- 1Department of Chemistry and Biochemistry, Utah State University, Logan, UT, United States
- 2Crop Physiology Laboratory, Utah State University, Logan, UT, United States
N2 fixation is essential to the sustainability and operation of nitrogen systems but is energetically expensive. We developed a model and used sensitivity analysis to identify the impact of aerobic and anaerobic waste digestion, crop harvest index, rates of recovery of recalcitrant N, and the rate of N2 fixation in a system combining nitrogen fixation and recycling. The model indicates that the rate of N2 fixation, loss from reactors, fertilization efficiency, and crop harvest index have the largest impact on maintaining bioavailable N. N recoveries from aerobic and anaerobic digestion, as well as direct-to-soil fertilization, are not well characterized, but the case studies using this model indicate that their efficiencies are critical to N recovery. The findings of this model and its presented case studies can be used as a guide in the design of closed-loop habitats both on Earth and in space. These results reveal a clear need for continued research in the areas of N-efficient digestion, fertilization, and fixation.
Introduction
Effective nitrogen (N) management that combines processes of N2 fixation (NF), waste removal, and recycling (R) is essential to the long-term success of closed-loop food production systems (Langenfeld et al., 2021). Acquiring N through N2 is vital for replenishing inevitable losses in systems that incorporate extensive recycling. Due to limitations resulting from the industrial requirements (such as high pressure, temperature, and energy demands) of abiotic N2 fixation methods (Haber-Bosch process), these methods will be difficult to implement for many closed systems, such as those developed for space missions (Giddey et al., 2020). Biological alternatives, capable of utilizing sustainable resources and operating under ambient conditions, are a potentially viable alternative to incorporate in the N management systems (Cherkasov et al., 2015). Fermenters employing N2-fixing heterotrophs may also be able to accept wastewater carbon (JH et al., 1979; Kulkarni et al., 2013; Pechter et al., 2016) and fixed N (Malofeeva, 1979) as substrates for microbial growth, thereby recycling waste N while accelerating N2 fixation.
Fermenters must be scaled to meet the colony’s N demands. Without recycling, these demands are significant and would lead to exorbitant fermenter volumes. Adding a recycling system reduces the N2 fixation demand from the entire colonial N consumption to the amount lost through recycling inefficiencies (Langenfeld et al., 2021). Recycling losses can be reduced by improving the efficiency of any factor, such as N harvestable from fermenters, N uptake by plants, crop harvest index, and process efficiency for both aerobic digestion (AE) and anaerobic digestion (AN). The rate of N2 fixation can also be optimized by ensuring optimal growth conditions, which in turn depend on temperature, pH, pressure, lighting, and media composition. The achievement of feasible fermenter volumes (dependent on the system used and available colonial infrastructure at the time of the mission) may be realized by the optimization of these system variables.
Here we present approaches for N management in a closed-loop habitat accounting for a variety of N management regiments, which account for N loss, N2 fixation, and N recycling. Two approaches featuring both recycling (R) and N2 fixation (NF) are compared: first, recycling and fixation occurring as separate processes, where reactors have the sole function of adding fixed N to the system (Separate NFR); second, combining recycling with N2 fixation as a means of supplying nutrients to N2-fixing bacteria and accelerating NF (Combined NFR) (Figure 1). The determination of the ideal regiment through these approaches is followed by sensitivity analyses, which test key variables for their overall influence on the demands and effectiveness of the greater system.
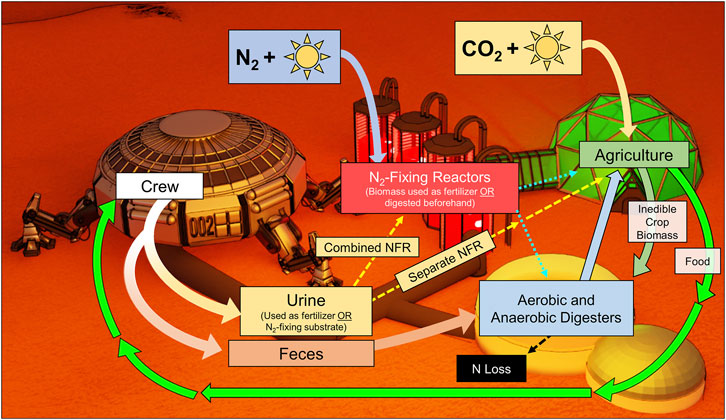
FIGURE 1. A Martian system encompassing both biological N2 fixation and recycling (NFR) may recycle urinary N using agriculture directly (Separate NFR) or reactors (Combined NFR). Biomass from N2-fixing reactors may also be used as fertilizer, either directly or after digestion.
Materials and methods
Each N system presented in this work utilizes the balance
where ∆N is the net change of N for the colony of size x crewmembers (CM) at time t, Nin is the mass of N from N2 fixed, Nrec is the N recycled, and Nout is maximum N loss without recycling. Nin, Nrec, Nout values have units of g CM−1 d −1. The recommended protein intake for a working CM is 90–100 g d−1, or about 14 g N d−1 (ND) (Langenfeld et al., 2021). For non-agricultural systems, in which all food consumed is food brought as cargo, ND is equal to the amount of N lost (Nout). For agricultural systems, Nout scales with each downstream inefficiency incurred from N2 fixation to food consumption. Each of the five models presented here accounts for these losses and are described in Figure 2.
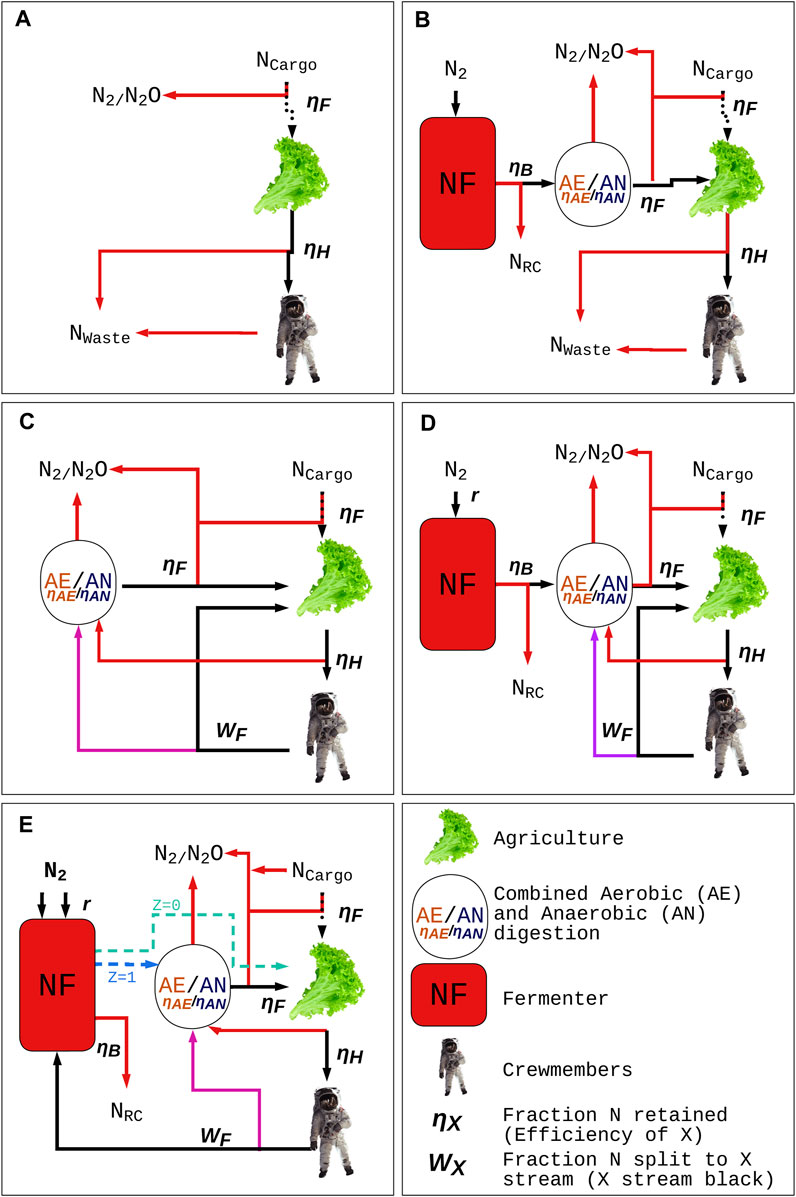
FIGURE 2. Five N management regiments modelled in this work. (A) -R, -NF: No recycling and no N2 fixation occurs, resulting in the most rapid N depletion. (B) +R: Recycling occurs without N2 fixation, dampening N depletion. (C) +NF: N2 fixation occurs without recycling, dampening N depletion. (D) Separate NFR: Recycling and N2 fixation occur in tandem, with fixed N externally supplied to the loop. (E) Combined NFR: Recycling occurs partially through N2 fixation, with fixed N internally supplied to the loop. Here, NCargo represents a supply of N brought to the colony that is expected to serve as an initial supply.
−NF, −R: no fixation, no recycling model (A)
Maximum N depletion occurs when no recycling or N2 fixation is performed. N loss is therefore assumed to be equal to potential losses incurred during fertilization and harvest, as well as the total N consumed by crewmembers (ND). Maximum depletion is modeled over time as
Where ηF is equal to the efficiency of fertilization (the fraction of N converted to crop biomass) and ηH is equal to the efficiency of harvest (the fraction of edible crop biomass).
+NF: fixation without recycling model (B)
The + NF Depletion model represents a system in which N losses are still equal to the losses incurred during fertilization, harvest, and consumption by crewmembers. However, ΔN for the colony is increased by the addition of N to the system by an NF fermenter. Attempting to replenish all lost N using the +NF system would likely demand very large reactor (>2500 L CM−1) and energy requirements, so depletion is still expected to occur over time and will be reduced by the addition of fixed N. NF depletion is modeled over time as
where Nout is as defined in Eq. 3, r is the volumetric rate of N2 fixation (g L−1 d−1), V is the reactor volume per crewmember (L CM−1), ηB is the fraction of N harvestable from the fermenter, η[x] is a function (Eq. 6) for calculating the weighted average of aerobic (AE) and anaerobic (AN) digestion efficiencies (ηAE andηAN, respectively, representing the fraction of N retained) based on flow fraction x, and WC is the fraction of microbial biomass fed to AE (with remainder to AN). Z is equal to 1 if biomass is treated in digesters prior to agriculture integration, or 0 otherwise.
+R: recycling without fixation model (C)
The + R Depletion model represents a system in which N losses are reduced through the recycling of various waste sources including inedible crop biomass (ICB) and human waste in urinary and fecal forms. AE and AN digestion processes are used to recycle ICB and feces, while N from urine in the form of urea is recycled directly back to crops. Inefficiencies stemming primarily from AE and AN result in gradual depletion over time. These losses may also be compounded by any N loss during fertilization. +R depletion is modeled over time as
where Nout is as defined in Eq. 3, WC is the fraction of ICB fed to AE (with remainder to AN), WW is the fraction of feces fed to AE (with remainder to AN), WF is the fraction of human waste N converted to feces (with remainder to urine), and ηU is the fraction of urine N recyclable. The function η[x] is as defined in Eq. 6. This assumes a complete conversion of consumed N to waste products in humans and complete feed of all waste to either AE or AN in the colony.
Separate NFR model (D)
The Separate NFR Model represents the first of the two proposed systems in which NF and R occur simultaneously and separately. This system forms a closed loop using R processes implementing AE and AN, with an NF fermenter acting to supply N from outside of the loop. The transfer of urea directly to agriculture (post-salts removal) serves to simplify the loop closure and to produce effective crop growth (Rathnaweera et al., 2019). The losses incurred because of recycling processes can be made up for through NF and drastically reduce the fermenter size required to break even. The Separate NFR system is modeled as
where Nin is as defined in Eq. 5, Nrec is as defined in Eq. 8, and Nout is as defined in Eq. 3.
Combined NFR model (E)
The Combined NFR Model makes the NF fermenter a part of the loop instead of an external N supplier. R processes are a part of the loop in this model as well. By cycling urine through the NF fermenter, heterotrophic microbial growth can be accelerated as fixed N and carbon sources are provided. Though N2-fixing microbes typically stop expressing nitrogenase in the presence of fixed N, the photoheterotrophic Rhodopseudomonas palustris (R. palustris) nifA* mutant expresses nitrogenase constitutively and may be able to fix N2 while consuming fixed N (Adessi et al., 2012; Heiniger et al., 2012). For organisms that do not constitutively express nitrogenase, limited amounts of fixed N may be used to accelerate growths at the beginning of fermenter cycles, which may also result in a greater rate of NF in addition to accomplishing N recycling (Lee et al., 1995). The increase in NF in both cases is assumed to be representable by a scalar multiplier of the standard fixation rate r. The Combined NFR Model is modeled as
where a is a scalar multiplier to account for an altered rate when fed with urea and other waste components, the η[x] function is as defined in Eq. 6, and Nrec is as defined in Eq. 8. As in +NF -R (B), Z is equal to 1 if biomass is treated in digesters prior to agriculture integration, or 0 otherwise. The second term in Eq. 10 represents the additional N loss incurred should reactor N be treated with digestor processes.
Running N models
With Formulas 1–10, simulations for each of the 5 N regiments were run. A literature review was conducted to determine likely values for each efficiency variable included. N2 fixation rates were estimated experimentally (Supplementary Table S1; Supplementary Table S2; Supplementary Figure S1). Maxima and minima were also tested to determine the maximum possible effect of each variable on N management. Table 1 shows the default values used in standard models, other feasible values tested, maxima, and minima. N depletion or accumulation (kg) over the course of 1 year (365 days) was compared using the Combined NFR model.
Equations 1–10 do not account for likely interdependencies between system variables. Digestion was assumed to positively impact fertilization efficiency by allocating greater bioavailable N to plants. The effect of digester efficiencies (ηAE and ηAN) on biomass fertilization efficiency (ηF) was accounted for by setting fertilization efficiency equal to 100% in digestive systems, and 90% otherwise.
Minimum Reactor Volumes (MRVs) were defined as the minimum reactor volume required to keep N supplies constant over time under a set of given conditions (such that Nin + Nrec = Nout). MRVs were useful in quantifying the influence of individual variables and regiments on N management and summarizing the results shown in sensitivity analyses. The influence (I) of a variable (X) on the system volume was quantified by taking the difference in MRVs at the maximum and minimum values (Eq. 11). The MRVs used in this work were calculated using the Combined NFR model.
Results
Figure 3 demonstrates the results of case studies evaluating all 5 N management regiments in which N2-fixing microbial biomass is either digested or not. At reactor volumes around 200 L CM−1 and above, combined with the assumptions of Table 1, the combined regiments outperform the separate regiments regardless of whether reactor biomass is digested. At higher reactor volumes (>700 L CM−1 with Table 1 defaults), the combined NFR model increasingly outperforms the separate NFR model (Supplementary Figure S1). However, lower digester efficiencies, lower reactor volumes, and higher urine recyclability all increase the performance of separate NFR models relative to the combined. Any or some combination of these factors may lead to separate NFRs performing superiorly.
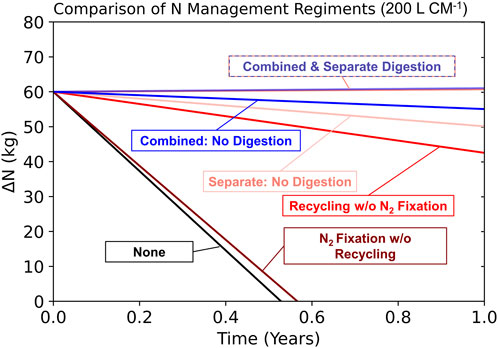
FIGURE 3. Comparison of 5 N regiments in which reactor biomass is digested or not using an N2-fixing reactor volume of 200 L/CM and an initial N supply of 60 kg.
Based on the Combined NFR model, individual variable tests were run using Table 1 values. The results of Figure 4 represent variable tests with a reactor size of 280 L CM−1 when digestion is bypassed. Digestion typically resulted in 10% lower amounts of N by the end of the year (365 days), and thus a 10% downward shift in each Figure 4 curve. WC and WW variables were omitted due to having no effect on N management, which is always the case in systems where aerobic and anaerobic N efficiencies are equal.
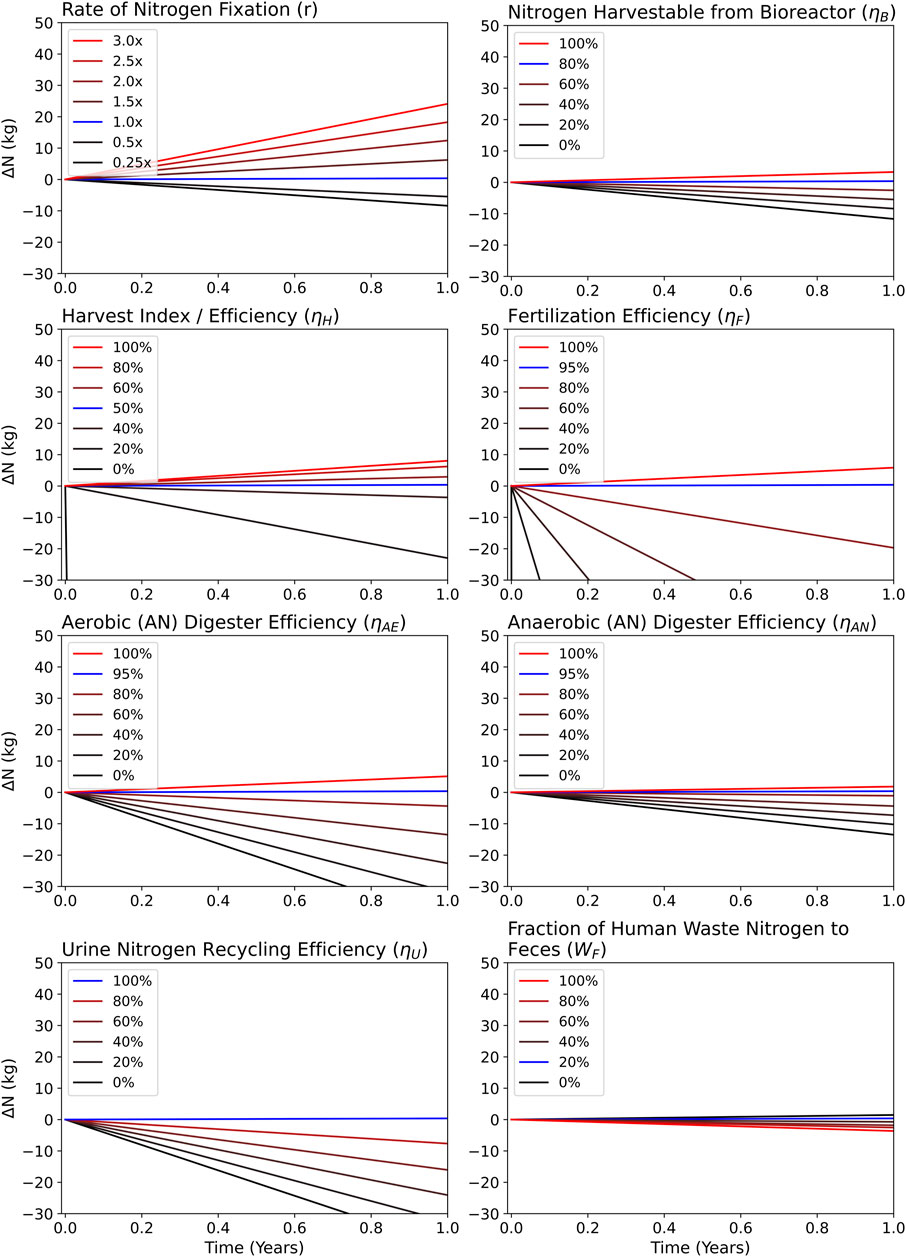
FIGURE 4. Results of sensitivity analyses measuring ΔN over time in which specified variables were changed between a series of relevant values.
Variables such as ηAE and ηAN became much less significant to N management when digestion was bypassed. When undergoing digestion, ηAN had an influence (IηAN) on MRV equal to about 615 L CM−1, which was lowered to about 244 L CM−1 when digestion was bypassed (60% decrease). ηAE was even more affected, lowering from an influence (IηAE) of nearly 8,000 L CM−1 to 700 L CM−1, representing a 91% decrease (Table 2).
The influence values of N management variables are shown in Table 2. Bioreactor harvest index (ηB), crop harvest index (ηC), and fertilization efficiency (ηF) had the most significant influence factors. The influence of urine recyclability (ηU) was consistent throughout digestion and no digestion. If a variable with a high influence dropped in efficiency, it required a significant increase in reactor volume to make up for the negative impact on N management. Conversely, efficiency drops in low-influence variables could be made up for by minor increases to reactor volume.
Discussion
The models presented in this work represent a mass balance of N and provide a means of comparing N management regiments under changing conditions. The sensitivity analyses demonstrated in Figure 4 offer insights into the overall influence of each variable on the system size, which will play a role in its power and resource demands, as well as overall equivalent system mass (ESM) (Levri et al., 2000; Ho et al., 2022). Not all inefficiencies are accounted for by this model, but the primary service of the model is its ability to be altered to account for additional demands or situations. The efficiencies and rates for model variables may be easily iterated as relevant systems are engineered and optimized. The purpose of testing minimum (as low as 0%) and maximum (often 100%, or as high as 300% in the case of r) is to illustrate the overall potential influence of each variable on the model and to guide future research efforts that seek to develop space technology.
Reactor volumes in the ranges specified (300–720 + L CM−1 d−1) could be prohibitive to early colonization efforts. Obtaining the most accurate estimates for reactor volumes possible will be essential in the design of mission systems. Additionally, while R. palustris was the foundation to the rates specified in the presented model, other organisms such as Azotobacter vinelandii (A. vinelandii) should also be considered. A. vinelandii tends to grow aerobically and up to 11 times the rate of R. palustris, which would likely correspond to a significantly lower reactor size (Valgardson, 2020). However, this would come at the cost of an increased oxygen demand. Microbiological N2-fixing systems advantageously operate at ambient temperatures and pressures using light or fixed carbon. However, reactor sizes and/or reagent demands may still impose a heavy burden on space missions. Smaller-scale alternatives, which may include electrochemical ammonia synthesis or future modifications to the Haber-Bosch process, may yet prove viable depending on the circumstances of a future mission.
The remainder of this discussion will include a breakdown of each variable tested, its role in a future N management and life support system, the basis for the variables tested, and any clear barriers preventing their optimization. Based on this model and the results displayed in Figures 3, 4, variables will be considered for their merit in current research endeavors and for their potential to benefit a sustainable habitat. The values assumed for each variable defined are the result of earth-based systems and will expectedly vary in space operations. Values may be iteratively modified to estimate performance as more research is performed on these or similar space systems.
Nitrogen demand (ND)
Daily protein intake required to sustain a healthy human diet varies anywhere from 0.8 to 1.6–2.0 g per kg bodyweight, depending on the level of activity and fitness of the individual (Forslund et al., 1999; Friedman and Lemon, 1989; Organization and others, 1985). Assuming N makes up 16% of protein mass and a mean crew body mass of 80 kg, this offers a range between 10.2 and 20.5 g CM−1 d−1. Moderately trained endurance athletes have been shown to break even on an N dose of 12 g d−1 and have a recommended intake around 16 g d−1 (Meredith et al., 1989; Tarnopolsky, 2004). A conservative estimate to maintain the health of physically active crewmembers was considered to be 14 g CM−1 d−1 and is a foundational assumption for the data presented in Figures 3, 4.
The selected N consumption rate of 14 g CM−1 d−1 assumes no N production besides dietary usage. Pharmaceutical and other types of production would add to the daily N demand of the colony. This value also assumes complete consumption of incoming fixed N supplies, and neither accounts for a surplus of nutrients nor its storage. With these factors considered, the N consumption rate should be treated as a bare minimum to support a healthy human diet, with zero additional demands or accumulation.
Fixation rate (r)
The N2 fixation rate r is very influential in the presented NF regiments, acting as the only significant means by which additional N may enter the closed-loop system. The rate itself is dependent on many variables in chemical engineering design, including N2 solubility in water, the N2-fixing organism (including its growth rate and metabolism), media composition, and reactor conditions (temperature, pH, pressure, mixing, and lighting where applicable). The reactor type (continuous flow stirred tank reactor (CFSTR), batch, plug flow, etc.) also will play a significant role in the rate of production. Future studies on scale-ups are essential to narrow down required operating values for microbiological N2-fixing systems.
To simplify the model, a constant daily output of reactor biomass was assumed based on observed laboratory data without accounting for growth kinetics. A more sophisticated reaction model may be developed in which a specific reactor type is selected and incorporated into the N management model. For a batch kinetic model, influxes of N would occur according to an estimated harvest interval, rather than a constant influx of N assumed in the presented model. On the other hand, for a CFSTR kinetic model, a constant influx of N may indeed be valid.
Regardless of the type of reactor modeled, downtimes should also be accounted for to estimate the effects of maintenance on N management. Reactor maintenance may result in several days or weeks in which no additional N is being added to the system, which would need to be accounted for in the design of any such system. The effects of random equipment failure, possibly resulting in even longer downtimes, may be easily modeled. The exact downtimes for maintenance or failure are dependent on the specific components and systems used.
For organisms capable of constitutive nitrogenase expression in the presence of fixed N, wastewater components may be added to create combined NFR systems. Both inhibition and facilitation of growth may occur depending on the composition of this waste stream. Determining inhibitory and facilitative components of wastewater will be essential in the design of wastewater treatment systems intending to recycle waste streams upstream to agriculture and/or fermenters. We consider a 65% increase in growth to be possible in streams containing reasonably high amounts of urea as well as trace amounts of acetic acid, propionic acid, glutamic acid, and other organic compounds supportive of growth (Verostko et al., 2004).
An in-depth analysis of reactor kinetics is beyond the scope of this work. However, such analyses will be an essential component of ongoing modeling and design of N management systems. Subsequent studies focusing on modeling reactor kinetics and output may be able to implement a similar finite differences approach as presented here. Additionally, a variety of software and libraries exist to support the modeling of chemical systems (Charlton & Parkhurst, 2011; Müller et al., 2011).
Future research on N2 fixation within the scope of this model must seek determination of 1) performance in upscaled systems; 2) ideal organisms across system types; 3) ideal reactor types and designs; 4) operational profiles with a variety of substrates and growth conditions; and 5) alternatives to biological N2 fixation systems. Assessing N2 fixation systems not only in terms of volume, but also mass, power, reliability, and resource demands will allow for a more direct assessment and determination of N2 fixation capabilities in life support systems.
Crop harvest index (ηH)
Crop harvest index ηH is very influential and appears to be one of the most controllable factors in N management, determined chiefly by the types of produce grown and species selected. Maximizing the percentage of high-harvest index produce such as potatoes, lettuce, beets, spinach, and onions would minimize waste between fertilization and consumption by humans. This is, of course, limited by the nutritional demands and dietary requirements of the colony. A crop harvest index of 50% has generally been a realistic average for a system that offers a greater variety of produce, including those with lower harvest indices, such as tomatoes and wheat. Different species of the same crop type appear to exhibit some variation in harvest indices as well: U.K. winter wheat has exhibited a range of 0.43–0.54 (Gent & Kiyomoto, 1989), while U.S. spring wheat may vary from 0.31 to 0.51 (McLaren, 1981). A selection of crops to meet the nutritional demands of a colony while maintaining a high harvest index will have a significant effect on colonial N management.
Bioreactor harvest index (ηB)
The bioreactor harvest index ηB is very influential in NF regiments, directly limiting the amount of N that can be added to the system. N “losses” described by this variable do not consist of N physically leaving the system, but rather represent a fraction of precipitated N that is effectively locked into the reactor system, unavailable to organisms and/or harvesting mechanisms (Marti et al., 2008; Feyissa et al., 2020; Achilleos et al., 2022). These inefficiencies result from processes such as struvite precipitation, recalcitration, and biofilm formation.
In laboratory experiments with R. palustris, biofilm formation was observed to occur on the sides of a batch reactor (Supplementary Figure S1). When biomass is harvested from media via centrifugation, the accumulation of biofilm ultimately has a negative effect on production, as biofilm blocks light entry and cannot be accessed via pumping. In batch systems, however, biofilm formation may become advantageous. Instead of liquid media harvests via pumping and centrifugation, biofilm reactors could allow larger amounts of biomass to simply be scraped off a scaffolding, similar to the operation of rotating algal biofilm reactors (Christenson & Sims, 2012). Successful biomass harvests without centrifugation would save a significant amount of energy over time (Young, 2011). N loss during bioreactor harvests has been estimated at 20%, with higher losses devastating N management in the presented model.
As growth kinetics are more accurately accounted for, the bioreactor harvest index will become more significant to account for N which may not be harvestable. The bioreactor harvest index may be reactor-dependent, with some designs and types allowing for more thorough harvests than others. Batch reactor systems may facilitate the removal of biofilm layers during harvest periods better than CFSTRs. Given that R. palustris depends on light to perform N fixation, and that biofilm accumulation inhibits light entry into the media, the reactor may require thorough biofilm harvests.
Fertilization efficiency (ηF)
Fertilization efficiency ηF is a measure of the fraction of N that is integrated into crop biomass in the agricultural stage and is very influential in all the regiments in this model. On Earth, ηF is predicted to be approximately 50% or less for large farms due to factors such as leaching and runoff (Smil, 1997; Ruiz et al., 2020). Nitrogen use efficiency is typically between 30% and 80% in terrestrial agriculture (Dobermann, 2005).
In open-loop N management systems, which encompasses most industrial terrestrial agriculture, fertilization losses result from a variety of inefficient practices. Nitrate leaching occurs when excess nitrate is applied. This anion is repelled by negatively charged soil particles and leaches into the surrounding environment. In poorly aerated root-zones, anaerobic conditions can lead to denitrification, which releases nitrous oxide and dinitrogen gases. Nitrous oxide is especially potent due to its greenhouse warming potential 300 times that of carbon dioxide and its destruction of atmospheric ozone (Griffis et al., 2017). When ammonium is applied to alkaline soils, equilibrium can promote the production of ammonia gas leading to increased losses. In well-aerated root-zones with pH control these losses should be minimized. A fertilization efficiency near 100% appears to be possible in precision liquid hydroponic systems using a mass balance guided approach to match nutrient delivery with plant requirements (Langenfeld et al., 2022). Runoff and leaching would represent major losses for other valuable resources such as water, but agricultural systems on Mars will use closed root-zones to eliminate these losses.
Continued research on fertilization efficiency is required to validate the high efficiencies assumed in this model. This would include measuring and optimizing N efficiency using biomass in a wider variety of agricultural system types. A soil-based approach to biomass fertilization may incur additional loss from foregoing biomass digestion (Foereid et al., 2021). Accurately accounting for N in soil-based, hydroponic, and aeroponic agricultural systems will be essential in the design of an N-efficient life support system. The results of this model suggest that high fertilization efficiencies, independent of digestion, would best support N management in a closed loop.
Aerobic and anaerobic digester (AE/AN) efficiency (ηAE/ηAN)
Little research has been done on N-efficient AE and AN systems. It has been shown in existing work that AE and AN have efficiencies around 75% and 50%, respectively (Novak et al., 2011). These were the approximate efficiency values assumed in the previous life support N-recycling models presented (Langenfeld et al., 2021). The surrounding research and literature around earth-based AE and AN processes differ in metrics, with the primary goal of such systems being the removal of N from waste streams regardless of form. Thus, reported efficiencies of 50% or 75% may refer to fixed N harvested from water and converted to usable forms (i.e., microbial biomass), concentrated in fixed forms (i.e., ammonium and nitrates), or lost to the environment as N2. In space life support systems, the majority of N must be kept in bioavailable forms for digestive recycling systems to work properly. The design and optimization of digestive and other recycling systems to achieve this goal represents an important pathway for future research.
Barring losses during bioreactor harvests and fertilization, AE and AN processes represent the only source of loss in the regiments incorporating these processes. N losses through AE and AN are proportional to the total N flow imposed on them. Reducing flow through these systems as much as possible while maintaining recyclability on each N stream will reduce losses and sensitivity to digester inefficiencies.
Exploring alternatives to biomass digestion is of particular importance to the combined NFR model. Otherwise, waste N from urine would be subjected to digestive losses that are avoided in the separated NFR model, significantly reducing the predicted gains that come from combining NF with recycling. The treatment of microbial biomass with sulfuric acid appears to be a viable alternative to digestion and can preserve N content (Valgardson, 2020).
N forms fed to plants are an important consideration in choosing AE or AN. Plants can take up amino acids, urea, nitrate, or ammonium as forms of N. Amino acids and urea can be taken up by plants in small amounts, but urea is largely hydrolyzed to ammonium prior to root uptake. Nitrate uptake is slower than ammonium uptake, but it may be stored in the vacuole and redistributed to cells as needed. Many studies show that plants given some ammonium in addition to nitrate grow better than those fed nitrate only (Zhang et al., 2019; Daiane et al., 2021). Ammonium is taken up much faster than the other three combined and can lead to potential toxicities because it cannot be stored by the plant like nitrate (Marschner, 2011). Excessive ammonium can lead to plant toxicity by inducing cationic nutrient deficiencies and decreasing rhizosphere pH.
AE remains a significant process in N management even without treating additional N from the fermenter. Maximizing the efficiency of AE will be important in minimizing the losses from crop harvest inefficiencies and the digestion of human feces. AN, however, appears to have a somewhat-limited influence in these circumstances, treating a smaller fraction of crop biomass (20%) and 50% of feces, which makes up only 10% of waste that ultimately undergoes digestion in the combined NFR model. AN maintains relevancy as a means of converting waste N to ammonia, which is an efficient N source for plants when supplied in limited amounts alongside nitrate (Zhu et al., 2021; Wang et al., 2022). AE differs from AN digestion further in terms of resource demands, energy, and time: AE requires O2 and high energy input while accomplishing digestion in a short time. Conversely, AN can be performed without O2 and with low energy input with longer digestion times. Although AE appears to be far more significant in N management, the extent to which it can be implemented may be limited by the energy demands and O2 production of the colony.
Urine recyclability (ηU)
About 90% of the N in urine is contained in urea (Kirchmann and Pettersson, 1994). Urea is hydrolyzed to ammonium via urease. This enzyme is ubiquitous among microorganisms and is also found in plants (Kryštofová et al., 2007). If urine contamination occurs after leaving the body, rapid hydrolysis may occur. Hydrolysis increases the pH of the urine, which can lead to ammonia volatilization (Ray et al., 2018). Acid addition is required to optimize recovery efficiency. A near-100% recovery of urea N from urine is achievable if contamination is minimized and acidic conditions are maintained (Langenfeld et al., 2021).
The remaining N in urine can be attributed to a variety of low-concentration ammonium salts, which are considerably more difficult to recycle. A variety of systems have been developed that can recover N in these forms. Bioelectrochemical (BEC) systems have been shown to be highly effective at recovering ammonium from wastewater and energy from organics. BEC systems convert ammonium to volatile ammonia, which may be recovered in liquid form. Recoveries of ammonium in BEC systems have been estimated between 94% and 97% (Wu & Modin, 2013). Recovery up to 80% has also been achieved through electrodialysis (Shi, Li). Membrane systems such as forward osmosis systems have been able to recover between 50% (Volpin et al., 2018) and 66% (Singh et al., 2019) of total urinary ammonia.
A combination of sterilization and acidification (for urea recovery) followed by BEC, electrodialysis, and/or membrane filtration methods (for ammonium recovery) would potentially allow for maximal N recovery from urine (Shi et al., 2018; Li et al., 2021). Effective capture of volatile ammonia will be necessary for systems such as BEC and will help ensure minimal losses during other steps.
Fraction biomass to AE (WC) and fraction feces to AE (WW)
Of these WX values, WC is the only variable that appears capable of significantly altering N management. This becomes the case when fermenter biomass is treated with digestion, in which a greater amount of N will be entering the digestion processes. Assuming that AE remains more efficient than AN, maximizing WC is valuable in minimizing digestive losses. This, however, must be balanced by any ammonia demand that has to be met via AN. The higher energy demand of AE must also be considered with increased inputs.
When fermenter biomass bypasses digestion processes, WC and WF appear to diminish drastically in their impacts on the system. The primary factors governing the proportion of biomass or waste to enter AE versus AN will likely be how much power is available to support AE, combined with the needs of nitrate or ammonia for crop fertilization and soil health. Assuming ample power and sufficient ammonia availability for fertilization, maximizing WC and WW would minimize N losses.
Fraction feces of waste (WF)
The fraction of N in human waste going to feces WF seems to be mostly dependent on diet, but rarely exceeds 20%, with the remainder exiting in urine (LAPID, 2004). This represents the fraction of N in human waste that must undergo digestion to be recycled, as urinary N can directly reenter the agricultural or NF steps (post-salts removal). The amount of N going to feces thus corresponds to higher losses, dependent on the efficiency of AE and AN. WF is not anticipated to exceed 20% by a significant margin, however, and is therefore unlikely to significantly alter N management.
Earth applications
The significance of boundary estimations for natural and man-made closed-loop systems has been well-established as a means of determining unsustainable practices and establishing sustainability milestones (Rockström et al., 2009; Steffen et al., 2015; Willett et al., 2019; Cousins et al., 2022). Additionally, poor N management has resulted in catastrophic effects on ecosystems across the U.S. and world at large (Glibert et al., 2014; Yarandi et al., 2021; Demertzioglou et al., 2022). The National Academy of Engineering has listed effective management of the N cycle as one of the grand challenges of the 21st century (Katsouleas et al., 2013). The application of similar boundary systems to Earth N management may help to identify key variables that can be changed to better control the flow of N into crops and the environment.
Applying the model presented here to N management in closed-loop systems on Earth naturally brings with it a host of challenges (Eid, 2000). While N management on an early Mars colony will be small-scale and precisely controlled, Earth N management encompasses global ammonia production from industrial and biotic processes amounting to over 200 million tons per year (Yarandi et al., 2021), its imprecise application to croplands, its application in other uses (i.e., pharmaceuticals, refrigeration, and textiles), and its entry into the environment as runoff and through volatilization. Earth’s N cycle occurs vastly through an established network linking industrial and natural N activity together, summarized by Figure 5.
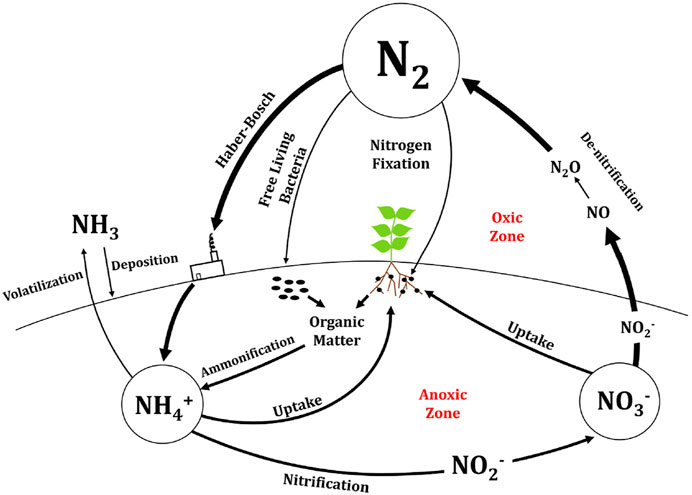
FIGURE 5. The nitrogen cycle on Earth (Langenfeld et al., 2021).
Applying N boundaries to N management on Earth can be simplified through a variety of means. The scope of the model may be restricted to specific regions and emphasize the practices and necessary changes in smaller communities. Additionally, some N uses may be ignored if they are negligible in comparison to agricultural N use. In-depth discussion on the application of this system to Earth N management is beyond the scope of this work. However, this model represents a prototype for a future analyses of N boundaries in space and on Earth.
Conclusion
Our model facilitates the comparison of 5 N management regiments, emphasizing the ease of variable manipulation. Two regiments employed methods of implementing N recycling and N2 fixation, in which urinary N was either recycled directly to crops (separate NFR) or used as a substrate to boost microbial N2 fixation (combined NFR). Combined NFR proved to generally outperform its counterpart, although this is often dependent on high fertilization efficiencies, < 75% digester efficiencies, and larger reactor volumes being present.
A series of variables relevant to N management systems, introduced by previous literature, were evaluated for their overall impacts on N management through a sensitivity analysis. The usage of aerobic and anaerobic digestion processes may have a significant impact on colonial N management, which may be minimized by incorporating alternative methods that still promote N conservation. Digester processes have the greatest effect when fixed N is cycled through them. According to this model, a system that bypasses digestion of fixed N while minimizing inputs to less efficient digestors (anaerobic) maximizes N conservation. The rate of N fixation, fertilization efficiencies, and fermenter and crop harvest indices also have significant impacts on the system. The optimization of each of these factors warrants future research in system design and operation.
This model exposes various gaps in knowledge on N efficiency throughout fixation, digestion, and fertilization processes. The merit of the presented approaches is limited by the accuracy of these variables. Future research must seek to improve the model itself and the variables it incorporates. These approaches will be refined by addressing issues including, 1) reactor and digester operation in a space environment; 2) infrastructural limitations on system operations, including upscaling effects, regular operational gaps for maintenance, and random system or component failures; 3) other sources of N loss.
Variables may be refined by continued experimentation in relevant subsystems. For the N2 fixation rate (r), optimization and modeling with regard to species, reactor types, and growth conditions is essential. For digesters, it is essential to determine and obtain maximum efficiency values, specifically regarding the percentage of bioavailable N that can be extracted from waste teams for recycled use. For agricultural systems, expanding data surrounding the performance of soil-based, hydroponic, and aeroponic fertilization using biomass versus inorganic fixed N is essential. Continued research in these and similar topics represents a next step in advancing the work presented here and raising its practical importance in the planning, design, and execution of future colonization efforts.
Data availability statement
The datasets presented in this study can be found in online repositories. The names of the repository/repositories and accession number(s) can be found in the article/Supplementary Material.
Author contributions
TW, DM, and NL contributed to the original manuscript draft. LS and BB guided the editing process, literature search, and figure deisgn. All authors contributed to the article and approved the submitted version.
Funding
Work supported by NASA under grand or cooperative agreement award number NNX17AJ31G.
Conflict of interest
The authors declare that the research was conducted in the absence of any commercial or financial relationships that could be construed as a potential conflict of interest.
Publisher’s note
All claims expressed in this article are solely those of the authors and do not necessarily represent those of their affiliated organizations, or those of the publisher, the editors and the reviewers. Any product that may be evaluated in this article, or claim that may be made by its manufacturer, is not guaranteed or endorsed by the publisher.
Supplementary material
The Supplementary Material for this article can be found online at: https://www.frontiersin.org/articles/10.3389/fspas.2023.1176576/full#supplementary-material
References
Achilleos, P., Roberts, K. R., and Williams, I. D. (2022). Struvite precipitation within wastewater treatment: A problem or a circular economy opportunity? Heliyon 8, e09862. doi:10.1016/j.heliyon.2022.e09862
Adessi, A., McKinlay, J. B., Harwood, C. S., and De Philippis, R. (2012). A Rhodopseudomonas palustris nifA* mutant produces H2 from -containing vegetable wastes. Int. J. hydrogen energy 37, 15893–15900. doi:10.1016/j.ijhydene.2012.08.009
Charlton, S. R., and Parkhurst, D. L. (2011). Modules based on the geochemical model PHREEQC for use in scripting and programming languages. Comput. Geosciences 37, 1653–1663. doi:10.1016/j.cageo.2011.02.005
Cherkasov, N., Ibhadon, A. O., and Fitzpatrick, P. (2015). A review of the existing and alternative methods for greener nitrogen fixation. Chem. Eng. Process. Process Intensif. 90, 24–33. doi:10.1016/j.cep.2015.02.004
Christenson, L. B., and Sims, R. C. (2012). Rotating algal biofilm reactor and spool harvester for wastewater treatment with biofuels by-products. Biotechnol. Bioeng. 109, 1674–1684. doi:10.1002/bit.24451
Cousins, I. T., Johansson, J. H., Salter, M. E., Sha, B., and Scheringer, M. (2022). Outside the safe operating space of a new planetary boundary for per-and polyfluoroalkyl substances (PFAS). Environ. Sci. Technol. 56, 11172–11179. doi:10.1021/acs.est.2c02765
Daiane, d. S. L. W., Daniele, F. d. O., Hudson, d. O. R., Guilherme, F. F., Luiz, A. A. G., Erica, C. A. L., et al. (2021). Nitrate concentration and nitrate/ammonium ratio on lettuce grown in hydroponics in Southern Amazon. Afr. J. Agric. Res. 17, 862–868. doi:10.5897/ajar2020.15087
Demertzioglou, M., Genitsaris, S., Mazaris, A. D., Kyparissis, A., Voutsa, D., Kozari, A., et al. (2022). A catastrophic change in a European protected wetland: From harmful phytoplankton blooms to fish and bird kill. Environ. Pollut. 312, 120038. doi:10.1016/j.envpol.2022.120038
Dobermann, A. R. (2005). Nitrogen use efficiency-state of the art. Nebraska: Agronomy–Faculty Publications, 316.
Eid, U. (2000). Closing the loop in wastewater management and sanitation. Bonn, Germany: Deutsche Gesellschaft für Technische Zusammenarbeit (GTZ) GmbH, 30–31.
Feyissa, A., Yang, F., Feng, J., Wu, J., Chen, Q., and Cheng, X. (2020). Soil labile and recalcitrant carbon and nitrogen dynamics in relation to functional vegetation groups along precipitation gradients in secondary grasslands of South China. Environ. Sci. Pollut. Res. 27, 10528–10540. doi:10.1007/s11356-019-07583-9
Foereid, B., Szocs, J., Patinvoh, R. J., and Sárvári Horváth, I. (2021). Effect of anaerobic digestion of manure before application to soil–benefor nitrogen utilisation? Int. J. Recycl. Org. Waste Agric. 10, 89–99. doi:10.30486/IJROWA.2020.1897538.1055
Forslund, A. H., El-Khoury, A. E., Olsson, R. M., Sjödin, A. M., Hambraeus, L., and Young, V. R. (1999). Effect of protein intake and physical activity on 24-h pattern and rate of macronutrient utilization. Am. J. Physiology-Endocrinology Metabolism 276, E964–E976. doi:10.1152/ajpendo.1999.276.5.e964
Friedman, J. E., and Lemon, P. W. R. (1989). Effect of chronic endurance exercise on retention of dietary protein. Int. J. sports Med. 10, 118–123. doi:10.1055/s-2007-1024886
Gent, M. P., and Kiyomoto, R. K. (1989). Assimilation and distribution of photosynthate in winter wheat cultivars differing in harvest index. Crop Sci. 29 (1), 120–125. doi:10.2135/cropsci1989.0011183x002900010028x
Giddey, S., Kulkarni, A., Ju, H., Paul, G., Lippi, R., and Alexander, D. (2020). “High performing catalyst for ammonia synthesis,“ in International Conference on Nanostructured Materials (NANO 2020) (Melbourne, Australia: Engineers Australia), 355.
Glibert, P. M., Maranger, R., Sobota, D. J., and Bouwman, L. (2014). The Haber Bosch–harmful algal bloom (HB–HAB) link. Environ. Res. Lett. 9, 105001. doi:10.1088/1748-9326/9/10/105001
Griffis, T. J., Chen, Z., Baker, J. M., Wood, J. D., Millet, D. B., Lee, X., et al. (2017). Nitrous oxide emissions are enhanced in a warmer and wetter world. Proc. Natl. Acad. Sci. 114, 12081–12085. doi:10.1073/pnas.1704552114
Hay, R. K. M. (1995). Harvest index: A review of its use in plant breeding and crop physiology. Ann. Appl. Biol. 126, 197–216. doi:10.1111/j.1744-7348.1995.tb05015.x
Heiniger, E. K., Oda, Y., Samanta, S. K., and Harwood, C. S. (2012). How posttranslational modification of nitrogenase is circumvented in Rhodopseudomonas palustris strains that produce hydrogen gas constitutively. Appl. Environ. Microbiol. 78, 1023–1032. doi:10.1128/aem.07254-11
Ho, D., Makrygiorgos, G., Hill, A., and Berliner, A. J. (2022). Towards an extension of equivalent system mass for human exploration missions on Mars. npj Microgravity 8, 30–10. doi:10.1038/s41526-022-00214-7
Jh, E. L. E. Y., Knobloch, K., and Tw, H. A. N. (1979). “Variability in enzymatic-activities under different growth-conditions,” in RHODOPSEUDOMONAS-PALUSTRIS (America: American Chemical Society), 255–256.
Katsouleas, T., Miller, R., and Yortsos, Y. (2013). The NAE grand challenge scholars program. Bridge 43, 53–56.
Kirchmann, H., and Pettersson, S. (1994). Human urine-chemical composition and fertilizer use efficiency. Fertilizer Res. 40, 149–154. doi:10.1007/bf00750100
Kryštofová, O., Hradecký, J., Zehnálek, J., Havel, L., Trnková, L., and Kizek, R. (2007). Comparison of spectrophotometry to electrochemical methods of urease determination.
Kulkarni, G., Wu, C.-H., and Newman, D. K. (2013). The general stress response factor EcfG regulates expression of the C-2 hopanoid methylase HpnP in Rhodopseudomonas palustris TIE-1. J. Bacteriol. 195, 2490–2498. doi:10.1128/jb.00186-13
Langenfeld, N. J., Kusuma, P., Wallentine, T., Criddle, C. S., Seefeldt, L. C., and Bugbee, B. (2021). Optimizing nitrogen fixation and recycling for food production in regenerative life support systems. Front. Astronomy Space Sci. 8, 105. doi:10.3389/fspas.2021.699688
Langenfeld, N. J., Pinto, D. F., Faust, J. E., Heins, R., and Bugbee, B. (2022). Principles of nutrient and water management for indoor agriculture. Sustainability 14, 10204. doi:10.3390/su141610204
Lapid, D. G. (2004). Ecological sanitation, an approach to sustainable sanitation. Quezon City, Philippines: Center for Advanced Philippine Studies, Inc., 2–7.
Lee, I.-Y., Stegantseva, E.-M., Savenkova, L., and Park, Y.-H. (1995). Effects of Nitrogen and Oxygen Supply on Production of Poly-β-Hydroxybutyrate in Azotobacter chroococcum. J. Microbiol. Biotechnol. 5, 100–104.
Levri, J. A., Vaccari, D. A., and Drysdale, A. E. (2000). Theory and application of the equivalent system mass metric. Toulouse, France: SAE International.
Li, Y., Wang, R., Shi, S., Cao, H., Yip, N. Y., and Lin, S. (2021). Bipolar membrane electrodialysis for ammonia recovery from synthetic urine: Experiments, modeling, and performance analysis. Environ. Sci. Technol. 55, 14886–14896. doi:10.1021/acs.est.1c05316
Marti, N., Bouzas, A., Seco, A., and Ferrer, J. (2008). Struvite precipitation assessment in anaerobic digestion processes. Chem. Eng. J. 141, 67–74. doi:10.1016/j.cej.2007.10.023
McLaren, J. S. (1981). Field studies on the growth and development of winter wheat. J. Agric. Sci. 97 (03), 685–697. doi:10.1017/s0021859600037059
Meredith, C. N., Zackin, M. J., Frontera, W. R., and Evans, W. J. (1989). Dietary protein requirements and body protein metabolism in endurance-trained men. J. Appl. Physiology 66, 2850–2856. doi:10.1152/jappl.1989.66.6.2850
Müller, M., Parkhurst, D. L., and Charlton, S. R. (2011). Programming PHREEQC calculations with C++ and Python a comparative study. EXCHANGE 1, 632–636.
Novak, J. T., Banjade, S., and Murthy, S. N. (2011). Combined anaerobic and aerobic digestion for increased solids reduction and nitrogen removal. Water Res. 45, 618–624. doi:10.1016/j.watres.2010.08.014
Organization, W. H. (1985). “Energy and protein requirements: Report of a joint FAO/WHO/UNU expert consultation,” in Energy and protein requirements: Report of a joint FAO/WHO/UNU expert consultation (Switzerland: WHO), 206.
Pechter, K. B., Gallagher, L., Pyles, H., Manoil, C. S., and Harwood, C. S. (2016). Essential genome of the metabolically versatile alphaproteobacterium Rhodopseudomonas palustris. J. Bacteriol. 198, 867–876. doi:10.1128/jb.00771-15
Rathnaweera, D. N., Pabodha, D., Sandaruwan, C., Priyadarshana, G., Deraniyagala, S. P., and Kottegoda, N. (2019). Urea modified calcium carbonate nanohybrids as a next generation fertilizer. Sri Lanka: Kotelawala Defence University.
Ray, H., Saetta, D., and Boyer, T. H. (2018). Characterization of urea hydrolysis in fresh human urine and inhibition by chemical addition. Environ. Sci. Water Res. Technol. 4, 87–98. doi:10.1039/c7ew00271h
Rockström, J., Steffen, W., Noone, K., Persson, Å., Chapin, F. S., Lambin, E. F., et al. (2009). A safe operating space for humanity. nature 461, 472–475. doi:10.1038/461472a
Ruiz, S. A., McKay Fletcher, D., Boghi, A., Williams, K., Duncan, S., Scotson, C., et al. (2020). Image-based quantification of soil microbial dead zones induced by nitrogen fertilization. Sci. Total Environ. 727, 138197. doi:10.1016/j.scitotenv.2020.138197
Salisbury, F. B., Gitelson, J. I., and Lisovsky, G. M. (1997). Bios-3: Siberian experiments in bioregenerative life support. BioScience 47, 575–585. doi:10.2307/1313164
Shi, L., Hu, Y., Xie, S., Wu, G., Hu, Z., and Zhan, X. (2018). Recovery of nutrients and volatile fatty acids from pig manure hydrolysate using two-stage bipolar membrane electrodialysis. Chem. Eng. J. 334, 134–142. doi:10.1016/j.cej.2017.10.010
Singh, N., Dhiman, S., Basu, S., Balakrishnan, M., Petrinic, I., and Helix-Nielsen, C. (2019). Dewatering of sewage for nutrients and water recovery by Forward Osmosis (FO) using divalent draw solution. J. Water Process Eng. 31, 100853. doi:10.1016/j.jwpe.2019.100853
Smil, V. (1997). Global population and the nitrogen cycle. Sci. Am. 277, 76–81. doi:10.1038/scientificamerican0797-76
Steffen, W., Richardson, K., Rockström, J., Cornell, S. E., Fetzer, I., Bennett, E. M., et al. (2015). Sustainability. Planetary boundaries: Guiding human development on a changing planet. science 347, 1259855. doi:10.1126/science.1259855
Tarnopolsky, M. (2004). Protein requirements for endurance athletes. Eur. J. Sport Sci. 4, 1–15. doi:10.1080/17461390400074102
Valgardson, K. (2020). Utilizing earth's microbiology to develop the framework for a manufactured martian nitrogen cycle. United States: Utah State University.
Verostko, C. E., Carrier, C., and Finger, B. W. (2004). Ersatz wastewater formulations for testing water recovery systems. SAE Trans., 1008–1024. doi:10.4271/2004-01-2448
Volpin, F., Chekli, L., Phuntsho, S., Cho, J., Ghaffour, N., Vrouwenvelder, J. S., et al. (2018). Simultaneous phosphorous and nitrogen recovery from source-separated urine: A novel application for fertiliser drawn forward osmosis. Chemosphere 203, 482–489. doi:10.1016/j.chemosphere.2018.03.193
Wang, R., Zhang, Z., Lv, F., Lin, H., Wei, L., and Xiao, Y. (2022). Optimizing the bacterial community structure and function in rhizosphere soil of sesame continuous cropping by the appropriate nitrate ammonium ratio. Rhizosphere 23, 100550. doi:10.1016/j.rhisph.2022.100550
Willett, W., Rockström, J., Loken, B., Springmann, M., Lang, T., Vermeulen, S., et al. (2019). Food in the anthropocene: The EAT–lancet commission on healthy diets from sustainable food systems. Lancet 393, 447–492. doi:10.1016/s0140-6736(18)31788-4
Wu, X., and Modin, O. (2013). Ammonium recovery from reject water combined with hydrogen production in a bioelectrochemical reactor. Bioresour. Technol. 146, 530–536. doi:10.1016/j.biortech.2013.07.130
Yarandi, M. S., Mahdinia, M., Barazandeh, J., and Soltanzadeh, A. (2021). Evaluation of the toxic effects of ammonia dispersion: Consequence analysis of ammonia leakage in an industrial slaughterhouse. Med. gas Res. 11, 24. doi:10.4103/2045-9912.310056
Young, A. M. (2011). Zeolite-based algae biofilm rotating photobioreactor for algae biomass production. United States: Utah State University.
Zhang, J., Lv, J., Dawuda, M. M., Xie, J., Yu, J., Li, J., et al. (2019). Appropriate ammonium-nitrate ratio improves nutrient accumulation and fruit quality in pepper (Capsicum annuum L). Agronomy 9, 683. doi:10.3390/agronomy9110683
Keywords: life support systems, nitrogen management, biological N2 fixation, sustainabilty, waste digestion, crop harvest index, nitrogen recovery, space system
Citation: Wallentine T, Merkley D, Langenfeld NJ, Bugbee B and Seefeldt LC (2023) Approaches to nitrogen fixation and recycling in closed life-support systems. Front. Astron. Space Sci. 10:1176576. doi: 10.3389/fspas.2023.1176576
Received: 28 February 2023; Accepted: 03 July 2023;
Published: 03 August 2023.
Edited by:
Parag Vaishampayan, National Aeronautics and Space Administration, United StatesCopyright © 2023 Wallentine, Merkley, Langenfeld, Bugbee and Seefeldt. This is an open-access article distributed under the terms of the Creative Commons Attribution License (CC BY). The use, distribution or reproduction in other forums is permitted, provided the original author(s) and the copyright owner(s) are credited and that the original publication in this journal is cited, in accordance with accepted academic practice. No use, distribution or reproduction is permitted which does not comply with these terms.
*Correspondence: Tyler Wallentine, dHlsZXIud2FsbGVudGluZUB1c3UuZWR1