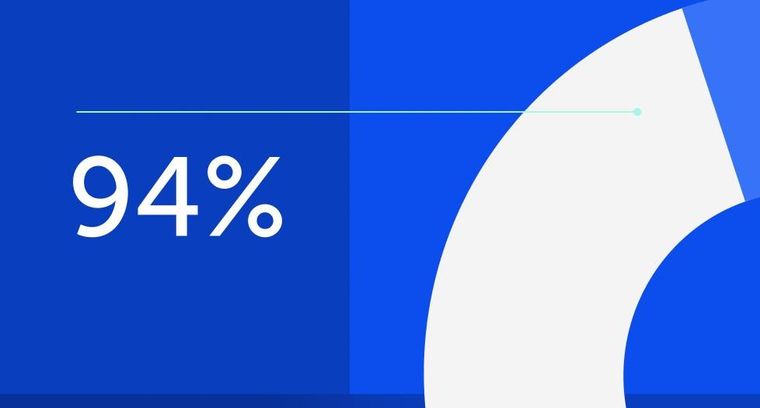
94% of researchers rate our articles as excellent or good
Learn more about the work of our research integrity team to safeguard the quality of each article we publish.
Find out more
PERSPECTIVE article
Front. Astron. Space Sci., 13 February 2023
Sec. Space Physics
Volume 10 - 2023 | https://doi.org/10.3389/fspas.2023.1139230
This article is part of the Research TopicThe Future of Space Physics 2022View all 66 articles
Climate change is characterized by global surface warming associated with the increase of greenhouse gas population since the start of the industrial era. Growing evidence shows that the upper atmosphere is experiencing appreciable cooling over the last several decades. The seminal modeling study by Roble and Dickinson (1989) suggested potential effects of increased greenhouse gases on the ionosphere and thermosphere cooling which appear consistent with some observations. However, several outstanding issues remain regarding the role of CO2, other important contributors, and impacts of the cooling trend in the ionosphere and thermosphere: for example, (1) what is the regional variability of the trends? (2) the very strong ionospheric cooling observed by multiple incoherent scatter radars that does not fit with the prevailing theory based on the argument of anthropogenic greenhouse gas increases, why? (3) what is the effect of secular changes in Earth’s main magnetic field? Is it visible now in the ionospheric data and can it explain some of the regional variability in the observed ionospheric trends? (4) what is the impact of long-term cooling in the thermosphere on operational systems? (5) what are the appropriate strategic plans to ensure the long-term monitoring of the critical space climate?
Growing evidence shows that the upper atmosphere has been experiencing appreciable cooling over the last several decades (e.g., Laštovička, 2017, see Figure 1A). This has been connected to the increase in greenhouse gas concentrations since the start of the industrial age, which drives global warming near the Earth’s surface, but causes global cooling in the middle and upper atmosphere (Figure 1B, after Roble and Dickinson, 1989). Greenhouse gases act as a cooling agent in the thermosphere. Infrared emissions by CO2 at 15 μm and NO at 5.3 μm transfers thermal energy up into the thinner and thinner atmosphere without being trapped as is the case when emitted in the dense lower atmosphere, and therefore these gases provide efficient cooling in the thermosphere. However, the upper atmosphere, especially the ionosphere, is also very responsive to a wide range of other forcings, both from above, including (long-term) variability in solar irradiation and geomagnetic disturbances, and from below, including various wave activities, violent surface activities (e.g., volcanic eruptions) and gradual Earth magnetic field changes. Detecting, analyzing, and modeling the relatively weak signals over the long term are non-trivial tasks. In the following, we discuss several challenges the community needs to address.
FIGURE 1. Ionospheric and thermospheric long-term trends: (A) observations in electron density (solid line on the left) and neutral temperature (solid on the right), after Laštovička et al. (2006); (B) incoherent scatter radar ion temperature observations at Millstone Hill (red), Sondrestrom (black), and Poker Flat (blue), after Zhang et al. (2016); (C) simulated thermospheric temperature changes due to doubling CO2 mix ratio at ∼ 60 km altitudes (the left solid curve; the right dashed curve is for CO2 halved), after Roble and Dickinson (1989).
Satellite drag data indicated that the global average thermospheric density is reducing at a rate of 2–3% per decade at 400 km between the 1960s and 2000s (e.g. Emmert, 2004; 2015). The CO2 trend at 105–110 km was 7–8% per decade from 2004 to 2012 as reported by Yue et al. (2015); Rezac et al. (2018) using TIMED SABER data. This rate is noticeably larger than a General Circulation Model (GCM) simulation, and the corresponding simulated density trends appear to be smaller than indicated by observations (Emmert et al., 2008). The long-time series of CO2 measurements in the thermosphere are currently not available to provide adequate trend analysis, and the modeling results have not been fully validated.
Dramatic differences were found in the trends determined from incoherent scatter radar (ISR)-based ion temperature (Ti) at multiple locations (Zhang et al., 2011; Oliver et al., 2013; Zhang and Holt, 2013; Ogawa et al., 2014; Zhang et al., 2016) and trends inferred from model simulations. Figure 1C provides an analysis of the ISR Ti trends measured at Millstone Hill and elsewhere where the trends were centered around ∼15K/decade at ∼250 km altitudes during the day, equivalent to 75K in 50 years. GCM simulations conducted by, e.g., Roble and Dickinson (1989), Qian et al. (2011), and Solomon et al. (2018) showed consistently that global mean exosphere temperature will drop at 2–5K/decade due to the increasing CO2 mixing ratio. These results indicated that the observed strong ionospheric cooling could be caused by important additional sources, beyond the greenhouse effect.
Gravity wave (GW) activity has important direct influences on the ionospheric and thermospheric dynamics, and also GW vertical transport of momentum and energy associated with wave dissipation and diffusive mixing through the mesosphere may modify the thermospheric thermal status. Oliver et al. (2013) indicated that GW activity at ionospheric altitudes could be increasing at Millstone Hill. They further speculated this increase could be related to the surface climate change affecting ocean-atmospheric interaction. It is not clear how general this process is as commented by Laštovička (2015). Limited observations on the ground and from space showed that GW trends in the middle atmosphere are very regional and unstable (e.g., Hoffmann et al., 2011; Jacobi, 2014; Liu et al., 2017). To provide direct physical insights whole atmospheric models that can properly resolve GWs may be used to examine the long-term GW trends at different layers of the atmosphere and influences on the ionosphere and thermosphere. Cnossen (2020) reported an initial effort using the Whole Atmosphere Community Climate Model eXtension (WACCM-X), a comprehensive coupled general circulation model, however, with parameterized GWs. High-resolution models explicitly resolving GWs (Becker and Vadas, 2020; Becker et al., 2022), on the other hand, are mechanistic and cannot currently provide a comprehensive view of the whole Earth system, particularly, when the ionosphere is considered.
The solar activity variability over solar cycles is large, however, a general declining activity since the 1950s (Solar Cycle 19) seems evident. Geomagnetic disturbances are caused by interplanetary coronal mass ejections (ICMEs), which are strongly correlated with solar activity, and high-speed stream (HSS), which is originated from coronal holes, less dependent on solar activity, but appear periodically on the visible solar disk. These disturbances have substantial influences on the upper atmosphere (Mikhailov and Perrone, 2016), including the CO2 mixing ratio (Liu et al., 2021). Most trend analyses attempted to remove (solar and) geomagnetic activity effects via regression with certain (solar and) geomagnetic indices or by using quiet-time data or monthly averages. While the ion temperature Ti and neutral densities are strongly positively correlated with enhanced magnetic disturbances, the peak electron density in the F2 layer, NmF2, has a very complicated relationship with these indices and therefore it is possible that some trend analysis is, to some degree, contaminated by geomagnetic disturbances. An improved understanding of these effects and sophisticated techniques (e.g., using machine learning algorithms) to deal with this challenge are needed.
The strength of the geomagnetic field has been decreasing at an average rate of 16 nT per year over the past 180 years (Gillet et al., 2013), accompanied by movement of the magnetic poles and magnetic equator (Livermore et al., 2020). Both types of changes are potentially important drivers of long-term change in the upper atmosphere, especially in regions where the magnetic equator and magnetic poles have shifted their positions considerably. These drivers have been recognized in various simulation studies where secular changes of Earth’s main magnetic field were considered in addition to long-term trends in trace gas (including CO2) emissions (e.g., Yue et al., 2008; 2018; Cnossen, 2020; Qian et al., 2021). At Millstone Hill, the magnetic apex latitude has decreased from 57° to 52° and the magnetic dip angle from 71° to 67° between 1950 and 2020 (Figure 2), and less heating related to high latitude magnetosphere-ionosphere coupling is available. The main field change can also modify the ionospheric dynamics including the Sq pattern and the location of equatorial electrojets (Cnossen and Richmond, 2013; Soares et al., 2020; Elias et al., 2021). Since the effects of magnetic field changes vary strongly with location, further studies with different types of observations from diversified locations and model-data comparisons are highly needed to clarify the relative contribution of main field changes to long-term trends.
FIGURE 2. Long-term variations of the main magnetic field at Millstone Hill based on the IGRF model.
Consequences of some long-term changes in the upper atmosphere could appear subtle over short time scales but the accumulated effect can be significant in the long term. For example, incoherent scatter radar observations at Millstone Hill and elsewhere suggested that the ion temperature has decreased by 100 + K above 300 km since the space age. Neutral density at 400 km has reduced by 10–25% for the same time period. Although the ionosphere and thermosphere are subject to larger changes between day and night and across a solar cycle, the increased cooling in the background have gradually become a permanent feature. As the increase in CO2 concentration will continue in this century and the CO2 mixing ratio will be likely close to being doubled from its pre-industrial levels by the end of this century, really substantial ionosphere and thermosphere changes can be anticipated.
Potential risks of space debris surviving in orbitals harmful to spacecraft and humans in space become larger due to global cooling and the associated drop in upper atmosphere air density, as this reduces the drag on space debris, increasing its lifetime (Lewis et al., 2011). Brown et al. (2021) indicated that if the 1.5°C warming limit target in the surface atmosphere is met, objects in Low Earth Orbit (LEO) will by 2050 have orbital lifetimes around 30% longer than comparable objects from the year 2000. Radio propagation and communication systems use the ionosphere as a reflection or refraction or transmission medium and they depend on the ionospheric plasma density. For example, sea level monitoring using the altimeter to sense radio wave propagation needs calibration with proper TEC climatology for earlier observations with the single frequency system (Scharroo and Smith, 2010). It is clear that future designs should take into consideration the propagation condition changes (Elias et al., 2017) due to changes in the height and number density of the background ionosphere and in the main magnetic field.
The scientific community needs to maintain and develop the important capability to monitor space climate over the long-term. Not only long-term data availability but also stability and cross-calibration of the observing system are important aspects.
Various satellite missions provide important observations of neutral density, CO2 mixing ratio, thermospheric infrared cooling power, GW activity, and topside plasma density (e.g. Emmert, 2015; Yue et al., 2015; Liu et al., 2017; Mlynczak et al., 2018; Cai et al., 2019). The longevity of these missions can be up to 1–2 decades.
Long-term monitoring is relatively easier to achieve using ground-based observational systems, complementary to mission-based in situ space observations. These systems are characterized by a clear separation between temporal and spatial ambiguity as well as consistency and stability of observing environment and maintenance. Ionosonde records can span easily over 50 years; continuous ISR data at Millstone Hill available for research started in the 1960s. They remain critically important tools for space climate monitoring.
A list of important ground-based techniques that can enable atmospheric long-term monitoring include magnetometers, ionosondes, incoherent scatter radars, Fabry-Perot Interferometers, All-sky imagers, SuperDARN HF radars, and LIDARs. The communities use them to understand and predict short-term space weather and furthermore to establish climatology and detect climate changes. It is essential that our space weather and space climate monitoring systems can detect a comprehensive set of physical characteristics, from neutral and plasma state parameters (densities and temperatures), to their dynamical behaviors, and to geomagnetic main field and perturbations; it is important also that they are sensitive to spatial variability and time variation in all scales, from short to long-term (Pulkkinen, 2007; Kerridge, 2019).
To maintain efficient long-term investments in monitoring the space climate, proper observational configuration and networks to measure key physical parameters appear a practical approach. It is necessary to balance the needs for building cutting edging new instruments and ensuring the longevity of existing key facilities.
The original contributions presented in the study are included in the article/supplementary material, further inquiries can be directed to the corresponding author.
S-RZ provided the original idea and wrote the first draft, and finalized the article. IC provided comments and edits. Other co-authors (JL, AE, XY, CJ, WW, and LG) read the manuscript and provided comments for improvement.
Research at MIT Haystack Observatory was partially supported by the US NSF award AGS-1952737. S-RZ acknowledges also supports from US NSF AGS-2033787, AGS-2149698, US NASA 80NSSC21K1315 and 80NSSC21K1310. IC was supported by a Natural Environment Research Council (NERC) Independent Research Fellowship (NE/R015651/1). JL acknowledges support by the Czech Science Foundation under grant 21-03295S. WW and LQ acknowledge support by the US NASA 80NSSC21K1315. XY acknowledges the Project of Stable Support for Youth Team in Basic Research Field, Chinese Academy of Sciences (CAS) (YSBR-018), and the International Partnership Program of CAS (Grant 183311KYSB20200003). CJ acknowledges support by the German Research Foundation (DFG) through grant #JA 836/43-1.
ISSI (International Space Science Institute, Bern) and ISSI-Beijing have supported the international team research on “Climate Change in the Upper Atmosphere” (2016 and 2017) led by S-RZ; IC and S-RZ thank also ISSI support for the international team on “Impacts of Climate Change on the Middle and Upper Atmosphere and Atmospheric Drag of Space Objects” (2022 and 2023) led by J. A. Añel and IC.
The authors declare that the research was conducted in the absence of any commercial or financial relationships that could be construed as a potential conflict of interest.
All claims expressed in this article are solely those of the authors and do not necessarily represent those of their affiliated organizations, or those of the publisher, the editors and the reviewers. Any product that may be evaluated in this article, or claim that may be made by its manufacturer, is not guaranteed or endorsed by the publisher.
Becker, E., Vadas, S. L., Bossert, K., Harvey, V. L., Zülicke, C., and Hoffmann, L. (2022). A high-resolution whole-atmosphere model with resolved gravity waves and specified large-scale dynamics in the troposphere and stratosphere. J. Geophys. Res. Atmos. 127, e2021JD035018. doi:10.1029/2021jd035018
Becker, E., and Vadas, S. L. (2020). Explicit global simulation of gravity waves in the thermosphere. J. Geophys. Res. Space Phys. 125, e2020JA028034. doi:10.1029/2020ja028034
Brown, M. K., Lewis, H. G., Kavanagh, A. J., and Cnossen, I. (2021). Future decreases in thermospheric neutral density in low Earth orbit due to carbon dioxide emissions. J. Geophys. Res. Atmos. 126, e2021JD034589. doi:10.1029/2021jd034589
Cai, Y., Yue, X., Wang, W., Zhang, S.-R., Liu, L., Liu, H., et al. (2019). Long-term trend of topside ionospheric electron density derived from DMSP data during 1995-2017. J. Geophys. Res. Space Phys. 124, 10708–10727. 2019JA027522. doi:10.1029/2019ja027522
Cnossen, I. (2020). Analysis and attribution of climate change in the upper atmosphere from 1950 to 2015 simulated by waccm-x. J. Geophys. Res. Space Phys. 125, e2020JA028623. doi:10.1029/2020ja028623
Cnossen, I., and Richmond, A. D. (2013). Changes in the Earth’s magnetic field over the past century: Effects on the ionosphere-thermosphere system and solar quiet (sq) magnetic variation. J. Geophys. Res. Space Phys. 118, 849–858. doi:10.1029/2012ja018447
Elias, A. G., de Haro Barbas, B. F., Zossi, B. S., Medina, F. D., Fagre, M., and Venchiarutti, J. V. (2021). Review of long-term trends in the equatorial ionosphere due the geomagnetic field secular variations and its relevance to space weather. Atmosphere 13, 40. doi:10.3390/atmos13010040
Elias, A. G., Zossi, B. S., Yiğit, E., Saavedra, Z., and de Haro Barbas, B. F. (2017). Earth’s magnetic field effect on muf calculation and consequences for hmf2 trend estimates. J. Atmos. Solar-Terrestrial Phys. 163, 114–119. doi:10.1016/j.jastp.2017.03.004
Emmert, J., Picone, J., and Meier, R. (2008). Thermospheric global average density trends, 1967–2007, derived from orbits of 5000 near-Earth objects. Geophys. Res. Lett. 35, L05101. doi:10.1029/2007gl032809
Emmert, J. T. (2015). Altitude and solar activity dependence of 1967–2005 thermospheric density trends derived from orbital drag. J. Geophys. Res. Space Phys. 120, 2940–2950. doi:10.1002/2015ja021047
Emmert, J. T., Picone, J. M., Lean, J. L., and Knowles, S. H. (2004). Global change in the thermosphere: Compelling evidence of a secular decrease in density. J. Geophys. Res. 109, A02301. doi:10.1029/2003ja010176
Gillet, N., Jault, D., Finlay, C., and Olsen, N. (2013). Stochastic modeling of the Earth’s magnetic field: Inversion for covariances over the observatory era. Geochem. Geophys. Geosystems 14, 766–786. doi:10.1002/ggge.20041
Hoffmann, P., Rapp, M., Singer, W., and Keuer, D. (2011). Trends of mesospheric gravity waves at northern middle latitudes during summer. J. Geophys. Res. Atmos. 116, D00P08. doi:10.1029/2011JD015717
Jacobi, C. (2014). Long-term trends and decadal variability of upper mesosphere/lower thermosphere gravity waves at midlatitudes. J. Atmos. Solar-Terrestrial Phys. 118, 90–95. doi:10.1016/j.jastp.2013.05.009
Kerridge, D. (2019). “Objectives of geomagnetic and aeronomy studies,” in Geomagnetism, aeronomy and space weather: A journey from the Earth’s core to the sun (Cambridge University Press), 4.
Laštovička, J. (2017). A review of recent progress in trends in the upper atmosphere. J. Atmos. Solar-Terrestrial Phys. 163, 2–13. doi:10.1016/j.jastp.2017.03.009
Laštovička, J., Akmaev, R. A., Beig, G., Bremer, J., and Emmert, J. T. (2006). Atmosphere: Global change in the upper atmosphere. Science 314, 1253–1254. doi:10.1126/science.1135134
Laštovička, J. (2015). Comment on “Long-term trends in thermospheric neutral temperatures and density above Millstone Hill” by W. L. Oliver et al. J. Geophys. Res. Space Phys. 120, 2347–2349. doi:10.1002/2014ja020864
Lewis, H. G., Saunders, A., Swinerd, G., and Newland, R. J. (2011). Effect of thermospheric contraction on remediation of the near-Earth space debris environment. J. Geophys. Res. Space Phys. 116. doi:10.1029/2011JA016482
Liu, H., Tao, C., Jin, H., and Abe, T. (2021). Geomagnetic activity effects on co2-driven trend in the thermosphere and ionosphere: Ideal model experiments with gaia. J. Geophys. Res. Space Phys. 126, e2020JA028607. doi:10.1029/2020JA028607
Liu, X., Yue, J., Xu, J., Garcia, R. R., Russell, J. M., Mlynczak, M., et al. (2017). Variations of global gravity waves derived from 14 years of saber temperature observations. J. Geophys. Res. Atmos. 122, 6231–6249. doi:10.1002/2017JD026604
Livermore, P. W., Finlay, C. C., and Bayliff, M. (2020). Recent north magnetic pole acceleration towards siberia caused by flux lobe elongation. Nat. Geosci. 13, 387–391. doi:10.1038/s41561-020-0570-9
Mikhailov, A. V., and Perrone, L. (2016). Geomagnetic control of the midlatitude daytime foF1 and foF2 long-term variations: Physical interpretation using European observations. J. Geophys. Res. Space Phys. 121, 7193–7203. doi:10.1002/2016ja022716
Mlynczak, M. G., Hunt, L. A., Russell, J. M., and Marshall, B. T. (2018). Thermosphere climate indexes: Percentile ranges and adjectival descriptors. J. Atmos. Solar-Terrestrial Phys. 174, 28–31. doi:10.1016/j.jastp.2018.04.004
Ogawa, Y., Motoba, T., Buchert, S. C., Häggström, I., and Nozawa, S. (2014). Upper atmosphere cooling over the past 33 years. Geophyical Res. Lett. 41, 5629–5635. doi:10.1002/2014gl060591
Oliver, W. L., Zhang, S.-R., and Goncharenko, L. P. (2013). Is thermospheric global cooling caused by gravity waves? J. Geophys. Res. Space Phys. 118, 3898–3908. doi:10.1002/jgra.50370
Pulkkinen, T. (2007). Space weather: Terrestrial perspective. Living Rev. Sol. Phys. 4, 1–60. doi:10.12942/lrsp-2007-1
Qian, L., Laštovička, J., Roble, R. G., and Solomon, S. C. (2011). Progress in observations and simulations of global change in the upper atmosphere. J. Geophys. Res. 116, A00H03. doi:10.1029/2010ja016317
Qian, L., McInerney, J. M., Solomon, S. S., Liu, H., and Burns, A. G. (2021). Climate changes in the upper atmosphere: Contributions by the changing greenhouse gas concentrations and Earth’s magnetic field from the 1960s to 2010s. J. Geophys. Res. Space Phys. 126, e2020JA029067. doi:10.1029/2020ja029067
Rezac, L., Yue, J., Yongxiao, J., Russell, J. M., Garcia, R., López-Puertas, M., et al. (2018). On long-term saber co2 trends and effects due to nonuniform space and time sampling. J. Geophys. Res. Space Phys. 123, 7958–7967. doi:10.1029/2018JA025892
Roble, R. G., and Dickinson, R. E. (1989). How will changes in carbon dioxide and methane modify the mean structure if the nesosphere and thermosphere? Geophyical Res. Lett. 16, 1441–1444. doi:10.1029/GL016i012p01441
Scharroo, R., and Smith, W. H. F. (2010). A global positioning system–based climatology for the total electron content in the ionosphere. J. Geophys. Res. Space Phys. 115. doi:10.1029/2009JA014719
Soares, G., Yamazaki, Y., Cnossen, I., Matzka, J., Pinheiro, K., Morschhauser, A., et al. (2020). Evolution of the geomagnetic daily variation at tatuoca, Brazil, from 1957 to 2019: A transition from sq to eej. J. Geophys. Res. Space Phys. 125, e2020JA028109. doi:10.1029/2020ja028109
Solomon, S. C., Liu, H.-L., Marsh, D. R., McInerney, J. M., Qian, L., and Vitt, F. M. (2018). Whole atmosphere simulation of anthropogenic climate change. Geophyical Res. Lett. 45, 1567–1576. doi:10.1002/2017gl076950
Yue, J., Russell, J., Jian, Y., Rezac, L., Garcia, R., López-Puertas, M., et al. (2015). Increasing carbon dioxide concentration in the upper atmosphere observed by saber. Geophys. Res. Lett. 42, 7194–7199. doi:10.1002/2015GL064696
Yue, X., Hu, L., Wei, Y., Wan, W., and Ning, B. (2018). Ionospheric trend over wuhan during 1947-2017: Comparison between simulation and observation. J. Geophys. Res. Space Phys. 123, 1396–1409. doi:10.1002/2017ja024675
Yue, X., Liu, L., Wan, W., Wei, Y., and Ren, Z. (2008). Modeling the effects of secular variation of geomagnetic field orientation on the ionospheric long term trend over the past century. J. Geophys. Res. Space Phys. 113. doi:10.1029/2007ja012995
Zhang, S.-R., Holt, J. M., Erickson, P. J., Goncharenko, L. P., Nicolls, M. J., McCready, M., et al. (2016). Ionospheric ion temperature climate and upper atmospheric long-term cooling. J. Geophys. Res. Space Phys. 121, 8951–8968. doi:10.1002/2016ja022971
Zhang, S.-R., Holt, J. M., and Kurdzo, J. (2011). Millstone Hill ISR observations of upper atmospheric long-term changes: Height dependency. J. Geophys. Res. 116, A00H05. doi:10.1029/2010ja016414
Keywords: long-term trends, climate, ionosphere, thermosphere, geospace, observation
Citation: Zhang S-R, Cnossen I, Laštovička J, Elias AG, Yue X, Jacobi C, Yue J, Wang W, Qian L and Goncharenko L (2023) Long-term geospace climate monitoring. Front. Astron. Space Sci. 10:1139230. doi: 10.3389/fspas.2023.1139230
Received: 06 January 2023; Accepted: 30 January 2023;
Published: 13 February 2023.
Edited by:
Benoit Lavraud, UMR5804 Laboratoire d’astrophysique de Bordeaux (LAB), FranceReviewed by:
Veronika Barta, Institute of Earth Physics and Space Science (EPSS, ELKH), HungaryCopyright © 2023 Zhang, Cnossen, Laštovička, Elias, Yue, Jacobi, Yue, Wang, Qian and Goncharenko. This is an open-access article distributed under the terms of the Creative Commons Attribution License (CC BY). The use, distribution or reproduction in other forums is permitted, provided the original author(s) and the copyright owner(s) are credited and that the original publication in this journal is cited, in accordance with accepted academic practice. No use, distribution or reproduction is permitted which does not comply with these terms.
*Correspondence: Shun-Rong Zhang, c2h1bnJvbmdAbWl0LmVkdQ==
Disclaimer: All claims expressed in this article are solely those of the authors and do not necessarily represent those of their affiliated organizations, or those of the publisher, the editors and the reviewers. Any product that may be evaluated in this article or claim that may be made by its manufacturer is not guaranteed or endorsed by the publisher.
Research integrity at Frontiers
Learn more about the work of our research integrity team to safeguard the quality of each article we publish.