliary compartment has been added to burn surplus hydrogen and generate water.
In C1, the thermophilic anaerobic bacteria can convert up to 70% of the waste under experimental conditions (Lasseur et al, 2010). This is because cellulose and hemicellulose can only be hydrolyzed slowly, further hampered by the fact that both are often intermeshed with lignin in lignocellulose, which is even more difficult to break down (Liebetrau et al, 2017). In this model it is assumed that all organic waste is fully converted in C1. In the MELiSSA system up to 71% of the organic nitrogen in the waste is converted into ammonium (Clauwaert et al, 2017). In our stoichiometric model it is assumed that this is 100%. The VFA spectrum produced by C1 consists of four VFAs: acetic acid, propionic acid, butyric acid and valeric acid. These VFAs are characteristically produced during acidogenesis (Jiang et al, 2013; Khan et al, 2016).
In the original conceptualization of MELiSSA, it was assumed that in addition to the microalgae and crops from C4, the edible purple sulfur bacteria from C2 would also serve as a food supply for the crew (Lasseur et al, 1996; Albiol et al, 2000; Figure 1). However, because of close proximity, there is always a chance that pathogens associated with fecal matter in C1 pass through membrane filtration and end up with the edible bacteria in C2 (Clauwaert et al, 2017). Therefore, the produced biomass from this compartment is sent back to C1 for anaerobic degradation. This is in correspondence with other MELiSSA modeling studies (Poughon et al, 2000; Waters et al, 2004; Thiron, 2020). The overall diet of the crew should only contain a limited amount of microbial single-cell protein, because of increased nucleic acid contents and lower palatability (Tusé and Miller, 1984; Tranquille and Emeis, 1996). The crew is already using another microbial food source (Limnospira from C4a, Figure 1), and this might be an additional argument to avoid consuming the bacteria from C2. Theoretically, the assimilation of the VFAs by purple sulfur bacteria in C2 could generate a net CO2 output (Hendrickx and Mergeay, 2007). However, in practice, this has not been observed yet in MELiSSA bioreactor experiments with C1 effluent as VFA source (Mastroleo et al, 2020).
Analogous to C4, this compartment also consists of two subcompartments, C3a and C3b. C3a processes ammonia and produces nitrate. C3b is a urine treatment unit based on C3a, and also produces nitrate. It is assumed that all incoming ammonia and urea are converted to nitrate.
The assumptions about the human metabolism are summarized in Table 3. The reference caloric value of the higher plants is estimated to be 4,000 kcal/kg dry weight (DW). This is based on the reported caloric values of the four crops that constitute the ideal plant (adjusted for their moisture content): bread wheat and durum wheat (Kaleta and Górnicki, 2013; Knowledge4 Policy, 2021; WebMD, 2021), potato (Decker and Ferruzzi, 2013; U.S. Food & Drug Administration, 2017), and soybean (Food Data Central, 2019b). The reference caloric value of Limnospira (commercially available as Spirulina) is estimated to be 3,000 kcal/kg DW (Food Data Central, 2019a). Solid output of the human metabolism is described as feces and urea. All other body wastes such as dead skin cells, sweat solids, hair, nails, etc. (Hu et al, 2010) are assumed to be part of the feces. H2O is only integrated in the stoichiometry as a metabolite, and as such the amounts do not represent actual water consumption. It is assumed that the anabolism and catabolism of the crew are in dynamic equilibrium, and that the crew neither gains or loses weight.
This section describes the results of the stoichiometry development, and the simulation results.
For each compartment a set of chemical equations with fixed stoichiometric coefficients has been developed, making sure that all types of output are also used as input in the loop, thus enabling full closure. The equations are partially based on existing equations from the MELiSSA literature, but were tailored to serve the needs of this study based on additional literature.
The first compartment of the MELiSSA loop is an anaerobic digester with a consortium of thermophilic bacteria that receives fecal material and other wastes, such as inedible plant material (Michel et al, 2005; Mastroleo, 2009; Poughon et al, 2013). Anaerobic digestion is a natural process that typically consists of four subsequent stages: hydrolysis, acidogenesis, acetogenesis and methanogenesis. Volatile fatty acids are intermediate products in this process, while methane is the main end product (Hendrickx and Mergeay, 2007; Khan et al, 2016). In the MELiSSA system a partial anaerobic transformation is used with a maximal yield of volatile fatty acids (VFAs), all while avoiding the production of methane. The VFAs are useful biomolecules that can be utilized as nutrients for other microorganisms downstream in the MELiSSA loop. Such partial anaerobic digestion is achieved by operating the bioreactor under slightly acidified conditions (pH 5.3) which inhibits methanogenic activity (Mastroleo, 2009; Clauwaert et al, 2017).
Organic waste typically consists of polymers (carbohydrates and proteins), as well as fats (Liebetrau et al, 2017). These cannot be taken up by the microbial cells and have to be broken down into smaller compounds first. During hydrolysis, bacteria excrete hydrolytic enzymes which split these substances into sugars, amino acids, fatty acids, and glycerol. These water-soluble hydrolysis products are then converted into volatile fatty acids, CO2 and hydrogen during anaerobic fermentation (or acidogenesis) (Lauwers et al, 2013; Liebetrau et al, 2017). C1 receives input from three different sources: feces from the crew, inedible plant material, and biomass from C2. The next paragraphs describe the stoichiometries of the fermentation of, respectively, carbohydrates, proteins, and lipids.
Carbohydrate fermentation in the MELiSSA loop has been described in multiple studies, and results in the production of VFAs, CO2 and hydrogen. In Hendrickx et al (2006) only one generic formula representing all VFAs is used, while in all the other consulted MELiSSA studies just two VFAs are produced (e.g., Dussap et al, 1993; Poughon et al, 2009). In some studies this is complemented with the production of bacterial biomass (Guirado & Podhajsky, 2008; Thiron, 2020). As stated above, in the current stoichiometry, four VFAs are used and no biomass production is needed in the equation of this compartment. As a consequence, a new stoichiometry had to be calculated. Proportions of acetic acid, propionic acid, butyric acid and valeric acid were used from (Yin et al, 2016), describing the anaerobic fermentation of glucose as model carbohydrate at pH 6. In below equation, the proportions of these four VFAs were used since the MELiSSA C1 bioreactor also operates at a low pH of 5.3 (Clauwaert et al, 2017). CO2 and hydrogen are produced in equal proportions, in line with other published balanced carbohydrate fermentation reactions (Dussap et al, 1993; Yin et al, 2016).
Just like with carbohydrates, there are several stoichiometric descriptions of the fermentation of proteins in the MELiSSA literature. Older studies use acetic acid and butyric acid as VFA output (e.g., Dussap & Gros, 1991), subsequent studies use up to 5 VFAs (e.g., Guirado & Podhajsky, 2008; Thiron, 2020). In these latter studies, microbial biomass is also included as an output. As biomass output is not necessary in this part of our stoichiometric model, and as a slightly differing empirical formula for protein is used, a new stoichiometric balance needs to be calculated. Proteins are polymers consisting of amino acids. During anaerobic fermentation, proteins are first broken down into their individual amino acids. The subsequent fermentation of these amino acids can be described using a single reaction step, and results in the production of VFAs, CO2, hydrogen and ammonia (Ramsay and Pullammanappallil, 2001; Poughon et al, 2009). In stoichiometric equations, CO2, hydrogen and ammonia are generally being produced in a similar order of magnitude (Poughon, 2007b; Thiron, 2020). Tepari (2019) investigated protein fermentation during anaerobic wastewater treatment, and used bovine serum albumin (BSA) as model protein. At pH 5, only acetic acid (37.4%), propionic acid (13.7%) and butyric acid (48.9%) were produced, and no valeric acid. These proportions were used to calculate the following stoichiometric equation:
As described above, tripalmitin is used as model lipid in this model. Since in previous MELiSSA modeling studies, palmitic acid is used, a new stoichiometric equation has to be calculated. When lipids such as triacylglycerols are anaerobically digested, they are first hydrolyzed to glycerol and long-chain fatty acids (LCFAs) (Mackie et al, 1991; Li et al, 2002; Cirne et al, 2007). Subsequently, the glycerol is degraded into VFAs, while the LCFAs are broken down into acetic acid and hydrogen through beta-oxidation (Weng and Jeris, 1976; Ceron-Chafla et al, 2021; Holohan et al, 2022). Firstly, the glycerol and LCFA fermentations will be individually described, after which they will be integrated into one equation.
The hydrolysis of tripalmitin can be written as follows:
In experiments by Yin et al, 2016, glycerol was subjected to (non-strict) anaerobic fermentation at pH 6. The study presents three individual stoichiometric reactions, each describing the degradation of glycerol into acetic acid, propionic acid, and butyric acid. The experiment resulted in a production of 32% acetic acid, 52% propionic acid, and 16% butyric acid. Integrating the three individual stoichiometric reactions, taking into account the above mentioned proportion, leads to the following single equation:
The stoichiometric equation for palmitic beta-oxidation is described by Li et al (2018):
The purpose of MELiSSA C2 is to recycle the VFAs produced in C1. The purple non-sulphur phototrophic bacteria Rhodospirillum rubrum is utilized for this. Under anaerobic conditions, it uses light as an energy source to convert VFAs into biomass (Mastroleo, 2009; De Meur et al, 2020). Because we use a custom composition of Rhodospirillum biomass in this model, a new stoichiometry had to be calculated. Anaerobic digestion of organic matter may lead to fluctuating proportions of different VFAs (Alloul et al, 2018). Because of the variable VFA spectrum coming out of C1, it was decided to develop a individual equation for each VFA. The assimilation process consumes CO2 and NH3, and for acetic acid also hydrogen (Dussap et al, 1993; Fulget, 1996; Thiron, 2020). As explained above, no net CO2 production occurs in the following equations.
In some cases, ammonia and urea can be taken up directly by plants or microorganisms grown for food production. However, in a BLSS it is desirable to convert ammonia and urea to nitrate because it is a less volatile and less reactive molecule (Clauwaert et al, 2017; De Paepe et al, 2018; Sachdeva et al, 2021). MELiSSA C3 receives ammonia from C2 and urine from C5, and transforms both into nitrate for the plants and microalgae in C4 (Duatis and Moreno, 2009; 2010). Ammonia is processed in a nitrifying bioreactor, while urine is processed separately in a modified version of the nitrifying bioreactor, the so-called Urine Treatment Unit (UTU) (Guirado and Podhajsky, 2008). As both bioreactors process different compounds, two separate compartments are defined in the stoichiometric model: 3a and 3b.
The nitrifying bioreactor as defined in the MELiSSA project is a cylindrical packed-bed bioreactor with polystyrene beads that are colonized by a biofilm of Nitrosomonas europaea and Nitrobacter winogradsky (Cruvellier et al, 2016; Garcia-Gragera et al, 2021). The nitrification of ammonia happens in two steps, each step carried out by one of the bacterial species (Cruvellier et al, 2016):
Taken together this results in:
In a recent MELiSSA study of a UTU concept (Christiaens et al, 2019), showed how human urine can be nitrified with the use of a synthetic microbial community of ureolytic heterotrophic bacteria and nitrifying bacteria cultivated in a continuous stirred tank reactor. First, during ammonification, the ureolytic heterotrophic bacteria break down urea into ammonia and CO2, a process catalyzed by the enzyme urease (Nicolau et al, 2010; De Paepe et al, 2018). Subsequently, the ammonia is further converted into nitrate by the nitrifying bacteria as described above. The stoichiometric equation describing the ammonification of urea can be found in (Guirado and Podhajsky, 2008):
Taken together with (13), this results in:
The edible microalgae Limnospira platensis and indica (previously Arthrospira platensis and indica) are cyanobacteria that consume nitrate and CO2 that are produced in other compartments of the loop, and generate oxygen and food for the crew (Clauwaert et al, 2017; Alemany et al, 2019; Sachdeva et al, 2021). Limnospira is grown inside a gas lift photobioreactor with illumination and a gas injection system (Garcia-Gragera et al, 2021; Poughon et al, 2021). Since a custom biomass composition is used in our model, a new stoichiometric balance had to be calculated (generated biomass is dry mass):
Edible crops are grown in C4b of the MELiSSA loop (Pannico et al, 2022). The goal of this compartment is in line with that of C4a: recycling nitrate and CO2, and producing oxygen and food (Lasseur et al, 2010; Peiro et al, 2020). In the current model, a harvest index of 50% is used, which means that half of the plant biomass is edible, while the other half is not. The non-edible part is considered as waste and is used as feed for the thermophilic anaerobic bacteria in C1. This can be summarized in the general equation:
This corresponds to the following stoichiometric equation (generated biomass is dry mass):
The general equation for the human metabolism can be summarized as follows (Guirado and Podhajsky, 2008):
To determine the final stoichiometric equation, a few preparatory calculations are needed. Firstly, the right proportion between edible higher plant biomass and Limnospira biomass needs to be established. In the model, 90% of the caloric content of the food comes from the higher plants, and 10% comes from Limnospira. Higher plants and Limnospira are considered to have a caloric content of respectively 4,000 and 3000 kcal/kg. This amounts to a target daily food intake of 675 g DW (27.86 mol) of higher plant biomass and 100 g DW (4.56 mol) of Limnospira biomass per crew member. Using the biomass compositions, this can then be translated into corresponding values for carbohydrates, proteins, and lipids. For oxygen, CO2, and urea, reported daily values were transformed into moles (Table 2), and this was then used for calculating a stoichiometry with realistic proportions between these compounds and biomass uptake. The amount of feces is calculated by solving the stoichiometric equation. Below equation describes the metabolism of one crew member:
One auxiliary process has been added to the loop with the function to burn any excess hydrogen. This is in correspondence with other MELiSSA modeling studies such as (Poughon et al, 2000).
The results of the stoichiometric spreadsheet model running in steady state conditions can be found in Table 4. The overall input and output values of all compounds in the loop are listed, for a crew of six over a period of 1 day (the time unit used in the ABM for which this stoichiometry was developed). All different stoichiometric equations were successfully balanced with each other, which resulted in a very high closure level with 12 out of 14 values reaching full closure, with no material loss. Oxygen displays an almost neglectable loss of 0.00021%, and CO2 0.00001%. This is assumed to be caused by the numerical limitations of the software tools (e.g., number of decimals and rounding). The values correspond to respectively 2.13 mg O2 and 1.47 mg CO2 material loss per person per day. Over the course of a year, this would amount to 4.67 g O2 and 3.21 g CO2 for the entire crew. The VFA spectrum (weight-based) at steady state consists of 57.20% acetic acid, 12.59% propionic acid, 29.98% butyric acid and 0.23% valeric acid.
TABLE 4. Input and output values of the different compounds, after reaching steady state. Values for a crew of six, over a period of 1 day.
This study presents a stoichiometric model of the MELiSSA BLSS, which describes the main metabolic processes in all compartments using a series of static chemical equations. In steady state, 100% of the necessary food and oxygen for the crew, as well as 100% of the compounds required for all other organisms in the loop, are generated. Using the assumption that all waste compounds in the loop can be broken down again, full closure was achieved in the static model by balancing the size of each compartment relative to all the other compartments. This is the first time a fully closed static MELiSSA model is described that can provide all the food and oxygen for astronauts. The model is a useful tool for theoretical research on autonomous BLSSs for long-duration space exploration, allowing tracing of the elements over the entire cycle.
However, in practice, no system can be 100% closed. There are always potential outfluxes from the system, for example, through a buildup of recalcitrant organic matter (Hendrickx and Mergeay, 2007; Lasseur et al, 2010; Zhang et al, 2018) or the accumulation of different precipitates such as carbonates and phosphates (De Paepe et al, 2018; Christiaens et al, 2019). Recalcitrant organic matter consists mainly of plant fibers that do not rapidly biodegrade. Plant cell walls are composed of cellulose, hemicellulose, pectin, and lignin and are among the least degradable polymers in a BLSS (Hendrickx and Mergeay, 2007; Zhang et al, 2018). As a result, up to about 70% of the organic waste could be digested in MELiSSA lab experiments (Farges et al, 2008; Lasseur et al, 2010). The MELiSSA community has investigated additional methods to break down the remaining recalcitrant matter using hydrothermal and chemical oxidation, and anaerobic and hyperthermophilic cellulose-degrading bacteria (Hendrickx & Mergeay, 2007; Lasseur et al, 2010). The most efficient results were obtained by using supercritical water oxidation (SCWO) in which up to 98% of all organic matter could be broken down (Hendrickx and Mergeay, 2007; Zhang et al, 2018). Hydrogen peroxide was used as an oxidizer in these tests, which is a compound that can be generated within a BLSS loop (Tikhomirov et al, 2011; Vijapur et al, 2017; Nelson et al, 2020). Nevertheless, it is safe to assume that, in reality, there will always be some level of material loss in any BLSS loop. It would therefore be interesting to investigate what accumulates over a long period of time and then calculate the dimensions of the reserve storage required to mitigate the resulting material shortages.
The interaction between compartments C1 and C2 represents a subcycle in this model. The Rhodospirillum biomass generated in C2 is fed back into C1 as organic waste. The goal of this subcycle is to produce a surplus of CO2, H2, and NH3 that is needed in the other compartments of the MELiSSA loop. Steady state of this subcycle is reached gradually until enough biomass from C2 is sent back to C1 to ensure that the exact amount of VFAs is produced to sustain a stable Rhodospirillum culture. If too few VFAs are provided, C2 will underperform due to a lack of Rhodospirillum growth. If too many VFAs are provided, the Rhodospirillum culture will not be able to process all of them, leading to accumulation and negative impacts on the mass flows and efficiency of the entire loop. At steady state, this subcycle generates the following surplus (per day, for a crew of six): 213 mol CO2, 434 mol H2, and 126 mol NH3. The subcycle also explains the dominant amount of protein present in the loop, despite the food provided to the crew containing much less protein (Table 4). Due to the need for a significant mass of Rhodospirillum to process the VFAs and their high protein content (72%), a lot of protein is present in the loop in general. Bio-electrochemical oxidation is a potentially more efficient approach to convert the VFAs into CO2. In a study by Luther et al, 2018, using bio-electrochemical oxidation within a microbial electrolysis cell, 80%–100% of the VFAs in the effluent of MELiSSA’s C1 were converted into CO2 in 7 days. With further process development and optimization, bio-electrochemical oxidation can potentially entirely replace the use of Rhodospirillum in C2.
(Jiang et al, 2013) conducted a series of experiments on anaerobic digestion of food waste. At a pH of 5.0, the resulting VFA spectrum consisted of 60.40% acetic acid, 8.32% propionic acid, 31.13% butyric acid, and 0.15% valeric acid, which falls within the same range as the results achieved in our model at steady state. Another study by Lim et al, 2008 also involved anaerobic digestion of food waste. At a pH of 5.5, the resulting VFA spectrum had a more variable composition, but acetic acid was generally the dominant VFA. These reported results are consistent with the results of our static model, which suggests that the theoretical VFA outcome is a potentially realistic scenario. However, it must be noted that VFA spectra generated during anaerobic fermentation are highly variable and depend on various factors such as substrate composition and inoculum (Poughon et al, 2013; Khan et al, 2016), pH and temperature (Shin et al, 2004; Jiang et al, 2013; Khan et al, 2016), and bioreactor design and operation (Lim et al, 2008; Bharathiraja et al, 2016). For instance, in experiments using food waste by Shin et al (2004), and in MELiSSA experiments using a synthetically composed organic waste (Luther et al, 2018; Zhang et al, 2019), both acetic acid and butyric acid were the main VFAs, with butyric acid being dominant.
Growing enough plants to meet the food requirements of a human crew typically results in the production of more oxygen than the crew needs. This is because the harvest index of the crops is often less than 100%, meaning that only a portion of the grown biomass is used for food (Jones, 2003). However, the unused biomass still generates oxygen. In a closed loop system, this could lead to an accumulation of excess oxygen (as e.g., observed in Poughon et al, 2000; Gros et al, 2003) and, eventually, the collapse of the system. In our model, the excess oxygen is used to hydrolyze solid waste in C1, in the form of H2O. The hydrogen-burning auxiliary helps to transform any remaining oxygen gas into H2O. Since oxygen originates from splitting H2O during photosynthesis, the amount of surplus oxygen matches the amount of surplus hydrogen in the system.
In our model, the human crew consumes 21.51 mol/person/day of CO2, which is very close to the target value of 22.72 mol/person/day used for calculating the stoichiometry. However, the human crew only uses 24.29 mol/person/day of oxygen, much lower than the target value of 52.50 mol/person/day. The proportion between consumed oxygen and produced CO2 should be 2.31, but in our model, this proportion is 1.13, precisely because of the lower oxygen consumption. Remarkably, other BLSS modeling studies show the same tendency. In MELiSSA studies, the proportion is 1.20 (Fulget, 1996; Thiron, 2020) and in Lunar Palace studies, it ranges from 1.13 to 1.38 (Hu et al, 2010; Fu et al, 2016). This suggests that the description of the O2 consumption in all of these studies is not entirely complete. This could be due to the fact that in reality, more compounds are oxidized than represented in the simplified stoichiometric equation for human metabolism. The total mass of consumed food per person per day corresponds to 781 g DW/day. This is slightly higher than the range of baseline values used in MELiSSA studies and reference documents. Adjusted to a daily food intake of 3,000 kcal/person, the values in these studies and documents range from 509 to 748 g DW/day (e.g. (Dussap et al, 1993; Poughon et al, 2000; Thiron, 2020)).
The composition of feces was calculated by solving the stoichiometric equation for human metabolism, using the general characterization of feces reported in the literature as a starting point (Rose et al, 2015). After solving the equation for human metabolism, the resulting feces composition was found to be 66% carbohydrates, 28% proteins, and 6% lipids, corresponding to the empirical formula CH1.6676O0.6028N0.07715. This formula is similar to the one used in BLSS studies by Hu et al, 2010 and Fu et al, 2016, which is CH1.70O0.60N0.05. Because the MELiSSA diet is a vegan diet, it is expected that there is a high fiber intake and a resulting high amount of fiber in the feces (Brodribb et al, 1980; Kay, 1982; Forsum et al, 1990). As a result, the overall carbohydrate fraction could be quite significant. It should be noted that the fraction of protein in the feces could be an underestimation, as bacteria can compose up to half of the fecal solids and may have a significant protein content (Cummings, 2001; Rose et al, 2015). Intestinal bacterial growth is not included in the human stoichiometry, which is justifiable as it means more digestion is done by the same groups of microbes in C1. The amount of feces produced is 253 g DW/person/day. Jenkins et al, 2001 measured 160 ± 24 g DW/person/day for a vegetarian diet. It should be noted that no other solid body wastes have been included in the stoichiometry, and as such the ‘feces’ in the equation represent a more general representation of all wastes combined.
Further development of the stoichiometric model could focus on increasing its granularity. This could involve expanding the model to include more elements beyond CHON, incorporating a greater diversity of crops, and using a more detailed description of human metabolism. Nitrogen, phosphorus, and potassium are the three major macro-elements for plants (Usherwood and Segars, 2001; Kanazawa et al, 2008). Therefore, including the latter two elements in the next version of the model seems like a logical step. Fertilizers typically contain these three elements, but it is crucial that they are applied in the correct ratio (Usherwood and Segars, 2001; Chun et al, 2017), which must also be factored into the model. Diverse plant crops could be used instead of the concept of an ‘ideal plant’, allowing for a more detailed description of the crew’s daily diet. Integrating water usage beyond it's role as a metabolite will result in a more accurate description of the entire water budget. For example, water transpiration, water excretion, and free water inside the plant could be factored in. By integrating solid body wastes other than feces, the stoichiometry of human metabolism could also be further refined.
The raw data supporting the conclusion of this article will be made available by the authors, without undue reservation.
AV and AP conceived and designed the study and performed the MELiSSA literature review. AV did the background research for the stoichiometry, while AP developed the tool in Excel to balance the chemical equations. The stoichiometric spreadsheet model was created in Excel by AP. AV wrote the first draft of the manuscript, and AP contributed sections. All authors contributed to the article and approved the submitted version.
The first, and shorter, version of the stoichiometry was compiled in collaboration with Jason Kiem from SmartCrops BV. Prof. Siegfried Vlaeminck from the University of Antwerp provided validation of this initial version and offered suggestions for further development. The authors would like to express their gratitude to Christophe Lasseur and Christel Paille from MELiSSA at ESTEC, as well as Prof. Claude-Gilles Dussap from Polytech Clermont, for providing input and feedback throughout the research. Ralph Lindeboom from Delft University of Technology also provided valuable feedback on a previous version of the manuscript.
The authors declare that the research was conducted in the absence of any commercial or financial relationships that could be construed as a potential conflict of interest.
All claims expressed in this article are solely those of the authors and do not necessarily represent those of their affiliated organizations, or those of the publisher, the editors and the reviewers. Any product that may be evaluated in this article, or claim that may be made by its manufacturer, is not guaranteed or endorsed by the publisher.
Albiol, J., Gòdia, F., Montesinos, J., Perez, J., Vernerey, A., Cabello, F., et al. (2000). Biological life support system demostration facility: the melissa pilot plant. SAE Tech. Pap. doi:10.4271/2000-01-2379
Alemany, L., Peiro, E., Arnau, C., Garcia, D., Poughon, L., Cornet, J.-F., et al. (2019). Continuous controlled long-term operation and modeling of a closed loop connecting an air-lift photobioreactor and an animal compartment for the development of a life support system. Biochem. Eng. J. 151, 107323. doi:10.1016/j.bej.2019.107323
Alloul, A., Ganigué, R., Spiller, M., Meerburg, F., Cagnetta, C., Rabaey, K., et al. (2018). Capture–ferment–upgrade: a three-step approach for the valorization of sewage organics as commodities. Environ. Sci. Technol. 52, 6729–6742. doi:10.1021/acs.est.7b05712
Audas, C., Ortega Ugalde, S., Paille, C., Lamaze, B., and Lasseur, C. (2022). Life support systems beyond low Earth orbit advocates for an improved resources management approach. Ecol. Eng. Environ. Prot. 2022, 5–13. doi:10.32006/eeep.2022.1.0513
Bartsev, S. I., Gitelson, J. I., Lisovsky, G. M., Mezhevikin, V. V., and Okhonin, V. A. (1996). Perspectives of different type biological life support systems (BLSS) usage in space missions. Acta Astronaut. 39, 617–622. doi:10.1016/S0094-5765(97)00012-X
Begon, M., and Townsend, C. R. (2021). Ecology: from individuals to ecosystems. New Jersey, United States: John Wiley & Sons.
Bharathiraja, B., Sudharsanaa, T., Bharghavi, A., Jayamuthunagai, J., and Praveenkumar, R. (2016). Biohydrogen and Biogas – an overview on feedstocks and enhancement process. Fuel 185, 810–828. doi:10.1016/j.fuel.2016.08.030
Bjørnstad, O. N. (2015). Nonlinearity and chaos in ecological dynamics revisited. Proc. Natl. Acad. Sci. 112, 6252–6253. doi:10.1073/pnas.1507708112
Brodribb, J., Condon, R. E., Cowles, V., and DeCosse, J. J. (1980). Influence of dietary fiber on transit time, fecal composition, and myoelectrical activity of the primate right colon. Dig. Dis. Sci. 25, 260–266. doi:10.1007/BF01308515
Carillo, P., Morrone, B., Fusco, G. M., De Pascale, S., and Rouphael, Y. (2020). Challenges for a sustainable food production system on board of the international space station: a technical review. Agronomy 10, 687. doi:10.3390/agronomy10050687
Ceron-Chafla, P., Chang, Y., Rabaey, K., van Lier, J. B., and Lindeboom, R. E. F. (2021). Directional selection of microbial community reduces propionate accumulation in glycerol and glucose anaerobic bioconversion under elevated pCO2. Front. Microbiol. 12, 675763. doi:10.3389/fmicb.2021.675763
Christiaens, M. E. R., De Paepe, J., Ilgrande, C., De Vrieze, J., Barys, J., Teirlinck, P., et al. (2019). Urine nitrification with a synthetic microbial community. Syst. Appl. Microbiol. 42, 126021. doi:10.1016/j.syapm.2019.126021
Chun, J.-H., Kim, S., Arasu, M. V., Al-Dhabi, N. A., Chung, D. Y., and Kim, S.-J. (2017). Combined effect of Nitrogen, Phosphorus and Potassium fertilizers on the contents of glucosinolates in rocket salad (Eruca sativa Mill). Saudi J. Biol. Sci. 24, 436–443. doi:10.1016/j.sjbs.2015.08.012
Cirne, D. G., Paloumet, X., Björnsson, L., Alves, M. M., and Mattiasson, B. (2007). Anaerobic digestion of lipid-rich waste—effects of lipid concentration. Renew. Energy 32, 965–975. doi:10.1016/j.renene.2006.04.003
Ciurans Molist, C., Bazmohammadi, N., Vasquez, J. C., Dussap, C.-G., Guerrero, J., and Gòdia, F. (2020). Hierarchical control of space closed ecosystems - expanding microgrid concepts to bioastronautics. IEEE Ind. Electron. Mag. 15, 16–27. doi:10.1109/MIE.2020.3026828
Clauwaert, P., Muys, M., Alloul, A., De Paepe, J., Luther, A., Sun, X., et al. (2017). Nitrogen cycling in Bioregenerative Life Support Systems: challenges for waste refinery and food production processes. Prog. Aerosp. Sci. 91, 87–98. doi:10.1016/j.paerosci.2017.04.002
Cooper, M., Perchonok, M., and Douglas, G. L. (2017). Initial assessment of the nutritional quality of the space food system over three years of ambient storage. npj Microgravity 3, 17. doi:10.1038/s41526-017-0022-z
Cornet, J.-F., Dussap, C. G., and Gros, J.-B. (1998). “Kinetics and energetics of photosynthetic micro-organisms in photobioreactors,” in Bioprocess and algae reactor Technology, apoptosis advances in biochemical engineering biotechnology (Berlin, Heidelberg: Springer), 153–224. doi:10.1007/BFb0102299
Cornet, J.-F., Dussap, C. G., and Leclercq, J.-J. (2001). “Simulation, design and model based predictive control of photobioreactors,” in Engineering and Manufacturing for biotechnology focus on biotechnology. Editors M. Hofman, and P. Thonart (Dordrecht: Springer Netherlands), 227–238. doi:10.1007/0-306-46889-1_15
Cornet, J.-F., Favier, L., and Dussap, C.-G. (2003). Modeling stability of photoheterotrophic continuous cultures in photobioreactors. Biotechnol. Prog. 19, 1216–1227. doi:10.1021/bp034041l
Cornet, J. F., and Dussap, C. G. (2000). Kinetic and stoichiometric analysis of Rhodospirillum rubrum growth under carbon substrate limitations in rectangular photobioreactors. MELiSSA Technical Note 45.5. Laboratoire de Génie Chimique et Biochimique Available at: https://www.melissafoundation.org/download/469 (Accessed March 23, 2023).
Cruvellier, N., Poughon, L., Creuly, C., Dussap, C.-G., and Lasseur, C. (2016). Growth modelling of Nitrosomonas europaea ATCC® 19718 and nitrobacter winogradskyi ATCC® 25391: a new online indicator of the partial nitrification. Bioresour. Technol. 220, 369–377. doi:10.1016/j.biortech.2016.08.090
Cummings, J. (2001). “The effect of dietary fiber on fecal weight and composition,” in CRC handbook of dietary fiber in human nutrition. Editor G. Spiller (Florida, United States: CRC Press), 183–252. doi:10.1201/9781420038514.ch4.4
De Meur, Q., Deutschbauer, A., Koch, M., Bayon-Vicente, G., Cabecas, P., Ruddy, W., et al. (2020). New perspectives on butyrate assimilation in Rhodospirillum rubrum S1H under photoheterotrophic conditions. BMC Microbiol. 20, 126. doi:10.1186/s12866-020-01814-7
De Paepe, J., Lindeboom, R. E. F., Vanoppen, M., De Paepe, K., Demey, D., Coessens, W., et al. (2018). Refinery and concentration of nutrients from urine with electrodialysis enabled by upstream precipitation and nitrification. Water Res. 144, 76–86. doi:10.1016/j.watres.2018.07.016
Deangelis, D., and Grimm, V. (2014). Individual-based models in ecology after four decades. F1000prime Rep. 6, 39. doi:10.12703/P6-39
Decker, E. A., and Ferruzzi, M. G. (2013). Innovations in food chemistry and processing to enhance the nutrient profile of the white potato in all forms. Adv. Nutr. 4, 345S–350S. doi:10.3945/an.112.003574
Duatis, J., Guirado, V., and Podhajsky, S. (2008). MELiSSA adaptation for space. Phase II. Preliminary life support system design. MELiSSA Technical Note 88.3. NTE SA (Werfen Group) Available at: https://www.melissafoundation.org/download/174.
Duatis, J., and Moreno, D. (2009). MELiSSA adaptation for space. Phase II. LIfe support system recommended design for a demonstration in a moon base. Barcelona, Spain: NTE-SENER. MELiSSA Technical Note 88.5.
Duatis, J., and Moreno, D. (2010). MELiSSA adaptation for space. Phase II. Recommendations and future work. PreliminaryDevelopment plan. MELiSSA technical note 88.6. NTE-SENER Available at: https://www.melissafoundation.org/download/238 (Accessed March 23, 2023).
W. Dubitzky, J. Southgate, and H. Fuß (Editors) (2011). “Adaptation and self-organizing systems,” Understanding the dynamics of biological systems (New York, NY: Springer). doi:10.1007/978-1-4419-7964-3
Dussap, C.-G., Cornet, J.-F., and Gros, J.-B. (1993). Simulation of mass fluxes in the MELISSA microorganism based ecosystem. Warrendale, PA SAE Int., 932125. doi:10.4271/932125
Dussap, C. G., and Gros, J. B. (1991). Simulation des flux de matières dans l’écosystème MELiSSA. MELiSSA Technical Note 14.1. Laboratoire de Génie Chimique et Biochimique Available at: https://www.melissafoundation.org/download/600 (Accessed March 23, 2023).
Escobar, C., and Nabity, J. (2017). “Past, present, and future of closed human life support ecosystems-a review,” in Proceedings of the 47th International Conference on Environmental Systems, Charleston, South Carolina, July 2017, 16–20.
Ewert, M. K., Chen, T. T., and Powell, C. D. (2022). Life support baseline values and assumptions document. Available at: https://ntrs.nasa.gov/citations/20210024855 (Accessed April 1, 2023).
Farges, B., Poughon, L., Creuly, C., Cornet, J.-F., Dussap, C.-G., and Lasseur, C. (2008). Dynamic aspects and controllability of the MELiSSA project: a bioregenerative system to provide life support in space. Appl. Biochem. Biotechnol. 151, 686–699. doi:10.1007/s12010-008-8292-2
Finn, C. K. (1998). Steady-state system mass balance for the BIO-plex. Warrendale, PA: SAE International. doi:10.4271/981747
FoodData Central (2019a). Seaweed, spirulina, dried. Available at: https://fdc.nal.usda.gov/fdc-app.html#/food-details/170495/nutrients (Accessed March 23, 2023).
FoodData Central (2019b). Soybeans, mature cooked, boiled, without salt. Available at: https://fdc.nal.usda.gov/fdc-app.html#/food-details/174271/nutrients (Accessed March 23, 2023).
Forrest, S., and Mitchell, M. (2016). Adaptive computation: the multidisciplinary legacy of John H. Holland. Commun. ACM 59, 58–63. doi:10.1145/2964342
Forsum, E., Eriksson, C., Göranzon, H., and Sohlström, A. (1990). Composition of faeces from human subjects consuming diets based on conventional foods containing different kinds and amounts of dietary fibre. Br. J. Nutr. 64, 171–186. doi:10.1079/BJN19900019
Fu, Y., Li, L., Xie, B., Dong, C., Wang, M., Jia, B., et al. (2016). How to establish a bioregenerative life support system for long-term crewed missions to the moon or mars. Astrobiology 16, 925–936. doi:10.1089/ast.2016.1477
Fulget, N. (1996). Complete loop control: first study. MELiSSA technical note 28.3. ADERSA. Available at: https://www.melissafoundation.org/download/552 (Accessed March 23, 2023).
Fulget, N., Poughon, L., Richalet, J., and Lasseur, Ch. (1999). Melissa: global control strategy of the artificial ecosystem by using first principles models of the compartments. Adv. Space Res. 24, 397–405. doi:10.1016/S0273-1177(99)00490-1
Garcia-Gragera, D., Arnau, C., Peiro, E., Dussap, C.-G., Poughon, L., Gerbi, O., et al. (2021). Integration of nitrifying, photosynthetic and animal compartments at the MELiSSA pilot plant. Front. Astron. Space Sci. 8, 750616. doi:10.3389/fspas.2021.750616
Garland, J. L. (1989). A simple, mass balance model of carbon flow in a controlled ecological life support system. Available at: https://ntrs.nasa.gov/citations/19900001255 (Accessed March 23, 2023).
Gòdia, F., Albiol, J., Pérez, J., Creus, N., Cabello, F., Montràs, A., et al. (2004). The MELISSA pilot plant facility as an integration test-bed for advanced life support systems. Adv. Space Res. 34, 1483–1493. doi:10.1016/j.asr.2003.08.038
Gorce, B., Garnier, L., Ssi Yan Kai, H., Schini, P.-Y., Lefevre, A., Afuera, E., et al. (2022). “Conceptual design of an environment control and life support system for a mars transit mission,” in MELiSSA Conference 2022, Toulouse, France, November 2022, 8–10. Available at: https://www.melissafoundation.org/download/932 (Accessed March 23, 2023).
Gros, J. B., Poughon, L., Lasseur, C., and Tikhomirov, A. A. (2003). Recycling efficiencies of C,H,O,N,S, and P elements in a biological life support system based on micro-organisms and higher plants. Adv. Space Res. 31 (1), 195–199. doi:10.1016/S0273-1177(02)00739-1
Guirado, V., and Podhajsky, S. (2008). MELiSSA adaptation for space. Phase II. Summary of the European life support systems technologies. MELiSSA Technical Note 88.2. NTE SA (Werfen Group) Available at: https://www.melissafoundation.org/download/173 (Accessed March 23, 2023).
Häder, D. (2020). On the way to mars—flagellated algae in bioregenerative life support systems under microgravity conditions. Front. Plant Sci. 10, 1621. doi:10.3389/fpls.2019.01621
Hendrickx, L., De Wever, H., Hermans, V., Mastroleo, F., Morin, N., Wilmotte, A., et al. (2006). Microbial ecology of the closed artificial ecosystem MELiSSA (Micro-Ecological Life Support System Alternative): reinventing and compartmentalizing the Earth’s food and oxygen regeneration system for long-haul space exploration missions. Res. Microbiol. 157, 77–86. doi:10.1016/j.resmic.2005.06.014
Hendrickx, L., and Mergeay, M. (2007). From the deep sea to the stars: human life support through minimal communities. Curr. Opin. Microbiol. 10, 231–237. doi:10.1016/j.mib.2007.05.007
Holohan, B. C., Duarte, M. S., Szabo-Corbacho, M. A., Cavaleiro, A. J., Salvador, A. F., Pereira, M. A., et al. (2022). Principles, advances, and perspectives of anaerobic digestion of lipids. Environ. Sci. Technol. Wash. 56, 4749–4775. doi:10.1021/acs.est.1c08722
Hu, E., Bartsev, S., and Liu, H. (2010). Conceptual design of a bioregenerative life support system containing crops and silkworms. Adv. Space Res. 45, 929–939. doi:10.1016/j.asr.2009.11.022
Imhof, B., Weiss, P., Vermeulen, A., Flynn, E., Hyams, R., Kerrigan, C., et al. (2017). “Space architectures,” in Star Ark: a Living, Self-Sustaining Spaceship. Editor R. Armstrong (Cham: Springer International Publishing), 287–340. doi:10.1007/978-3-319-31042-8_12
Jenkins, D., Kendall, C., Popovich, D., Vidgen, E., Mehling, C., Vuksan, V., et al. (2001). Effect of a very-high-fiber vegetable, fruit, and nut diet on serum lipids and colonic function. Metabolism Clin. Exp. 50, 494–503. doi:10.1053/meta.2001.21037
Jiang, J., Zhang, Y., Li, K., Wang, Q., Gong, C., and Li, M. (2013). Volatile fatty acids production from food waste: effects of pH, temperature, and organic loading rate. Bioresour. Technol. 143, 525–530. doi:10.1016/j.biortech.2013.06.025
Johnson, C., Boles, H., Spencer, L., Poulet, L., Romeyn, M., Bunchek, J., et al. (2021). Supplemental food production with plants: a review of nasa research. Front. Astronomy Space Sci. 8. doi:10.3389/fspas.2021.734343
Jones, H. (2003). “Design rules for life support systems,” in 33rd International Conference on Environmental Systems (ICES), Vancouver, B.C., Canada, 7-10 July 2003. Available at: https://ntrs.nasa.gov/citations/20040012725 (Accessed March 23, 2023).
Kaleta, A., and Górnicki, K. (2013). Criteria of determination of safe grain storage time - a review. Adv. Agrophysical Res., 295–318.
Kanazawa, S., Ishikawa, Y., Tomita-Yokotani, K., Hashimoto, H., Kitaya, Y., Yamashita, M., et al. (2008). Space agriculture for habitation on Mars with hyper-thermophilic aerobic composting bacteria. Adv. Space Res. 41, 696–700. doi:10.1016/j.asr.2007.09.040
Khan, M. A., Ngo, H. H., Guo, W. S., Liu, Y., Nghiem, L. D., Hai, F. I., et al. (2016). Optimization of process parameters for production of volatile fatty acid, biohydrogen and methane from anaerobic digestion. Bioresour. Technol. 219, 738–748. doi:10.1016/j.biortech.2016.08.073
Knowledge4Policy (2021). Nutritional value of whole grains. Available at: https://knowledge4policy.ec.europa.eu/health-promotion-knowledge-gateway/whole-grain-nutritional-value-whole-2_en (Accessed March 23, 2023).
Kobayashi, M., and Kobayashi, M. (1995). “Waste remediation and treatment using anoxygenic phototrophic bacteria,” in Anoxygenic photosynthetic bacteria advances in photosynthesis and respiration. Editors R. E. Blankenship, M. T. Madigan, and C. E. Bauer (Dordrecht: Springer Netherlands), 1269–1282. doi:10.1007/0-306-47954-0_62
Lasseur, C., Brunet, J., De Weever, H., Dixon, M., Dussap, G., Godia, F., et al. (2010). MELiSSA: the European project of closed life support system. Gravitational Space Res. 23. http://citeseerx.ist.psu.edu/viewdoc/download?doi=10.1.1.464.3630andrep=rep1andtype=pdf
Lasseur, Ch., Verstraete, W., Gros, J. B., Dubertret, G., and Rogalla, F. (1996). Melissa: a potential experiment for a precursor mission to the moon. Adv. Space Res. 18, 111–117. doi:10.1016/0273-1177(96)00097-X
Lauwers, J., Appels, L., Thompson, I. P., Degrève, J., Van Impe, J. F., and Dewil, R. (2013). Mathematical modelling of anaerobic digestion of biomass and waste: power and limitations. Prog. Energy Combust. Sci. 39, 383–402. doi:10.1016/j.pecs.2013.03.003
Li, Y. Y., Sasaki, H., Yamashita, K., Seki, K., and Kamigochi, I. (2002). High-rate methane fermentation of lipid-rich food wastes by a high-solids co-digestion process. Water Sci. Technol. 45, 143–150. doi:10.2166/wst.2002.0420
Liebetrau, J., Weinrich, S., Sträuber, H., and Kretzschmar, J. (2017). “Anaerobic fermentation of organic material: biological processes and their control parameters,” in Encyclopedia of sustainability science and Technology. Editor R. A. Meyers (New York, NY: Springer), 1–30. doi:10.1007/978-1-4939-2493-6_962-1
Lim, S.-J., Kim, B. J., Jeong, C.-M., Choi, J., Ahn, Y. H., and Chang, H. N. (2008). Anaerobic organic acid production of food waste in once-a-day feeding and drawing-off bioreactor. Bioresour. Technol. 99, 7866–7874. doi:10.1016/j.biortech.2007.06.028
Loader, C., Garland, J., Raychaudhuri, S., and Wheeler, R. (1997). A simple mass balance model of nitrogen flow in a bioregenerative life support system. Life support & biosphere Sci. Int. J. earth space 4, 31–41.
Luther, A., Beyaert, A., Brutsaert, M., Lasseur, C., Rebeyre, P., and Clauwaert, P. (2018). “Bio-electrochemical oxidation for CO2 recovery in regenerative life support systems,” in 42nd COSPAR Scientific Assembly Abstracts (COSPAR (Committee on Space Research)), California, USA, July 2018. Available at: http://hdl.handle.net/1854/LU-8573095 (Accessed March 23, 2023).
Macelroy, R. D., and Averner, M. M. (1978). Space ecosynthesis: an approach to the design of closed ecosystems for use in space. Available at: https://ntrs.nasa.gov/citations/19780018797 (Accessed March 23, 2023).
Mackie, R. I., White, B. A., and Bryant, M. P. (1991). Lipid metabolism in anaerobic ecosystems. Crit. Rev. Microbiol. 17, 449–479. doi:10.3109/10408419109115208
Maclean, H., Dochain, D., Waters, G., Dixon, M., Chaerle, L., and Van Der Straeten, D. (2010). “Identification of simple mass balance models for plant growth - towards food production on manned space missions,” in Proceedings IFAC-CAB 2010 Conference, Leuven, Belgium, July 2010 (International Federation of Automatic Control), 7–9. 335–340. Available at: https://dial.uclouvain.be/pr/boreal/object/boreal:87876 (Accessed March 23, 2023).
Mastroleo, F. (2009). Molecular characterization of the life support bacterium Rhodospirillum rubrum S1H cultivated under space related environmental conditions. Université de Mons-Hainaut, Mons, Belgium. Available at: https://researchportal.sckcen.be/files/4517866/Molecular_characterization_of_the_life_support_bacterium_Rhodospirillum_rubrum_S1H_cultivated_under_space_related_environmental_conditions_thesis.Felice.20.01.09.BIS.without.pdf (Accessed March 23, 2023).
Mastroleo, F., Moussalli, C., Raeymaekers, L., Smolders, C., Leysen, L., Coninx, I., et al. (2020). “Investigating volatile fatty acids conversion to CO2 by the MELiSSA bacterium Rhodospirillum rubrum,” in MELiSSA Conference 2020, France, November 2020, 3–5. online.
MedlinePlus, (2023). Urine 24-hour volume. Available at: https://medlineplus.gov/ency/article/003425.htm (Accessed March 23, 2023).
MELiSSA Foundation (2023). Melissa space research program. Available at: https://www.melissafoundation.org/page/melissa-project (Accessed March 23, 2023).
MELiSSA (1989). Modelling. MELiSSA technical note 4. Available at: https://www.melissafoundation.org/download/609 (Accessed March 23, 2023).
Michel, N., De Wever, H., Dotremont, C., and Van Hoof, V. (2005). Engineering of the waste compartment. MELiSSA technical note 71.9.4. ECo process assistance. VITO Available at: https://www.melissafoundation.org/download/327 (Accessed March 23, 2023).
Molders, K., Quinet, M., Decat, J., Secco, B., Dulière, E., Pieters, S., et al. (2012). Selection and hydroponic growth of potato cultivars for bioregenerative life support systems. Adv. Space Res. 50, 156–165. doi:10.1016/j.asr.2012.03.025
Moore, K. J., and Hatfield, R. D. (1994). “Carbohydrates and forage quality,” in Forage quality, evaluation, and utilization (New Jersey, United States: John Wiley & Sons, Ltd), 229–280. doi:10.2134/1994.foragequality.c6
Muys, M., Sui, Y., Schwaiger, B., Lesueur, C., Vandenheuvel, D., Vermeir, P., et al. (2019). High variability in nutritional value and safety of commercially available Chlorella and Spirulina biomass indicates the need for smart production strategies. Bioresour. Technol. 275, 247–257. doi:10.1016/j.biortech.2018.12.059
Nelson, G. J., Vijapur, S. H., Hall, T. D., Brown, B. R., Peña-Duarte, A., and Cabrera, C. R. (2020). Electrochemistry for space life support. Electrochem. Soc. Interface 29, 47–52. doi:10.1149/2.F06201IF
Nelson, M., Pechurkin, N. S., Allen, J. P., Somova, L. A., and Gitelson, J. I. (2010). Closed ecological systems, space life support and biospherics. Environ. Biotechnol., 517–565. doi:10.1007/978-1-60327-140-0_11
Nicolau, E., Rodríguez-Martínez, J. A., Fonseca, J., Richardson, T.-M. J., Flynn, M., Griebenow, K., et al. (2010). Bioelectrochemical oxidation of urea with urease and platinized boron doped diamond electrodes for water recycling in space applications. ECS Trans. 33, 1853–1859. doi:10.1149/1.3484676
Page, V., and Feller, U. (2013). Selection and hydroponic growth of bread wheat cultivars for bioregenerative life support systems. Adv. Space Res. 52, 536–546. doi:10.1016/j.asr.2013.03.027
Pannico, A., Cimini, G., Quadri, C., Paradiso, R., Bucchieri, L., Rouphael, Y., et al. (2022). A plant characterization unit for closed life support: hardware and control design for atmospheric systems. Front. Astron. Space Sci. 9, 820752. doi:10.3389/fspas.2022.820752
Paradiso, R., Micco, V., Buonomo, R., Aronne, G., Barbieri, G., and De Pascale, S. (2013). Soilless cultivation of soybean for bioregenerative life-support systems: a literature review and the experience of the MELiSSA project - food characterisation phase I. Plant Biol. Stuttg. Ger. 16, 69–78. doi:10.1111/plb.12056
Peiro, E., Pannico, A., Colleoni, S., Bucchieri, L., Rouphael, Y., De Pascale, S., et al. (2020). Air distribution in a fully-closed higher plant growth chamber impacts crop performance of hydroponically-grown lettuce. Front. Plant Sci. 11, 537. doi:10.3389/fpls.2020.00537
Pilo Teniente, S. (2015). Simulation of the gas phase integration between compartments CIVa and CV of the MELiSSA Pilot Plant. Barcelona, Spain: Universitat Politècnica de Catalunya. Available at: https://upcommons.upc.edu/handle/2117/86047 (Accessed March 23, 2023).
Poughon, L., Creuly, C., Farges, B., Dussap, C.-G., Schiettecatte, W., Jovetic, S., et al. (2013). Test of an anaerobic prototype reactor coupled with a filtration unit for production of VFAs. Bioresour. Technol. 145, 240–247. doi:10.1016/j.biortech.2012.12.052
Poughon, L., Creuly, C., Godia, F., Leys, N., and Dussap, C.-G. (2021). Photobioreactor Limnospira indica growth model: application from the MELiSSA plant pilot scale to ISS flight experiment. Front. Astronomy Space Sci. 8. doi:10.3389/fspas.2021.700277
Poughon, L., Dussap, C. G., Cornet, J. F., and Gros, J. B. (1994). Melissa: behavior of the ecosystem under different light radiant energy inputs. Warrendale, PA SAE Int., 941347. doi:10.4271/941347
Poughon, L. (2007a). Dynamic modelling of a coupled MELiSSA crew - compartment C4a with Matlab-Simulink. MELiSSA Technical Note 83.1. Laboratoire de Génie Chimique et Biochimique Available at: https://www.melissafoundation.org/download/199 (Accessed March 23, 2023).
Poughon, L., Farges, B., Dussap, C. G., Godia, F., and Lasseur, C. (2009). Simulation of the MELiSSA closed loop system as a tool to define its integration strategy. Adv. Space Res. 44, 1392–1403. doi:10.1016/j.asr.2009.07.021
Poughon, L., Gros, J. B., and Dussap, C. G. (2000). “MELISSA loop: first estimate of flow rates and concentrations through the loop,” in 30th International Conference on Environmental Systems (ICES), Toulouse, France, 10-13 July 2000 (SAE Technical Paper Series) (Warrendale, PA: SAE International).
Poughon, L., Laroche, C., Creuly, C., Dussap, C.-G., Paille, C., Lasseur, C., et al. (2020). Limnospira indica PCC8005 growth in photobioreactor: model and simulation of the ISS and ground experiments. Life Sci. Space Res. 25, 53–65. doi:10.1016/j.lssr.2020.03.002
Poughon, L. (2007b). MELiSSA loop mass balance modelling with MatLab/simulink. MELiSSA Technical Note 79.2. Laboratoire de Génie Chimique et Biochimique Available at: https://www.melissafoundation.org/download/197 (Accessed March 23, 2023).
Poughon, L. (1994a). MELiSSA simulation and modelling. Spirulina modelling. Variable global stoichiometric equation of Spirulina platensis in different light conditions. Effects of light on diet composition. Best conditions to insure maximal mass recycling. MELiSSA Technical Note 17.3. Laboratoire de Génie Chimique et Biochimique Available at: https://www.melissafoundation.org/download/572 (Accessed March 23, 2023).
Poughon, L. (1994b). MELiSSA simulation and modelling. Study of partial conversion. The stoichiometric assumptions: origin and effects. The main consequences of the partial conversion of components. MELiSSA Technical Note 17.2. Laboratoire de Génie Chimique et Biochimique Available at: https://www.melissafoundation.org/download/571 (Accessed March 23, 2023).
Poulet, L., Dussap, C.-G., and Fontaine, J.-P. (2018). A physical modeling approach for higher plant growth in reduced gravity environments. Astrobiology 18, 1093–1100. doi:10.1089/ast.2017.1804
Poulet, L., Dussap, C.-G., and Fontaine, J-P. (2020). Development of a mechanistic model of leaf surface gas exchange coupling mass and energy balances for life-support systems applications. Acta Astronaut. 175, 517–530. doi:10.1016/j.actaastro.2020.03.048
Putnam, D. F. (1971). Composition and concentrative properties of human urine. Available at: https://ntrs.nasa.gov/citations/19710023044 (Accessed March 23, 2023).
Ramsay, I. R., and Pullammanappallil, P. C. (2001). Protein degradation during anaerobic wastewater treatment: derivation of stoichiometry. Biodegradation 12, 247–257. doi:10.1023/A:1013116728817
Rose, C., Parker, A., Jefferson, B., and Cartmell, E. (2015). The characterization of feces and urine: a review of the literature to inform advanced treatment Technology. Crit. Rev. Environ. Sci. Technol. 45, 1827–1879. doi:10.1080/10643389.2014.1000761
Sachdeva, N., Poughon, L., Gerbi, O., Dussap, C.-G., Lasseur, C., Leroy, B., et al. (2021). Ground demonstration of the use of Limnospira indica for air revitalization in a bioregenerative life-support system setup: effect of non-nitrified urine–derived nitrogen sources. Front. Astronomy Space Sci. 8. doi:10.3389/fspas.2021.700270
Shin, H.-S., Youn, J.-H., and Kim, S.-H. (2004). Hydrogen production from food waste in anaerobic mesophilic and thermophilic acidogenesis. Int. J. Hydrogen Energy 29, 1355–1363. doi:10.1016/j.ijhydene.2003.09.011
Stasiak, M., Gidzinski, D., Jordan, M., and Dixon, M. (2012). Crop selection for advanced life support systems in the ESA MELiSSA program: durum wheat (Triticum turgidum var durum). Adv. Space Res. 49, 1684–1690. doi:10.1016/j.asr.2012.03.001
Tepari, E. A. (2019). Evaluation of biohydrogen production from Co-fermentation of carbohydrates and proteins. Available at: https://ir.lib.uwo.ca/etd/6566.
Teuling, E., Wierenga, P. A., Schrama, J. W., and Gruppen, H. (2017). Comparison of protein extracts from various unicellular green sources. J. Agric. Food Chem. 65, 7989–8002. doi:10.1021/acs.jafc.7b01788
Thiron, B. (2020). Global control of a life support system: flow control and optimisation. Stockholm, Sweden: KTH School of Electrical Engineering and Computer Science. Available at: http://urn.kb.se/resolve?urn=urn:nbn:se:kth:diva-278509 (Accessed March 23, 2023).
Tikhomirov, A. A., Ushakova, S. A., Velichko, V. V., Tikhomirova, N. A., Kudenko, Yu. A., Gribovskaya, I. V., et al. (2011). Assessment of the possibility of establishing material cycling in an experimental model of the bio-technical life support system with plant and human wastes included in mass exchange. Acta Astronaut. 68, 1548–1554. doi:10.1016/j.actaastro.2010.10.005
Tranquille, N., and Emeis, J. J. (1996). Rhodospirillum rubrum food acceptability study for MELiSSA. MELiSSA Technical Note 30.1. TNO Prevention and Health Available at: https://www.melissafoundation.org/download/554 (Accessed March 23, 2023).
Tusé, D., and Miller, M. W. (1984). Single-cell protein: current status and future prospects. C R C Crit. Rev. Food Sci. Nutr. 19, 273–325. doi:10.1080/10408398409527379
U.S. Food & Drug Administration (2017). Nutrition information for raw vegetables. Available at: https://www.fda.gov/food/food-labeling-nutrition/nutrition-information-raw-vegetables (Accessed March 23, 2023).
Usherwood, N., and Segars, W. (2001). Nitrogen interactions with phosphorus and potassium for optimum crop yield, nitrogen use effectiveness, and environmental stewardship. TheScientificWorldJournal 1, 57–60. Suppl 2. doi:10.1100/tsw.2001.97
Vermeulen, A. C. J., Papic, A., and Brazier, F. (2018a). “Modeling and simulating the MELiSSA loop to understand the effects of system interaction on survivability during long-duration interstellar missions: an agent-based approach,” in AgroSpace-MELiSSA Conference, Rome, Italy, May 16–18, 2018. doi:10.5281/zenodo.7791088
Vermeulen, A. C. J., Sirenko, M., Papic, A., Kiem, J., Theys, A., and Brazier, F. (2018b). “Evolving asteroid Starships: a bio-inspired approach for interstellar space systems,” in Proceedings of the 69th International Astronautical Congress (IAC), Bremen, Germany, October 1–5, 2018.
Vermeulen, A. C., Papic, A., Kiem, J., Hallak, D., and Brazier, F. (2019). “Modeling and simulating a regenerative life support system to understand the effects of system interaction on survivability during deep space missions: an agent-based approach,” in Proceedings of the 70th International Astronautical Congress (IAC), Washington DC, October 21–25, 2019.
Vermeulen, A. C. J., Hubers, C., de Vries, L., and Brazier, F. (2020a). What horticulture and space exploration can learn from each other: the Mission to Mars initiative in the Netherlands. Acta Astronaut. 177, 421–424. doi:10.1016/j.actaastro.2020.05.015
Vermeulen, A. C. J., Papic, A., Kiem, J., Hallak, D., and Brazier, F. (2020b). “Exploring the impact of irregular metabolic efficiencies and the space environment on the survivability of a regenerative life support system through agent-based modeling,” in MELiSSA Conference 2020, November 3–5, 2020,online. doi:10.5281/zenodo.7791110
Vijapur, S. H., Hall, T. D., Snyder, S., Inman, M., Taylor, E. J., and Skinn, B. (2017). Electrochemical peroxide generation. ECS Trans. 77, 947–962. doi:10.1149/07711.0947ecst
Volk, T., and Rummel, J. D. (1987). Mass balances for a biological life support system simulation model. Adv. Space Res. 7, 141–148. doi:10.1016/0273-1177(87)90045-7
Vrati, S. (1984). Single cell protein production by photosynthetic bacteria grown on the clarified effluents of biogas plant. Appl. Microbiol. Biotechnol. 19, 199–202. doi:10.1007/BF00256454
Waters, G. C. R., Dixon, M. A., Masot, A., Albiol, J., and Godia, F. (2004). “Static mass balance studies of the MELiSSA pilot plant: integration of a higher plant chamber,” in 34th International Conference on Environmental Systems (ICES), Colorado Springs, CO, 19-22 July 2004 (SAE Technical Paper Series) (Warrendale, PA: SAE International).
WebMD (2021). Are there health benefits of durum wheat? Available at: https://www.webmd.com/diet/are-there-health-benefits-of-durum-wheat (Accessed March 23, 2023).
Weng, C., and Jeris, J. S. (1976). Biochemical mechanisms in the methane fermentation of glutamic and oleic acids. Water Res. 10, 9–18. doi:10.1016/0043-1354(76)90151-2
Yin, J., Yu, X., Wang, K., and Shen, D. (2016). Acidogenic fermentation of the main substrates of food waste to produce volatile fatty acids. Int. J. Hydrogen Energy 41, 21713–21720. doi:10.1016/j.ijhydene.2016.07.094
Zambelli, A., León, A., and Garcés, R. (2015). “Mutagenesis in sunflower,” in Sunflower. Editors Sunflower, E. Martínez-Force, N. T. Dunford, and J. J. Salas (Urbana, Illinois: AOCS Press), 27–52. doi:10.1016/B978-1-893997-94-3.50008-8
Zhang, D., Clauwaert, P., Luther, A., López Barreiro, D., Prins, W., Brilman, D. W. F., et al. (2018). Sub- and supercritical water oxidation of anaerobic fermentation sludge for carbon and nitrogen recovery in a regenerative life support system. Waste Manag. 77, 268–275. doi:10.1016/j.wasman.2018.04.008
Keywords: space exploration, human spaceflight, bioregenerative life support, waste processing, food production, ecosystem modeling, simulation, MELiSSA
Citation: Vermeulen ACJ, Papic A, Nikolic I and Brazier F (2023) Stoichiometric model of a fully closed bioregenerative life support system for autonomous long-duration space missions. Front. Astron. Space Sci. 10:1198689. doi: 10.3389/fspas.2023.1198689
Received: 01 April 2023; Accepted: 07 July 2023;
Published: 16 August 2023.
Edited by:
Cyprien Verseux, University of Bremen, GermanyReviewed by:
Lucie Poulet, UMR6602 Institut Pascal (IP), FranceCopyright © 2023 Vermeulen, Papic, Nikolic and Brazier. This is an open-access article distributed under the terms of the Creative Commons Attribution License (CC BY). The use, distribution or reproduction in other forums is permitted, provided the original author(s) and the copyright owner(s) are credited and that the original publication in this journal is cited, in accordance with accepted academic practice. No use, distribution or reproduction is permitted which does not comply with these terms.
*Correspondence: Angelo C. J. Vermeulen, YS5jLmoudmVybWV1bGVuQHR1ZGVsZnQubmw=
Disclaimer: All claims expressed in this article are solely those of the authors and do not necessarily represent those of their affiliated organizations, or those of the publisher, the editors and the reviewers. Any product that may be evaluated in this article or claim that may be made by its manufacturer is not guaranteed or endorsed by the publisher.
Research integrity at Frontiers
Learn more about the work of our research integrity team to safeguard the quality of each article we publish.