- 1The Aerospace Corporation, El Segundo, CA, United States
- 2John Hopkins Applied Physics Laboratory, Laurel, MD, United States
- 3Laboratory for Atmospheric and Space Physics, University of Colorado, Boulder, CO, United States
- 4Electrical and Computer Engineering Department, Boston University, Boston, MA, United States
- 5Earth, Planetary, and Space Sciences Department, University of California, Los Angeles, Los Angeles, CA, United States
- 6Atmospheric and Ocean Sciences Department, UCLA, Los Angeles, CA, United States
- 7Space Science Institute, Boulder, CO, United States
- 8Department of Physics, University of Texas, Arlington, TX, United States
- 9Department of Physics and Astronomy and Space Science Center, University of New Hampshire, Durham, NH, United States
- 10Department of Physis and Astronomy, Dartmouth College, Hanover, NH, United States
- 11NCAR HAO, Boulder, CO, United States
- 12NASA Goddard Space Flight Center, Greenbelt, MD, United States
- 13The Catholic University of America, Department of Physics, Washington, DC, United States
- 14Department of Physics and Astronomy, University of Calgary, Calgary, AB, Canada
- 15Department of Physics and Astronomy, Clemson University, Clemson, SC, United States
- 16Department of Climate and Space Sciences and Engineering, University of Michigan, Ann Arbor, MI, United States
An important question that is being increasingly studied across subdisciplines of Heliophysics is “how do mesoscale phenomena contribute to the global response of the system?” This review paper focuses on this question within two specific but interlinked regions in Near-Earth space: the magnetotail’s transition region to the inner magnetosphere and the ionosphere. There is a concerted effort within the Geospace Environment Modeling (GEM) community to understand the degree to which mesoscale transport in the magnetotail contributes to the global dynamics of magnetic flux transport and dipolarization, particle transport and injections contributing to the storm-time ring current development, and the substorm current wedge. Because the magnetosphere-ionosphere is a tightly coupled system, it is also important to understand how mesoscale transport in the magnetotail impacts auroral precipitation and the global ionospheric system response. Groups within the Coupling, Energetics and Dynamics of Atmospheric Regions Program (CEDAR) community have also been studying how the ionosphere-thermosphere responds to these mesoscale drivers. These specific open questions are part of a larger need to better characterize and quantify mesoscale “messengers” or “conduits” of information—magnetic flux, particle flux, current, and energy—which are key to understanding the global system. After reviewing recent progress and open questions, we suggest datasets that, if developed in the future, will help answer these questions.
1 Introduction
The question of how mesoscale phenomena contribute to the global response of the geospace system has remained unanswered for decades, but has come to the forefront of our science in recent years thanks to 1) the availability of multipoint datasets (e.g., Cluster, THEMIS) that allowed repeated observations on the appropriate (meso)scales combined with ground-based (synoptic) imaging and magnetometer networks, and 2) the impressive resolution improvements in our global models made possible by larger computing power. “Mesoscale” is regime specific, broadly defined as larger than kinetic scale phenomena and smaller than global responses. In the magnetotail, mesoscale is defined as larger than the ion gyroradius (roughly hundreds km and greater) up to a few Earth radii (RE). In the ionosphere, mesoscale phenomena range from tens to ∼500 km in width (see Table 1 for summary). The range is approximate, not exact—for example, some consider up to 1,000 km “mesoscale” in the ionosphere. As simulations are starting to achieve mesoscale resolution, it has become apparent how global, statistical models fail to capture the dynamic and powerful phenomena that occur on mesoscales. This knowledge gap propagates down and across different regimes in space physics and aeronomy. For example, mesoscale plasma flows in the magnetotail map to the ionosphere and have important effects on neutral wind response (Deng et al., 2019) and neutral density perturbations (Deng et al., 2009; Sheng et al., 2021). The difficulty in characterizing mesoscale phenomena to determine their relationship to the global response therefore has far-reaching effects.
Through a community-wide effort to improve understanding of the role of mesoscale phenomena in the magnetosphere-ionosphere (MI) system, an NSF Geospace Environment Modeling (GEM) Focus Group on Magnetotail Dipolarizations and their Effects in the Inner Magnetosphere organized discussions over the last 6 years, concluding in 2023 (2017–2023). Discussions often focused on the question of whether or not mesoscale phenomena like Bursty Bulk Flows (BBFs) (Angelopoulos et al., 1994; 1997) and Dipolarizing Flux Bundles (DFBs) (Liu et al., 2014) are the major source of current, magnetic flux transport, and energy transport in the system. Much of the discussion coalesced around Earth’s transition region, the region spanning ∼6–12 RE where the dipole magnetic field near Earth transitions to a stretched magnetotail. The transition region is where earthward-traveling mesoscale phenomena brake and deposit their information (e.g., energy, particles, magnetic flux), but how that information is ultimately dissipated to the inner magnetosphere or ionosphere remains a mystery. Realizing the importance in understanding how the transition region filters energy/information, a new GEM focus group was formed in 2022 to specifically study it (“Mesoscale drivers of the nightside transition region: ionospheric and magnetotail evaluations”). Meanwhile, for the past ∼6–7 years (2016–2022), an Air Force Office of Scientific Research (AFOSR) supported Multidisciplinary University Research Initiative (MURI) project focused on improving the specification of the energy and momentum inputs from the magnetosphere into the upper atmosphere, especially at mesoscales. This group has been active in NSF’s Coupling, Energetics and Dynamics of Atmospheric Regions (CEDAR) Program, as well as at GEM. The following presents a summary of some of the GEM and MURI discussions to orient the reader on the field’s status, as well as outstanding questions and suggestions for a path forward. We acknowledge that works outside this scope will be missed in this review.
2 A summary of the current debates and discussions
When the GEM focus group on dipolarizations first began, the community sat down and defined terminology to ensure language was consistent (see Table 1). Dipolarization is recognized as the stretched magnetotail returning to a more dipolar state. Initially studied at geosynchronous orbit (e.g., McPherron, 1972), the Bz increase was interpreted as a reconfiguration of the magnetotail often seen at the onset of the substorm expansion phase. It would slowly return to the stretched, pre-dipolarization level during the substorm recovery, making the timescale on the order of an hour to an hour and a half. Table 1 refers to this reconfiguration as “global” or “large-scale” dipolarization to differentiate it from mesoscale dipolarizing flux bundles (DFBs), which were named much later during the THEMIS mission (Liu et al., 2014). Dipolarizing flux bundles can be thought of as narrow flux tubes racing earthward and becoming more dipolar after forming from reconnection. The mechanics of the earthward motion are described in papers like Li et al. (1998) (in terms of force balance) or Wolf et al. (2002; 2006; 2009) (in terms of low entropy bubbles). They are embedded within fast plasma flows (often, bursty bulk flows (Angelopoulos et al., 1994; 1997)) and are on the order of 1–3 RE wide in azimuth. A satellite observes them as a sudden increase in Bz (the dipolarization front) followed by an elevated Bz for tens of seconds to minutes. We note that the lifetime of the DFB is not easy to track observationally, as the satellite only observes it for the duration the DFB is passing over the satellite. The DFB decelerates as it approaches Earth until it either crashes into the dipolar region of Earth’s magnetosphere and “piles-up” magnetic flux at the transition region between Earth’s dipolar field lines and its stretched magnetotail, or is diverted around Earth’s dipolar region towards the dayside. The magnetic flux “pile-up” was reported by Baumjohann et al. (1999) as a tailward propagation of the dipolar region. Although they used the term “dipolarization front” to describe the increase in Bz as it propagated tailward, nomenclature today uses the term to refer to the kinetic-scale Bz increase that marks the earthward-leading edge of the dipolarizing flux bundle separating the cold ambient plasma sheet from the hot, tenuous plasma inside the DFB. Dipolarization fronts were heavily studied with the Cluster mission (Nakamura et al., 2004) and later by THEMIS and MMS. See Fu et al. (2020) for a more extensive review on both dipolarization fronts and the particle acceleration related to them. On a tangential but related note, dipolarizations in the inner magnetosphere have been observed with Bz increasing to values larger than the perfect dipole, bringing into question the terminology choice as technically they are “over-dipolarizations”. The question of whether the earthward magnetic flux transport via mesoscale DFBs is enough to account for the global dipolarization seen near geosynchronous orbit (GEO) and within Earth’s transition region was debated and is summarized in Section 2.1.
Historically, large-scale dipolarization was considered the cause of the substorm current wedge (see Kepko et al., 2015 for a thorough review). As the drift rates of electrons and protons across the region of enhanced Bz is altered, the total current across the dipolarized region is reduced and the cross-tail current in the undisturbed region splits, with some portion flowing downward to the ionosphere. As the field lines bend away from midnight on the eastern and western sides of the dipolarization, field-aligned currents must form. Later works suggested that an alternative cause of the substorm current wedge is a compilation of smaller “wedgelets” of field-aligned currents that form from the earthward-traveling DFBs. This question has been debated and is discussed in Section 2.2.
Also historically observed with global dipolarizations are particle injections. Typically observed as sudden increases in particle fluxes across multiple energies, they were named “injections” because they transport—or, “inject”—particles into the inner magnetosphere from the plasma sheet, energizing them in the process [See Gabrielse et al. (2017b) for a more comprehensive historical review.]. Reeves et al. (1990) constrained their scale size at GEO to a few hours in magnetic local time (MLT). Injections can also result in a sudden flux decrease across energy channels if the sign of the phase space density radial gradient is switched, such that the new, injected population has a lower phase space density than the original population. These flux decreases were first reported by Sergeev et al. (1992) as drifting electron holes at geosynchronous orbit, but were further studied at greater distances from Earth as well (e.g.; Cohen et al., 2019; Liu et al., 2019). Following the theme, mesoscale injections are related to DFBs and the DFB-related electric fields which transport and energize particles earthward. As Section 2.3 discusses, open questions remain whether mesoscale injections contribute to the large-scale injections seen at GEO, and if they are important contributors to the ring current—or if they are simply ripples on top of the global particle transport into Earth’s inner magnetosphere.
How much energy is transported from the magnetosphere to the ionosphere on mesoscales is also a topic that is getting wide attention from both GEM and CEDAR communities. Section 2.4 does not attempt to summarize all the work that has been done in this area, but rather touches on some works and ideas specific to the GEM Dipolarization Focus Group and MURI project referenced above to whet the reader’s appetite and highlight the importance of continuing to probe the contributions by mesoscales in magnetosphere-ionosphere coupling.
2.1 In terms of magnetic flux
Many papers came out of the GEM debate discussing whether mesoscale transport of magnetic flux can explain the large-scale dipolarization that occurs at Earth’s transition region (see Figure 1 for schematic illustration). Most conclude that “yes”, mesoscale flux transport is adequate to explain the majority of magnetic flux transport globally, with one dissenting paper suggesting 50–1000 DFBs are required to fully explain all the flux transport—and that is only if the frozen in condition is maintained (Lui, 2015). Figure 2 (from Birn et al., 2019) plots the magnetic flux over time at seven different locations across the tail (in YGSM) from a magnetohydrodynamics (MHD) model. They found that the total flux transported earthward from the reconnection site was ∼203MWb, commensurate with estimates from data of 100 MWb–360 MWb by Angelopoulos et al. (1994). This flux was associated with up to seven DFB events localized across the tail, not one single mesoscale transport event. Merkin et al. (2019) utilized the Lyon–Fedder–Mobarry (LFM) global MHD code (Figure 3) to determine that the total amount of earthward flux entering the 8 RE region between 20:00 and 04:00 magnetic local time (MLT) was ∼50 MWb over roughly 30 min after substorm onset, which is in line with expectations from observations in the plasma sheet (e.g., Liu et al., 2014).
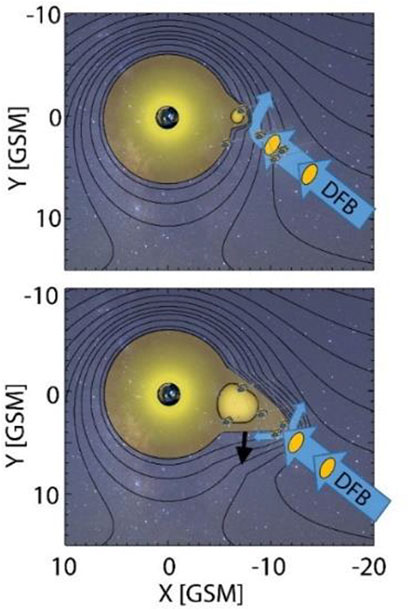
FIGURE 1. Modified from Gabrielse et al., 2019a. Illustration of mesoscale messengers of magnetic flux (DFBs) & particle flux (injections) as building blocks of the global dipolarization/injection.
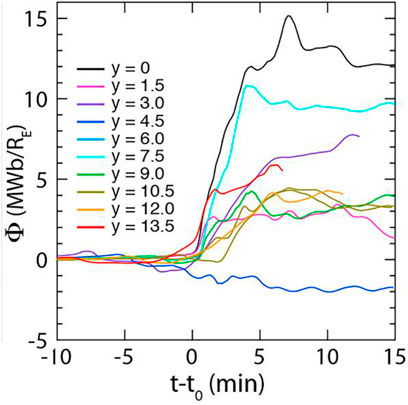
FIGURE 2. From Birn et al., 2019. Evolution of magnetic flux (Φ) values in the simulation for 10 evenly spaced YGSM values (indicated by different colors) at x = −13.5RE, relative to the onset of dipolarization (t-t0 = 0).
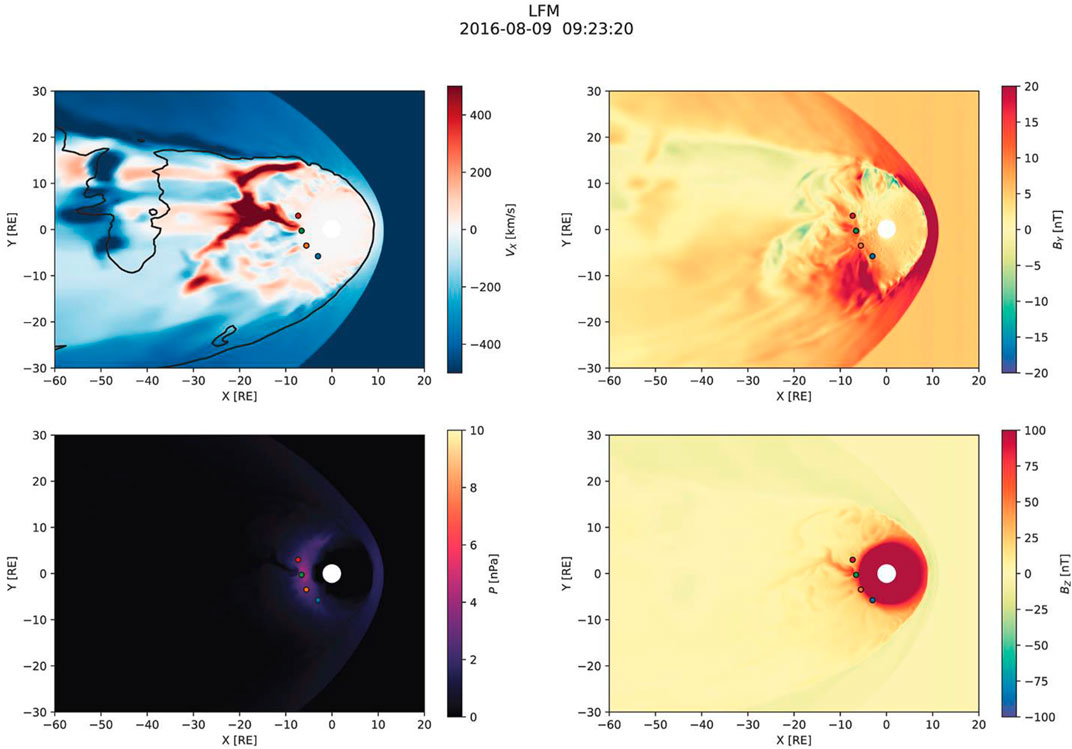
FIGURE 3. From Merkin et al. (2019). Overview of the simulation around the time of substorm onset. The solar magnetic equatorial plane is shown in each panel. Magnetospheric spacecraft are marked with blue (GOES-13), orange (GOES-14), green (GOES-15), and red (MMS-1) circles which indicate the spacecraft positions projected to the plane. The black contour in the upper left panel indicates the Bz = 0 isocontour. Note bottom right shows a mesoscale DFB impinging on the inner magnetosphere as the narrow red finger around X = −10, Y = 0. In the upper left, the red finger at the same location shows earthward plasma flow up to 400 km/s.
Most papers contributing to the debate utilized MHD modeling (Birn et al., 2019; Merkin et al., 2019; McPherron et al., 2020), highlighting the difficulty the field faces to answer the question with data or with multi-scale physics.
While modeling seems to be converging on consensus, observations are needed for validation purposes as model includes all the physics involved. Furthermore, because this field heavily relies on MHD modeling, kinetic-scale physical processes are not modeled. Kinetic-scale physics are expected to contribute to instabilities in the transition region that could destabilize the plasma sheet, accelerate particles, and lead to a global dipolarization. Therefore, models that include kinetic-scale physics are also required (e.g., Shou et al., 2021; Ukhorskiy et al., 2022; see also Sorathia et al., 2022 White Paper submitted to the National Academy of Sciences).
2.2 In terms of current
Electrical currents, running along magnetic field lines connecting the magnetosphere to the ionosphere, are important to MI-coupling. Several papers resulted from community discussions on whether the large-scale substorm current wedge was comprised of “wedgelets” of current, or if it is one coherent current system resulting from a larger-scale phenomenon (e.g., global dipolarization). Results varied: Some papers argued that it is one large phenomenon (e.g., Ohtani and Gjerloev, 2020) (see Figure 4A for schematic from the ground); or that it is one large-scale current due to the pileup of multiple mesoscale structures (e.g., Yang et al., 2011; Birn and Hesse, 2014; Liu et al., 2015a; Birn et al., 2019); or that it is comprised of multiple wedgelets that form simultaneously and continuously (Liu et al., 2015b) (see Figure 4B for schematic from the ground); or that “both the large-scale and multiple-wedgelet picture occur for different events” (Nishimura et al., 2020).
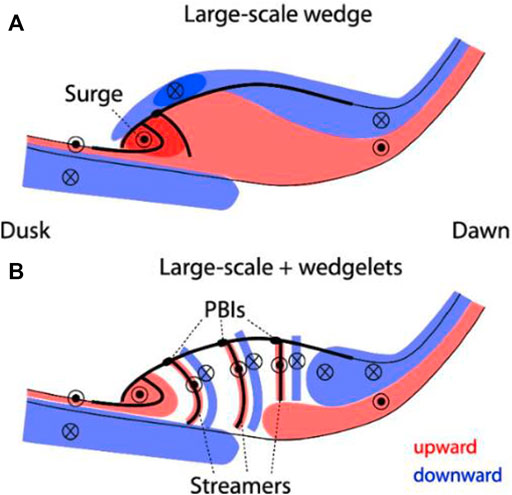
FIGURE 4. From Nishimura et al. (2020) (A) Schematic of large-scale SCW. (B) Schematic of SCW formed by a compilation of wedgelets.
Liu et al. (2015a) state, “If DFBs arrive continually for several tens of minutes, a long-lasting substorm current wedge (SCW) can be sustained by wedgelets. Alternatively, our wedgelet scenario may account for only the first few minutes of a long-lasting SCW … ” The latter scenario was also shown by Birn et al. (2019). Sergeev et al. (2014) demonstrated that even an individual flow burst significantly modifies the pressure and entropy distribution in the inner magnetosphere, which continues well after the flows fade away (30 min–50 min, compared to the flow lifetime of 10 min–15 min). They provided a possible explanation by citing Yao et al. (2012) and Birn and Hesse (2013), who suggested the modified plasma pressure and entropy during the flow braking provides the main contribution to the large-scale field-aligned currents of the SCW via the
Ohtani and Gjerloev (2020), on the other hand, used ground magnetometer data to conclude that: although the SCW may evolve from a wedgelet formed at the onset of substorms, wedgelets are probably not a primary constituent of the SCW for most of the subsequent expansion phase.
Nishimura et al. (2020) used THEMIS ground-based all-sky-imagers (ASIs) and magnetometers to claim that both Figures 4A, B scenarios do occur, with Figure 4B occurring 65% of the time. Could it be that two substorm “modes” exist, and that both sides of this substorm debate are not only valid, but true? If that is the case, what leads to the two different responses? Although great progress was made, more questions have arisen.
2.3 In terms of particle transport and injections
Historically, particle injections were observed at geosynchronous orbit (GEO) and were spatially constrained to be a few hours of MLT wide (e.g., Reeves et al., 1990). As more satellites were launched with apogees in the plasma sheet, particle energization and transport related to BBFs and DFBs were increasingly studied. Particle energization was observed in decades past, but whether mesoscale particle “injections” were in fact “injections” (depositing electrons onto trapped drift orbits) became a hot topic, along with whether mesoscale injections contributed to the wider (global) GEO injections or if the two were distinctly different. Once again, models are heavily used to approach the answer (e.g., Birn and Hesse, 1994; 2004; 2013; Ukhorskiy et al., 2017; 2018; Wang et al., 2018; Eshetu et al., 2019) including some data-informed models (e.g., Li et al., 1998; Gabrielse et al., 2012; 2016; 2017a). MHD and analytical models utilizing particle tracing codes in defined electromagnetic fields have shown that coherent dipolarization fronts/DFBs can, in fact, carry energetic electrons (e.g., Gabrielse et al., 2017a; Eshetu et al., 2019) and ions (e.g., Ukhorskiy et al., 2018) earthward by trapping them in gradient-B drifts around the peak in Bz (sometimes referred to as “magnetic islands”) (see Figure 5). A similar gradient-B trapping mechanism is shown to occur due to localized magnetic dips driven by ion injections and the resulting diamagnetic motion of the injected ions (e.g., He et al., 2017; Xiong et al., 2017), causing dispersionless flux enhancements at multiple energies away from the injection source (Yin et al., 2021). Because particles with lower pitch angles may not be trapped, the dips may serve as pitch angle filters responsible for the anisotropic ion distributions and the excitation of electromagnetic ion cyclotron (EMIC) waves in the inner magnetosphere (Yin et al., 2022). These studies highlight the importance of including mesoscale magnetic field variations in building our understanding of particle dynamics and transport between the plasma sheet and inner magnetosphere.
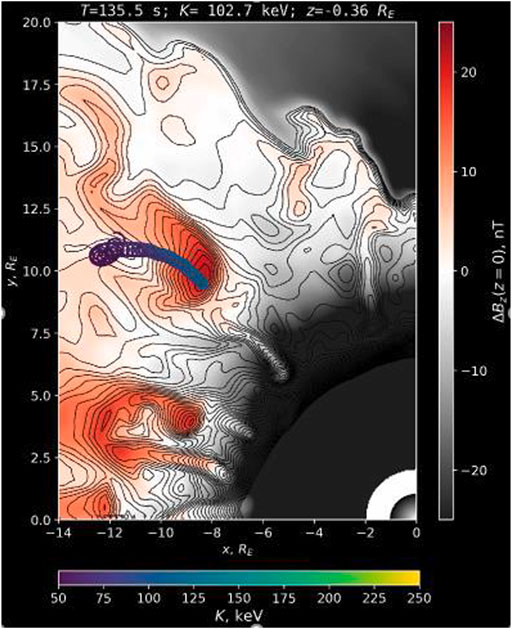
FIGURE 5. From Ukhorskiy et al. (2018). Ion trapped magnetically drifting about the local peak in Bz from the mesoscale, earthwardtraveling DFB.
Existing observations leave much unanswered, however. How much mesoscale injections contribute to the radiation belts and ring current particle populations is still up for debate. Previous works (e.g., Sergeev et al., 2000; Ohtani et al., 2006; Takada et al., 2006; Dubyagin et al., 2011; Sergeev et al., 2012) showed that the majority of bursty bulk flows observed in the plasma sheet brake in the transition region and do not penetrate to the inner magnetosphere. Takada et al. (2006) showed that 30% seen at > 15 RE were also seen inside 10 RE. Ohtani et al. (2006) could not find most flow bursts seen at 10 RE at GEO, and only saw 4 dipolarizations at GEO out of 106 flow bursts seen at Geotail (where Geotail was anywhere from ∼10–30 RE). Combined results between Dubyagin et al. (2011), who showed 80% of flow bursts seen at 11 RE were also seen at 9 RE, and Sergeev et al. (2012), who found only 36%–38% of flow bursts seen farther out by Geotail or THEMIS were accompanied by a flow burst seen at GEO, shows that most flow bursts stop between 6.6–9 RE. The two papers also showed that the penetration depth of the flow burst/injection was relative to its entropy, PVγ, agreeing with theoretical work by Pontius and Wolf (1990) suggesting low entropy flux tubes can penetrate more deeply into the inner magnetosphere.
More recently, Runov et al. (2021) attempted to correlate dipolarizations observed by THEMIS at 10–12 RE in the near-midnight magnetotail and energetic ion injections observed by the Los Alamos National Laboratory (LANL) satellites at 6.6 RE (GEO) during storms’ main phase. They found only 21 out of 39 dipolarizations at THEMIS were associated with dipolarizations at GEO, and only 10 out of those 21 dipolarizations at GEO had an associated ion injection. This indicates that dipolarizations are necessary but not sufficient for ion injection at (cis) GEO—where “cis”-GEO means inside, or earthward of, GEO. Taken together with results from Liu et al. (2016) who studied cis-GEO injections with Van Allen Probes, the important parameter in injection observation is, unsurprisingly, the electric field. An electric field is required to energize the particles, and we note that an electric field is present when a fast plasma flow moves in Earth’s magnetic field.
Gkioulidou et al. (2014) used Van Allen Probes data to suggest that mesoscale injections contribute ∼30% to the ring current. But more satellites in coordinated orbits would better help constrain their input. Using ground-based riometers to observe injection-related precipitation along with satellites in the tail, Spanswick et al. (2010) demonstrated that the global particle injection initiated near the Earth’s magnetic field transition region. It then propagated earthward towards GEO as well as tailward. To put these observations in context with the growing story of earthward-traveling mesoscale injections, Gabrielse et al. (2019a) studied injections using multiple satellites, riometers, and ground-based ASIs. Their conclusion was that mesoscale electron injections are distinct from global electron injections at GEO, forming differently as predecessors and likely contributors to the global electron injection. Whereas the global electron injection was clearly related to the expanding global dipolarization, the ion injection was only observed at the satellite while the fast flow and its electric field existed at the satellite (giving it a shorter lifetime). Turner et al. (2017) also used a multi-satellite study to constrain the injection’s azimuthal scale size. They found multiple injections occurred during the event studied and constrained the size of some injections to a few RE wide; however, other injections were as wide as 12 h in MLT (For more discussion on mesoscale injections and their contribution to the inner magnetosphere, see the white paper submitted by Claudepierre et al., 2022, to the National Academy of Sciences.)
It is clear that even with multiple, uncoordinated satellite observation points, constraining the injection’s scale size and propagation direction is elusive. If instead a coordinated fleet with a dense coverage spanning the transition region into the inner magnetosphere was launched to constrain the appropriate spatial and temporal scale sizes, significant progress could be made.
In a creative use of available datasets, Adewuyi et al. (2021) used TWINS Energetic Neutral Atom (ENA) imaging and THEMIS ASIs to map the ion temperature to the plasma sheet to infer locations and sizes of the fast flows and particle heating in the magnetotail (see Figure 6). However, limited spatial and temporal resolution (2 min) makes it difficult to pin down the dynamical evolution of that heating. Imagers with higher temporal-spatial resolution, especially in coordination with the above-mentioned fleet of spacecraft, would be pivotal in addressing these open questions. Furthermore, TWINS has been decommissioned so these techniques are unavailable to study future events. Along those lines, the THEMIS white-light ASIs that have been used in a plethora of studies only have a few operational years left. The Canadian sponsored TREx mosaic of color ASIs will hopefully fill-in and improve the view from the ground, providing a 2D context with enhanced particle energy information for in situ observations, but they need our loud support to continue to obtain funding and to increase coverage. Losing the mosaic of ASIs across North America would be devastating to the field, and leveraging international partnerships is a strong path forward.
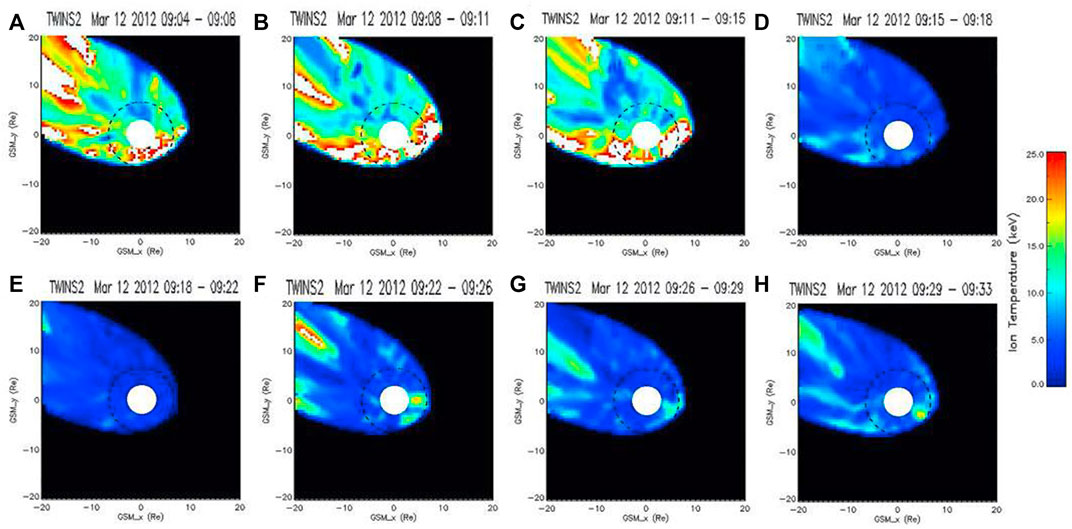
FIGURE 6. From Adewuyi et al. (2021). (A–H): TWINS ENA imaging used to plot ion temperature maps (color) in 2D downtail over time. White circle is Earth, x-axis is X-GSM, y-axis is Y-GSM. Magnetotail flow channels appear before the sudden stormcommencement (SSC), indicating the importance of mesoscale injections to the inner magnetosphere at SSC. ASI data (shown in Adewuyi et al. (2021)) was also mapped to the tail, showing a similar trend in the aurora.
2.4 In terms of energy transport and precipitation
How much of the energy transport throughout the system occurs via mesoscale phenomena as opposed to global activity? This is a broad topic that needs more attention than this short paper can provide. For example, previous studies (Codrescu et al., 1995; Matsuo et al., 2003; Deng et al., 2013) showed that mesoscale electric fields in the ionosphere (often resulting from mesoscale magnetotail flows mapping to the ionosphere) can play an equally important role to the Joule heating as the average large-scale electric field. Another component of energy transfer is via precipitation of particles from the magnetosphere that deposit their energy in the upper atmosphere. One paper from the GEM discussion concluded that mesoscale precipitation contributes ∼15% of the total energy dissipation into the ionosphere (Ohtani, 2019). Another paper showed mesoscale aurora contributes ∼60%–80% of the total precipitated energy flux during substorm expansion, then 35%–40% during the rest of the substorm (Gabrielse et al., 2021).
These mesoscale precipitation “messengers” or “conduits” of energy have meaningful impacts on the ionosphere and thermosphere, and are just starting to be included in General Circulation Models (GCMs). GCMs rely on global trends in the aurora (e.g., OVATION Prime-2013, Newell et al., 2014) to characterize ionosphere forcing from above. They do not capture the dynamic, intense precipitation from mesoscale discrete aurora, nor the fast ionospheric mesoscale plasma flows that are driven by mesoscale magnetosphere flows (e.g., Gallardo-Lacourt et al., 2014; Gabrielse et al., 2018; 2019b). Recent attempts have been made to include these features. For example, both Deng et al. (2019; Figure 7) and Sheng et al. (2021) superposed mesoscale plasma flows in the Global Ionosphere/Thermosphere Model (GITM) to analyze how they affect neutral winds and neutral density perturbations. They found intense neutral density perturbations that lasted at least 50 min after the flow stopped, and that if two flows occurred simultaneously that the neutral density perturbation had even finer structure.
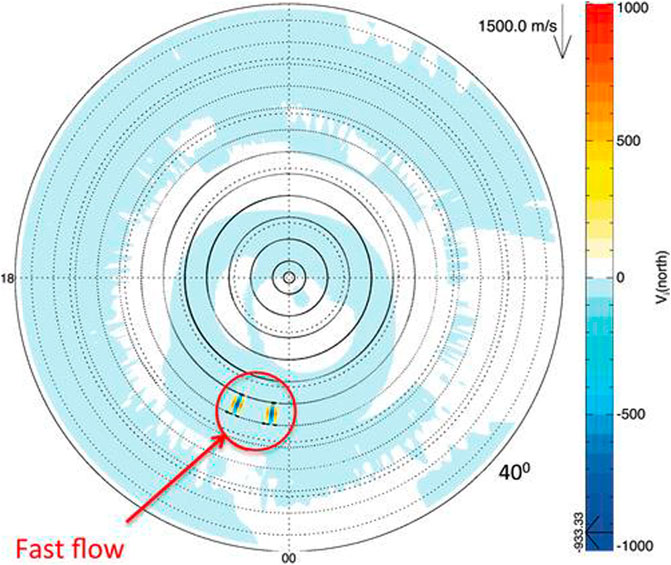
FIGURE 7. From Deng et al. (2019). Two flow bursts were added to GITM in the auroral zone and are separated by 15° in longitude or 1 h in Local Time. There is a high probability for multiple flow bursts to happen simultaneously in the auroral zone (Sergeev et al., 2004). Color is the difference in the ion drift in the north direction between the simulations with and without flow burst.
These dynamic, often discrete, auroral features are not included in our models, which rely on statistical maps of the (often) diffuse aurora. The diffuse aurora is also easier to model given that the physics of their formation (e.g., wave-particle interactions in the magnetosphere) are better understood. To address the knowledge gap, recent works provided 2D precipitation/conductance maps from ASIs (Lam et al., 2019; Nishimura et al., 2021; Gabrielse et al., 2021; Figure 8), but much is left to do. See Laundal et al. (2017) along with the white paper submitted to the National Academy of Science’s Decadal Survey by Ozturk et al. (2022) and/or Ozturk et al. (2021) for more details on the importance of correctly characterizing and modeling precipitation and conductance. Characterizing conductance correctly is important not just for ionosphere physics, but for magnetospheric physics as well. Feedback to the magnetotail can affect where reconnection occurs, for example (see Lotko et al., 2014; El-Alaoui et al., 2023).
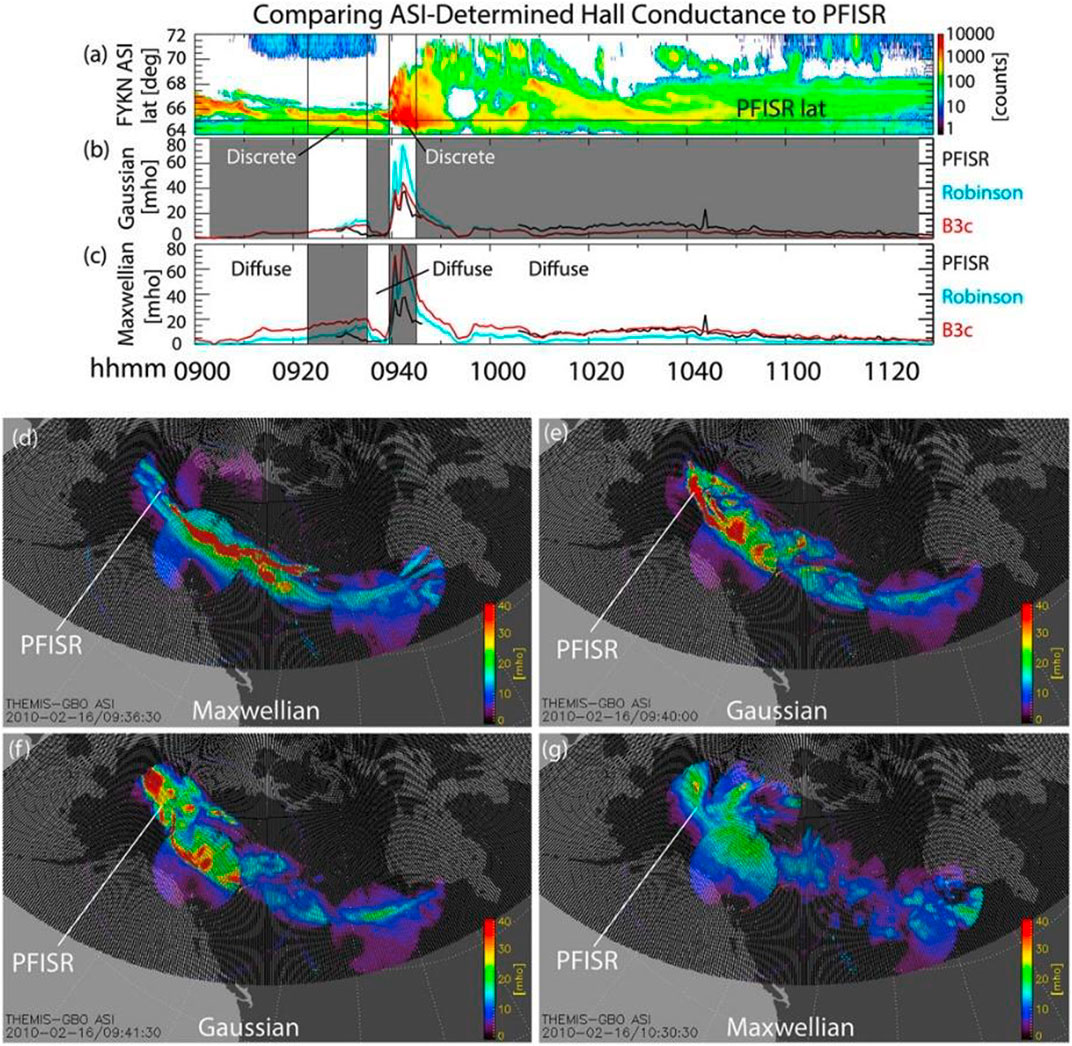
FIGURE 8. From Gabrielse et al. (2021). (A) Keogram of white light counts at the Fort Yukon ASI at the Poker Flat Incoherent Scatter Radar (PFISR) longitude. PFISR latitude is marked with horizontal line. Comparing PFISR conductance (σ) (black) with ASI inputs to the Boltzmann Three Constituent (B3C) auroral transport code (Strickland et al., 1976; 1993) (red) and Robinson formulae (blue) for discrete aurora with an electron population described by a Gaussian distribution (B) and diffuse aurora described by a Maxwellian distribution (C). The B3C version has a good match with PFISR. (D–G) are 2D plots of the Hall conductance determined by ASI inputs to B3C.
It is therefore crucial to understand and characterize these mesoscale phenomena in the magnetotail in order to improve not just our understanding of the magnetosphere, but of the coupled magnetosphere-ionosphere-thermosphere (MIT) system. This aligns very well with the objectives of NASA’s Geospace Dynamics Constellation (GDC) mission, which will measure the geomagnetic energy inputs into ionosphere on a broad range of scales.
3 What are we missing to understand the multi-scale interaction in the nightside magnetosphere-ionosphere system?
Section 2 was a non-inclusive summary of the current debates, progress, and open questions within the community on the topic of mesoscale phenomena in the magnetosphere-ionosphere and the overarching question of how they impact the global system. What is clear is the importance in better probing Earth’s magnetotail transition region to understand how energy (that initially came from the solar wind) is ultimately dissipated. As mesoscale flows brake and deposit magnetic flux and energy there, how does that energy move past the transition region and into the inner magnetosphere? How is the ring current powered? How is the energy dissipated and/or transferred into the ionosphere? As the transition region builds pressure from flux pileup, that pressure drives back the incoming flows and shields the inner magnetosphere from the solar wind electric field. Learning how the energy makes it past the transition region despite this shielding is key. Is it via mesoscale, bursty phenomena? Is it via a global response predicated by mesoscale messengers? etc.
With models reaching the point of mesoscale inclusion, the time is ripe to better study and understand how information is transported across space and scales via these mesoscale messengers, especially in the Earth’s magnetotail transition region where much energy conversion and transport takes place. The following summarizes what science we are missing to understand the multi-scale interaction in the nightside MI system along with suggestions for the tools that could help address it.
Understand 3D structure of plasma, fields, and energy conversion in the transition region
• Coordinated, 3D observations of the transition region that span several hours of MLT in width, several RE in length (6–12 RE), and a few RE north and south of the current sheet. We need the ability to measure field aligned currents (FACs), magnetic flux, particle flux and energies, and plasma moments.
• High temporal (tens of seconds,
• Global far ultraviolet (FUV) and multi-spectral imagers observing the ionosphere with adequate temporal and spatial resolution (seconds, ∼tens of km, for 90 consecutive minutes (substorm lifetime) or more).
• A suite of imager-bearing satellites with orbital planes flying over the auroral oval.
• Mosaic of ground-based color imagers, which would allow us to measure energy flux, mean energy, and conductance, as well as observe auroral features.
• Substantially enhanced coverage and resolution of 2-D flows in the ionosphere, such as is now becoming feasible with the recent new capabilities of the SuperDARN radars.
Determine multi-scale energy deposition and redistribution in the magnetosphereionosphere-thermosphere system
• 2D low-altitude measurements of particle flux, electric and magnetic fields along with auroral imaging to infer the distribution of energy deposition into the ionosphere in the form of precipitation and Poynting flux. Include measurement of neutrals.
• Array of multi-spectral photometers and/or imagers biased to the magnetic zenith to infer energy flux and energy in the auroral zone.
• A satellite constellation taking measurements across multiple local times, like NASA’s Geospace Dynamics Constellation (GDC).
Understand 3D structure of the plasma sheet, transport, and mapping to the ionosphere
• Datasets capable of observing mesoscale phenomena formation to understand how they are created. Could include a fleet of satellites distributed azimuthally and radially throughout the plasma sheet. Could also include ENA imagers which have shown 2D snapshots of the energized ions throughout the plasma sheet.
• Ability to couple auroral features with plasma sheet dynamics, e.g., pre-breakup auroral beads. One idea includes a spaceborne electron accelerator that provides precise mapping between in situ and ground-based measurements, as suggested by Borovsky et al. (2020).
Understand the effects in the inner magnetosphere: for example, what is the mesoscale contribution to the ring current, radiation belts, particle energization, and their participation in how particles gain access, etc.? More on this topic is discussed in the white paper by Claudepierre et al. (2022) submitted to the National Academy of Sciences.
• This may require a fleet of satellites to observe the inner magnetosphere’s evolution in both time and space across multiple magnetic local times and radial distances.
• ENA imaging may also help address this question by providing a 2D picture of the energized ions.
Understand non-MHD physics: e.g., ion kinetics, thin current sheets, electron physics representative of precipitation and arc-generation, and reconnection onset.
• Global models must be improved to include adequate temporal and spatial resolutions that are appropriately coupled between different geospace regions and include the critical non-ideal MHD physics within the plasma sheet. (See Sorathia et al., 2022 White Paper submitted to the National Academy of Sciences.).
• Basic physics in thin auroral arc formation must be included in global models.
The suggested tools could be part of one gargantuan effort, or they could be used as a series of focused investigations addressing parts of the problem sequentially, each coordinated with its predecessors, and building up to a constellation gradually.
4 Summary
In summary, mesoscale carriers or “messengers” of information such as those described in Table 1 have been increasingly studied in the past 5–10 years as their significance to the global system is clear, and modeling capabilities at required resolutions are improving. Groups in the GEM and CEDAR communities (like the MURI group) have been putting their efforts into understanding the role of mesoscale messengers due to the importance of correctly characterizing how energy, particles, and magnetic flux are transported, converted, and/or stored in the magnetotail transition region and in the coupled ionosphere. More questions have arisen, however, that require coordinated, dedicated missions to answer, along with improved models that capture higher spatial and temporal resolutions across various scale sizes.
Author contributions
All authors contributed to the writing of this review. They were all important participants in the GEM and/or MURI discussions summarized. CG wrote the first draft and ingested the comments and contributions by the other authors to make a final draft for submission. She also led the GEM Dipolarization Focus Group discussions that resulted in several papers summarized in this review. MG, SM, DM, and DT co-led the GEM Dipolarization Focus Group and discussions. YD was the PI of the AFOSR MURI program that contributed to this review. MC had discussions with CG during the paper drafting about mesoscales in the ionosphere.
Acknowledgments
We are grateful for the National Science Foundation’s Geospace Environment Modeling (GEM) program, which provided the forum for most of the discussions and works presented in this paper via the Dipolarization and their Effects in the Inner Magnetosphere Focus Group. We are also grateful to the AFOSR MURI program which funded a lot of the discussion on mesoscales in the ionosphere. We are similarly thankful to NSF’s Coupling, Energetics, and Dynamics of Atmospheric Regions (CEDAR) program for providing a forum for discussion as well. We note that a shorter version of this paper was submitted to the National Academies Decadal Survey call for White Papers, and will thus be included in the Bulletin of the AAS. The following grant numbers are gratefully acknowledged for funding the work summarized in this paper throughout the years, as well as the time it took to write this review: CG: AFOSR Grant FA9559-16-1-0364, NASA grants 80NSSC20K1790, NNH19ZDA001N-HGIO, NNH19ZDA001N-HGIO, and NSF grant AGS 1938599. JL: NASA grants 80NSSC22K0749, 80NSSC22K0751, 80NSSC20K1316, 80NSSC20K0714. MH: NASA grant 80NSSC17K0678. MC: NSF AGS 2225405. KS: NASA 80NSSC19K0241, 80NSSC20K1833, and the DRIVE Science Center for Geospace Storms under grant 80NSSC20K0601. YD: NASA grants 80NSSC20K1786, 80NSSC22K0061, 80NSSC20K0195 and 80GSFC22CA011, and AFOSR award FA9559-16-1-0364. SO: NASA grant 80NSSC19K0272. AK: NASA Awards 80NSSC19K0755, 80NSSC19K1195, and 80NSSC20K0701 and NSF Award AGS-2109543. RM: NSF 1602588. We gratefully acknowledge the valuable contributions from Dr. Adam Michael in organizing and running the GEM Focus Group on Dipolarizations.
Conflict of interest
The authors declare that the research was conducted in the absence of any commercial or financial relationships that could be construed as a potential conflict of interest.
Publisher’s note
All claims expressed in this article are solely those of the authors and do not necessarily represent those of their affiliated organizations, or those of the publisher, the editors and the reviewers. Any product that may be evaluated in this article, or claim that may be made by its manufacturer, is not guaranteed or endorsed by the publisher.
References
Adewuyi, M., Amy, M., Nishimura, T., Gabrielse, C., and Katus, R. M. (2021). Mesoscale features in the global geospace response to the March 12, 2012 storm. Front. Astron. Space Sci. 8. doi:10.3389/fspas.2021.746459
Angelopoulos, V., Kennel, C. F., Coroniti, F. V., Pellat, R., Kivelson, M. G., Walker, R. J., et al. (1994). Statistical characteristics of bursty bulk flow events. J. Geophys. Res. 99 (A11), 21257. doi:10.1029/94JA01263
Angelopoulos, V., Phan, T. D., Larson, D. E., Mozer, F. S., Lin, R. P., Tsuruda, K., et al. (1997). Magnetotail flow bursts: Association to global magnetospheric circulation, relationship to ionospheric activity and direct evidence for localization. Geophys. Res. Lett. 24, 2271–2274. doi:10.1029/97GL02355
Baumjohann, W., Hesse, M., Kokubun, S., Mukai, T., Nagai, T., and Petrukovich, A. A. (1999). Substorm dipolarization and recovery. J. Geophys. Res. 104 (A11), 24995–25000. doi:10.1029/1999JA900282
Birn, J., Hesse, M., Nakamura, R., and Zaharia, S. (2013). Particle acceleration in dipolarization events. J. Geophys. Res. 118, 1960–1971. doi:10.1002/jgra.50132
Birn, J., and Hesse, M. (1994). Particle acceleration in the dynamic magnetotail: Orbits in self-consistent three-dimensional MHD fields. J. Geophys. Res. 99, 109. doi:10.1029/93JA02284
Birn, J., and Hesse, M. (2013). The substorm current wedge in MHD simulations. J. Geophys. Res. Space Phys. 118, 3364–3376. doi:10.1002/jgra.50187
Birn, J., and Hesse, M. (2014). The substorm current wedge: Further insights from MHD simulations. J. Geophys. Res. Space Phys. 119, 3503–3513. doi:10.1002/2014JA019863
Birn, J., Liu, J., Runov, A., Kepko, L., and Angelopoulos, V. (2019). On the contribution of dipolarizing flux bundles to the substorm current wedge and to flux and energy transport. J. Geophys. Res. Space Phys. 124, 5408–5420. doi:10.1029/2019JA026658
Birn, J., Thomsen, M. F., and Hesse, M. (2004). Electron acceleration in the dynamic magnetotail: Test particle orbits in three-dimensional magnetohydrodynamic simulation fields. Phys. Plasmas 11 (5), 1825–1833. doi:10.1063/1.1704641
Borovsky, J. E., Delzanno, G. L., Dors, E. E., Thomsen, M. F., Sanchez, E. R., Henderson, M. G., et al. (2020), Solving the auroral-arc-generator question by using an electron beam to unambiguously connect critical magnetospheric measurements to auroral images, J. Atmos. Solar-Terrestrial Phys., 206, 105310–106826. doi:10.1016/j.jastp.2020.105310
Claudepierre, S., Chen, L., Delzanno, G. L., Gkioulidou, M., Goldstein, J., Ilie, R., et al. (2022). Scientific priorities for the Earth’s coupled inner magnetosphere: A system-of-systems perspective. Available at: https://baas.aas.org/.
Codrescu, M. V., Fuller-Rowell, T. J., and Foster, J. C. (1995). On the importance of E-field variability for Joule heating in the high-latitude thermosphere. Geophys. Res. Lett. 22, 2393–2396. doi:10.1029/95GL01909
Cohen, I. J., Mauk, B. H., Turner, D. L., Fennell, J. F., Blake, J. B., Reeves, G. D., et al. (2019). Drift-dispersed flux dropouts of energetic electrons observed in Earth's middle magnetosphere by the Magnetospheric Multiscale (MMS) mission. Geophys. Res. Lett. 46, 3069–3078. doi:10.1029/2019GL082008
Deng, Y., Fuller-Rowell, T. J., Ridley, A. J., Knipp, D., and Lopez, R. E. (2013). Theoretical study: Influence of different energy sources on the cusp neutral density enhancement. J. Geophys. Res. Space Phys. 118, 2340–2349. doi:10.1002/jgra.50197
Deng, Y., Heelis, R., Lyons, L., Nishimura, T., and Zhang, S. (2019). Impact of flow bursts in the auroral zone on the ionosphere and thermosphere. J. Geophys. Res. Space Phys. 124, 10459–10467. doi:10.1029/2019JA026755
Deng, Y., Maute, A., Richmond, A. D., and Roble, R. G. (2009). Impact of electric field variability on Joule heating and thermospheric temperature and density. Geophys. Res. Lett. 36, L08105. doi:10.1029/2008GL036916
Dubyagin, S., Sergeev, V., Apatenkov, S., Angelopoulos, V., Runov, A., Nakamura, R., et al. (2011). Can flow bursts penetrate into the inner magnetosphere? Geophys. Res. Lett. 38, L08102. doi:10.1029/2011GL047016
El-Alaoui, M., Walker, R. J., and McPherron, R. L. (2023). Effects of the ionospheric conductance on the dynamics of the magnetotail. J. Geophys. Res. Space Phys. 128, e2022JA030259. doi:10.1029/2022JA030259
Eshetu, W. W., Lyon, J. G., Hudson, M. K., and Wiltberger, M. J. (2019). Simulations of electron energization and injection by BBFs using high-resolution LFM MHD fields. J. Geophys. Res. Space Phys. 124, 1222–1238. doi:10.1029/2018JA025789
Fu, H., Grigorenko, E. E., Gabrielse, C., Liu, C., Lu, S., Hwang, K.-J., et al. (2020). Magnetotail dipolarization fronts and particle acceleration: A review. Sci. China Earth Sci. 63, 235–256. doi:10.1007/s11430-019-9551-y
Gabrielse, C., Angelopoulos, V., Harris, C., Artemyev, A., Kepko, L., and Runov, A. (2017a). Extensive electron transport and energization via multiple, localized dipolarizing flux bundlesflux bundles. J. Geophys. Res. Space Phys. 122, 5059–5076. doi:10.1002/2017JA023981
Gabrielse, C., Angelopoulos, V., Runov, A., and Turner, D. L. (2014). Statistical characteristics of particle injections throughout the equatorial magnetotail. J. Geophys. Res. Space Phys. 119, 2512–2535. doi:10.1002/2013JA019638
Gabrielse, C., Angelopoulos, V., Runov, A., and Turner, D. L. (2012). The effects of transient, localized electric fields on equatorial electron acceleration and transport toward the inner magnetosphere. J. Geophys. Res. 117, A10213. doi:10.1029/2012JA017873
Gabrielse, C., Harris, C., Angelopoulos, V., Artemyev, A., and Runov, A. (2016). The role of localized inductive electric fields in electron injections around dipolarizing flux bundlesfields in electron injections around dipolarizing flux bundles. J. Geophys. Res. Space Phys. 121, 9560–9585. doi:10.1002/2016JA023061
Gabrielse, C., Nishimura, T., Chen, M., Hecht, J. H., Kaeppler, S. R., Gillies, D. M., et al. (2021). Estimating precipitating energy flux, average energy, and Hall auroral conductance from THEMIS all-sky-imagers with focus on mesoscales. Front. Phys. 9. doi:10.3389/fphy.2021.744298
Gabrielse, C., Nishimura, Y., Lyons, L., Gallardo-Lacourt, B., Deng, Y., and Donovan, E. (2018). Statistical properties of mesoscale plasma flows in the nightside high-latitude ionosphere. J. Geophys. Res. Space Phys. 123, 6798–6820. doi:10.1029/2018JA025440
Gabrielse, C., Pinto, V., Nishimura, Y., Lyons, L., Gallardo-Lacourt, B., and Deng, Y. (2019b). Storm time mesoscale plasma flows in the nightside high-latitude ionosphere: A statistical survey of characteristics. Geophys. Res. Lett. 46, 4079–4088. doi:10.1029/2018GL081539
Gabrielse, C., Spanswick, E., Artemyev, A., Nishimura, Y., Runov, A., Lyons, L., et al. (2019a). Utilizing the Heliophysics/Geospace System Observatory to understand particle injections: Their scale sizes and propagation directions. J. Geophys. Res. Space Phys. 124, 5584–5609. doi:10.1029/2018JA025588
Gabrielse, C., Angelopoulos, V., Runov, A., Spanswick, E., and Turner, D. L. (2017b), "Pre-midnight preponderance of both ion and electron injections." Dawn¬-Dusk asymmetries in planetary plasma environments. Ed. H. Stein Washington, D.C.: American Geophysical Union and John Wiley & Sons, Inc, October. Print.
Gallardo-Lacourt, B., Nishimura, Y., Lyons, L. R., Zou, S., Angelopoulos, V., Donovan, E., et al. (2014). Coordinated SuperDARN THEMIS ASI observations of meso-scale flow bursts associated with auroral streamers. J. Geophys. Res. Space Phys. 119, 142–150. doi:10.1002/2013JA019245
Gkioulidou, M., Ukhorskiy, A. Y., Mitchell, D. G., Sotirelis, T., Mauk, B. H., and Lanzerotti, L. J. (2014). The role of small-scale ion injections in the buildup of Earth’s ring current pressure: Van Allen Probes observations of the 17 March 2013storm. J. Geophys. Res. Space Phys. 119, 7327–7342. doi:10.1002/2014JA020096
He, Z., Chen, L., Zhu, H., Xia, Z., Reeves, G. D., Xiong, Y., et al. (2017). Multiple-satellite observation of magnetic dip event during the substorm on 10 October 2013. Geophys. Res. Lett. 44, 9167–9175. doi:10.1002/2017GL074869
Kepko, L., McPherron, R. L., Amm, O., Apatenkov, S., Baumjohann, W., Birn, J., et al. (2015). Substorm current wedge revisited. Space Sci. Rev. 190, 1–46. doi:10.1007/s11214-014-0124-9
Lam, M. M., Freeman, M. P., Jackman, C. M., Rae, I. J., Kalmoni, N. M. E., Sandhu, J. K., et al. (2019). How well can we estimate pedersen conductance from the THEMIS white-light all-sky cameras. J. Geophys Res. Space Phys. 124, 2920–2934. doi:10.1029/2018JA026067
Laundal, K. M., Cnossen, I., Milan, S. E., Haaland, S. E., Coxon, J., Pedatella, N. M., et al. (2017). North–South asymmetries in Earth’s magnetic field. Space Sci. Rev. 206, 225–257. doi:10.1007/s11214-016-0273-0
Li, X., Baker, D. N., Temerin, M., Reeves, G., and Belian, R. (1998). Simulation of dispersionless injections and drift echoes of energetic electrons associated with substorms. Geophys. Res. Lett. 25 (20), 3763–3766. doi:10.1029/1998GL900001
Liu, J., Angelopoulos, V., Chu, X., Zhou, X.-Z., and Yue, C. (2015a). Substorm current wedge composition by wedgelets. Geophys. Res. Lett. 42, 1669–1676. doi:10.1002/2015GL063289
Liu, J., Angelopoulos, V., Zhang, X. J., Turner, D. L., Gabrielse, C., Runov, A., et al. (2016). Dipolarizing flux bundles in the cis-geosynchronous magnetosphere: Relationship between electric fields and energetic particle injections. J. Geophys. Res. 121, 1362–1376. doi:10.1002/2015JA021691
Liu, J., Angelopoulos, V., Zhou, X.-Z., and Runov, A. (2014). Magnetic flux transport by dipolarizing flux bundles. J. Geophys. Res. Space Phys. 119, 909–926. doi:10.1002/2013JA019395
Liu, J., Angelopoulos, V., Zhou, X.-Z., Yao, Z.-H., and Runov, A. (2015b). Cross-tail expansion of dipolarizing flux bundles. J. Geophys. Res. Space Phys. 120, 2516–2530. doi:10.1002/2015JA020997
Liu, Z.-Y., Zong, Q.-G., Zou, H., Wang, Y. F., and Wang, B. (2019). Drifting electron holes occurring during geomagnetically quiet times: BD-IES observations. J. Geophys. Res. Space Phys. 124, 8695–8706. doi:10.1029/2019JA027194
Lotko, W., Smith, R. H., Zhang, B., Ouellette, J. E., Brambles, O. J., and Lyon, J. G. (2014). Ionospheric control of magnetotail reconnection. Science 345, 184–187. doi:10.1126/science.1252907
Lui, A. T. Y. (2015). Dipolarization fronts and magnetic flux transport. Geosci. Lett. 2, 15. doi:10.1186/s40562-015-0032-1
Matsuo, T., Richmond, A. D., and Hensel, K. (2003). High-latitude ionospheric electric field variability and electric potential derived from DE-2 plasma drift measurements: Dependence on IMF and dipole tilt. J. Geophys. Res. (Space Phys. 108, 1005. doi:10.1029/2002JA009429
McPherron, R. L., El-Alaoui, M., Walker, R. J., and Richard, R. (2020). Characteristics of reconnection sites and fast flow channels in an MHD simulation. J. Geophys. Res. Space Phys. 125, e2019JA027701. doi:10.1029/2019JA027701
McPherron, R. L., Russell, C. T., and Aubry, M. P. (1973). Satellite studies of magnetospheric substorms on August 15, 1968. 9. Phenomenological model for substorms. J. Geophys. Res. 78, 3131–3149. doi:10.1029/JA078i016p03131
McPherron, R. L. (1972). Substorm related changes in the geomagnetic tail: The growth phase. Planet. Space Sci. 20 (9), 1521–1539. ISSN 0032-0633. doi:10.1016/0032-0633(72)90054-2
Merkin, V. G., Panov, E. V., Sorathia, K., and Ukhorskiy, A. Y. (2019). Contribution of bursty bulk flows to the global dipolarization of the magnetotail during an isolated substorm. J. Geophys. Res. Space Phys. 124, 8647–8668. doi:10.1029/2019JA026872
Nakamura, R., Baumjohann, W., Klecker, B., Bogdanova, Y., Balogh, A., Rème, H., et al. (2004). Motion of the dipolarization front during a flow burst event observed by cluster: Dipolarization and flow bursts. Geophys. Res. Lett. 31, 3-1–3-4. doi:10.1029/2002GL015763
Newell, P. T., Liou, K., Zhang, Y., Sotirelis, T., Paxton, L. J., and Mitchell, E. J. (2014). OVATION Prime-2013: Extension of auroral precipitation model to higher disturbance levels. Space weather. 12, 368–379. doi:10.1002/2014SW001056
Nishimura, Y., Deng, Y., Lyons, L. R., McGranaghan, R. M., and Zettergren, M. D. (2021). “Multiscale dynamics in the high-latitude ionosphere,” in Ionosphere dynamics and applications. Editors C. Huang, G. Lu, Y. Zhang, and L. J. Paxton (United States: Wiley), 49–65. doi:10.1002/9781119815617.ch3
Nishimura, Y., Lyons, L. R., Gabrielse, C., Weygand, J. M., Donovan, E. F., and Angelopoulos, V. (2020). Relative contributions of large-scale and wedgelet currents in the substorm current wedge. Earth, Planets Space 72, 106. doi:10.1186/s40623-020-01234-x
Ohtani, S., and Gjerloev, J. W. (2020). Is the substorm current wedge an ensemble of wedgelets?: Revisit to midlatitude positive bays. J. Geophys. Res. Space Phys. 125, e2020JA027902. doi:10.1029/2020JA027902
Ohtani, S., Singer, H. J., and Mukai, T. (2006). Effects of the fast plasma sheet flow on the geosynchronous magnetic configuration: Geotail and GOES coordinated study. J. Geophys. Res. 111, A01204. doi:10.1029/2005JA011383
Ohtani, S. (2019). Substorm energy transport from the magnetotail to the nightside ionosphere. J. Geophys. Res. Space Phys. 124, 8669–8684. doi:10.1029/2019JA026964
Ozturk, D. S. (2021). A collaborative approach to understanding auroral region magnetosphere-ionosphere thermosphere coupling through ionospheric conductivity. Heliophysics 2050 White Pap. 2021, 4067.
Ozturk, D. S., Lin, D., Gabrielse, C., Chen, M., Grandin, M., and Sivadas, N. (2022). The prominence of magnetospheric precipitation for the high-latitude multi-scale coupling of magnetosphere-ionosphere-thermosphere systems. Available at: https://baas.aas.org/.
Pontius, D. H., and Wolf, R. A. (1990). Transient flux tubes in the terrestrial magnetosphere. Geophys. Res. Lett. 17 (1), 49–52. doi:10.1029/GL017i001p00049
Reeves, G. D., Fritz, T. A., Cayton, T. E., and Belian, R. D. (1990). Multi-satellite measurements of the substorm injection region. Geophys. Res. Lett. 17 (11), 2015–2018. doi:10.1029/GL017i011p02015
Runov, A., Angelopoulos, V., Henderson, M. G., Gabrielse, C., and Artemyev, A. (2021). Magnetotail dipolarizations and ion flux variations during the main phase of magnetic storms. J. Geophys. Res. Space Phys. 126, e2020JA028470. doi:10.1029/2020JA028470
Runov, A., Angelopoulos, V., Sitnov, M. I., Sergeev, V. A., Bonnell, J., McFadden, J. P., et al. (2009). THEMIS observations of an earthward-propagating dipolarization front. Geophys. Res. Lett. 36, L14106. doi:10.1029/2009GL038980
Runov, A., Angelopoulos, V., Zhou, X.-Z., Zhang, X.-J., Li, S., Plaschke, F., et al. (2011). A THEMIS multi-case study of dipolarization fronts in the magnetotail plasma sheet. J. Geophys. Res. 116, A05216. doi:10.1029/2010JA016316
Sergeev, V. A., Angelopoulos, V., Gosling, J. T., Cattell, C. A., and Russell, C. T. (1996). Detection of localized, plasma-depleted flux tubes or bubbles in the midtail plasma sheet. J. Geophys. Res. 101, 10817–10826. doi:10.1029/96JA00460
Sergeev, V. A., Bösinger, T., Belian, R. D., Reeves, G. D., and Cayton, T. E. (1992). Drifting holes in the energetic electron flux at geosynchronous orbit following substorm onset. J. Geophys. Res. 97 (A5), 6541–6548. doi:10.1029/92JA00182
Sergeev, V. A., Chernyaev, I. A., Angelopoulos, V., Runov, A. V., and Nakamura, R. (2014). Stopping flow bursts and their role in the generation of the substorm current wedge. Geophys. Res. Lett. 41, 1106–1112. doi:10.1002/2014GL059309
Sergeev, V. A., Chernyaev, I. A., Dubyagin, S. V., Miyashita, Y., Angelopoulos, V., Boakes, P. D., et al. (2012). Energetic particle injections to geostationary orbit: Relationship to flow bursts and magnetospheric state. J. Geophys. Res. 117, A10207. doi:10.1029/2012JA017773
Sergeev, V. A., Liou, K., Newell, P. T., Ohtani, S.-I., Hairston, M. R., and Rich, F. (2004). Auroral streamers: Characteristics of associated precipitation, convection and field-aligned currents. Ann. Geophys. 22, 537–548. doi:10.5194/angeo-22-537-2004
Sheng, C., Deng, Y., Gabrielse, C., Lyons, L. R., Nishimura, Y., Heelis, R. A., et al. (2021). Sensitivity of upper atmosphere to different characteristics of flow bursts in the auroral zone. J. Geophys. Res. Space Phys. 126, e2021JA029253. doi:10.1029/2021JA029253
Sorathia, K., Bard, C., Delzanno, G. L., Dorelli, J., Gabrielse, C., and Lin, Y. (2022). First-principles geospace modeling in the next decade. Available at: https://baas.aas.org/.
Spanswick, E., Reeves, G. D., Donovan, E., and Friedel, R. H. W. (2010). Injection region propagation outside of geosynchronous orbit. J. Geophys. Res. 115, A11214. doi:10.1029/2009JA015066
Strickland, D. J., Book, D. L., Coffey, T. P., and Fedder, J. A. (1976). Transport equation techniques for the deposition of auroral electrons. J. Geophys Res. 81 (16), 2755–2764. doi:10.1029/JA081i016p02755
Strickland, D. J., Daniell, R. E., Jasperse, J. R., and Basu, B. (1993). Transport-theoretic model for the electron-proton-hydrogen Atom aurora, 2. Model results. J. Geophys Res. 98 (A12), 21533–21548. doi:10.1029/93JA01645
Takada, T., Nakamura, R., Baumjohann, W., Asano, Y., Volwerk, M., Zhang, T. L., et al. (2006). Do BBFs contribute to inner magnetosphere dipolarizations: Concurrent Cluster and Double Star observations. Geophys. Res. Lett. 33, L21109. doi:10.1029/2006GL027440
Turner, D. L., Fennell, J. F., Blake, J. B., Claudepierre, S. G., Clemmons, J. H., Jaynes, A. N., et al. (2017). Multipoint observations of energetic particle injections and substorm activity during a conjunction between Magnetospheric Multiscale (MMS) and Van Allen Probes. J. Geophys. Res. Space Phys. 122, 504. doi:10.1002/2017JA024554
Ukhorskiy, A. Y., Sitnov, M. I., Merkin, V. G., Gkioulidou, M., and Mitchell, D. G. (2017). Ion acceleration at dipolarization fronts in the inner magnetosphere. J. Geophys. Res. Space Phys. 122, 3040–3054. doi:10.1002/2016JA023304
Ukhorskiy, A. Y., Sorathia, K. A., Merkin, V. G., Crabtree, C., Fletcher, A. C., Malaspina, D. M., et al. (2022). Cross-scale energy cascade powered by magnetospheric convection. Sci. Rep. 12, 4446. doi:10.1038/s41598-022-08038-x
Ukhorskiy, A. Y., Sorathia, K. A., Merkin, V. G., Sitnov, M. I., Mitchell, D. G., and Gkioulidou, M. (2018). Ion trapping and acceleration at dipolarization fronts: High-resolution MHD and test-particle simulations. J. Geophys. Res. Space Phys. 123, 5580–5589. doi:10.1029/2018JA025370
Shoui, V., Tenishev, V., Chen, Y., Toth, G., and Ganushkina, N. 2021. Magnetohydrodynamic with adaptively embedded particle-in-cell model: MHD-AEPIC. J. Comput. Phys. 446, 110656. doi:10.1016/j.jcp.2021.110656
Sergeev, V., Sauvaud, J. A., Popescu, D., Kovrazhkin, R. A., Liou, K., Newell, P. T., et al. (2000), Multiple-spacecraft observation of a narrow transient plasma jet in the Earth's plasma sheet, Geophys. Res. Lett., 27, 851–854. doi:10.1029/1999GL010729
Wang, C.-P., Gkioulidou, M., Lyons, L. R., and Wolf, R. A. (2018). Spatial distribution of plasma sheet entropy reduction caused by a plasma bubble: Rice Convection Model simulations. J. Geophys. Res. Space Phys. 123, 3380–3397. doi:10.1029/2018JA025347
Wolf, R. A., Kumar, V., Toffoletto, F. R., Erickson, G. M., Savoie, A. M., Chen, C. X., et al. (2006). Estimating local plasma sheet PV5/3 from single-spacecraft measurements. J. Geophys. Res. 111, A12218. doi:10.1029/2006JA012010
Wolf, R. A., Toffoletto, F. R., Spiro, R. W., Hesse, M., and Birn, J. (2002). “Magnetospheric substorms: An inner-magnetospheric modeling perspective,” in Space weather study using multi-point techniques. Editor L.-H. Lyu (Amsterdam: Pergamon), 221–229.
Wolf, R. A., Wan, Y., Xing, X., Zhang, J.-C., and Sazykin, S. (2009). Entropy and plasma sheet transport. J. Geophys. Res. 114, A00D05. doi:10.1029/2009JA014044
Xiong, Y., Chen, L., Xie, L., Fu, S., Xia, Z., and Pu, Z. (2017). Relativistic electron’s butterfly pitch angle distribution modulated by localized back-ground magnetic field perturbation driven by hot ring current ions. Geophys. Res. Lett. 44, 4393–4400. doi:10.1002/2017GL072558
Yang, J., Toffoletto, F. R., Wolf, R. A., and Sazykin, S. (2011). RCM-E simulation of ion acceleration during an idealized plasma sheet bubble injection. J. Geophys. Res. 116, A05207. doi:10.1029/2010JA016346
Yao, Z. H., Pu, Z. Y., Fu, S. Y., Angelopoulos, V., Kubyshkina, M., Xing, X., et al. (2012). Mechanism of substorm current wedge formation: THEMIS observations. Geophys. Res. Lett. 39, L13102. doi:10.1029/2012GL052055
Yin, Z.-F., Zhou, X.-Z., Hu, Z.-J., Yue, C., Zong, Q.-G., Hao, Y.-X., et al. (2022). Localized excitation of electromagnetic ion cyclotron waves from anisotropic protons filtered by magnetic dips. J. Geophys. Res. Space Phys. 127, e2022JA030531. doi:10.1029/2022JA030531
Keywords: transition region, mesoscales, magnetotail, magnetosphere-ionosphere coupling, dipolarization, particle injections, magnetohydrodynamics-MHD, substorm current wedge
Citation: Gabrielse C, Gkioulidou M, Merkin S, Malaspina D, Turner DL, Chen MW, Ohtani S-i, Nishimura Y, Liu J, Birn J, Deng Y, Runov A, McPherron RL, Keesee A, Yin Lui AT, Sheng C, Hudson M, Gallardo-Lacourt B, Angelopoulos V, Lyons L, Wang C-P, Spanswick EL, Donovan E, Kaeppler SR, Sorathia K, Kepko L and Zou S (2023) Mesoscale phenomena and their contribution to the global response: a focus on the magnetotail transition region and magnetosphere-ionosphere coupling. Front. Astron. Space Sci. 10:1151339. doi: 10.3389/fspas.2023.1151339
Received: 26 January 2023; Accepted: 14 April 2023;
Published: 27 April 2023.
Edited by:
Jay R. Johnson, Andrews University, United StatesReviewed by:
Victor Sergeev, Saint Petersburg State University, RussiaMaria Alexeevna Shukhtina, Saint-Petrsburg State University, Russia
Xu-Zhi Zhou, Peking University, China
Gaeta Zimbardo, University of Calabria, Italy
Copyright © 2023 Gabrielse, Gkioulidou, Merkin, Malaspina, Turner, Chen, Ohtani, Nishimura, Liu, Birn, Deng, Runov, McPherron, Keesee, Yin Lui, Sheng, Hudson, Gallardo-Lacourt, Angelopoulos, Lyons, Wang, Spanswick, Donovan, Kaeppler, Sorathia, Kepko and Zou. This is an open-access article distributed under the terms of the Creative Commons Attribution License (CC BY). The use, distribution or reproduction in other forums is permitted, provided the original author(s) and the copyright owner(s) are credited and that the original publication in this journal is cited, in accordance with accepted academic practice. No use, distribution or reproduction is permitted which does not comply with these terms.
*Correspondence: Christine Gabrielse, Y2hyaXN0aW5lLmdhYnJpZWxzZUBhZXJvLm9yZw==