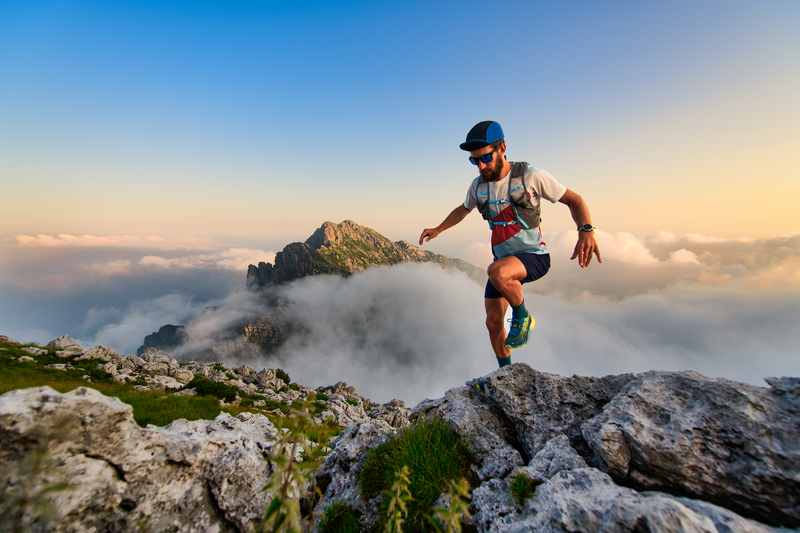
95% of researchers rate our articles as excellent or good
Learn more about the work of our research integrity team to safeguard the quality of each article we publish.
Find out more
REVIEW article
Front. Astron. Space Sci. , 15 June 2023
Sec. Space Physics
Volume 10 - 2023 | https://doi.org/10.3389/fspas.2023.1143909
This article is part of the Research Topic Reviews in Astronomy and Space Sciences View all 27 articles
Most stars generate winds and move through the interstellar medium that surrounds them. This movement creates a cocoon formed by the deflection of these winds that envelops and protects the stars. We call these “cocoons” astrospheres. The Sun has its own cocoon, the heliosphere. The heliosphere is an immense shield that protects the Solar System from harsh, galactic radiation. The radiation that enters the heliosphere affects life on Earth as well as human space exploration. Galactic cosmic rays are the dominant source of radiation and principal hazard affecting space missions within our Solar System. Current global heliosphere models do not successfully predict the radiation environment at all locations or under different solar conditions. To understand the heliosphere’s shielding properties, we need to understand its structure and large-scale dynamics. A fortunate confluence of missions has provided the scientific community with a treasury of heliospheric data. However, fundamental features remain unknown. The vision of the Solar wind with Hydrogen Ion charge Exchange and Large-Scale Dynamics (SHIELD) DRIVE Science Center is to understand the nature and structure of the heliosphere. Through four integrated research thrusts leading to the global model, SHIELD will: 1) determine the global nature of the heliosphere; 2) determine how pickup ions evolve from “cradle to grave” and affect heliospheric processes; 3) establish how the heliosphere interacts with and influences the Local Interstellar Medium (LISM); and 4) establish how cosmic rays are filtered by and transported through the heliosphere. The key deliverable is a comprehensive, self-consistent, global model of the heliosphere that explains data from all relevant in situ and remote observations and predicts the radiation environment. SHIELD will develop a “digital twin” of the heliosphere capable of: (a) predicting how changing solar and LISM conditions affect life on Earth, (b) understanding the radiation environment to support long-duration space travel, and (c) contributing toward finding life elsewhere in the Galaxy. SHIELD also will train the next-generation of heliophysicists, a diverse community fluent in team science and skilled working in highly transdisciplinary collaborative environments.
The vision of the Solar wind with Hydrogen Ion charge Exchange and Large-Scale Dynamics (SHIELD) DRIVE Science Center is to understand the nature and structure of the heliosphere. To realize our vision and create a new model for the heliosphere, SHIELD will enlist experts on observations, kinetic physics and MHD physics, and high energy particle transport and acceleration physics, and will facilitate their interaction in a Center environment. SHIELD will reach to the broader community for participation. Through four integrated research thrusts leading to the global model, SHIELD will: 1) determine the global nature of the heliosphere; 2) determine how pickup ions evolve from “cradle to grave” and affect heliospheric processes; 3) establish how the heliosphere interacts with and influences the LISM; and 4) establish how cosmic rays are filtered by and transported through the heliosphere. (Instead of LISM VLISM has been also coined to describe the region beyond the HP where interstellar material and fields are modified by the Sun).
The key deliverable is a comprehensive, self-consistent, global model of the heliosphere that explains data from all relevant in situ and remote observations and predicts the radiation environment. SHIELD will develop a “digital twin” of the heliosphere capable of: (a) predicting how changing solar and LISM conditions affect life on Earth, (b) understanding the radiation environment to support long-duration space travel, and (c) contributing toward finding life elsewhere in the Galaxy. SHIELD also will train the next-generation of heliophysicists, a diverse community fluent in team science and skilled working in highly transdisciplinary collaborative environments.
SHIELD is one of three DRIVE centers chosen for Phase II in 2022 after a two-year competition between 9 DRIVE centers selected for Phase I. The DRIVE centers came from the recommendation of the Decadal Survey 2013–2023 Solar and Space Physics “A Science for a Technological Society”, NRC 2012. As part of the (DRIVE—Diversify, Realize, Integrate, Venture, Educate) a critical element was the funding of Heliophysics DRIVE Science Centers.
Heliophysics Phase II DRIVE (Diversify, Realize, Integrate, Venture, Educate) Science Centers (DSCs) are intended to support science that cannot be effectively done by individual investigators or small teams, but requires the synergistic, coordinated efforts of a research center. The DRIVE Centers “must address grand challenge science goals that are both ambitious and focused enough to be achievable within the lifetime of the center [5 years-(2022–2027)]–in other words, science problems poised and ready for major advances.”
Stars are not inanimate luminous structures in the night sky. Most stars generate winds and move through the interstellar medium that surrounds them. This movement creates a cocoon formed by the deflection of these winds that envelops and protects the stars. We call these “cocoons” astrospheres.
The Sun has its own cocoon, the heliosphere: The heliosphere is an immense shield that protects the Solar System from harsh, galactic radiation. When Voyager 1 (V1) crossed the heliopause (HP), it discovered that the heliosphere shields 70% of galactic cosmic rays (GCRs) from Earth (Figure 1). The radiation that enters the heliosphere affects life on Earth as well as human space exploration. GCRs are the dominant source of radiation and the principal hazard (Elgart, 2021) affecting long-term space missions within our Solar System. Current global heliosphere models do not successfully predict the radiation environment at all locations or under different solar conditions. To understand the heliosphere’s shielding properties, we need to understand its structure and large-scale dynamics.
FIGURE 1. The heliosphere shields 70% of the harsh galactic radiation. Global models are far from being able to predict the radiation environment in all directions and conditions. SHIELD will provide for the community improved radiation prediction capabilities. The blue curve shows the integral counting rate of galactic cosmic rays from Voyager 1 (for more information see Cummings et al., 2016). The gray shading indicates the region between the solar termination shock and the heliopause.
The heliosphere is formed by: 1) the solar wind; 2) the ionized local interstellar medium (LISM) that surrounds and confines the heliosphere; 3) LISM neutrals that enter the heliosphere, become ionized and join the solar wind as hot pick-up ions (PUIs); and 4) GCRs that stream from the Galaxy and penetrate the heliosphere. A fortunate confluence of missions has provided the scientific community with a treasury of heliospheric data. The in situ measurements by the Voyager and New Horizons (NH) spacecraft, combined with the all-sky Energetic Neutral Atoms (ENA) images of the heliospheric boundary region by the Interstellar Boundary Explorer (IBEX) and Cassini missions, have revealed the size of the heliosphere in the nose region (V1 and V2), the key role PUIs play in the solar wind and heliosheath (V2, NH), and the existence of the IBEX ribbon and Cassini belt.
However, fundamental features remain unknown. Knowledge gaps include the basic shape of the heliosphere (Figure 2), the extent of its tail, the flow pattern and the width of the heliosheath, and the extent of the heliosphere’s influence on the LISM. Current global models fail to explain major observations (Box 1), a key driver of the SHIELD Drive Science Center.
BOX 1 Critical Observations to be Explained by SHIELD
• The acceleration mechanism and location for anomalous cosmic rays (ACRs) are not known. The Voyager spacecraft did not find evidence for acceleration at the termination shock (TS) but detected an increase in ACR intensities as they moved across the heliosheath (HS). SHIELD will determine where and how ACRs are accelerated.
• The HS is 30%–50% thinner than current models predict. SHIELD will determine physical mechanisms responsible for extracting energy from the HS.
• The flow patterns (Figure 3) determined by plasma and energetic particles instruments are drastically different at V1 and V2. SHIELD will reconcile the flow observations from different instruments with those derived from models.
• The magnetic field direction did not change at the heliopause (HP) crossings, a major surprise, and the LISM field direction has changed little since the HP. SHIELD will determine the extent of the influence of the heliosphere on the LISM.
• The significant increase in GCRs just prior to the HP at V1 and V2 and the unexpected GCR anisotropies observed in the LISM are not understood. SHIELD will determine the porosity of the HP and the shielding of GCRs by the HP in all directions. SHIELD also will determine the source of GCR anisotropies in the LISM.
• Proposed heliosphere shapes range from comet-like to “spherical” to “croissant-like” with a truncated heliotail. SHIELD will determine the physical drivers for the shape of the heliosphere and the length and structure of the heliotail.
• Models predict ENA intensities only half as large as those observed by IBEX. SHIELD will determine the source and distribution of ions in the heliosphere to explain IBEX data and prepare for ENA maps that will be produced by IMAP.
• IBEX detected a global feature, the ribbon, organized by the interstellar magnetic field (BISM). Cassini measured a similar, broader “belt” feature. Models proposed to explain these features rely on assumptions such as the draping of the BISM and the level of turbulence in the LISM. SHIELD will determine the source of the turbulence in the LISM and the direction of the interstellar magnetic field, which will help establish the origin of the ribbon and belt.
FIGURE 2. Fundamental features of the heliosphere are not well understood (Opher et al., 2020). SHIELD will determine the main physical drivers contributing to its shape.
FIGURE 3. The drastically different HS flows at V1 and V2 are a source of current debate. SHIELD will address why the flow speeds differ each other and from model predictions (Richardson et al., 2021).
Global models (e.g., Izmodenov & Alexashov, 2015; Opher et al., 2015; Pogorelov et al., 2015) have grown in sophistication and accelerated our understanding of the heliosphere. But they fail to explain many observations. Models do not integrate the influences of suprathermal particles, reconnection, turbulence phenomena, and other microprocesses. There are models of interplanetary shocks, of the termination shock, of cosmic ray (CR) transport, of turbulence, and of the heliosheath and heliosphere. However, no global model includes all the necessary physics. Microprocesses have spatial scales vastly separate from the global scales but are critical pieces of a heliospheric model and must be parameterized. The transport of CRs and the evolution of PUIs require kinetic transport models that are coupled self-consistently to a global MHD model. The next quantum leap in understanding the heliosphere will be realized by synthesizing knowledge in theory, modeling, and observations to create a comprehensive, self-consistent global model that connects all the physical processes that effect the heliosphere as a one system.
The vision of the Solar wind with Hydrogen Ion charge Exchange and Large-Scale Dynamics (SHIELD) DRIVE Science Center (DSC) is to understand the nature and structure of the heliosphere. SHIELD is built around four coupled research thrusts (RTs), each addressing a major science question required to create a novel, global heliosphere model. They are: 1) What is the global structure of the heliosphere?; 2) How do pickup ions evolve from cradle to grave, and how do they affect heliospheric processes?; 3) How does the heliosphere interact with and influence the interstellar medium?; and 4) How are cosmic rays filtered by and transported through the heliosphere? The key deliverable is a comprehensive, self-consistent, global model of the heliosphere that explains data from all relevant in situ and remote observations and predicts the radiation environment. SHIELD’s broadening impact goal is to attract, train and mentor the next-generation of team-based heliophysicists while accelerating knowledge integration, transfer, and communication across traditional boundaries.
SHIELD’s model will include all components of the heliosphere—thermal and suprathermal components of the solar wind, PUIs, ACRs, GCRs, and interstellar neutrals (Figure 4). In addition, the model will define, quantify, and simulate all relevant processes that affect these components, from microphysical processes such as reconnection and turbulence to PUI and cosmic ray transport and acceleration, with physics guided by theory and models that are constrained by observations. SHIELD will develop a “digital twin” of the heliosphere capable of: (a) predicting how changing solar and LISM conditions affect life on Earth, (b) understanding the radiation environment to support long-duration space travel, and (c) contributing toward finding life elsewhere in the Galaxy.
SHIELD research will inform the shape, size, and structure of the heliosphere, closing long-standing knowledge gaps. In addition to predicting how changing solar and LISM conditions affect the heliospheric radiation environment in support of long-duration space travel, SHIELD’s impact is expected to be felt in neighboring scientific communities. The heliosphere is a template for other astrospheres and is the only astrosphere where we can study in situ the processes that determine its structure and radiation environment. Knowledge gained from SHIELD can be extended to other astrospheres and to other times in the Solar System’s history. For example, when life started on Earth, the solar wind had different characteristics and the Sun was embedded in a different interstellar cloud. SHIELD will help predict the radiation environment at exoplanets in other astrospheres and throughout our Solar System’s history. Through the Institute for Habitable Astrospheres (IHA, https://shielddrivecenter.com/iha/), our sustainability initiative, SHIELD will collaborate cross-disciplinarily with astronomers, biologists, and chemists to determine questions and challenges concerning the origin of life that can be solved by combining expertise in radiation, biology, astrobiology, chemistry, and heliophysics.
There is a time urgency: IBEX and Cassini provide ENA data, and, in 2025, NASA will launch Interstellar Mapping and Acceleration Probe (IMAP) (McComas et al., 2018) to make high-resolution ENA measurements. Since ENA observations are line-of-sight images, a global model like SHIELD is required to pin down the sources of the ENAs and interpret the data. The synergy of a global and comprehensive model such as SHIELD with ENA data will transform our view of the heliosphere. Voyager has made the only in situ observations of the TS, HS, HP, and the LISM thus far. Observers from Voyager, several of whom participate in SHIELD, will benchmark our global model and pass their knowledge of the data to SHIELD’s young investigators, allowing Voyager’s legacy to continue.
SHIELD’s work responds directly to the Solar and Space Physics Decadal Survey’s (Solar and Space Physics, NRC, 2013) Science Goal 3, determine the interaction of the Sun with the Solar System and the interstellar medium. We investigate the fundamental processes of turbulence, reconnection, and wave-particle interactions that occur both within the heliosphere and throughout the Universe (Decadal Survey Science Goal 4). The coupling of microprocesses and global codes will explain key observations (Box 1) and thus transform the field of heliophysics. Figure 5 shows that the goals and research thrusts (RTs) of SHIELD are tightly interwoven, justifying the need for a Center. (See Section 5, Justification for DSC Mode of Support.)
FIGURE 5. Each science topic leads to Center-wide outcomes (red boxes) and toward the building of SHIELD’s comprehensive, self-consistent global model of the heliosphere. Years 1–5 corresponds to 5 years of Phase II of SHIELD.
SHIELD has gathered more than 45 experts with diverse viewpoints on current heliospheric puzzles (See Table 1 in Section 7.3). Our team includes experts specializing in all key physical processes that play a role in the distant solar wind, HS and LISM, including turbulence, reconnection, wave-particle interactions, PUI and GCR acceleration and transport, global MHD codes, ENA modeling, and localized kinetic models. To validate the SHIELD model, the team includes experts on in situ and remote observations from all the relevant instruments on Voyager, IBEX, Cassini, New Horizons, and IMAP. An established NASA science educator with expertise in literary, public outreach, communication, and evaluation leads the Broader Impacts activities. Knowledge integration is assured via SHIELD’s management approach, emphasizing shared decision making, team science, collaboration, robust communication as well as leadership and accountability of all team members (Section 8, Management.)
Solving SHIELD’s science questions requires an integrated approach. An example of how our experts in theory, modeling, and data analysis worked together in Phase I (when 30 original DSC were downsized in a period of 2 years to the final 3 awarded for a full DSC) is demonstrative of our synergy. The PUI team (RT2) used multiple models to derive how PUI spectra evolve at the TS. We first modeled the effect of turbulence on the supersonic solar wind (Nakanotani et al., 2021) (Nakanotani; throughout the text we added the names of people from the center that will be involved in each task-see Table 1) constrained by NH observations (Elliott). This solar wind solution was input into kinetic models [hybrid (Giacalone), PIC (Drake, Swisdak), and multi-ion (Zieger)] to find how reconnection and turbulence modify the ion spectra downstream of the TS. We validated these model results with Voyager observations (Richardson, Hill). A consensus PUI spectra was derived and used to model ENAs at IBEX (Shrestha et al., 2021) and Cassini energies (Gkioulidou et al., 2022) (Kornbleuth, Baliukin) using global MHD models (Opher). These model results were compared with ENA observations (Dialynas, Dayeh, Fuselier, Gkioulidou). This work, which shows the integration of the team, quantified the additional PUI acceleration that must occur to match ENA data and sets the stage for our Phase II work (see Section 2.2.2, RT2).
SHIELD made substantial progress on its science questions in Phase I, including several publications mentioned below. Table 2 profiles the key research accomplishments for each thrust and implications for Phase II.
SHIELD will unravel the main physical processes that drive the heliosphere, assembling them into a novel, global model of the heliosphere. It will inform how the heliosphere shields Earth from galactic cosmic rays and support future work on the habitability of other worlds. To fulfill the promise of this vision, we face a set of knowledge integration challenges: 1) modeling huge expanses of space while including small scale processes, 2) modeling a system that is time dependent on large and small-time scales, and 3) validating the models. (See Section 7.3, Risk Mitigation.) To this end, SHIELD is organized around four complementary and integrated research thrusts: 1) the Global Structure of the Heliosphere; 2) Evolution of PUIs; 3) the Influence of the Heliosphere on the LISM; and 4) Cosmic Ray Transport/Filtration (Figure 6). Each thrust is led by a Director (senior investigator) and Deputy Director (junior investigator), and integrates the expertise areas necessary for the research: observations, theory, numerical simulations, data analysis, localized kinetic physics, and MHD models.
FIGURE 6. SHIELD unites and leverages the expertise of more than 45 world-class researchers from 18 partner institutions to understand our heliospheric shield.
RT1 has as director Justyna Sokol and as Deputy Director Marc Kornbleuth.
State of Knowledge: The standard picture of a comet-like heliosphere (Baranov & Malama, 1993) has been the dominant paradigm since the 1960s (Davis, 1955; Parker, 1961; Axford et al., 1963; Dessler, 1967; Baranov & Malama, 1993). Recent modeling and observations challenge this paradigm (Drake et al., 2015; Opher et al., 2015) and suggest that the solar magnetic field tension organizes the HS solar wind into two jet-like structures at the poles. The BU model (Opher et al., 2020) (Figure 7: top middle panel) suggests that the heliosphere has a croissant-like shape with a truncated tail. Other models find an elongated tail [Figure 7: top left (Izmodenov & Alexashov, 2015) and top right (Pogorelov et al., 2015)]. Cassini (Dialynas et al., 2017) (Figure 7: bottom left) and IBEX ENA (McComas et al., 2013) (Figure 7: bottom right) data give contradictory HS shapes. Knowledge Gap: The size and shape of the heliosphere are not known. Current models have problems. First, most assume Maxwellian plasma distributions, but suprathermal particles contain most of the pressure in the outer Solar System. Second, the effects of CRs are generally not included (Scherer and Ferreira, 2005), and third, microprocesses are excluded. PUIs and CRs must be included to correctly model heliosphere energetics and derive plasma distributions in the HS, a critical step for making modeled ENA maps to compare with data. Approach: In Phase I, we compared the BU and Moscow models and found that in both models the heliotail plasma was confined by the solar magnetic field (Kornbleuth et al., 2021a) (AC2) (Figure 8). Plasma solutions in the nose of the heliosphere are similar, but reconnection in the BU model leads to a shorter heliotail compared to the Moscow model. Higher-energy (> 10 keV) ENA maps differ between these models, since they can see farther down the tail, so ENA data could determine which model is correct. A key advance in Phase I is the realization that a Rayleigh-Taylor-like instability (RT-I) may occur along the solar magnetic axis in the HS. This instability destroys the coherence of the heliospheric jets and generates turbulence which leads to magnetic reconnection, allowing LISM material to enter the heliotail (AC1) (Figure 9). The turbulence driven by the instability is macroscopic and may accelerate particles. In Phase II, we will explore the time evolution of the MHD solution and the mixing of solar and LISM plasma (Opher, Drake, Izmodenov, and Zank). Differences between the BU and Moscow models will be investigated (Kornbleuth and Baliukin), including why the BU model has a short heliotail. Simulations will show how the RT instability (AC1) develops under different solar cycle conditions (Powell). Next, we will explore the effects of solar-driven temporal changes (CMEs, solar cycle changes) on the global HS structure (Onubogu, Sokół). The effects of CRs and PUIs on the RT instability will be studied as well as the development of turbulence, and the consequences of this turbulence on the acceleration of HS PUIs (Ma and Opher) (RT2.3). The acceleration of PUIs and other ions will be modeled by hybrid codes (Giacalone and Du), PIC codes (Drake, Swisdak), and kinetic models (Zhao and Zank), using the MHD-derived RT-I turbulence. Model and observed high-energy ENA maps (> 10 keV), which probe the tail to larger distances, will be compared and we will make predictions for IMAP (Gkioulidou, Dialynas, Fuselier, Sokół, and Dayeh). The heliotail structure and evolution, measured by IBEX (> 0.5 eV) and IBEX polar maps, will be used to validate SHIELD (Fuselier, Dayeh, Sokół, Reisenfeld). All these tasks depend on the global structure of the heliosphere, which depends on the solar wind, PUIs (RT2), CRs (RT4) and LISM conditions (RT3). The PUI and CR acceleration (RT1) will be added to the MHD model. SHIELD will use the complete plasma distribution to create model ENA maps to compare with IBEX, Cassini and, in the future, IMAP data and constrain the shape of the heliosphere.
FIGURE 7. A major controversy: What is the shape of the heliosphere? Solving it is one of the goals of SHIELD.
FIGURE 8. Different (BU and Moscow) models provide different plasma solutions in the tail. Differences need to be understood that will lead to the Center Milestone M1.
FIGURE 9. SHIELD discovered an instability in the heliosheath, SHIELD will investigate its consequences for particle acceleration and structure of heliotail.
The next task will be to solve the discrepancy between observed and modeled HS flows (AC4). V2 HS flows derived from particle data using the CG effect and from direct plasma measurements do not agree. In Phase I, SHIELD used Voyager CRS observations to measure particle anisotropies and found that LECP (keV particles) and CRS (> 0.5 MeV) CG speeds agree with each other, but not with plasma data (AC4). These observations suggest that the V1 HS stagnation region may not be real (Figure 3), which would affect models of ENA production. In Phase I, we assembled an expert team to study these results. We found that if the speed profile at V1 were similar to that found at V2 by PLS, inconsistencies with magnetic flux conservation and the Rankine-Hugoniot conditions at the shock would be reconciled (Richardson et al., 2021) (AC4).
In Phase II, we will determine why the CG calculations give different speeds and if the plasma and particle speeds are decoupled (Richardson, Cummings, and Zank), which would affect particle transport. The high-energy ions may set up HS pressure gradients that invalidate the CG calculations (Roelof, 2021). SHIELD will investigate this possibility using the HD code coupled to CRs and PUIs. We will identify the origin of low-energy ENAs with implications for IBEX and IMAP (Fuselier with Galli) (AC3). Outcome: SHIELD will determine the flow speeds in the HS, the structure of the heliotail, the shape of the heliosphere, and its evolution in time during the solar cycle.
State of Knowledge: The thickness of the HS measured by Voyagers is thinner than models predict by almost a factor of 2. Mechanisms suggested to resolve this problem are solar cycle variations (Izmodenov et al., 2005; 2008), removal of hot HS ions by charge exchange (Malama et al., 2006; Opher et al., 2020), inclusion of thermal conductivity (Izmodenov et al., 2014), and escape of ACRs across the HP (Guo et al., 2010). All produce insufficient HS thinning. Knowledge Gap: The HS is much thinner than predicted. Approach: SHIELD will explore three mechanisms that may thin the HS: 1) charge exchange of neutral H with the full plasma distribution; 2) the inclusion of ACRs and their transport, and 3) the inclusion of thermal conduction. The SHIELD model will calculate accurate plasma distribution functions to test mechanism 1) (Powell, Ma, and Kornbleuth). Mechanism 2) will be tested using models of ACR escape (Guo et al., 2018) with transport parameters from the MHD code coupled to the CR transport code (Florinski and a postdoc). To explore mechanism 3), thermal conduction, already in SWMF, will be activated in OH (van der Holst, Toth) (see B3, Development of the SHIELD Model). These tasks will determine which mechanism, or combination of mechanisms, account for the HS thicknesses observed at V1 and V2. Outcome: SHIELD will determine why the HS is thinner than expected and illuminate the physics needed in the global model.
State of Knowledge: The structure of the HP and the degree of porosity determine the transport rates of energetic particles into and out of the heliosphere. Possible processes at the HP are magnetic reconnection (Swisdak et al., 2013), interchange instabilities (Florinski, 2015), flux transfer events (Schwadron & McComas, 2013), and turbulent reconnection (Grygorczuk et al., 2014). Knowledge Gap: The HP crossings at V1 and V2 are significantly different (e.g., the particle dropouts/enhancements were on the opposite sides of the HP). The variations of the HP structure and properties with time and location are not known. Approach: SHIELD will run localized PIC (Drake, Swisdak), hybrid (Giacalone) and multi-ion simulations (Zieger) at key HP locations using input from MHD simulations (Kornbleuth, Ma, Powell, Opher). Localized MHD simulations (Florinski) and theoretical studies (Florinski, Zank) will explore MHD instabilities at the HP. SHIELD results in the V1 and V2 directions will be compared with observations of the magnetic field at the HP (Szabo) to test model accuracy and validate SHIELD’s global HP results. Solar cycle variation effects on HP properties will be explored. Outcome: SHIELD will determine the HP porosity that affects cosmic ray entry into the HS and LISM CR intensities.
RT2 has director Gary Zank and as Deputy Director Matina Gkioulidou.
State of Knowledge: PUIs dominate the thermal energy of the solar wind from ∼20 AU to the HP (Richardson et al., 2008). New Horizons measures PUIs but Voyager cannot; HS suprathermal particle distributions below 28 keV are unknown. Turbulence plays a major role in heating the solar wind from the corona to the TS. PUIs provides a source of waves and turbulence that, through dissipation, heat the solar wind and mediate the transport of energetic particles (Lee et al., 1987; Williams & Zank, 1994; Zank, 1999; Smith et al., 2006; Smith et al., 2017). The solar wind turbulence transmitted across the TS is dominated by compressible modes. Heliospheric processes that effect the LISM PUIs are the ionization of solar wind and HS neutral H, and transmission of HS compressible turbulence (Burlaga et al., 2015; Zank et al., 2017a) across the HP and its conversion to incompressible modes (Burlaga et al., 2018; Zank et al., 2019; Zhao et al., 2020). Secondary charge exchange (CE) produces hot PUIs (Zank et al., 1996) that form the IBEX ribbon (Heerikhuisen et al., 2010). ENAs produced by CE from these PUIs serve as probes of the LISM and BISM. The CE of heliospheric neutrals can drive instabilities that generate weak interstellar turbulence (Florinski et al., 2010), heating the LISM, and weakly scattering the PUIs. Knowledge Gap: We do not understand how PUIs are accelerated at the TS, how they are transported and heated in the HS and LISM, or the distribution of ENAs produced when the PUIs are lost via charge exchange. The effect of compressible HS turbulence on the PUIs is an outstanding question. The origin of the compressible turbulence in the LISM is not known (Burlaga et al., 2018; 2020; Zank et al., 2019; Zhao et al., 2020). Approach: Localized simulations (hybrid, PIC, and multi-fluid models) and theory (Zank et al., 2017a; 2017b; 2021) will be used to investigate how PUIs are affected by turbulence, reconnection, and non-linear waves. Outcome: The simulations and theory will determine the PUI properties and how best to incorporate the PUIs into the SHIELD model (B3, Development of the SHIELD Model).
NH observations and theoretical models (Zank et al., 1996a; Zank et al., 2010) show that shocks preferentially heat PUIs (Zirnstein et al., 2018). Zank, Adhikari, and Zhao will include turbulence generated by PUIs in localized MHD simulations to study which processes heat the PUIs (AC9). Interplanetary shocks observed by NH will be simulated using PIC simulations (Swisdak, Drake) (Drake et al., 2010) and multi-ion fluid simulations (Zieger, Keebler) (Zieger et al., 2015; 2020). Turbulence parameters will be constrained by Voyager (Fraternale et al., 2019) (Richardson, Szabo) and NH (Keebler et al., 2022) data (Elliott, Hill). Outcome: SHIELD will predict the PUI spectra upstream of the TS for input into global MHD models and production of ENA maps.
SHIELD made progress in Phase I toward understanding the transmission and acceleration of PUIs at the TS. Plasma and magnetic field parameters upstream of the TS in the nose, the flank, and tail-ward directions were determined by a solar-wind/PUI/turbulence model (Nakanotani et al., 2021) (AC9). Hybrid simulations, which included large-scale turbulence upstream of the TS, found that the 10–50 keV PUI intensity was similar at all three TS locations. However, we found significant differences in the distribution of 0.5–1 keV ENAs coming from the tail region of the heliosphere compared to those from the nose and flank directions. PIC simulations that included electron physics and provided better estimates of the reflected (accelerated) PUI population were also performed (Drake, Swisdak). The transmitted > 4 keV ion intensities are comparable to those from the hybrid model and predicted by theory (Zank et al., 2010; Wang et al., 2023) verifying the results. We used the ion spectra produced by the hybrid simulations (Giacalone et al., 2021) as input to model ENA spectra (Gkioulidou et al., 2022). The model ENA intensities were lower than those measured (Figure 10). We will identify additional physical process for the energization of PUIs (Nakanotani, Zank, Zhao, Wang, Du, Guo, Giacalone, Drake, Opher) and compare with ENA and ion data (Dialynas, Gkioulidou, Dayeh, Fuselier, Decker, Kornblueth, Wang). Outcome: SHIELD will predict the PUI spectra in the downstream the TS for input into global MHD models and production of ENA maps.
FIGURE 10. Discrepancy between modeled ENAs and observations needs to be explained (Gkioulidou et al., 2021).
The difference between predicted and observed ENA distributions (Figure 10) could be a signature of PUI acceleration in the HS. Turbulence in the HS is highly compressible, different from the supersonic solar wind. The transport theory for PUIs in compressible turbulence will be developed (Zank, Zhao) using a stochastic differential equation (SDE) code. Hybrid simulations (Giacalone, Du, Guo) will model the evolution and acceleration of HS particles by compressible turbulence. PIC simulations will test if reconnection energizes HS PUIs (Drake et al., 2010; Dahlin et al., 2016; 2017) (Swisdak, Drake). Multi-ion simulations (Zieger) will determine if compressible turbulence is generated by dispersive magnetosonic waves (Zieger et al., 2020). Outcome: SHIELD will predict the PUI spectra in the HS needed for global MHD models and production of ENA maps.
SHIELD will develop a transport model for heliospheric PUIs in the LISM. A theoretical transport equation (Zank, 2014) will be derived that will be solved via a SDE method (Zhao et al., 2019) (Zank, Florinski, Zhao). Complementary hybrid simulations (Giacalone, Florinski) will model PUI creation, wave/turbulence generation, and transport in the LISM to ensure that the theoretical transport formalism is valid. Outcome: SHIELD will predict the PUI spectra in the LISM needed for global MHD models and production of ENA maps.
RT3 have as a director Vladimir Florinski and as Deputy Director Drew Turner.
State of Knowledge: The LISM is strongly influenced by solar transients (Gurnett et al., 2015; 2021) (Figure 11). The magnetic field was predicted to rotate at the HP, but a surprise was that B did not change direction at either the V1 or V2 crossing. BISM remains near the solar direction 33 AU past the HP. Constraining the direction of the pristine BISM is important for models of the IBEX ribbon (McComas et al., 2009) and the Cassini belt. Krimigis et al. (2009) Another V1 discovery was that the plasma density increased by a factor of ∼30 at the HP and then continued to slowly increase for an additional 7 AU (Gurnett et al., 2013; Gurnett and Kurth, 2019), indicative of a plasma depletion layer (PDL) in the LISM (Zwan & Wolf, 1976; Wang & Richardson, 2004; Cairns & Fuselier, 2017). Knowledge Gap: We do not know how far the heliospheric influence extends into the LISM. Central questions include: 1) What controls the draping of BISM at the HP? 2) What are the intensity and direction of BISM and the plasma density in the pristine LISM? 3) How does the density change with distance? What are the properties and geometry of the PDL? Approach: SHIELD will compare BISM from MHD models with observations (Kornbleuth, Baliukin, Izmodenov, Opher, Ma, Powell, Richardson) to see if reconnection (Opher et al., 2017) and/or instabilities (Florisnki, 2015) (Florinski) twist the BISM to the solar direction and how far from the HP this twist occurs (AC10). We will derive PUI energy spectra and combine them with in situ inner/outer heliosheath ion energy spectra to obtain energetic particle spectra on both sides of the HP (Cummings, Decker). Existing theories and orbit/scattering models of keV to GeV charged particles will be used to determine the strength of coupling between particle species exhibiting diffusive behavior and the background plasma to establish the effect on pressure balance (Florinski). Global MHD models will evaluate the properties and geometry of the PDL using enhanced resolution near the HP (Florinski, Toth, Opher). Zank and Florinski will revisit the theory of tangential discontinuities in the presence of energetic particles. The SWMF anisotropic pressure (CGL) model will be used to perform local-scale CGL-MHD simulations within 10 AU of the HP (Florinski, Toth) to determine the PDL pressure anisotropy and relate it to the observed plasma depletion and magnetic field enhancement. Outcome: SHIELD will reconstruct the magnetic field pattern and the distribution of plasma and energetic particle pressures, their anisotropies, and current flows within the entire HP boundary layer. Draping of B and plasma in LISM will inform conditions in LISM.
FIGURE 11. Effect of the heliosphere is seen throughout the LISM (Nikoukar et al., 2022). SHIELD addresses how far this influence extends.
State of Knowledge: Large solar events cross the TS and collide with the HP, injecting compressive waves and structures into the LISM (Burlaga et al., 2013; Gurnett et al., 2015; Washimi et al., 2015; 2017; Burlaga and Ness, 2016; Kim et al., 2017). Interstellar shocks and pressure fronts are typically weak, having a compression ratio of less than 1.5. Knowledge Gap: LISM transients are not resolved by current models (Kim et al., 2017), making it difficult to relate LISM structures to their precursor solar events. The measured width of the shocks or pressure fronts was about 0.1 AU, which is four orders of magnitude larger than the ion inertial length in the LISM (a scale that defines the shock thickness). Mostafavi and Zank (2018) argued for collisional broadening of the shocks using an analytic model; however kinetic models have not reproduced these results. Approach: High resolution MHD simulations, using an adaptively refined mesh, will model the propagation of transients (Zieger, Toth, Mostafavi). More sophisticated and accurate theoretical models such as Braginskii type fluid models are also being utilized to study the nature of shocks in the VLISM/LISM (Ghanbari, Zank). The global heliospheric model provides TS and HP locations (RT1). Shocks will be modeled on kinetic scales using hybrid particle-mesh models, resolving ion dynamics only, with a very long simulation box (Florinski, Giacalone). Outcome: SHIELD will explain the timing and magnetic field signatures of the shock waves and pressure fronts driven by events inside the heliosphere and observed in the LISM by the Voyagers.
State of Knowledge: V1 observed both transverse and longitudinal magnetic fluctuations in the LISM (Burlaga et al., 2015; 2018; Fraternale et al., 2020) with a Kolmogorov power spectrum. Fluctuations were primarily compressive near the HP, but a transverse component appeared 2 years after the HP crossing while the compressive component showed little change. Magnetic fluctuation intensity was a factor of 100 times higher than the spectrum of interstellar fluctuations from radio astronomy observations (Haverkorn et al., 2008). The compressive component may result from refraction of solar wind waves near the HP (Zank et al., 2017b). Knowledge Gap: We do not understand the source of the LISM turbulence. A global model of turbulent transport in the LISM has not been developed and the distribution of turbulent fluctuations throughout the outer heliosheath remains a complete mystery. The transverse fluctuations may result from nonlinear interactions between magnetosonic waves and incompressible 2D structures (Zank et al., 2019), but this hypothesis does not explain why the total power in the magnetic fluctuations increases with distance from the HP. Compressive turbulence could have a very different effect on particle pitch-angle scattering in the LISM than slab and 2D turbulence. A closure on the question of the IBEX ribbon origin (Heerikhuisen et al., 2010; Florinski et al., 2016) and GCR penetration of the HP transition layer is impossible without a detailed knowledge of scattering and cross field diffusion in the LISM. Approach: SHIELD will use measured LISM turbulence spectra (Florinski), particle transport simulations (Florinski) and theory (Zank) to address the nature of the LISM turbulence. We will investigate how the magnetic shear at the HP effects the cosmic rays. We will also develop theoretical underpinnings for energetic charged particle transport in compressive and anisotropic turbulence (Florinski). Direct numerical simulations of the orbits of a very large number of test particles in pre-specified, data-driven magnetic turbulence models will supplement and verify the theory (Giacalone & Jokipii, 1999; Giacalone, 2005). The inclusion of anisotropic magnetic turbulence is especially important for GCR transport in the LISM. We will model the transport of multi-component turbulence in the LISM using an updated turbulence model including both incompressible and weakly compressive fluctuations (Zank, van der Holst). Results will be used to model GCR transport in the outer HS and their filtration by the HP to assess how Earth is protected from GCRs in RT4 (Florinski, Toth, Opher). These simulations will be based on integration of a large number of guiding center averaged trajectories backward in time from the observation point (Florinski et al., 2010) with pitch angle scattering and perpendicular diffusion included as stochastic processes that will feed into the overall SHIELD model (through SPECTRUM). Outcome: SHIELD will establish physically realistic transport coefficients as a function of particle energy appropriate for particle transport in the LISM, which will feed into predicting the radiation environment in heliosphere.
State of Knowledge: Astrospheres are obstacles to the interstellar gas and sources of waves and particles in the LISM. The heliosphere is a source of ENAs produced by charge exchange with interstellar material; the leading IBEX ribbon explanation requires that ENAs fill the space around the HP to a distance of several hundred AU (Möbius et al., 2013). Knowledge Gap: A global picture of the interstellar environment near the heliosphere is far from complete. The turbulent layer that exists in front of the HP could extend to the bow wave, whose location is unknown. Do turbulent fluctuations continue to grow away from the HP or begin to decrease past a certain distance? What is the primary location of the gyrating PUIs producing the IBEX ribbon? How do PUI induced instabilities contribute to the outer HS turbulence? Approach: Global simulations of ENA transport will determine the ENA distributions in the LISM (RT1). Our goal is to explain the secondary distributed flux and make predictions for the IMAP mission (Opher). We will model secondary PUI populations in the presence of turbulent fluctuations to determine the width and strength of the ribbon. The team will revisit existing theories of wave transmission across a magnetic discontinuity and nonlinear wave conversion in a low-beta plasma (Zank). To determine the turbulence extinction distance, weak Coulomb collisions will be added in a BGK or Fokker-Planck approximation (Zieger, Zank). Semi-analytic and empirical models will be developed to track evolution of the power spectrum of magnetic fluctuations with heliocentric distance (Florinski, Zank). Outcome: SHIELD will describe the extent and properties of the ribbon producing region and explain the evolution of turbulent magnetic fluctuations with distance from the HP.
RT4 has as director Joe Giacalone and as Deputy Director Romina Nikoukar.
State of Knowledge: GCRs are observed at multiple locations in the heliosphere and vary with the solar cycle, radial distance, and heliolatitude. V1 and V2 observed rapid CGR increases inside the HP. In the LISM, GCR anisotropies are observed, likely associated with transient disturbances. ACRs can cross the HP and are observed in the LISM. Knowledge Gap: The transport of GCRs and ACRs near to and across the HP are not understood; neither is the magnetic field configuration leading to the GCR anisotropies. Approach: Transport of GCR will be studied with the coupled SHIELD code (Florinski, Toth) using the pitch angle transport option to study the GCR anisotropy. The decay of ACRs outside the HP at V1 and V2 provide a constraints on ACR transport in the LISM. Giacalone and Florinski will use SHIELD to numerically integrate the trajectories of a large number of 10–100’s of MeV ACRs near the HP (including anisotropic turbulence), model their escape into the LISM, and compare their output with Voyager observations (Cummings, Hill, Stone). A new feature SHIELD found is a magnetic trap (AC11) on the northern flank of the HP. At the center of the trap, the magnetic field is especially weak, offering a preferred location for CRs to cross the HP. We are modeling the HP crossing points of CRs to elucidate how the magnetic trap modifies the effectiveness of the heliospheric shield (Florinski et al., 2021). SHIELD will develop code for community use on our website to calculate GCR and ACR intensities and energies at any point inside the heliosphere. A similar web interface, Helmod (https://helmod.org), is in operation, but a new run must be performed every time a new position is entered by the user. We will perform many runs using the method of backward-tracing stochastic integration of the Parker equation (similar to GALPROP) at multiple locations and create a lookup table of values which can be accessed quickly by the user. Similar look up tables will be made as more sophisticated methods become available from the coupled SHIELD model. SHIELD will also consider solar cycle variation of GCR modulation, including the effects of the varying HCS position and diffusion coefficients. Outcome: SHIELD will determine GCR transport and the radiation environment throughout the heliosphere.
State of Knowledge: Voyager observed multiple regions with anisotropic GCR distributions in the LISM due to dropouts of >211 MeV proton intensities perpendicular to the magnetic field. Cosmic ray electrons remain isotropic. Knowledge Gap: The cause of these anisotropies or why electrons behave differently than protons is not known. Approach: We will analyze data for these events and compare these data with existing theories (Kota, Giacolone). The energy dependence of these events will be determined (Nikoukar, Hill) by using LECP pulse height analysis data in a wide energy range (from MeV to GeV). A combination of magnetic trapping and cooling downstream of solar-induced transient disturbances and GCRs escaping from a single compression in the draping region has been proposed to explain observed anisotropies. Outcome: SHIELD will elucidate the physical processes that suppress GCR intensities near 90°C pitch angle and be used to improve GCR models of modulation and transport.
State of Knowledge: A surprise of the TS crossings was that neither Voyager spacecraft found evidence for ACR acceleration at the TS (Giacalone, 2012). Before Voyager, the paradigm was that diffusive shock acceleration of PUIs created ACRs at the TS (Fisk et al., 1974; Pesses et al., 1981; Giacalone, 2012). ACR intensities did not change at the TS, but slowly increased as the spacecraft moved deeper into the HS (Decker et al., 2010). Hypotheses for the ACR acceleration mechanism and location are: 1) in the flanks of the TS (Cummings et al., 2008); 2) in “hot spots” along a turbulent TS (McComas & Schwadron, 2006; Kota, 2010); 3) by reconnection (Guo et al., 2010; Opher et al., 2011; Keebler et al., 2022); 4) by turbulence processes (Zank et al., 2015); 5) by second-order Fermi acceleration; and 5) by turbulence generated by multi-ion magnetosonic waves (Dialynas et al., 2020). Knowledge Gap: We need to understand where and how ACRs are accelerated. Approach: SHIELD will explore acceleration of ACRs at the TS and in the HS using the coupled ACR transport and MHD models. Opher will explore consequences of the RT instability for ACR acceleration. SHIELD output will be compared with V1 and V2 ACR data from V1 and V2 (Florinski, Cummings); model runs will determine the contribution of reconnection, turbulence and TS conditions to ACRs. ACR anisotropies when V2 was outside and inside the sector zone (defined by the heliolatitudinal extent of the heliospheric current sheet will be compared (Hill); preliminary work suggests an energy dependence in the anisotropies that will constrain ACR acceleration models. Outcomes: SHIELD will determine where and how ACRs are accelerated and predict the ACR spectra and intensities throughout the heliosphere.
At the end of 5 years of Phase II (2022–2027), SHIELD will deliver a self-consistent, comprehensive global model of the heliosphere as a system that incorporates all critical physical processes (Figure 5; where year 1–5 corresponds to 5 years of Phase II of SHIELD). Toth with van der Holst leads the development of the SHIELD code. The global MHD model is the OH (Opher et al., 2003; 2009; 2020) component of SWMF (Toth et al., 2005; 2012), which is time dependent, can treat the thermal solar wind and PUIs (Opher et al., 2020) separately, and includes a kinetic treatment of the neutral H atoms (Michael et al., 2021). The suprathermal PUI and CR transport is done by the SPECTRUM (Florinski, 2015; Guo et al., 2018) particle code that will be inserted into the SWMF framework and coupled to OH (Kornbleuth, Opher). Solar wind turbulence is simulated with the Alfven Wave Solar Model (AWSoM) (van der Holst et al., 2014) model that is already part of the SWMF framework; SHIELD will extend AWSoM to handle compressible fluctuations (van der Holst, Zank). Turbulence and MHD parameters will be fed into SPECTRUM from OH. Both SPECTRUM and AwSOM parameters will be constrained by localized models, theory and observations. OH will be coupled to SPECTRUM, and AwSOM will drive the solar wind solution. This task builds on the prior experience of Florinski (Florinski et al., 2003; 2004; 2008) who has already developed a Parker-SDE solver (Florinski & Pogorelov, 2009; Florinski et al., 2013). To simulate PUI transport, a pitch angle transport module will be added (Chalov & Fahr, 2000; Malama et al., 2006; Guo & Florinski, 2014) to SPECTRUM. In the pitch angle option, the gyrotropic distribution is evolved by the focused transport equation (FTE) that depends on coefficients related to the mean and the fluctuating components of the magnetic field computed by AwSOM. The pitch-angle diffusion coefficients are obtained from quasi-linear theory; the perpendicular diffusion coefficient is from nonlinear guiding center theory (Zank et al., 1996b; Shalchi et al., 2004; le Roux et al., 2010) and the velocity diffusion coefficient is estimated from the intensity of the large-scale velocity fluctuations (Chalov et al., 1997; Florinski et al., 2003). In Phase I, SHIELD implemented a more efficient algorithm to evaluate the source terms for kinetic neutrals (Powell et al., 2021). SHIELD has already coupled OH to AMPS, a code that describes neutrals kinetically (Michael et al., 2022). We will perform the coupling between OH to FLEKS being developed now by Yuxi Chen. We will use the OH multi-fluid version to set the initial solution, then couple OH to SPECTRUM and update the solution. The description of kinetic neutrals will be extended (Chen, Kornbleuth, Michaels) to the new version of the code. When completed and validated, the model will be open source (DMP).
The PI provides overall leadership; the PM provides strategy and technical support and ensures integration. The RT Directors, Deputy Directors, Code Coupling Director, and BI Director execute thrusts. The roles are detailed in Sections 1.4, 2.2, 7, and Table 1.
The breadth of physics, of data analysis required for model verification, and of associated coding are well beyond the expertise of any single or even pair of PIs or institution. A global model inclusive of all relevant physical processes at all spatial resolutions (microphysics and macrophysics) can only be done in a Center environment with a tight focus on knowledge integration. We capture the kinetic transport of PUIs and CRs with the kinetic code SPECTRUM coupled self-consistently to a fluid code (SWMF/OH). The input is provided by localized simulations that treat from first principles kinetic effects like turbulence (Giacalone), reconnection (Drake, Swisdak) and acceleration of particles (Zank). SHIELD will couple SPECTRUM (Florinski) to the MHD global model (Opher, Toth) with input from localized codes. To benchmark the global code as well as the localized model and theory output, we use data from Voyager (Richardson, Cummings, Nikoukar, Gkioulidou, Hill, Dialynas), NH (Elliot, Zirnstein), IBEX (Fuselier, Dayeh, Reisenfeld) and Cassini (Dialynas).
SHIELD began with a large team, recognizing a broad collaboration is needed to realize Center goals and build team-based processes. This strategic decision positions us well for Phase II; it resulted in tight integration and early critical outputs. The team grew with Nikoukar studying GCR anisotropies, Gkioulidou with IMAP expertise, and van der Holst with expertise in turbulence and the AwSOM code. SHIELD also appointed a new BI director.
Figure 12 shows the management plan of SHIELD. The Center Director (CD) is Merav Opher, Professor of Astronomy at BU and William Bentinck-Smith Fellow at Harvard Radcliffe Institute, 2021–2022. Opher is responsible for the Center’s direction, progress and evolution. She ensures that SHIELD’s research, workforce development, diversity, outreach, and sustainability goals are met, and that the Center’s schedule, reports, and budget remain on track. A leader in the global modelling of the heliosphere and the comparisons of these models with data, Opher’s work has challenged the decades old paradigm of a comet-like heliosphere. She will ensure the Center works effectively within NASA policies and guidelines. The Project Manager (PM) is John Richardson (MIT). Richardson is responsible for day-to-day management, provides strategic, technical and logistical guidance, and ensures integration of Center activities. Additionally, he identifies and brings new members into SHIELD and assumes responsibility for SHIELD programming in the CD’s absence. Richardson is PI of the Voyager plasma experiment and a leader in observational studies of the solar wind, including making the first plasma observations of the TS, HS, HP, and LISM. Opher and Richardson work with the Executive Committee (EC), Advisory Boards, and NASA to ensure SHIELD has appropriate guidance, institutional resources and administrative support.
The EC serves as the core administrative team. Led by the CD and PM, the EC is comprised of the Research Thrust (RT) Directors, Code Coupling Director, and Broader Impacts Director. RT Directors (Sokol, Zank, Florinski, Giacalone) provide management, oversight and scientific direction for SHIELD. They are responsible for driving and integrating the research, executing on short- and long-term goals and milestones, and mentoring SHIELD’s young investigators (YIs). RT Directors meet with their teams bi-weekly, and with the CD and PM monthly. Deputy Directors (DDs, usually postdocs and early career researchers) work with the RT Directors and are part of the EC. DDs implement and augment the research by working across multiple RTs, run the weekly RT meetings and prepare annual reports. Two of the six DDs [Kornbleuth, Gkioulidou*, Nikoukar*, van der Holst, Turner, Gross] are female, advancing SHIELD’s diversity goals. The Code Coupling Director (Tóth) leads the coupling and BI Deputy (Gross) of the codes and his team meets weekly. The BI Director (Buxner) directs training, outreach and diversity programs, and ensures tight integration between the research and BI activities; her team also meets weekly. SHIELD will hire a full-time Project Administrator to provide administrative, programmatic, and financial support services. Collaborators (non-funded) support SHIELD research and BI activities. Table 1 profiles the team.
SHIELD is structured to ensure transparency in decision-making, coordination across the research and BI thrusts, and the flexibility to make timely and appropriate changes in programmatic direction. The ability to react to new discoveries is particularly important for the outer heliosphere where new data and models are changing current views. Agility and teaming are prioritized; protocols support effective resource allocation, continual strategic planning and robust communication. The success of Phase I is a direct result of rigorous operational protocols; key elements are retained in Phase II.
Decision Making: Decision making is the responsibility of the CD, following consultation with the PM, EC and Advisory Boards. The CD, PM, and EC evaluate projects annually to assess alignment and progress of thrusts, using performance indicators. Gaps are identified and mitigated. Evaluation leads to resource recommendations with final decisions made by the Director in close consultation with the PM. The CD communicates decisions to and receives concurrence from the EC, EABs and NASA. The CD shares decisions with SHIELD team and updates the strategic plan.
Oversight: Active oversight has and will continue to ensure effective operations and management. The External Advisory Board (EAB), a Postdoc and Student Advisory Board (PSAB), and a Sustainability Board (SB) ensure effective oversight, operation and management. EAB: The EAB provides guidance and oversight with regard to vision, scientific direction and strategy, project execution and progress, conflict resolution, risk mitigation, and sustainability. It meets yearly to review SHIELD; findings are documented in an annual report. Board members also meet with the CD and PM as needed. Members are: Ralph McNutt (Chair), Chief Scientist, Space Science, Space Exploration Sector, JHU/APL; Jeffrey Hughes, Professor of Astronomy, BU; Mae Jemison, PI, 100 Year Starship; Janet Luhmann, Senior Fellow, Space Sciences Laboratory PI, IMPACT, SSL/UC-Berkeley; Sara Seager, Class of 1941 Professor of Physics and Planetary Science, MIT; Alan Stern, Associate VP, Space Science and Engineering Division, SwRI; PI, New Horizons; and Janet Vertesi, Associate Professor of Sociology, Princeton U. PSAB: The PSAB will be formed from SHIELD postdocs and students. SHIELD will support 5 postdoctoral fellows (1 at BU 1 at UAH, 1 at SwRI, 1 at UA, and a SHIELD postdoc, funded by CERIF, to be competed for by the SHIELD institutions). The co-Is and Directors will nominate 1-2 PhD students to serve on the PSAB, which will meet quarterly with the EC to provide input and suggestions on strategy, mentoring, professional development, and Center communications, supporting SHIELD’s reverse mentoring (Jordan & Sorell, 2019) goals (Section 8.4, Mentoring). The PSAB will elect its own chairperson; each will serve a one-year term to allow multiple postdocs and students to develop leadership skills. Assignments will be for 2 years, assuring continuity. The PSAB will present to the EAB during its annual meeting. SB: The Sustainability Board will advise SHIELD in formalizing and launching the Center’s sustainability initiative, the Institute for Habitable Astrospheres (IHA). The IHA, using SHIELD’s global model, will support convergent research focused on the origin of life that can be solved by combining expertise in radiation, biology, chemistry, and heliophysics. A one-day workshop will kickstart the IHA. The Board is composed of Avi Loeb (Harvard), Sara Seager (MIT) (co-directors of IHA), Alan Stern, and Ralph McNutt. The SB will meet with the CD, PM, and DDs annually.
Risk Management and Conflict Resolution: Clear procedures are in place to mitigate risks and manage and resolve conflict. The CD and PM have successfully resolved conflicts, e.g., differences about publications, through consensus building and shared decision making. As conflicts arise and initial recommendations are made, the CD and PM solicits comments from EC. In the unlikely event differences cannot be resolved through consensus building, the EAB is engaged to reach an outcome that protects NASA’s investment. RTs that fail to deliver against milestones will be terminated or redirected, although SHIELD management will be mindful of individual researchers’ career development. The CD, in consultation with the EC and the EAB, may reallocate resources, re-direct funding between thrusts, and add, remove, or re-assign Directors. Advice and concurrence from NASA will be sought when required. SHIELD’s full risk mitigation plan is articulated in its Strategic Plan (C7); select operational risks are noted in Table 3.
As established, SHIELD’s research is tightly integrated. This coupling is an inherent risk. The dependency of the various thrusts and tasks, and in some cases, their linear nature, could potentially delay or stop work in other areas if key tasks are not completed on time. The CD has funding in reserve (CERIF) and will make available the necessary resources (human, financial, technical) to advance critical tasks. The monthly EC meetings will enable early identification and mitigation of challenges and risks.
SHIELD is designed to assimilate current knowledge of the outer heliosphere into a single model while identifying areas where our understanding of the physics is lacking and filling these gaps. This research requires close communications within each thrust and a close integration of efforts between the thrusts to achieve the Center’s vision (Figure 5). Thrust integration is advanced by active participation of the EC in Center activities. The CD and PM participate in all thrusts, the development of the SHIELD model and BI. EAB member Vertisi, who has published extensively on what institutions and flows of people are required (Vertesi, 2020) to craft scientific knowledge, advised SHIELD on our broad approach. We exploit team science, support multi-directional information flow and leverage established infrastructure and managerial experience to realize objectives. The PM plays a major role in establishing and ensuring that communication lines remain open and efficient, that data is constantly collected and fully up to date, and that research and BI thrusts are on schedule and achieving specific milestones. Additional points of integration are SHIELD’s students and young investigators and supported BI activities. Training opportunities are provided for young investigators, post-doctoral researchers, graduate students and undergraduates, who are not only mentored in the practice of team science but have opportunities to pursue collaborative research through the Central Education, Recruitment, and Impact Fund (CERIF), discussed below. To promote integration, SHIELD allocated funds to send postdocs and students to partner institutions to support cross-fertilization and new skill acquisition.
SHIELD will rely on the extensive research from organizational science and the science of team science (Guimera et al., 2005; Jones et al., 2008; Börner et al., 2010; NRC, 2015), to advance our team-based approach and address key challenges. Our management model supports collaboration at every level; top down, bottom up, and horizontally. SHIELD has already established a culture of collaboration as evidenced by its Phase I accomplishments and publications. As discussed in BI, SHIELD will provide training for young investigators focused on team science, reflexivity training, team coordination, and communication. Effective communication and collaboration are central to integrating SHIELD’s research, building and supporting teams, and developing strategic directions. Table 4 shows our meeting schedule, which was used successfully in Phase 1 and will be maintained in Phase II. Frequent interactions keep collaborations active and ensure progress. Quarterly Center-wide virtual seminars are another vehicle for collaboration, knowledge sharing and engagement with the wider heliospheric community. State-of-the-art technology enables productive virtual meetings, facilitates long-distance collaboration and provides platforms for file storage and sharing.
SHIELD recognizes the urgent need to build a broad coalition to realize its ambitious vision and longer-term sustainability goals (IHA). We welcome and invite participation of outside researchers to help realize strategic objectives. In Phase I, for instance, SHIELD established a workgroup to investigate Compton-Getting (CG) observations, which included members from two RTs and three members from outside SHIELD (identified by PM Richardson). The CG group is responsible for two SHIELD publications (Cummings et al., 2021; Richardson et al., 2021). Engagement with Broader Community: SHIELD seeks to attract talented researchers whose interests match or expand SHIELD and contribute to a Center culture that is diverse with respect to gender, age, racial/ethnic background, and sexual orientation. SHIELD will: 1) maintain an active public website to share information and progress as well as enable researchers to join as “affiliate” members; 2) engage the broader community by making 1 day of its annual meetings a workshop open to everyone; 3) use the IHA to engage the broader community; and 4) invite experts and role models to participate in BI activities (Section 8.4).
Evaluation: All scientific and BI activities (education, training, diversity, and outreach) will be assessed internally and externally. The EC and the EAB will evaluate the research; an external evaluator will assess BI activities and determine if SHIELD is fulfilling its goals. Each thrust will submit an annual report that summarizes progress in its scientific and BI activities and proposes plans for the following year. These documents will form the basis of SHIELD’s annual report. BI programs will be evaluated at the start of the program for baseline assessments; annual surveys and interviews with SHIELD participants will provide summative data related to the overall program success. Performance indicators used by the EC to evaluate the status of the RTs include: 1) progress towards SHIELD’s milestones, 2) scientific and technological advances (as measured by publications, conference presentations, invited presentations and seminars, impact factors); 3) level of interaction both within the RT and with other RTs; 4) evidence of mentorship and training of young scientists. RT Directors will provide quarterly reports listing notable advances and difficulties as well as publications (See Decision Making and Risk Management). Criteria used to evaluate the status of the BI activities include: 1) the number and diversity of participating faculty, scientists, young investigators, and students at all levels; 2) inclusion of the participation of women, URMs, persons with disabilities, and LGBTQIA + individuals in BI activities; 3) integration into Center research; and 4) evidence of effective inter-institutional collaboration. The EAB will complete an independent assessment and report findings and recommendations. SHIELD will use all findings to enable continuous improvement. Continuous Improvement: The EC will solicit seed proposals each year to bring new ideas, expertise, and personnel, including students, to the Center through CERIF. CERIF: This new initiative will support 5 graduate students (1 allocated to IHA) to establish direct linkages with collaborators to deepen appreciation for SHIELD’s team-based science. The EC will evaluate proposals with advice from the EAB; final selection of the awarded graduate students will be made by the CD, with a preference for diverse researchers. Applicants will be encouraged and funded to visit and interact with SHIELD partners. After 1–2 years, the results of these seed projects will be reviewed, after which the activity will either be incorporated into the RT or BI or phased out.
The strategic plan (SP) is a living document. It is updated annually. The CD and PM lead strategic planning and assessment exercises and convene the EAB, PSAB and SB annually. SHIELD’s SP provides a detailed research plan, timeline and risk mitigation strategies.
An important lesson learned from Phase I is the centrality of team-based science. SHIELD established team processes, prioritized team composition and supported virtual collaboration. The team realized relatively early the need to have flexible funding to support team science, which resulted in CERIF in Phase II. CERIF will support students, seed projects, research exchanges, and will be used to manage risks. A second lesson learned is the need to elevate the Center’s knowledge transfer and professional development activities. SHIELD lacked a formal mechanism to develop young and diverse scientists. The new Bench-Strength-Development program provides multi-level and reverse mentoring, training, exchanges, and professional development, and prepares each young scientist to become an independent investigator able to execute team-based science within SHIELD and any other future endeavors. Another lesson is the need to focus dually on both the Center’s 5-year goal and longer-term vision. In Phase I, the EAB urged a spin-off Institute to ensure broad impact and the continuation of SHIELD well beyond the 5 years of the DSC. In Phase II, SHIELD will advance the IHA as discussed in Section 1.3 and Section 7.3, Oversight. The CD, PM and DDs will work in concert with the Sustainability Board to develop a workshop series broadly focused on habitability. SHIELD’s model will be open source and archived with CCMC to remain a living tool for the community.
Figure 13 shows the overall structure of the Broadening Impacts activites of SHIELD. In Phase I, SHIELD launched signature education and outreach programming, spanning K-12 to young investigators, in pursuit of its vision to change the face of heliophysics. As part of a campaign to bring diverse voices to STEM, SHIELD initiated You Can’t Be What You Can’t See testimonials and Creating Science Quartets (Box 2) webinars. This innovative programming, the brainchild of CD Opher and Phase I BI Director Wong, collects stories and testimonials from graduate students, young researchers and their mentors on finding community and overcoming challenges. Testimonials have touched on topics such as the impostor syndrome, gender fluidity, the immigrant experience, and the impact learning disabilities can have on trajectories in STEM fields. SHIELD’s webinars profile eminent scientists and trailblazers, emerging scholars, and NASA managers who talk on career development, space physics, and scientific discovery. Notable speakers include: Fran Bagenal (UC-Boulder), Suzy Dodd (NASA), Nicky Fox (NASA), Margaret Kivelson (UCLA), Andrea Razzaghi (NASA), and Tom Krimigis (APL). The reception from these heart-warming stories and un-shielded approach to outreach has been overwhelming. Phase II builds upon this rich and successful tapestry with new programming focused on broadening participation, professional development and mentoring, and knowledge sharing. Specific aims are to: 1) increase the recruitment, inclusion, and retention of traditionally underrepresented (URM) groups (with a predominate focus on racial/ethnic minorities, women, LGBTQIA+, and first-generation college students) pursuing STEM careers and entering post-secondary education; and 2) train, mentor, and build leadership skills for emerging and early career scientists, teaching team-based science. CD Opher is one of the very few women in space physics. A non-US born citizen who identifies as LGTBQ, Opher knows first-hand the challenges of working and succeeding in this predominately masculine field. She and BI Director Buxner are personally vested in nurturing a Center culture that is inclusive, promotes a sense of belonging, and provides academic and personal supports to retain URM students in particular. For more details on the broader BI effort see Buxner et al., 2023.
Box 2 Select Phase I BI Accomplishments
Broadening Impacts.
• Launched ‘pathway for scientists’ series, Creating Science Quartets: Bringing Diverse Voices, with enrollment exceeding 100. Upcoming seminars focus on the Latin and Black experience in STEM, respectively.
• Established You Can’t Be What You Can’t See initiative, a video/testimonial series showcasing diverse PhD students and junior researchers in space physics. 9 stories profiled.
• Established monthly webinar series (12 webinars as of 10/21), focused on career development (improving writing skills and delivering effective scientific presentations), universal themes such as imposter syndrome and immigration, and Young Voices (a platform for PhD students and postdocs to discuss research and career paths).
Communication and Outreach.
• Launched website: Viewed by 2000 users since April 2020.
• Established social media presence (Facebook, YouTube).
• Hosted virtual ‘happy hours’ to build community.
• Interviewed by popular news outlets, including NPR.
Knowledge Sharing.
• Published 12 joint articles (4 or more senior investigators).
• Appointed four young scientists, 2 females, to co-lead RTs.
The heliophysics community, like most other science communities, has diversity and inclusion problems (NRC, 2013), particularly around gender and ethnicity. The physics workforce is majority white and male; it is also aging and retirement-eligible, necessitating the need to accelerate efforts to train the next-generation of space physics workers. Professional societies advise science education better to prepare, “future scientists with the knowledge and skills required for effective knowledge integration and collaboration within a science team or larger group” (NRC, 2015) while establishing cultures where students from marginalized communities can thrive. These challenges drive SHIELD’s education and human-resource-development, broadening participation and outreach goals to train a new type of heliophysicist, one fluent in team science and capable of working in highly transdisciplinary, collaborative environments and make measurable improvements in the diversity of the heliophysics workforce pathway.
Sanlyn Buxner, Senior Research Scientist at the Planetary Science Institute and Director of Graduate Studies in Teaching, Learning, and Sociocultural Studies at UA, will be the BI Director. Buxner brings over 15 years of experience recruiting and supporting diverse undergraduate and graduate students. Nicholas Gross (BU) is the Deputy Director of BI and has extensive education experience within the space physics community, including past service as an Education co-director for a NSF Science and Technology Center. He will organize SHIELD’s REU program and provide critical support to the Summer School. Experts supporting the core team are Sarah Hokanson (BU-Provost Office) who will focus on mentoring and Cherilyn Morrow (PUNCH Mission), who will focus on communication. Judi Burgess, Director of Labor Relations (BU), serves as an advisor on diversity programming.
Aim 1: Broadening Participation: SHIELD proposes to increase the inclusion of URM groups (women, racial/ethnic minorities, LGBTQIA+, individuals with a self-reported disability, first generation) pursuing STEM careers and entering post-secondary education; 2) increase retention and promote development of female and URM young investigators through professional development, mentoring, and skills training; and 3) provide diversity, equity, inclusion and access (DEIA) training and other tools to promote inclusive learning environments. The centerpiece of this effort is the SHIELD Distributed REU Program. Undergraduate research experiences have raised awareness of the discipline; fifty (50%) of space physics graduate students were involved in an undergraduate research experience (NRC, 2013). SHIELD will support a summer REU program.
Gross and Buxner will recruit and support 5 diverse undergraduate students each year to participate in a 12-week research experience at BU, UAH, or UM. Over 5 years, ∼25 undergraduates will be supported, with at least one-third of students being from a targeted URM groups (female, racial/ethnic minorities, LGBTQIA+, or persons with disability. SHIELD will partner with other successful space science training programs including L’SPACE, which has over 5,000 alumni, over half of whom attended or graduated from a MSI.
AIP identified three major factors critical to supporting and retaining groups underrepresented in STEM. These include: 1) the need for students to feel a sense of belonging, 2) the need to perceive themselves, and be perceived by others, as future physicists and astronomers, and 3) the need for effective teaching and mentoring students.
To organize this distributed summer REU program, SHIELD will leverage existing REU programs at BU, UAH, and UM. This approach will permit students to use existing housing arrangements and participate in social activities. Each REU student will have a mentoring plan and at least one mentor. SHIELD will work with these participants to build a virtual REU SHIELD community to collaborates on space science research. Early career scientists and graduate researchers engaged in SHIELD will join the mentoring team for the REU students. REU students will be expected to produce an abstract and poster and funded to present their work at a professional conference. REU participants also will receive instruction about the SHIELD research enterprise, ethics, scientific writing, presentation, and pathways to graduate school or other STEM careers. Mentorship and leadership skill development will be accomplished via SHIELD’s new Bench-Strength-Development (BSD) program.
SHIELD will also establish a DEIA Action Workgroup to advise on center climate issues and recommend policies, procedures and practices that will bring about more equitable outcomes for everyone. Burgess will advise on the development of this workgroup. She has significant relationships with HBCUs and served with CD Opher on BU Diversity and Inclusion (D&I) Action Team. Finally, SHIELD will train its leaders to become DEIA advocates. Training in overall DEIA efforts will be conducted by Hokanson each quarter and at the annual team meeting.
Aim 2: Education and Human Resources Development (EdHRD): EdHRD programs are built around four initiatives: 1) training/skills development; 2) SHIELD Summer School; 3) Bench-Strength-Development; and 4) Mentoring/mentoring training. Training/Skills Development: A suite of programs will support and train new, emerging, and established SHIELD scientists, while furthering BP goals of the center. Professional Development (PD): PD courses for SHIELD students, early career and other SHIELD members will cover topics in science, communication, DEIA, mentoring, and management. Onboarding Training: All associated students and early career scientists will attend training in team science and working in transdisciplinary collaborative environments led by Opher, Buxner and Gross. Each year, new students and scientists will develop a common set of foundational skills while building teamwork and interpersonal connections. Other training will focus on reflexivity training, team coordination, and communication. Skills Training: Organized by Buxner, ongoing online interactive workshops will include (a) publication and presentation preparation; (b) proposal writing; (c) organizing conference sessions; (d) networking; and (e) outreach to internal and external stakeholders. Students will outline and chart career paths as they work toward presenting research to peers in annual meetings such as the AGU. Communication Training will focus on communicating effectively with scientific and non-scientific audiences. In addition to the resources of the SHIELD team, Morrow (PE lead, PUNCH Mission) will provide a workshop series on communicating with broad audiences. Students and early career scientists will have multiple opportunities to present at outreach events, annual meetings and conferences. Summer School: The Heliophysics SMD Decadal Survey calls out summer school as an important opportunity to train graduate students in the concepts and tools of space physics. Current summer school opportunities are routinely oversubscribed, suggesting demand for similar programming. SHIELD will offer a Summer School at BU building on the prior experience of CD Opher [who ran a successful NSF CAREER Summer School (Opher, 2012)] and Gross (an organizer and instructor for both the LWS Heliophysics Summer Schools and the Boulder Space Weather Summer School). Recruitment will be done through minority professional societies and MSIs as well as L’SPACE. We expect that 20 participants a year will attend the Summer School. Our target is to have 50% of the participants be from URM groups. SHIELD’s Summer School will adopt proven techniques from undergraduate education research including flipped classroom (Abeysekera & Dawson 2005, peer instruction (Crouch and MazurPeer, 2001), tutorials, and project based learning (https://www.pblworks.org/what-is-pbl). Bench Strength Development (BSD): Creating a strong and diverse bench of talent is vitally important for the success and sustainability of SHIELD. BSD is a new Phase II initiative to bring young investigators (YIs) into leadership roles within SHIELD and provide training and support to prepares postdocs and early career faculty to lead team-based science efforts. As noted in Section 2, RTs are led by a senior and junior investigator (Deputy); two of the six deputies are non-US born females. YIs will co-mentored by a RT Director and a co-I from another institution, supporting cross-training. BSD programming will enable YIs to: 1) increase content knowledge and gain technical skill in a team-based environment; 2) receive mentorship; 3) develop leadership skills; 4) enhance oral and written communication and presentation skills; and 5) build internal and external networks. Through BSD, reverse mentoring is also supported. YIs will provide guidance, strategy, and new ways of thinking to senior investigators through co-leadership of thrusts, presentations to EC and EAB, and participation in annual meetings (Sections 1 and 2). The CD and RT Directors will oversee and implement BSD; the BI Director will provide input. Mentoring and Mentoring Training: Mentoring and professional development are critically important components of SHIELD’s EdHRD. SHIELD will support multi-mentoring (De Janasz & Sullivan, 2004) and make available to postdocs and graduate students at least two mentors from different institutions. Mentors will be assigned by the EC. Creation of specific annual goals, meeting schedules, deliverables, and measures of progress will help to ensure effective impact and positive outcomes. Hokanson in collaboration with Gross and Buxner will run a 2-hour Inclusive Mentoring Workshop at the SHIELD annual meeting to improve research mentorship skills, from developing individual development plans to establishing and maintaining productive mentoring relationships with diverse mentees. Hokanson will conduct two more annual trainings for new team members and mentees. Training also will be provided for mentees with monthly check-ins facilitated by Gross and Buxner, and a yearly evaluation by the External Evaluator. All mentees, including DDs, postdocs, CERIF-supported graduate students, and REU students, will have a mentoring plan on file with SHIELD and will be included in the evaluation effort. Mentoring will be assessed using research-based instruments (Fleming et al., 2013) and Social Network Analysis (Ginexi, 2017).
Aim 3: Heliophysics Community Building and Outreach: SHIELD’s community building activities include formal and informal interactions to address issues unique to intersectionality. Building on Phase I, we will continue to sponsor a broad set of activities open to the whole space physics community with specific focus on undergraduate and graduate students, and post-doctoral researchers. Webinars: The Webinar series (Box 2) will continue. It will be expanded to include speakers from all adjacent fields of heliophysics and target 10 webinars a year divided between “Young Voices” and “Creating Science Quartets.” Public Outreach: SHIELD will provide emerging scientists (graduate students, postdocs) and early career scientists with opportunities to present their work and their experience in becoming a scientist. Morrow, in collaboration with Gross and Buxner, will conduct regular communications training for emerging and early career scientists. This will be part of the professional development workshops offered over the course of SHIELD. Podcasts: Facilitated by Buxner, SHIELD scientists will join an ongoing initiative of the PUNCH Mission public engagement program to participate in SciHArt, a podcast series facilitated by student (young professional) hosts who work with the Fiske Planetarium at the UC Boulder. The primary audience is STEM-interested learners at the late high-school, undergraduate, and early graduate levels. SciHArt interviews leaders in science, engineering, and science communication who are in different phases of their career journey, from undergraduate researchers to senior professionals playing leadership roles on NASA missions. SHIELD will provide up to five scientists a year for interviews, beginning with each Deputy Director and including early career scientists. Likewise, we will expand the reach of the SciHArt audience through our outreach networks. Video Testimonials: SHIELD will continue to support its “You Can’t Be What You Can’t See” video/testimonial series, which profile and provide support for emerging scientists from diverse backgrounds. In Phase II, SHIELD will invite researchers from the heliophysics community to video interviews regarding their experience, the challenges and barriers they faced, and how they persevered. These videos are important for inclusivity building and are featured on our website and Youtube channel. SHIELD will target 5 videos a year. Website: The SHIELD website (https://shielddrivecenter.com) will be maintained/expanded. As the public face of the Center, the website will feature SHIELD research, scientific highlights, publications, access BI programming and information to become an affiliate SHIELD member.
The SHIELD global model consists of the Outer Heliosphere (OH) component of SWMF code (UM and BU), the Particle Tracker (PT) component FLEKS (UM) (that is being currently developed), the particle transport code SPECTRUM (UAH), and the Alfven Wave Solar atmosphere Model (AWSoM) that simulates turbulence in the solar wind. (b) These codes are coupled to provide a comprehensive self-consistent model of the Outer Heliosphere (c) The SHIELD model will produce multi-component number densities, temperatures, flow velocities, particle distributions, and magnetic fields with both 3D, 2D and line plots. 3D file sizes are around 10 GB (SWMF/PT). File formats are both in binary and ascii. The restart files for a typical three-dimensional domain can reach 100 GB. (d) Several 3D and 2D files are generated per run and stored at NASA Ames Pleiades. Each person performing the runs will be responsible for the long-term storage (as in lou NASA Ames); (e) Documentation: SWMF is documented in Latex and XML that are compiled into PDF manuals. The source code is also documented. The full SWMF is on the UM GitLab server (http://gitlab.umich.edu/swmf_software), and a major part of it, including BATS-R-US used in this project is mirrored to GitHub (https://github.com/MSTEM-QUDA) under a non-commercial open-source license. The Opher group will produce the documentation for OH, Chen for FLEKS/PT, and Florinski for SPECTRUM. All will be available at Gitlab.; (f) SHIELD output will be delivered Years 1-5 to CCMC. As each milestone is completed; (g) The entire model will be delivered to CCMC.
[A] (a) The UA hybrid model describes particle evolution in turbulence.; (b) It derives the particle distributions needed for the global model. (c, d) Output and data volume: 1) multi-component number densities, flow velocities, magnetic-field vectors, electric-field vectors, energetic particles fluxes in several energy ranges, as color-coded images plotted over the entire 2D spatial domain at selected simulation times. Each simulation produces 10–20 50B images. 2) reduced 1D distribution functions vs. momentum and flux vs. energy for each ion species, averaged over portions of the simulation domain at several simulation times. There are 20–30 100 kB files in ascii format 3) Reduced 1D line plots of plasma parameters and energetic particle fluxes as a function of a given spatial direction in the simulation domain for several pre-chosen cuts through the domain. There are 10–20 1 Mb ascii. 4) A table of simulation parameters, and conversions for each simulation performed in excel format (<100 kB); (e) planned documentation will be produced and available at Gitlab (f) Year 5 (g) Archived at CCMC.
[B] PIC code p3d: (a) A particle-in-cell (PIC) code that gives a nearly complete physical description of a plasma and accurately simulates processes at kinetic scales. (b) SHIELD uses it to study small-scale processes like shock acceleration and reconnection. (c) The data products (binary) are snapshots of number densities, temperatures, magnetic fields, and particle distributions in time and restart files can be used to advance a simulation from a particular point in time. (d) A single three-dimensional PIC run can produce 50 TB of data. e) Planned documentation will be produced and available at Gitlab f) These will be stored as runs are made in years 1–5. (g). Due to its volume such data are usually stored at the facility where they are produced. The High Performance Storage System (HPSS) at the National Energy Research Scientific Computing Center (NERSC) has been used for archival storage since 1998.
[C] Data Outputs: We plan to use only publicly available spacecraft data, so archiving is not needed.
[1] SHIELD uses SWMF/OH (fortran); PT (FLEKS): C++; SPECTRUM: (C++)which all will transition to open source; The SWMF is developed and maintained under Git, which is a distributed version control system. The full SWMF is on the U of M GitLab server (http://gitlab.umich.edu/swmf_software), and a major part of it, including BATS-R-US used in this project, is mirrored to GitHub (https://github.com/MSTEM-QUDA) which provides open-source access under a non-commercial license. The OH component will be released as open source by Opher’s group. The UAH transport code SPECTRUM will track CR and PUI trajectories and couple to the SWMF/OH and PT (FLEKS code). The code is written in C++ with elements of CUDA. It will be released under the MIT open-source license and made into a GitHub project, which will ensure its free accessibility by the community. The code is already documented using Doxygen; a user manual will be written before the code is released. SWMF/FLEKS is being developed now (Chen et al., 2021) and will be openly accessible at the U of M GitLab server for download as well as in the CCMC. OH application will be released as open-source by Opher’s group.
[2] Hybrid model: Fortran code that manipulates distribution functions into other forms and reduces them to produce densities will be placed in CCMC. The hybrid code will be available on github as open-source access under a non-commercial license as part of the proposal.
[3] PIC code: p3d is primarily written in Fortran and uses the C and m4 pre-processors. Parallelization is done via MPI. A user manual will be written before the code is released. Several Fortran and IDL programs that can be used to read and analyze the data will be uploaded as well. p3d will be available on as open-source access under a non-commercial license as part of the proposal.
The data management and coordination of data acquisition, analysis, and archiving of is overseed by the P.I. (Prof. Merav Opher). The Coupling Code Group director Dr. Gabor Toth will oversee the task to make the SHIELD model open source. The UAH postdoc will aid with SPECTRUM. Marc Kornblueth from BU will aid with SWMF/OH. Localized models: The UA Postdoc will lead the archiving of the hybrid code. For p3d, the team member that performs a numerical simulation will be responsible for archiving the data. That includes saving the data, as well as any codes necessary for interpreting it, in an accessible format to the relevant high-performance storage system.
All authors listed have made a substantial, direct, and intellectual contribution to the work and approved it for publication.
This work is supported by NASA grant 18-DRIVE18_2-0029 as part of the NASA/DRIVE program titled “Our Heliospheric Shield”, 80NSSC22M0164, https://shielddrivecenter.com. Some of the figures were produced by AH, Graphic Designer, adamhong.com.
The authors declare that the research was conducted in the absence of any commercial or financial relationships that could be construed as a potential conflict of interest.
All claims expressed in this article are solely those of the authors and do not necessarily represent those of their affiliated organizations, or those of the publisher, the editors and the reviewers. Any product that may be evaluated in this article, or claim that may be made by its manufacturer, is not guaranteed or endorsed by the publisher.
ACR, Anomalous cosmic ray; BI, Broadening Impacts; BP, Broadening Participation; BSD, Bench Strength Development; CD, Center Director; CE, Charge exchange; CR, Cosmic ray; EAB, External Advisory Board; EC, Executive Committee; GCR, Galactic cosmic rays; HP, Heliopause; HS, Heliosheath; IHA, The Institute for Habitable Astrospheres; IMAP, Interstellar Mapping and Acceleration Probe; LISM, Local interstellar medium; LECP, Low energy charge particle; NH, New Horizons; OH, Outer Heliosphere; PM, Project Manager; PI, Principal Investigator; PSAB, Postdoc and Student Advisory Board; PLS, Plasma science experiment; RT, Research Thrusts; TS, Termination Shock; URM, Under-represented Minority; V1, Voyager 1; V2, Voyager 2.
Abeysekera, L., and Dawson, P. (2005). Motivation and cognitive load in the flipped classroom: Definition, rationale and a call for research. High. Educ. Res. Dev.34 (1), 1–14. doi:10.1080/07294360.2014.934336
Axford, W. I., Dessler, A. J., Gottlieb, B., Dessler, A. J., and Gottlieb, B. (1963). Termination of solar wind and solar magnetic field. Astrophysical J.137, 1268. doi:10.1086/147602
Baranov, V. B., and Malama, Y. G. (1993). Model of the solar wind interaction with the local interstellar medium: Numerical solution of self-consistent problem. J. Geophys. Res.98 (A9), 15157–15163. doi:10.1029/93JA01171
Börner, K., Contractor, N., Falk-Krzesinski, H. J., Fiore, S. M., Hall, K. L., Keyton, J., et al. (2010). A multi-level systems perspective for the science of team science. Sci. Transl. Med.2 (49), 3001399. doi:10.1126/scitranslmed.3001399
Burlaga, L. F., Florinski, V., and Ness, N. F. (2015). In situ observations of magnetic turbulence in the local interstellar medium. Astrophysical J.804, L31. doi:10.1088/2041-8205/804/2/L31
Burlaga, L. F., Florinski, V., and Ness, N. F. (2018). Turbulence in the outer heliosheath. Astrophysical J.854, 20. doi:10.3847/1538-4357/aaa45a
Burlaga, L. F., Ness, N. F., Berdichevsky, D. B., Jian, L. K., Park, J., and Szabo, A. (2020). Voyager 1 and 2 observations of a change in the nature of magnetic fluctuations in the VLISM with increasing distance from the heliopause. Astronomical J.160, 40. doi:10.3847/1538-3881/ab94a7
Burlaga, L. F., and Ness, N. F. (2016). Observations of the interstellar magnetic field in the outer heliosheath:voyager 1. Astrophysical J.829, 134. doi:10.3847/0004-637x/829/2/134
Burlaga, L. F., Ness, N. F., and Stone, E. C. (2013). Magnetic field observations as voyager 1 entered the heliosheath depletion region. Science341 (6142), 147–150. doi:10.1126/science.1235451
Buxner, S., Gross, N., Opher, M., Wong, J., and Richardson, J. (2023). SHIELD DRIVE science center: Efforts in diversification and inclusion in heliophysics. Front. Space Sci. Astrophysics10, 1155843. in press. doi:10.3389/fspas.2023.1155843
Cairns, I. H., and Fuselier, S. A. (2017). The plasma depletion layer beyond the heliopause: Evidence, implications, and predictions forvoyager 2andnew Horizons. Astrophysical J.834, 197. doi:10.3847/1538-4357/834/2/197
Chalov, S. V., Fahr, H. J., and Izmodenov, V. (1997). Spectra of energized pick-up ions upstream of the two-dimensional heliospheric termination shock. II. Acceleration by Alfvenic and by large-scale solar wind turbulences. Astronomy Astrophysics320, 659.
Chalov, S. V., and Fahr, H. J. (2000). Pick-up ion acceleration at the termination shock and the post-shock pick-up ion energy distribution. Astronomy Astrophysics60, 381.
Chen, Y., Toth, G., Zhou, H., and Wang, X. (2021). Fleks: A flexible particle-in-cell code for multi-scale plasma simulations. ESS Open Archive. December 09. Avaialabe at: https://essopenarchive.org/doi/full/10.1002/essoar.10508070.3.
Crouch, C., Mazur, E., and Peer, I. (2001). Peer Instruction: Ten years of experience and results. Am. J. Phys.69, 970–977. doi:10.1119/1.1374249
Cummings, A. C., Stone, E. C., McDonald, F. B., Heikkila, B. C., Laf, N., and Webber, W. R. (2008). Anomalous cosmic rays in the heliosheath. AIP Conf. Proc.1039, 343.
Cummings, A. C., Stone, E. C., Heikkila, B. C., Lal, N., Webber, W. R., Jóhannesson, G., et al. (2016). Galactic cosmic rays in the local interstellar medium:voyager 1observations and model results. Astrophysical J.831, 18. doi:10.3847/0004-637x/831/1/18
Cummings, A. C., Stone, E. C., Richardson, J. D., Heikkila, B. C., Lal, N., and Kota, J. (2021). Astrophysical J.906 (2), 126. doi:10.3847/1538-4357/abc5c0
Dahlin, J., Drake, J. F., and Swisdak, M. (2017). The role of three-dimensional transport in driving enhanced electron acceleration during magnetic reconnection. Phys. Of Plasmas24 (9), 092110. doi:10.1063/1.4986211
Dahlin, J. T., Drake, J. F., and Swisdak, M. (2016). Parallel electric fields are inefficient drivers of energetic electrons in magnetic reconnection. Phys. Of Plasmas23 (12), 120704. doi:10.1063/1.4972082
Davis, L. (1955). Interplanetary magnetic fields and cosmic rays. Phys. Rev.100 (5), 1440–1444. doi:10.1103/physrev.100.1440
de Janasz, S. C., and Sullivan, S. E. (2004). Multiple mentoring in academe: Developing the professorial network. J. Vocat. Behav.64, 263–283. doi:10.1016/j.jvb.2002.07.001
Decker, R. B., Krimigis, S. M., Roelof, E. C., and Hill, M. E. (2010). Variations of low-energy ion distributions measured in the heliosheath. AIP Conf. Proc.1302, 51. doi:10.1063/1.3529990
Dessler, A. J. (1967). Solar wind and interplanetary magnetic field. Rev. Geophys. Space Phys.5, 1. doi:10.1029/rg005i001p00001
Dialynas, K., Galli, A., Dayeh, M. A., Cummings, A. C., Decker, R. B., Fuselier, S. A., et al. (2020). Combined ∼10 eV to ∼344MeV particle spectra and pressures in the heliosheath along the voyager 2 trajectory. Astrophysical J.905 (2), L24. doi:10.3847/2041-8213/abcaaa
Dialynas, K., Krimigis, S. M., Mitchell, D. G., Decker, R. B., and Roelof, E. C. (2017). The bubble-like shape of the heliosphere observed by Voyager and Cassini. Nat. Astron.1, 0115. doi:10.1038/s41550-017-0115
Drake, J., Opher, M., Swisdak, M., and Chamoun, J. N. (2010). A magnetic reconnection mechanism for the generation of anomalous cosmic rays. Astrophysical J.709, 963–974. doi:10.1088/0004-637x/709/2/963
Drake, J., Swisdak, M., and Opher, M. (2015). A model of the heliosphere with jets. Astrophysical J. Lett.808 (2), L44. doi:10.1088/2041-8205/808/2/l44
Elgart, S. (2021). Presentation by SMD director elgart, S robin (JSC-SK4). Houston, Texas: Space Radiation Element Scientist at NASA Johnson Space Center.
Fisk, L. A., Kozlovsky, B., and Ramaty, R. (1974). An interpretation of the observed oxygen and nitrogen enhancements in low-energy cosmic rays. Astrophysical J.190, L35. doi:10.1086/181498
Fleming, M., House, S., Hanson, V. S., Yu, L., Garbutt, J., McGee, R., et al. (2013). The mentoring competency assessment: Validation of a new instrument to evaluate skills of research mentors. Acad. Med.88 (7), 1002–1008. doi:10.1097/acm.0b013e318295e298
Florinski, V. , F., Ghanbari, K., Kleiman, J., Opher, M., Giacalone, J., Kota, J., et al. (2021). Magnetic trapping of galactic cosmic rays in the outer heliosheath. Astrophysical J.2023. in review.
Florinski, V., Decker, R. B., and le Roux, J. A. (2008). Pitch angle distributions of energetic particles near the heliospheric termination shock. J. Geophys. Res.113 (7), A07103. doi:10.1029/2007JA012859
Florinski, V., Ferreira, S. E. S., and Pogorelov, N. V. (2013). Galactic cosmic rays in the outer heliosphere: Theory and Models. Space Sci. Rev.176 (1), 147–163. doi:10.1007/s11214-011-9756-1
Florinski, V., Heerikhuisen, J., Niemiec, J., and Ernst, A. (2016). Theibexribbon and the pickup ion ring stability in the outer heliosheath. I. Theory and hybrid simulations. Astrophysical J.826, 197. doi:10.3847/0004-637x/826/2/197
Florinski, V. (2015). Magnetic flux tube interchange at the heliopause. Astrophysical J.813, 49. doi:10.1088/0004-637x/813/1/49
Florinski, V., and Pogorelov, N. V. (2009). FOUR-DIMENSIONAL transport of galactic cosmic rays in the outer heliosphere and heliosheath. Astrophysical J.701 (1), 642–651. doi:10.1088/0004-637x/701/1/642
Florinski, V., Zank, G. P., Heerikhuisen, J., Hu, Q., and Khazanov, I. (2010). Stability of a PICKUP ION ring-beam population in the outer heliosheath: Implications for theibexribbon. Astrophysical J.719 (2), 1097–1103. doi:10.1088/0004-637x/719/2/1097
Florinski, V., Zank, G. P., Jokipii, J. R., Stone, E. C., and Cummings, A. C. (2004). Do anomalous cosmic rays modify the termination shock?Astrophysical J.610, 1169–1181. doi:10.1086/421901
Florinski, V., Zank, G. P., and Pogorelov, N. V. (2003). Galactic cosmic ray transport in the global heliosphere. J. Geophys. Res.108 (6), 1228. doi:10.1029/2002JA009695
Fraternale, F., Pogorelov, N. V., and Burlaga, L. F. (2020). Signatures of intermittency and fine-scale turbulence in the very local interstellar medium. Astrophysical J. Lett.897, L28. doi:10.3847/2041-8213/ab9df5
Fraternale, F., Pogorelov, N. V., Richardson, J. D., and Tordella, D. (2019). Magnetic turbulence spectra and intermittency in the heliosheath and in the local interstellar medium. Astrophysical J.872, 40. doi:10.3847/1538-4357/aafd30
Fuselier, S. A., Galli, A., Richardson, J. D., Reisenfeld, D. B., Zirnstein, E. J., Heerikhuisen, J., et al. (2021). Energetic neutral atom fluxes from the heliosheath: Constraints from in situ measurements and models. Astrophysical J. Lett.915 (2), L26. doi:10.3847/2041-8213/ac0d5c
Giacalone, J., and Jokipii, J. R. (1999). The transport of cosmic rays across a turbulent magnetic field. Astrophysical J.520 (1), 204–214. doi:10.1086/307452
Giacalone, J., Nakanotani, M., Zank, G. P., Kota, J., Opher, M., and Richardson, J. D. (2021). Hybrid simulations of interstellar pickup protons accelerated at the solar-wind termination shock at multiple locations. Astrophysical J. Lett.911 (8), 27. doi:10.3847/1538-4357/abe93a
Giacalone, J. (2005). The efficient acceleration of thermal protons by perpendicular shocks. Astrophysical J.628, L37–L40. doi:10.1086/432510
Ginexi, E., Huang, G., Steketee, M., Tsakraklides, S., MacAllum, K., Bromberg, J., et al. (2017). Social network analysis of a scientist–practitioner research initiative established to facilitate science dissemination and implementation within states and communities. Res. Eval.26 (4), 316–325. doi:10.1093/reseval/rvx026
Gkioulidou, M., Opher, M., Kornbleuth, M., Dialynas, K., Dayeh, M. A., Zirnstein, E., et al. (2022). On the energization of pickup ions downstream of the heliospheric termination shock by comparing 0.52–55 keV observed energetic neutral atom spectra to ones inferred from proton hybrid simulations. Astrophysical J. Lett.931, 21. doi:10.3847/2041-8213/ac6beb
Grygorczuk, J., Czechowski, A., and Grzedzielski, S. (2014). Astrophysical J. Lett.789, L43. doi:10.1088/2041-8205/789/2/l43
Guimera, R., Uzzi, B., Spiro, J., and Amaral, L. A. N. (2005). Team assembly mechanisms determine collaboration network structure and team performance. Science308 (5722), 697–702. doi:10.1126/science.1106340
Guo, F., Jokipii, J. E., and Kota, J. (2010). Particle acceleration by collisionless shocks containing large-scale magnetic-field variations. Astrophysical J.725, 128–133. doi:10.1088/0004-637x/725/1/128
Guo, X., and Florinski, V. (2014). Galactic cosmic-ray modulation near the heliopause. Astrophysical J.793, 18. doi:10.1088/0004-637x/793/1/18
Guo, X., Florinski, V., and Wang, C. (2018). Effects of anomalous cosmic rays on the structure of the outer heliosphere. Astrophysical J.859, 157. doi:10.3847/1538-4357/aabf42
Gurnett, D. A., Kurth, W. S., Burlaga, L. F., and Ness, N. F. (2013). In situ observations of interstellar plasma with voyager 1. Science41 (6153), 1489–1492. doi:10.1126/science.1241681
Gurnett, D. A., and Kurth, W. S. (2019). Plasma densities near and beyond the heliopause from the Voyager 1 and 2 plasma wave instruments. Nat. Astron.3, 1024–1028. doi:10.1038/s41550-019-0918-5
Gurnett, D. A., Kurth, W. S., Stone, E. C., Cummings, A. C., Heikkila, B., Lal, N., et al. (2021). A foreshock model for interstellar shocks of solar origin: Voyager 1 and 2 observations. Astronomical J.161 (1), 11. doi:10.3847/1538-3881/abc337
Gurnett, D. A., Kurth, W. S., Stone, E. C., Cummings, A. C., Krimigis, S. M., Decker, R. B., et al. (2015). Precursors to interstellar shocks of solar origin. Astrophysical J.809, 121. doi:10.1088/0004-637x/809/2/121
Haverkorn, M., Brown, J. C., Gaensler, B. M., and McClure-Griffiths, N. M. (2008). The outer scale of turbulence in the magnetoionized galactic interstellar medium. Astrophysical J.680, 362–370. doi:10.1086/587165
Heerikhuisen, J., Pogorelov, N. V., Zank, G. P., Crew, G. B., Frisch, P. C., Funsten, H. O., et al. (2010). PICK-UP IONS IN the outer heliosheath: A possible mechanism for the interstellar boundary EXplorer ribbon. Astrophysical J.708, L126–L130. doi:10.1088/2041-8205/708/2/l126
Izmodenov, V., Malama, Y. G., and Ruderman, M. S. (2008). Modeling of the outer heliosphere with the realistic solar cycle. J. Adv. Space Res.41, 318–324. doi:10.1016/j.asr.2007.06.033
Izmodenov, V., Malama, Y., and Ruderman, M. S. (2005). Solar cycle influence on the interaction of the solar wind with Local Interstellar Cloud. Astronomy Astrophysics429, 1069–1080. doi:10.1051/0004-6361:20041348
Izmodenov, V. V., Alexashov, D. B., and Ruderman, M. S. (2014). Electron thermal conduction as A possible physical mechanism to make the inner heliosheath thinner. Astrophysical J. Lett.795 (1), 3. article id. L7. doi:10.1088/2041-8205/795/1/L7
Izmodenov, V. V., and Alexashov, D. B. (2015). Three-dimensional kinetic-mhd model of the global heliosphere with the heliopause-surface fitting. Astrophysical J. Suppl. Ser.220 (2), 14. article id 32. doi:10.1088/0067-0049/220/2/32
Jones, B. F., Wuchty, S., and Uzzi, B. (2008). Multi-university research teams: Shifting impact, geography, and stratification in science. Science322 (5905), 1259–1262. doi:10.1126/science.1158357
Jordan, J., and Sorell, M. (2019). Why reverse mentoring works and how to do it right. Harvard Business Review. Available at: https://hbr.org/2019/10/why-reverse-mentoring-works-and-how-to-do-it-right.
Keebler, T. B., Toth, G., Zieger, B., and Opher, M. (2022). MSWIM2D: Two-dimensional outer heliosphere solar wind modeling. Astrophysical J. Suppl.260, 43. doi:10.3847/1538-4365/ac67eb
Kim, T. K., Pogorelov, N. V., and Burlaga, L. F. (2017). Modeling shocks detected by voyager 1 in the local interstellar medium. Astrophysical J. Lett.843, L32. article id. doi:10.3847/2041-8213/aa7b2b
Kornbleuth, M., Opher, M., Baliukin, I., Dayeh, M. A., Zirnstein, E., Gkioulidou, M., et al. (2021b). Signature of a heliotail organized by the solar magnetic field and the role of nonideal processes in modeled IBEX ENA maps: A comparison of the BU and Moscow MHD models. Astrophysical J.921, 164. doi:10.3847/1538-4357/ac1e2a
Kornbleuth, M., Opher, M., Baliukin, I., Gkioulidou, M., Richardson, J., Zank, G., et al. (2021a). The development of a split-tail heliosphere and the role of non-ideal processes: A comparison of the BU and Moscow models. Astrophysical J.923 (2), 13pp–179. doi:10.3847/1538-4357/ac2fa6
Kota, J. (2010). Particle acceleration at near-perpendicular shocks: The role of field-line topology. Astrophysical J.23, 393.
Krimigis, S. M., Mitchell, D. G., Roelof, E. C., Hsieh, K. C., and McComas, D. J. (2009). Imaging the interaction of the heliosphere with the interstellar medium From saturn with cassini. Sci.326 (5955), 971.
le Roux, J. A., Webb, G. M., Shalchi, A., and Zank, G. P. (2010). A generalized nonlinear guiding center theory for the collisionless anomalous perpendicular diffusion of cosmic rays. Astrophysical J.716, 671. doi:10.1088/0004-637X/716/1/671
Lee, M. A., and Ip, W.-H. (1987). Hydromagnetic wave excitation by ionized interstellar hydrogen and helium in the solar wind. J. Geophys. Res.92 (10), 11041.
Malama, Y. G., Izmodenov, V. V., and Chalov, S. V. (2006). Modeling of the heliospheric interface: Multi-component nature of the heliospheric plasma. Astronomy Astrophysics445, 693–701. doi:10.1051/0004-6361:20053646
McComas, D. J., Allegrini, F., Bochsler, P., Bzowski, M., Christian, E. R., Crew, G. B., et al. (2009). Global observations of the interstellar interaction from the interstellar boundary explorer (IBEX). Science326, 959–962. doi:10.1126/science.1180906
McComas, D. J., Christian, E. R., Schwadron, N. A., Fox, N., Westlake, J., Allegrini, F., et al. (2018). Interstellar mapping and acceleration probe (IMAP): A new NASA mission. Space Sci. Rev.214 (8). article id 116. doi:10.1007/s11214-018-0550-1
McComas, D. J., Dayeh, M. A., Funsten, H. O., Livadiotis, G., and Schwadron, N. A. (2013). The heliotail revealed by theinterstellar boundary explorer. Astrophysical J.771, 77. doi:10.1088/0004-637x/771/2/77
McComas, D. J., and Schwadron, N. A. (2006). An explanation of the Voyager paradox: Particle acceleration at a blunt termination shock. Geophys. Res. Lett.33, 4. doi:10.1029/2005GL025437
Michael, A. T., Opher, M., Toth, G., Tenishev, V., and Drake, J. F. (2021). The impact of kinetic neutrals on the heliotail. Astrophysical J.906, 27.
Michael, A. T., Opher, M., Toth, M., Tenishev, V., and Borovikov, D. (2022). The solar wind with hydrogen ion exchange and large-scale dynamics (shield) code: A self-consistent kinetic–magnetohydrodynamic model of the outer heliosphere. Astrophysical J.924, 105. doi:10.3847/1538-4357/ac35eb
Möbius, E., Liu, K., Funsten, H., Gary, S. P., and Winske, D. (2013). Analytic model of theibexribbon with neutral solar wind based ION PICKUP beyond the heliopause. Astrophysical J.766, 129. doi:10.1088/0004-637x/766/2/129
Mostafavi, P., and Zank, G. P. (2018). The structure of shocks in the very local interstellar medium. Astrophysical J. Lett.854, L15. article id. L15. doi:10.3847/2041-8213/aaab54
Nakanotani, M., Zank, G. P., Adhikari, L., Zhao, L.-L., Giacalone, J., Opher, M., et al. (2021). Downwind solar wind and Pioneer 10 observations. Astrophysical J.901 (2), L23.
Nikoukar, R., Hill, M. E., Brown, L., Krimigis, S. M., Decker, R. B., Roelof, E. C., et al. (2022). On the energy dependence of galactic cosmic ray anisotropies in the very local interstellar medium. Astrophysical J.934, 41. doi:10.3847/1538-4357/ac6fe5
NRC (2015). Enhancing the effectiveness of team science. Washington, DC: The National Academies Press. doi:10.17226/19007
NRC (2013). Solar and space physics: A science for a technological society. Washington, DC: The National Academies Press. Chapter 4, page 92. doi:10.17226/13060
Opher, M., Bibi, F. A., Toth, G., Richardson, J. D., Izmodenov, V. V., and Gombosi, T. I. (2009). A strong, highly-tilted interstellar magnetic field near the Solar System. Nature462, 1036–1038. doi:10.1038/nature08567
Opher, M. (2012). BU summer school on plasma processes in space physics. Available at: http://people.bu.edu/mopher/PlasmaSummerSchool/Welcome.html.
Opher, M., Drake, J. F., Swisdak, M., Zieger, B., and Toth, G. (2017). The twist of the draped interstellar magnetic field ahead of the heliopause: A magnetic reconnection driven rotational discontinuity. Astrophysical J. Lett.839, L12. doi:10.3847/2041-8213/aa692f
Opher, M., Drake, J. F., Zank, G., Toth, G., Powell, E., Shelley, W., et al. (2021). A turbulent heliosheath driven by the Rayleigh–taylor instability. Astrophysical J.922, 181. doi:10.3847/1538-4357/ac2d2e
Opher, M., Drake, J. F., Zieger, B., and Gombosi, T. I. (2015). Magnetized jets driven by the Sun: The structure of the heliosphere revisited. Astrophysical J. Lett.800, L28. doi:10.1088/2041-8205/800/2/l28
Opher, M., Drake, J., Swisdak, M., Schoeffler, K. M., Richardson, J. D., Decker, R. B., et al. (2011). Astrophysical J.734, 71. doi:10.1088/0004-637x/734/1/71
Opher, M., Liewer, P. C., Gombosi, T. I., Manchester, W. B., DeZeeuw, D. L., Sokolov, I., et al. (2003). Probing the edge of the solar system: Formation of an unstable jet-sheet. Astrophysical J.591, L61–L65. doi:10.1086/376960
Opher, M., Loeb, A., Drake, J., and Gabor, T. (2020). A small and round heliosphere suggested by magnetohydrodynamic modelling of pick-up ions. Nat. Astron.4, 675–683. doi:10.1038/s41550-020-1036-0
Pesses, M. E., Eichler, D., and Jokipii, J. R. (1981). Cosmic ray drift, shock wave acceleration, and the anomalous component of cosmic rays. Astrophysical J.246, L85. doi:10.1086/183559
Pogorelov, N. V., Borovikov, S. N., Heerikhuisen, J., and Zhang, M. (2015). The heliotail. Astrophysical J. Lett.812 (1), 7. article id. L6. doi:10.1088/2041-8205/812/1/L6
Powell, E., Opher, M., Toth, G., Tenishev, V., Michael, A., Kornbleuth, M., et al. (2021). AGU fall meeting abstracts, SH15F-2075.
Richardson, J. D., Cummings, A., Stone, E. C., Burlaga, L., and Opher, M. (2021). Using magnetic flux conservation to determine heliosheath speeds. Astrophysical J. Lett.919, L28. doi:10.3847/2041-8213/ac27b1
Richardson, J. D., Kasper, J. C., Wang, C., Belcher, J. W., and Lazarus, A. J. (2008). Cool heliosheath plasma and deceleration of the upstream solar wind at the termination shock. Nature454 (7200), 63–66. doi:10.1038/nature07024
Scherer, K., and Ferreira, S. E. S. (2005). A heliospheric hybrid model: Hydrodynamic plasma flow and kinetic cosmic ray transport. Astrophys. Space Sci. Trans.1, 17–27. doi:10.5194/astra-1-17-2005
Schwadron, N. A., and McComas, D. J. (2013). Astrophysical J. Lett.778, L33. doi:10.1088/2041-8205/778/2/l33
Shalchi, A., Bieber, J. W., and Matthaeus, W. H. (2004). Nonlinear guiding center theory of perpendicular diffusion in dynamical turbulence. Astrophysical J.615, 805–812. doi:10.1086/424687
Shrestha, B. L., Zirnstein, E. J., Heerikhuisen, J., and Zank, G. P. (2021). Strength of the termination shock inferred from the global distributed energetic neutral atom flux from IBEX. The Astrophy. J. Supplement Series254 (2), 14.
Smith, C. W., Aggarwal, P., Argall, M. R., Burlaga, L. F., Bzowski, M., Cannon, B. E., et al. (2017). Observations of low-frequency magnetic waves due to newborn interstellar pickup ions using ACE, Ulysses, and Voyager data. J. Phys. Conf. Ser.900 (1), 012018. article id.
Smith, C. W., Isenberg, P. A., Matthaeus, W. H., and Richardson, J. D. (2006). Turbulent heating of the solar wind by newborn interstellar pickup protons. Astrophysical J.638, 508–517. doi:10.1086/498671
Swisdak, M., Drake, J. F., and Opher, M. (2013). Astrophysical J. Lett.774, L8. doi:10.1088/2041-8205/774/1/l8
Toth, G., Sokolov, I. V., Gombosi, T. I., Chesney, D. R., Clauer, C. R., and De Zeeuw, D. L. (2005). Space weather modeling framework: A new tool for the space science community. J. Geophys. Res. Space Phys.110 (12). CiteID A1222.
Toth, G., van der Holst, B., Sokolov, I. V., De Zeeuw, D. L., Gombosi, T. I., Fang, F., et al. (2012). Adaptive numerical algorithms in space weather modeling. J. Comput. Phys.231 (3), 870–903. doi:10.1016/j.jcp.2011.02.006
van der Holst, B., Sokolov, I. V., Meng, X., Jin, M., Manchester IV, W. B., Toth, G., et al. (2014). ALFVÉN WAVE SOLAR MODEL (AWSoM): Coronal heating. Astrophysical J.782, 81. doi:10.1088/0004-637x/782/2/81
Vertesi, J. A. (2020). Shaping science: Organizations, decisions, and culture on NASA’s teams. University of Chicago Press.
Wang, B., Zank, G. P., Shrestha, B., Kornbleuth, M., and Opher, M. (2023). Relating energetic ion spectra to energetic neutral atoms. Astrophysical J.944, 198. submitted. doi:10.3847/1538-4357/acb437
Wang, C., and Richardson, J. D. (2004). Interplanetary coronal mass ejections observed by Voyager 2 between 1 and 30 AU. J. Geophys. Res. Space Phys.109, A06104. Issue A6, CiteID A06104. doi:10.1029/2004ja010379
Washimi, H., Tanaka, T., and Zank, G. P. (2017). Time-varying heliospheric distance to the heliopause. Astrophysical J. Lett.846, L9. article id. doi:10.3847/2041-8213/aa8556
Washimi, H., Zank, G. P., Hu, Q., Tanaka, T., and Munakata, K. (2015). Astrophysical J.809, 16. doi:10.1088/0004-637x/809/1/16
Williams, L. L., and Zank, G. P. (1994). Effect of magnetic field geometry on the wave signature of the pickup of interstellar neutrals. J. Geophys. Res.99 (10), 19229. doi:10.1029/94ja01657
Zank, G. P., Adhikari, L., Hunana, P., Shiota, D., Bruno, R., and Telloni, D. (2017a). Theory and transport of nearly incompressible magnetohydrodynamic turbulence. Astrophysical J.835, 147. doi:10.3847/1538-4357/835/2/147
Zank, G. P., Du, S., and Hunana, P. (2017b). The origin of compressible magnetic turbulence in the very local interstellar medium. Astrophysical J.842, 114. doi:10.3847/1538-4357/aa7685
Zank, G. P., Heerikhuisen, J., Pogorelov, N. V., Burrows, R., and McComas, D. (2010). Astrophysical J.708, 1092–1106. doi:10.1088/0004-637x/708/2/1092
Zank, G. P., Hunana, P., Mostafavi, P., le Roux, J. A., Li, G., Webb, G. M., et al. (2015). Diffusive shock acceleration and reconnection acceleration processes. Astrophysical J.814–2. doi:10.1088/0004-637X/814/2/137
Zank, G. P. (1999). Interaction of the solar wind with the local interstellar medium: A theoretical perspective. Space Sci. Rev.89 (4), 413–688. doi:10.1023/a:1005155601277
Zank, G. P., Nakanotani, M., and Webb, G. M. (2019). Compressible and incompressible magnetic turbulence observed in the very local interstellar medium by voyager 1. Astrophysical J.887, 116. doi:10.3847/1538-4357/ab528c
Zank, G. P., Nakanotani, M., Zhao, L. L., Du, S., Adhikari, L., Che, H., et al. (2021). Flux ropes, turbulence, and collisionless perpendicular shock waves: High plasma beta case. Astrophysical J.913, 127. doi:10.3847/1538-4357/abf7c8
Zank, G. P., Pauls, D. H., and Williams, L. L. (1996a). Interaction of the solar wind with the local interstellar medium: A multifluid approach. J. Geophys. Res. (Space)101 (21), 639.
Zank, G. P., Pauls, H. L., Cairns, I. H., and Webb, G. M. (1996b). Interstellar pickup ions and quasi-perpendicular shocks: Implications for the termination shock and interplanetary shocks. J. Geophys. Res.101, 457–477. doi:10.1029/95ja02860
Zank, G. P. (2014). Transport processes in space physics and astrophysics. Lect. Notes Phys.877. doi:10.1007/978-1-4614-8480-6
Zhao, L.-L., Zank, G. P., and Adhikari, L., Astrophysical J., 879:012001, doi:10.1088/1742-6596/1332/1/0120012019).A nearly incompressible turbulence-driven solar wind model,
Zhao, L.-L., Zank, G. P., and Burlaga, L. F. (2020). Turbulence in the very local interstellar medium (VLISM). Astrophysical J.900, 166. doi:10.3847/1538-4357/ababa2, no.
Zieger, B., Opher, M., Toth, G., and Florisnki, V. (2020). Dispersive fast magnetosonic waves and shock-driven compressible turbulence in the inner heliosheath. J. Geophys. Res.125 (10). article id. E28393.
Zieger, B., Opher, M., Toth, G., Decker, R., and Richardson, J. (2015). Constraining the pickup ion abundance and temperature through the multifluid reconstruction of the Voyager 2 termination shock crossing. J. Geophys. Res.120, 7130–7153. doi:10.1002/2015ja021437
Zirnstein, E. J., McComas, D. J., Kumar, R., Elliott, H., Szalay, J., Olkin, C., et al. (2018). In situ observations of preferential pickup ion heating at an interplanetary shock. PRL121, 075102. doi:10.1103/physrevlett.121.075102
Keywords: heliosphere, space physics, solar wind, interstellar medium, magnetic field, galactic cosmic ray (GCR)
Citation: Opher M, Richardson J, Zank G, Florinski V, Giacalone J, Sokół JM, Toth G, Buxner S, Kornbleuth M, Gkioulidou M, Nikoukar R, Van der Holst B, Turner D, Gross N, Drake J, Swisdak M, Dialynas K, Dayeh M, Chen Y, Zieger B, Powell E, Onubogu C, Ma X, Bair E, Elliott H, Galli A, Zhao L, Adhikari L, Nakanotani M, Hill ME, Mostafavi P, Du S, Guo F, Reisenfeld D, Fuselier S, Izmodenov V, Baliukin I, Cummings A, Miller J, Wang B, Ghanbari K, Kota J, Loeb A, Burgess J, Hokanson SC, Morrow C, Hong A and Boldon A (2023) Solar wind with Hydrogen Ion charge Exchange and Large-Scale Dynamics (SHIELD) DRIVE Science Center. Front. Astron. Space Sci. 10:1143909. doi: 10.3389/fspas.2023.1143909
Received: 13 January 2023; Accepted: 10 May 2023;
Published: 15 June 2023.
Edited by:
Joseph E. Borovsky, Space Science Institute, United StatesReviewed by:
Jingnan Guo, University of Science and Technology of China, ChinaCopyright © 2023 Opher, Richardson, Zank, Florinski, Giacalone, Sokół, Toth, Buxner, Kornbleuth, Gkioulidou, Nikoukar, Van der Holst, Turner, Gross, Drake, Swisdak, Dialynas, Dayeh, Chen, Zieger, Powell, Onubogu, Ma, Bair, Elliott, Galli, Zhao, Adhikari, Nakanotani, Hill, Mostafavi, Du, Guo, Reisenfeld, Fuselier, Izmodenov, Baliukin, Cummings, Miller, Wang, Ghanbari, Kota, Loeb, Burgess, Hokanson, Morrow, Hong and Boldon. This is an open-access article distributed under the terms of the Creative Commons Attribution License (CC BY). The use, distribution or reproduction in other forums is permitted, provided the original author(s) and the copyright owner(s) are credited and that the original publication in this journal is cited, in accordance with accepted academic practice. No use, distribution or reproduction is permitted which does not comply with these terms.
*Correspondence: Merav Opher, mopher@bu.edu
Disclaimer: All claims expressed in this article are solely those of the authors and do not necessarily represent those of their affiliated organizations, or those of the publisher, the editors and the reviewers. Any product that may be evaluated in this article or claim that may be made by its manufacturer is not guaranteed or endorsed by the publisher.
Research integrity at Frontiers
Learn more about the work of our research integrity team to safeguard the quality of each article we publish.