- 1Université Paris-Saclay, INRAE, AgroParisTech, MICALIS, MetaGenoPolis, Jouy-en-Josas, France
- 2Directorate of Technology, Engineering and Quality, European Space Agency, Noordwijk, Netherlands
Humans are microbial, ecosystems and symbioses. The relationship that humans have with their microbiomes is an essential element to maintaining health and wellbeing. Recent changes in lifestyles may have fostered an alteration of this symbiosis, which is frequently associated with chronic disorders. Here, we will review the state of the art on the central role of human-microbes symbiosis in health and disease, highlighting the innovations expected from the emerging knowledge on host-microbes symbiosis, for diagnosis, preventive nutrition, and a medicine of the ‘microbial human’. Since microbiome science also impacts several sustainable development goals of the Planetary Boundaries Initiative, we will also explore how microbiome science could help to provide sustainability tools and strategies aligned with the life support systems sought by the Micro-Ecological Life Support Systems Alternative (MELiSSA) Project lead by the European Space Agency (ESA).
1 Introduction
The microbiota is the complex and dynamic community of microbes with which humans interact on a constant basis. Human-associated microbes are present on the skin and at every mucosal interface of the human body, but the highest densities are found in the large intestine. It is estimated that in the human body there are overall 50 trillion bacteria as well as a multitude of archaea, phages, fungi, and yeasts.
These microbes exert several functions that pertain to four essential domains of regulation, acting not only as endocrine and metabolic, immuno-inflammatory and neuro-vegetative regulators, but also as antimicrobial protectors.
These functions are expressed at 3 key interfaces: 1) food-microbes, 2) microbes-microbes, and 3) microbes-human cells. It is hypothesized that they can provide protective benefits to the human host by preventing infections as well as the onset and progression of a large array of chronic conditions that have increased in incidence. These chronic conditions are seen today by the World Health Organization (WHO) as an epidemic, considering they represent a major public health burden: obesity, inflammatory diseases, liver conditions, some cancers, neuro-degenerative and neuro-psychiatric disorders (Malard et al., 2021a).
1.1 Features of a health-associated microbiota
Although the establishment of a concise definition of the ‘normal’ microbiota has remained challenging, the identification of structural and functional alterations of the gut microbiota has been evaluated in numerous clinical conditions, aiming to document a plausible link. Molecular characterization of the human intestinal microbiota has gone through a technical revolution with the application of high throughput sequencing that has given insights into inter-individual similarities and differences in microbiota in health and disease. Analyzing hundreds of individual microbiota profiles revealed that healthy individuals share a core microbiota. It gathers a rather small number of bacterial species that are present in every healthy individual (Tap et al., 2009; Qin et al., 2010). Interestingly, this core microbiome is less than 20 species out of the average 300 species of each dominant intestinal microbiome. In addition, the microbiota appears remarkably stable over time for each person, except in early life or at old age, as well as upon intake of therapeutic treatments or drastic changes in life habits or nutrition (Falony et al., 2016; Zhernakova et al., 2016).
This indicates that it would be possible to point at bacterial species that are consistently associated with dietary recommendations known to be beneficial - or conversely detrimental - for human health.
Table 1 summarizes the two sets of bacterial taxa proposed based on studies of volunteers of the UK-Twin cohort. Due to functional redundancy, diverse microbial species will often contribute to similar functions irrespective of their taxonomic assignment, hence a normal/health-associated microbiota may be better specified on a functional standpoint rather than in terms of composition. Indeed, a series of metabolites derived from microbiome metabolism have gained interest as they are associated with health or risk/disease as shown on Table 2 (Martinez et al., 2017).
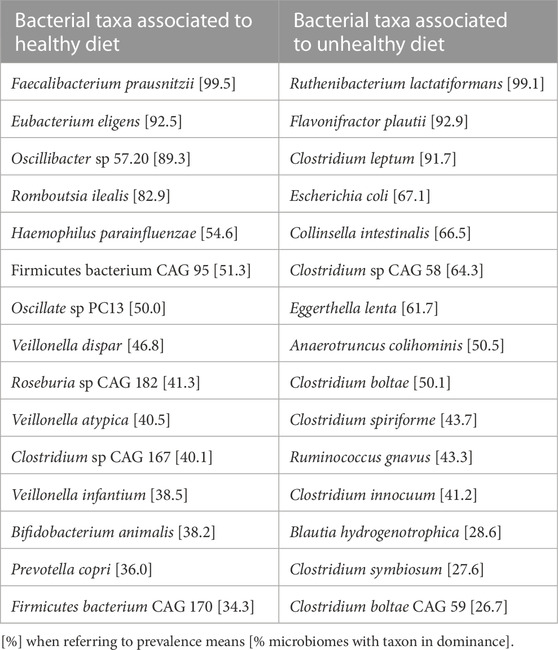
TABLE 1. Bacterial taxa associated to a healthy or unhealthy diet (Asnicar et al., 2021). Taxa are ranked from the most to the least prevalent [%]. When the genome does not correspond to a cultured strain, a Co-Abundant Genes-cluster (CAG) is indicated.
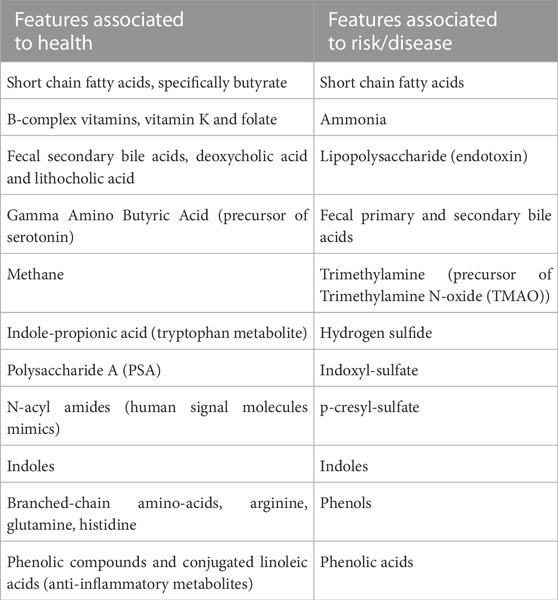
TABLE 2. Microbiome-derived features and metabolites that may be associated with health or risk/disease (Martinez et al., 2017).
In addition, early shotgun metagenomics-based microbiome studies highlighted two unexpected elements of stratification of the microbiota with health relevance.
Firstly, microbiome richness was recognized as a stratifier. Microbiomes show a broad heterogeneity in health and disease. In some studies, a separate fraction of the population shows a low richness microbiome, and this may even be a predictive feature in several contexts. For example, a low richness microbiome will predict.
- Non-response to calory restriction in obesity (Cotillard et al., 2013)
- Severity/progression in acute liver conditions (Qin et al., 2014; Sole et al., 2018)
- Non-response to cancer immunotherapies and a lesser progression-free survival in melanoma (Gopalakrishnan et al., 2018) and lung cancer (Routy et al., 2018)
- Reduced survival rate following hematopoietic stem cell transplantation (HSCT) in blood cancer therapy (Taur et al., 2014)
- A lesser global survival of patients receiving HSCT during blood-cancer therapy (Peled et al., 2020)
- A higher mortality from acute graft versus host disease during blood-cancer therapy (Taur et al., 2014; Jenq et al., 2015)
Secondly, four ecological configurations which stratify the human population have been described and are also known as enterotypes (Arumugam et al., 2011; Costea et al., 2017a). One combines the genus Bacteroides as dominant taxon and a low richness. This enterotype (B2) is associated with the highest prevalence of altered metabolic and inflammatory markers conferring a higher suspicion of cardiometabolic risk for this segment of the population (Vieira-Silva et al., 2020).
1.2 Altered host-microbes symbiosis
Host-microbes symbiosis guarantees maintenance of health and wellbeing, yet for more than half a century our inner microbial inhabitants and symbiosis have received little consideration. Today, the epidemiological evidence is that an uncontrolled increase in the incidence of so-called non-transmissible chronic diseases (Bach, 2002) is characterized by an alteration in the homeostasis of the host-microbes symbiosis. Host-microbe symbiosis is affected by every life event, from birth to nutrition, lifestyles, exposure to ubiquitous chemicals in the environment, food, and medical therapies. Individual and societal changes of these parameters may have contributed to the alteration of the microbiota that is documented in many chronic diseases (Blottière and Doré, 2016). But beyond the mere alteration of the microbiota, chronic diseases are typically characterized by the concomitant alteration of host parameters such as intestinal permeability, inflammatory tone, and oxidative stress. In addition, not only physiological, but also psychological stress will impact host parameters at the intestinal level, specifically permeability and inflammation via cortisol (Madison and Kiecolt-Glaser, 2019; Ilchmann-Diounou and Menard, 2020; Varanoske et al., 2022). For the past 30 years, microbiota alteration observed in chronic diseases has been documented and recognized as ‘dysbiosis’. A major paradigm shift is now leading to dysbiosis being recognized as a disruption of host-microbes symbiosis. The microbiome is in constant functional interaction with several features of the human host, such that any alteration of the microbiome will affect the host and conversely. As described earlier, the main host features that will determine the overall configuration of the symbiosis landscape are gut permeability, inflammation, and oxidative stress (van de Guchte et al., 2018; van de Guchte et al., 2020). Figure 1 illustrates the overall concept of these interacting features of symbiosis. Beyond simple bidirectional host-microbes interactions, recent research shows that alteration of these features can lead to positive feedbacks establishing a vicious circle whereby the whole system can enter alternative stable states sustained by circular causalities (Scheffer et al., 2001; Kéfi et al., 2014; van de Guchte et al., 2018; van de Guchte et al., 2020). This may lead to a loss of the healthy microbiome functions explained above (direct anti-microbial protection as well as endocrino-metabolic, immuno-inflammatory and neuro-vegetative regulation). For example, the ecological perturbation of the microbiome due to a course of antibiotic will induce a loss of the barrier function, which may cause nosocomial infection with Clostridioides difficile, or a proliferation of the previously subdominant pathogen (Ramirez et al., 2020; Raplee et al., 2021).
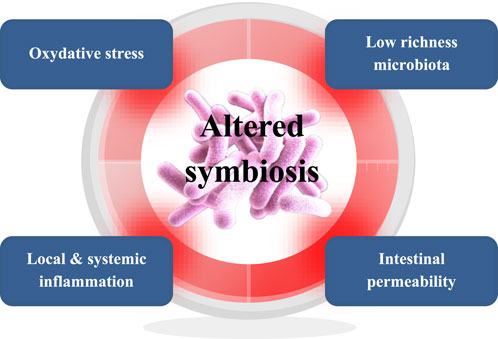
FIGURE 1. Overall concept of host-microbes symbiosis with interacting features such as microbiome richness and composition, intestinal permeability, inflammatory tone, and oxidative stress signals. These features can be triggers of a vicious circle that upon initiation can lead to ‘critical transition’ towards an alternative stable state of pre-disease or disease (Van de Guchte et al., 2018).
Early evidence of causality was recently provided by the demonstration that symbiosis, reconstructed by microbiota transfer from patients to initially germ-free or microbiome depleted animals, can mimic the diseased phenotype or at least be accompanied by a metabolic, inflammatory, or infectious risk. Examples that support this evidence were documented in obesity (Ridaura et al., 2013), Crohn’s disease (Schaubeck et al., 2016), severe alcoholic hepatitis (Llopis et al., 2016), or depression (Kelly et al., 2016), and autism (Sharon et al., 2019). Similarly, Fecal Microbiota Transfer (FMT), now recognized by regulatory agencies for the treatment of recurrent C. difficile infection, is being tested in various clinical settings with the primary incidence of restoring symbiotic homeostasis and reducing symptoms of the clinical condition. This approach has restored insulin sensitivity in diabetic subjects (Vrieze et al., 2012) or soothed the inflammatory context in ulcerative colitis (Paramsothy et al., 2017; Costello et al., 2019). It was also demonstrated to impact severe hepatic conditions (Philips et al., 2017) as well as autism (Kang et al., 2017; Kang et al., 2019) and Graft versus Host Disease (Biliński et al., 2022).
1.3 Factors impacting the microbiome
Rather than a sole alteration of the microbiome, the common thread in numerous chronic conditions is an altered host-microbes symbiosis which is known to be under the influence of numerous intrinsic and environmental factors.
Among the intrinsic host factors, genetics, ethnic origin, age (but mainly in early life and old age) and sex will potentially play pivotal roles (Zhernakova et al., 2016). In addition, the overall health state is also associated with alterations of the microbiome where gut transit state is a key player. This intrinsic factor has a marked influence on the microbiome that ‘proxis’, such as ‘time since previous stool passage’ or the score on the Bristol stool scale at the moment of fecal sample collection, are highly associated with microbiome variations. Among the key features to consider, microbiome gene richness and its enterotype are also highly relevant.
Aside from intrinsic factors, modulators of the microbiome arise from elements of environmental exposure where both medical treatments and diet will rank highest (Falony et al., 2016; Asnicar et al., 2021). Antibiotics, transit modulators, proton pump inhibitors, biological immunomodulators and psychotics can have a significant impact on the microbiome. Among dietary factors, plant-based food is most beneficially impactful and both quantity and diversity will matter greatly. Dietary fibers and associated polyphenols are seen as the active ingredients and their diversity in molecular types will matter, as it will mobilize a range of enzymatic competences among microbes that are rather specialized in their substrate range and glycohydrolytic activities (Ranaivo et al., 2022).
2 Innovative strategies
Microbiome science has reached a point of maturity, impacting preventive nutrition and medicine via monitoring and diagnostic innovations as well as translational therapeutic developments (Wilkinson et al., 2021).
2.1 Diagnostics and predictive models
Despite the recognition of the importance of host-microbes symbiosis in a large array of immune-mediated clinical conditions microbiome configuration and host related symptoms have never been combined in the context of innovative diagnostic models. The two most obvious parameters that could be combined immediately are immune status and microbiome profile. The former is commonly assessed by Medical Biology Laboratories (blood cell counts, CRP, calprotectin) and the latter is today amenable to standardized assessment. As recently tested in the context of pediatric ulcerative colitis, mapping the symbiosis status based on a combination of a microbiome parameter and a measure of inflammatory tone, not only allows to position each patient distant from healthy controls, but it also allows to anticipate the potential for each patient to enter remission during a 1-year clinical management under standard care with immuno-suppressants and/or corticoids (van de Guchte et al., 2021). Nevertheless, the relevance of such novel approaches will require qualification and verification of the biomarker’s associations, and in turn the evaluation of their potential to assist the decision process of healthcare professionals, with ensuing benefits for the patient. Aside from Standard Operating Procedures and reference materials that are available for Medical Biology Laboratories to implement these assessments, the complete pipeline will have to be in place for medical doctors to prescribe, obtain and use these new predictors for their clinical value to be evaluated in diverse clinical indications.
Statistical approaches that can generate predictive models linking microbiome features with relevant metadata are captured under the term Machine Learning (ML). Among ML approaches, supervised ML refers to algorithms that take a set of features and the target values to predict, while unsupervised ML algorithms take as input a set of features and identify any structure within them. ML methods applied to microbiome data, which often suffers from high-dimensionality, sparsity, and compositionality issues, require specific caution in their application and associated data management. ML models are already trained towards the identification of microbiome signatures with diagnostic or predictive value in various disease conditions (Boolchandani et al., 2019; Aryal et al., 2020; Bezek et al., 2020; Fernández-Edreira et al., 2021; Marcos-Zambrano et al., 2021; Liñares-Blanco et al., 2022).
2.2 Prevention and therapy
Similarly, for preventive or therapeutic reasons, it seems appropriate to develop and validate innovative strategies that will ensure a more efficient preservation and/or restoration of symbiosis by targeting at the same time host and microbiome parameters. This is a paradigm shift from former strategies whereby single bioactives (pre-, pro- or post biotics) were used with the aim to provide and complement a given lost function. Combining bioactives targeting several of the triggers mentioned above could improve the potential to act on feed-back loops and break vicious circles that would otherwise remain unaffected or strongly resilient when tackling only one of many triggers. Although not yet implemented in the clinic, a recent publication showed in a mouse model that three food-grade bioactives targeting microbiota, gut permeability, inflammation, and oxidative stress could correct impaired behavior (anxiety and depression) to the same extent as an antidepressant (Faucher et al., 2022). Following the same rationale, we have also described how host-microbes systems modeling suggests that combining immunosuppressant and FMT would likely increase the potential to set in remission infants with pediatric ulcerative colitis (Paramsothy et al., 2017; Costello et al., 2019; van de Guchte et al., 2021). Strong expectations can also be put in other severe conditions such as autism or life-threatening liver cirrhosis (Kang et al., 2017; Philips et al., 2017; Kang et al., 2019). Finally, with the aim to increase the rate of response to a very effective therapeutic approach in a cancer treatment for which the microbiome dictates the status of non-responder in more than half of the patients, a clinical trial combining FMT and immunotherapy has been initiated (NCT04988841). Such innovative strategies would apply equally to prevention in the general population as to therapies in chronic diseases. These innovative therapies should also be accompanied by hygiene and dietary recommendations ensuring a quality of life in nutritional and psychological terms to strengthen the subsequent maintenance of symbiosis.
3 Application for space-travel conditions
As stated in previous sections, several intrinsic and extrinsic aspects directly affect the human gut microbiome and spaceflight is certainly a factor playing a pivotal role. Astronauts endure several physiological and psychological changes while in space due to the exposure to “the space exposome” which gathers galactic cosmic radiation, altered gravity, confinement, nutrient supply, sleep deprivation and alteration of the circadian rhythms, (Crucian et al., 2018). Even though some of the side effects have been extensively documented such as: fluid redistribution, cognitive impairment and decline, bone loss, muscle atrophy, etc., the taxonomic and functional evolution of the astronaut gut microbiome and its impact on the overall astronaut health has drawn little attention (Cucinotta et al., 2014; Grimm et al., 2016; Demontis et al., 2017; Tanaka et al., 2017; Crucian et al., 2018; Jillings et al., 2020). However, deeper understanding on these areas is essential if we want to succeed in our objective of long-duration human space exploration beyond Low Earth Orbit (LEO).
To date, only a few studies have been devoted to monitoring the evolution of the gut microbiome of human space travelers and these studies have a limited sample size and diversity (Brown et al., 1976; Taylor et al., 1997; Ritchie et al., 2015; Casero et al., 2017; Turroni et al., 2017; Hao et al., 2018; Alauzet et al., 2019; Garrett-Bakelman et al., 2019; Jiang et al., 2019; Voorhies et al., 2019; Avila-Herrera et al., 2020; Liu et al., 2020; Urbaniak et al., 2020; Brereton et al., 2021; Chen et al., 2021; Morrison et al., 2021). Hence, they should be interpreted with caution and future studies of astronauts that include a larger number and diversity of individuals is required to determine the global patterns of microbiome evolution to spaceflight to enable the development of predictive models. Nevertheless, preliminary results from real space missions, such as the Astronauts’ Microbiome Project and the Twins Study, showed that microbiome richness composition remains substantially unchanged with progressive convergence in the microbiota composition among the crew members (Garrett-Bakelman et al., 2019; Voorhies et al., 2019). Overall abundance of the genera Lactobacillus and Bifidobacterium decreased which could contribute to alterations in the host immune functions. In addition, intestinal permeability of the host could be compromised due to the described combination of increased proportions of genera associated with chronic intestinal inflammation and decreased abundance of those with anti-inflammatory properties, represented by Parasutterella and Akkermansia, respectively. However, this dysbiosis was transient and reversible upon return to Earth which indicates a resilience of the microbial ecosystem of the gastrointestinal tract. In this respect, a direct association with this observed dysbiosis and the specific microsystem the crew is exposed to inside the spacecraft can be drawn. The habitable environments of spacecrafts are strictly sanitized and controlled by the Environmental Control and Life Support System (ECLSS) which up to date is mainly devoted to the physical and chemical purification and recycling of the air and water and it is highly dependent on continuous resupply of these and other crew consumables from Earth. In addition, these crew consumables, i.e., oxygen, water, food, hygienic material, etc., are also subjected to strict sanitation controls and transported in sterile packaging from Earth. Hence, due to these stringent hygienic and sterile conditions imposed in the habitable areas of the spacecraft, the crew is exposed to a completely novel and specific microsystem which is not a reflection of the habitable areas humans experience while on Earth and may contribute to the transient dysbiosis observed in the recent astronaut microbiome studies.
However, this situation will change with the integration of regenerative LSS in human exploration missions foreseen by space agencies where sustained human presence on Martian floor is targeted. Whereas during voyage, ECLSS will remain based only (or mostly) on physico-chemical recycling processes due to the intrinsic mass of the cycle components as well as the inherent mass, volume and energy requirements of the system, upon arrival on martial soil, the regenerative ECLSS could become the unlocking key to meeting human needs, as local resources and materials could be harnessed and transformed by the system itself into suitable inputs (Verseux et al., 2016). Regenerative LSS development has been supported by International Space Agencies, where the European Space Agency (ESA) was a pioneer by the implementation of the MELiSSA (Micro-Ecological Life-Support Systems Alternative) project in 1989 (Lasseur et al., 2010). Ground-based analog space simulations have recently analyzed the impact on the subjects’ health upon integration of regenerative LSS in the loop (Hao et al., 2018; Chen et al., 2021). This resulted in higher microbiome diversity and richness levels of bacteria associated with not only anti-inflammatory properties but also with increased ability to metabolize dietary polysaccharides highlighting its beneficial impact on the maintenance of a healthy gut microbiota among the crewmembers (Yilmaz et al., 2011; Field et al., 2014; Bowers et al., 2017; Kuehnast et al., 2022). Microbiome science has made useful progress that could support overtime monitoring of the microbiome during space travel. Highly standardized methods have been published (Costea et al., 2017b) and a complete value-chain involving medical biology laboratories is emerging delivering microbiome information to assist clinicians in their decision-making process. Moreover, strategies for the mitigation of alterations of host-microbes symbiosis for astronauts could benefit from recent evidence, provided in clinical trials, that proper conditioning of human stool collected before any stress can be used for near complete reconstruction of post-stress altered microbiome using an autologous procedure (Malard et al., 2021b). Finally, microbiome assessment and management could serve specific goals such as optimizing sustainability of waste fermentation or promoting health benefits of FMT. Ecological modeling of complex intestinal ecosystems management is already underway (Ianiro et al., 2022; Schmidt et al., 2022) and further efforts towards metabolic modeling could be most instrumental as shown in a few pioneering examples: i) butyrate production by the intestinal microbiota (Muñoz-Tamayo et al., 2011); ii) orientation towards primary (detrimental) or secondary (beneficial) bile acids (Kastl et al., 2022); or iii) modulation of immune-inflammatory context and/or gut-brain signaling by the modulation of tryptophan metabolism (Xue et al., 2022). In a recent review, the importance of establishment of a microbiome baseline determination and pre-flight preparations is highlighted (Kuehnast et al., 2022). The settlement of a previous knowledge on the healthy and diverse baseline microbiome and its taxonomic and/or functional fingerprint for each space traveler is suggested and a detailed protocol to conduct this baseline determination is provided. Implementation of this methodology would enable the identification of perturbations in the microbiome and if combined with meticulous metadata recording of the crew’s daily operations (i.e., exercise diary, food intake and composition, health parameters, etc.) statistical correlations could be established (Yilmaz et al., 2011; Field et al., 2014; Bowers et al., 2017).
Based on ongoing efforts, a roadmap for the development of predictive modelling can be suggested. This roadmap could become the baseline for future activities within the frame of the MELiSSA Project lead by ESA. Figure 2 highlights the specific objectives suggested above and for which predictive modeling would be most appropriate.
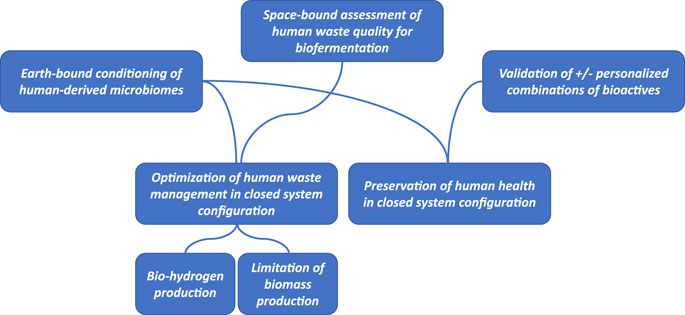
FIGURE 2. Outline of specific objectives that would benefit from the development of predictive modeling tools towards circularization of processes in a closed system configuration. Both optimization of waste management and preservation of human health are indicated as outcomes. Currently available procedures for the conditioning of human intestinal contents in a way that will preserve the integrity of the complex microbiome as a full ecosystem would allow both earth-bound and space-bound applications.
Author contributions
All authors listed have made a substantial, direct, and intellectual contribution to the work and approved it for publication.
Funding
This publication work was supported in part by the European Commission in the context of ERC-2017-AdG No. 788191, Horizon 2020 research and innovation programme under grant agreement No. 964590, and in the context of MetaGenoPolis grant ANR-11-DPBS-0001.
Conflict of interest
The authors declare that the research was conducted in the absence of any commercial or financial relationships that could be construed as a potential conflict of interest.
Publisher’s note
All claims expressed in this article are solely those of the authors and do not necessarily represent those of their affiliated organizations, or those of the publisher, the editors and the reviewers. Any product that may be evaluated in this article, or claim that may be made by its manufacturer, is not guaranteed or endorsed by the publisher.
References
Alauzet, C., Cunat, L., Wack, M., Lozniewski, A., Busby, H., Agrinier, N., et al. (2019). Hypergravity disrupts murine intestinal microbiota. Sci. Rep. 9, 9410. doi:10.1038/s41598-019-45153-8
Arumugam, M., Raes, J., Pelletier, E., Paslier, D., Yamada, T., Mende, D. R., et al. (2011). Enterotypes of the human gut microbiome. Nature 473, 174–180. doi:10.1038/nature09944
Aryal, S., Alimadadi, A., Manandhar, I., Joe, B., and Cheng, X. (2020). Machine learning strategy for gut microbiome-based diagnostic screening of cardiovascular disease. Hypertension 76, 1555–1562. doi:10.1161/HYPERTENSIONAHA.120.15885
Asnicar, F., Berry, S. E., Valdes, A. M., Nguyen, L. H., Piccinno, G., Drew, D. A., et al. (2021). Microbiome connections with host metabolism and habitual diet from 1,098 deeply phenotyped individuals. Nat. Med. 27, 321–332. doi:10.1038/s41591-020-01183-8
Avila-Herrera, A., Thissen, J., Urbaniak, C., Be, N. A., Smith, D. J., Karouia, F., et al. (2020). Crewmember microbiome may influence microbial composition of ISS habitable surfaces. PLoS One 15, e0231838. doi:10.1371/journal.pone.0231838
Bach, J. F. (2002). The effect of infections on susceptibility to autoimmune and allergic diseases. N. Engl. J. Med. 347, 911–920. doi:10.1056/NEJMra020100
Bezek, K., Petelin, A., Pražnikar, J., Nova, E., Redondo, N., Marcos, A., et al. (2020). Obesity measures and dietary parameters as predictors of gut microbiota phyla in healthy individuals. Nutrients 12, 2695. doi:10.3390/nu12092695
Biliński, J., Jasiński, M., and Basak, G. W. (2022). The role of fecal microbiota transplantation in the treatment of acute graft-versus-host disease. Biomedicines 10, 837. doi:10.3390/biomedicines10040837
Blottière, H. M., and Doré, J. (2016). Impact des nouveaux outils de métagénomique sur notre connaissance du microbiote intestinal et de son rôle en santé humaine. Medecine/Sciences 32, 944–951. doi:10.1051/medsci/20163211009
Boolchandani, M., D’Souza, A. W., and Dantas, G. (2019). Sequencing-based methods and resources to study antimicrobial resistance. Nat. Rev. Genet. 20, 356–370. doi:10.1038/s41576-019-0108-4
Bowers, R. M., Kyrpides, N. C., Stepanauskas, R., Harmon-Smith, M., Doud, D., Reddy, T. B. K., et al. (2017). Minimum information about a single amplified genome (MISAG) and a metagenome-assembled genome (MIMAG) of bacteria and archaea. Nat. Biotechnol. 35, 725–731. doi:10.1038/nbt.3893
Brereton, N. J. B., Pitre, F. E., and Gonzalez, E. (2021). Reanalysis of the Mars500 experiment reveals common gut microbiome alterations in astronauts induced by long-duration confinement. Comput. Struct. Biotechnol. J. 19, 2223–2235. doi:10.1016/j.csbj.2021.03.040
Brown, L. R., Fromme, W. J., Handler, S. F., Wheatcroft, M. G., and Johnston, D. A. (1976). Effect of Skylab missions on clinical and microbiologic aspects of oral health. J. Am. Dent. Assoc. 93, 357–363. doi:10.14219/jada.archive.1976.0502
Casero, D., Gill, K., Sridharan, V., Koturbash, I., Nelson, G., Hauer-Jensen, M., et al. (2017). Space-type radiation induces multimodal responses in the mouse gut microbiome and metabolome. Microbiome 5, 105. doi:10.1186/s40168-017-0325-z
Chen, Y., Xu, C., Zhong, C., Lyu, Z., Liu, J., Chen, Z., et al. (2021). Temporal characteristics of the oropharyngeal and nasal microbiota structure in crewmembers stayed 180 Days in the controlled ecological life support system. Front. Microbiol. 11, 617696. doi:10.3389/fmicb.2020.617696
Costea, P. I., Hildebrand, F., Manimozhiyan, A., Bäckhed, F., Blaser, M. J., Bushman, F. D., et al. (2017a). Enterotypes in the landscape of gut microbial community composition. Nat. Microbiol. 3, 8–16. doi:10.1038/s41564-017-0072-8
Costea, P. I., Zeller, G., Sunagawa, S., Pelletier, E., Alberti, A., Levenez, F., et al. (2017b). Towards standards for human fecal sample processing in metagenomic studies. Nat. Biotechnol. 35, 1069–1076. doi:10.1038/nbt.3960
Costello, S. P., Hughes, P. A., Waters, O., Bryant, R. V., Vincent, A. D., Blatchford, P., et al. (2019). Effect of fecal microbiota transplantation on 8-week remission in patients with ulcerative colitis: A randomized clinical trial. JAMA 32, 156–164. doi:10.1001/jama.2018.20046
Cotillard, A., Kennedy, S. P., Kong, L. C., Prifti, E., Pons, N., le Chatelier, E., et al. (2013). Dietary intervention impact on gut microbial gene richness. Nature 500, 585–588. doi:10.1038/nature12480
Crucian, B. E., Choukèr, A., Simpson, R. J., Mehta, S., Marshall, G., Smith, S. M., et al. (2018). Immune system dysregulation during spaceflight: potential countermeasures for deep space exploration missions. Front. Immunol. 9, 1437. doi:10.3389/fimmu.2018.01437
Cucinotta, F. A., Alp, M., Sulzman, F. M., and Wang, M. (2014). Space radiation risks to the central nervous system. Life Sci. Space Res. (Amst) 2, 54–69. doi:10.1016/j.lssr.2014.06.003
Demontis, G. C., Germani, M. M., Caiani, E. G., Barravecchia, I., Passino, C., and Angeloni, D. (2017). Human pathophysiological adaptations to the space environment. Front. Physiol. 8, 547. doi:10.3389/fphys.2017.00547
Falony, G., Joossens, M., Vieira-Silva, S., Wang, J., Darzi, Y., Faust, K., et al. (2016). Population-level analysis of gut microbiome variation. Science 352, 560–564. doi:10.1126/science.aad3503
Faucher, P., Dries, A., Mousset, P. Y., Leboyer, M., Dore, J., and Beracochea, D. (2022). Synergistic effects of Lacticaseibacillus rhamnosus GG, glutamine, and curcumin on chronic unpredictable mild stress-induced depression in a mouse model. Benef. Microbes 13, 253–264. doi:10.3920/BM2021.0188
Fernández-Edreira, D., Liñares-Blanco, J., and Fernandez-Lozano, C. (2021). Machine Learning analysis of the human infant gut microbiome identifies influential species in type 1 diabetes. Expert Syst. Appl. 185, 115648. doi:10.1016/j.eswa.2021.115648
Field, D., Sterk, P., Kottmann, R., De Smet, J. W., Amaral-Zettler, L., Cochrane, G., et al. (2014). Genomic standards consortium projects. Stand Genomic Sci. 9, 599–601. doi:10.4056/sigs.5559680
Garrett-Bakelman, F. E., Darshi, M., Green, S. J., Gur, R. C., Lin, L., Macias, B. R., et al. (2019). The nasa twins study: A multidimensional analysis of a year-long human spaceflight. Science 364, eaau8650. doi:10.1126/science.aau8650
Gopalakrishnan, V., Spencer, C. N., Nezi, L., Reuben, A., Andrews, M. C., Karpinets, T. V., et al. (2018). Gut microbiome modulates response to anti-PD-1 immunotherapy in melanoma patients. Science 359, 97–103. doi:10.1126/science.aan4236
Grimm, D., Grosse, J., Wehland, M., Mann, V., Reseland, J. E., Sundaresan, A., et al. (2016). The impact of microgravity on bone in humans. Bone 87, 44–56. doi:10.1016/j.bone.2015.12.057
Hao, Z., Li, L., Fu, Y., and Liu, H. (2018). The influence of bioregenerative life-support system dietary structure and lifestyle on the gut microbiota: A 105-day ground-based space simulation in lunar palace 1. Environ. Microbiol. 20, 3643–3656. doi:10.1111/1462-2920.14358
Ianiro, G., Punčochář, M., Karcher, N., Porcari, S., Armanini, F., Asnicar, F., et al. (2022). Variability of strain engraftment and predictability of microbiome composition after fecal microbiota transplantation across different diseases. Nat. Med. 28, 1913–1923. doi:10.1038/s41591-022-01964-3
Ilchmann-Diounou, H., and Menard, S. (2020). Psychological stress, intestinal barrier dysfunctions, and autoimmune disorders: an overview. Front. Immunol. 11, 1823. doi:10.3389/fimmu.2020.01823
Jenq, R. R., Taur, Y., Devlin, S. M., Ponce, D. M., Goldberg, J. D., Ahr, K. F., et al. (2015). Intestinal blautia is associated with reduced death from graft-versus-host disease. Biol. Blood Marrow Transplant. 21, 1373–1383. doi:10.1016/j.bbmt.2015.04.016
Jiang, P., Green, S. J., Chlipala, G. E., Turek, F. W., and Vitaterna, M. H. (2019). Reproducible changes in the gut microbiome suggest a shift in microbial and host metabolism during spaceflight. Microbiome 7, 113. doi:10.1186/s40168-019-0724-4
Jillings, S., van Ombergen, A., Tomilovskaya, E., Rumshiskaya, A., Litvinova, L., Nosikova, I., et al. (2020). Macro- and microstructural changes in cosmonauts’ brains after long-duration spaceflight. Sci. Adv. 6, eaaz9488. doi:10.1126/sciadv.aaz9488
Kang, D. W., Adams, J. B., Coleman, D. M., Pollard, E. L., Maldonado, J., McDonough-Means, S., et al. (2019). Long-term benefit of Microbiota Transfer Therapy on autism symptoms and gut microbiota. Sci. Rep. 9, 5821. doi:10.1038/s41598-019-42183-0
Kang, D. W., Adams, J. B., Gregory, A. C., Borody, T., Chittick, L., Fasano, A., et al. (2017). Microbiota transfer therapy alters gut ecosystem and improves gastrointestinal and autism symptoms: an open-label study. Microbiome 5, 10. doi:10.1186/s40168-016-0225-7
Kastl, A., Zong, W., Gershuni, V. M., Friedman, E. S., Tanes, C., Boateng, A., et al. (2022). Dietary fiber-based regulation of bile salt hydrolase activity in the gut microbiota and its relevance to human disease. Gut Microbes 14, 2083417. doi:10.1080/19490976.2022.2083417
Kéfi, S., Guttal, V., Brock, W. A., Carpenter, S. R., Ellison, A. M., Livina, V. N., et al. (2014). Early warning signals of ecological transitions: methods for spatial patterns. PLoS One 9, e92097. doi:10.1371/journal.pone.0092097
Kelly, J. R., Borre, Y., O’ Brien, C., Patterson, E., el Aidy, S., Deane, J., et al. (2016). Transferring the blues: depression-associated gut microbiota induces neurobehavioural changes in the rat. J. Psychiatr. Res. 82, 109–118. doi:10.1016/j.jpsychires.2016.07.019
Kuehnast, T., Abbott, C., Pausan, M. R., Pearce, D. A., Moissl-Eichinger, C., and Mahnert, A. (2022). The crewed journey to Mars and its implications for the human microbiome. Microbiome 10, 26. doi:10.1186/s40168-021-01222-7
Lasseur, C., Brunet, J., de Weever, H., Dixon, M., Dussap, G., Godia, F., et al. (2010). MELiSSA: the European project of closed life support system. Gravit. Space Res. 23, 3–12. Grand challenges in space synthetic biology. Available at: https://www.researchgate.net/publication/285646885_Grand_challenges_in_space_synthetic_biology (Accessed September 15, 2023).
Liñares-Blanco, J., Fernandez-Lozano, C., Seoane, J. A., and López-Campos, G. (2022). Machine learning based microbiome signature to predict inflammatory bowel disease subtypes. Front. Microbiol. 13, 872671. doi:10.3389/fmicb.2022.872671
Liu, Z., Luo, G., Du, R., Sun, W., Li, J., Lan, H., et al. (2020). Effects of spaceflight on the composition and function of the human gut microbiota. Gut Microbes 11, 807–819. doi:10.1080/19490976.2019.1710091
Llopis, M., Cassard, A. M., Wrzosek, L., Boschat, L., Bruneau, A., Ferrere, G., et al. (2016). Intestinal microbiota contributes to individual susceptibility to alcoholic liver disease. Gut 65, 830–839. doi:10.1136/gutjnl-2015-310585
Madison, A., and Kiecolt-Glaser, J. K. (2019). Stress, depression, diet, and the gut microbiota: human–bacteria interactions at the core of psychoneuroimmunology and nutrition. Curr. Opin. Behav. Sci. 28, 105–110. doi:10.1016/j.cobeha.2019.01.011
Malard, F., Dore, J., Gaugler, B., and Mohty, M. (2021a). Introduction to host microbiome symbiosis in health and disease. Mucosal Immunol. 14, 547–554. doi:10.1038/s41385-020-00365-4
Malard, F., Vekhoff, A., Lapusan, S., Isnard, F., D’incan-Corda, E., Rey, J., et al. (2021b). Gut microbiota diversity after autologous fecal microbiota transfer in acute myeloid leukemia patients. Nat. Commun. 12, 3084. doi:10.1038/s41467-021-23376-6
Marcos-Zambrano, L. J., Karaduzovic-Hadziabdic, K., Loncar Turukalo, T., Przymus, P., Trajkovik, V., Aasmets, O., et al. (2021). Applications of machine learning in human microbiome studies: A review on feature selection, biomarker identification, disease prediction and treatment. Front. Microbiol. 12, 634511. doi:10.3389/fmicb.2021.634511
Martinez, K. B., Leone, V., and Chang, E. B. (2017). Microbial metabolites in health and disease: navigating the unknown in search of function. J. Biol. Chem. 292, 8553–8559. doi:10.1074/jbc.R116.752899
Morrison, M. D., Thissen, J. B., Karouia, F., Mehta, S., Urbaniak, C., Venkateswaran, K., et al. (2021). Investigation of spaceflight induced changes to astronaut microbiomes. Front. Microbiol. 12, 659179. doi:10.3389/fmicb.2021.659179
Muñoz-Tamayo, R., Laroche, B., Walter, É., Doré, J., Duncan, S. H., Flint, H. J., et al. (2011). Kinetic modelling of lactate utilization and butyrate production by key human colonic bacterial species. FEMS Microbiol. Ecol. 76, 615–624. doi:10.1111/j.1574-6941.2011.01085.x
Paramsothy, S., Kamm, M. A., Kaakoush, N. O., Walsh, A. J., van den Bogaerde, J., Samuel, D., et al. (2017). Multidonor intensive faecal microbiota transplantation for active ulcerative colitis: A randomised placebo-controlled trial. Lancet 389, 1218–1228. doi:10.1016/S0140-6736(17)30182-4
Peled, J. U., Gomes, A. L. C., Devlin, S. M., Littmann, E. R., Taur, Y., Sung, A. D., et al. (2020). Microbiota as predictor of mortality in allogeneic hematopoietic-cell transplantation. N. Engl. J. Med. 382, 822–834. doi:10.1056/NEJMoa1900623
Philips, C. A., Pande, A., Shasthry, S. M., Jamwal, K. D., Khillan, V., Chandel, S. S., et al. (2017). Healthy donor fecal microbiota transplantation in steroid-ineligible severe alcoholic hepatitis: A pilot study. Clin. Gastroenterology Hepatology 15, 600–602. doi:10.1016/j.cgh.2016.10.029
Qin, J., Li, R., Raes, J., Arumugam, M., Burgdorf, K. S., Manichanh, C., et al. (2010). A human gut microbial gene catalogue established by metagenomic sequencing. Nature 464, 59–65. doi:10.1038/nature08821
Qin, N., Yang, F., Li, A., Prifti, E., Chen, Y., Shao, L., et al. (2014). Alterations of the human gut microbiome in liver cirrhosis. Nature 513, 59–64. doi:10.1038/nature13568
Ramirez, J., Guarner, F., Bustos Fernandez, L., Maruy, A., Sdepanian, V. L., and Cohen, H. (2020). Antibiotics as major disruptors of gut microbiota. Front. Cell. Infect. Microbiol. 10, 572912. doi:10.3389/fcimb.2020.572912
Ranaivo, H., Thirion, F., Béra-Maillet, C., Guilly, S., Simon, C., Sothier, M., et al. (2022). Increasing the diversity of dietary fibers in a daily-consumed bread modifies gut microbiota and metabolic profile in subjects at cardiometabolic risk. Gut Microbes 14, 2044722. doi:10.1080/19490976.2022.2044722
Raplee, I., Walker, L., Xu, L., Surathu, A., Chockalingam, A., Stewart, S., et al. (2021). Emergence of nosocomial associated opportunistic pathogens in the gut microbiome after antibiotic treatment. Antimicrob. Resist Infect. Control 10, 36. doi:10.1186/s13756-021-00903-0
Ridaura, V. K., Faith, J. J., Rey, F. E., Cheng, J., Duncan, A. E., Kau, A. L., et al. (2013). Gut microbiota from twins discordant for obesity modulate metabolism in mice. Science 341, 1241214. doi:10.1126/science.1241214
Ritchie, L. E., Taddeo, S. S., Weeks, B. R., Lima, F., Bloomfield, S. A., Azcarate-Peril, M. A., et al. (2015). Space environmental factor impacts upon murine colon microbiota and mucosal homeostasis. PLoS One 10, e0125792. doi:10.1371/journal.pone.0125792
Routy, B., le Chatelier, E., Derosa, L., Duong, C. P. M., Alou, M. T., Daillère, R., et al. (2018). Gut microbiome influences efficacy of PD-1–based immunotherapy against epithelial tumors. Science 359, 91–97. doi:10.1126/science.aan3706
Schaubeck, M., Clavel, T., Calasan, J., Lagkouvardos, I., Haange, S. B., Jehmlich, N., et al. (2016). Dysbiotic gut microbiota causes transmissible Crohn’s disease-like ileitis independent of failure in antimicrobial defence. Gut 65, 225–237. doi:10.1136/gutjnl-2015-309333
Scheffer, M., Carpenter, S., Foley, J. A., Folke, C., and Walker, B. (2001). Catastrophic shifts in ecosystems. Nature 413, 591–596. doi:10.1038/35098000
Schmidt, T. S. B., Li, S. S., Maistrenko, O. M., Akanni, W., Coelho, L. P., Dolai, S., et al. (2022). Drivers and determinants of strain dynamics following fecal microbiota transplantation. Nat. Med. 28, 1902–1912. doi:10.1038/s41591-022-01913-0
Sharon, G., Cruz, N. J., Kang, D. W., Gandal, M. J., Wang, B., Kim, Y. M., et al. (2019). Human gut microbiota from autism spectrum disorder promote behavioral symptoms in mice. Cell. 177, 1600–1618.e17. doi:10.1016/j.cell.2019.05.004
Sole, C., Llopis, M., Solà, E., da Silva, K., Guilly, S., Le-Chatelier, E., et al. (2018). Gut microbiome is profoundly altered in acute-on-chronic liver failure as evaluated by quantitative metagenomics. Relationship with liver cirrhosis severity. J. Hepatol. 68, S11–S12. doi:10.1016/S0168-8278(18)30240-X
Tanaka, K., Nishimura, N., and Kawai, Y. (2017). Adaptation to microgravity, deconditioning, and countermeasures. J. Physiological Sci. 67, 271–281. doi:10.1007/s12576-016-0514-8
Tap, J., Mondot, S., Levenez, F., Pelletier, E., Caron, C., Furet, J. P., et al. (2009). Towards the human intestinal microbiota phylogenetic core. Environ. Microbiol. 11, 2574–2584. doi:10.1111/j.1462-2920.2009.01982.x
Taur, Y., Jenq, R. R., Perales, M. A., Littmann, E. R., Morjaria, S., Ling, L., et al. (2014). The effects of intestinal tract bacterial diversity on mortality following allogeneic hematopoietic stem cell transplantation. Blood 124, 1174–1182. doi:10.1182/blood-2014-02-554725
Taylor, G. R., Graves, R. C., and Brockett, R. M. (1997). Skylab environmental and crew microbiology studies. NASA SP-3777. Washington, DC: NASA, Sci and Tech Inf Office, 53–63.
Turroni, S., Rampelli, S., Biagi, E., Consolandi, C., Severgnini, M., Peano, C., et al. (2017). Temporal dynamics of the gut microbiota in people sharing a confined environment, a 520-day ground-based space simulation, MARS500. Microbiome 5, 39. doi:10.1186/s40168-017-0256-8
Urbaniak, C., Lorenzi, H., Thissen, J., Jaing, C., Crucian, B., Sams, C., et al. (2020). The influence of spaceflight on the astronaut salivary microbiome and the search for a microbiome biomarker for viral reactivation. Microbiome 8, 56. doi:10.1186/s40168-020-00830-z
van de Guchte, M., Blottière, H. M., and Doré, J. (2018). Humans as holobionts: implications for prevention and therapy. Microbiome 6, 81. doi:10.1186/s40168-018-0466-8
van de Guchte, M., Burz, S. D., Cadiou, J., Wu, J., Mondot, S., Blottière, H. M., et al. (2020). Alternative stable states in the intestinal ecosystem: proof of concept in a rat model and a perspective of therapeutic implications. Microbiome 8, 153. doi:10.1186/s40168-020-00933-7
van de Guchte, M., Mondot, S., and Doré, J. (2021). Dynamic properties of the intestinal ecosystem call for combination therapies, targeting inflammation and microbiota, in ulcerative colitis. Ulcerative Colitis. Gastroenterol. 161, 1969–1981.e12. doi:10.1053/j.gastro.2021.08.057
Varanoske, A. N., McClung, H. L., Sepowitz, J. J., Halagarda, C. J., Farina, E. K., Berryman, C. E., et al. (2022). Stress and the gut-brain axis: cognitive performance, mood state, and biomarkers of blood-brain barrier and intestinal permeability following severe physical and psychological stress. Brain Behav. Immun. 101, 383–393. doi:10.1016/j.bbi.2022.02.002
Verseux, C., Baqué, M., Lehto, K., De Vera, J. P. P., Rothschild, L. J., and Billi, D. (2016). Sustainable life support on Mars - the potential roles of cyanobacteria. Int. J. Astrobiol. 15, 65–92. doi:10.1017/S147355041500021X
Vieira-Silva, S., Falony, G., Belda, E., Nielsen, T., Aron-Wisnewsky, J., Chakaroun, R., et al. (2020). Statin therapy is associated with lower prevalence of gut microbiota dysbiosis. Nature 581, 310–315. doi:10.1038/s41586-020-2269-x
Voorhies, A. A., Mark Ott, C., Mehta, S., Pierson, D. L., Crucian, B. E., Feiveson, A., et al. (2019). Study of the impact of long-duration space missions at the International Space Station on the astronaut microbiome. Sci. Rep. 9, 9911. doi:10.1038/s41598-019-46303-8
Vrieze, A., van Nood, E., Holleman, F., Salojärvi, J., Kootte, R. S., Bartelsman, J. F. W. M., et al. (2012). Transfer of intestinal microbiota from lean donors increases insulin sensitivity in individuals with metabolic syndrome. Gastroenterology 143, 913–916.e7. doi:10.1053/j.gastro.2012.06.031
Wilkinson, J. E., Franzosa, E. A., Everett, C., Li, C., Bae, S., Berzansky, I., et al. (2021). A framework for microbiome science in public health. Nat. Med. 27, 766–774. doi:10.1038/s41591-021-01258-0
Xue, H., Chen, X., Yu, C., Deng, Y., Zhang, Y., Chen, S., et al. (2022). Gut microbially produced indole-3-propionic acid inhibits atherosclerosis by promoting reverse cholesterol transport and its deficiency is causally related to atherosclerotic cardiovascular disease. Circ. Res. 131, 404–420. doi:10.1161/CIRCRESAHA.122.321253
Yilmaz, P., Kottmann, R., Field, D., Knight, R., Cole, J. R., Amaral-Zettler, L., et al. (2011). Minimum information about a marker gene sequence (MIMARKS) and minimum information about any (x) sequence (MIxS) specifications. Nat. Biotechnol. 29, 415–420. doi:10.1038/nbt.1823
Keywords: MELiSSA (Micro-Ecological Life Support System Alternative), microbiome, symbiosis, life support system, health and disease
Citation: Doré J and Ortega Ugalde S (2023) Human-microbes symbiosis in health and disease, on earth and beyond planetary boundaries. Front. Astron. Space Sci. 10:1180522. doi: 10.3389/fspas.2023.1180522
Received: 06 March 2023; Accepted: 11 September 2023;
Published: 22 September 2023.
Edited by:
Cyprien Verseux, University of Bremen, GermanyCopyright © 2023 Doré and Ortega Ugalde. This is an open-access article distributed under the terms of the Creative Commons Attribution License (CC BY). The use, distribution or reproduction in other forums is permitted, provided the original author(s) and the copyright owner(s) are credited and that the original publication in this journal is cited, in accordance with accepted academic practice. No use, distribution or reproduction is permitted which does not comply with these terms.
*Correspondence: Joel Doré, am9lbC5kb3JlQGlucmFlLmZy