- 1Centro de Química Estrutural, Departamento de Engenharia Química, Instituto Superior Técnico, Universidade de Lisboa, Lisboa, Portugal
- 2Institute for Bioengineering and Biosciences, Instituto Superior Técnico, Universidade de Lisboa, Lisboa, Portugal
- 3School of Physical Sciences, The Open University, Milton Keynes, United Kingdom
- 4Associate Laboratory i4HB—Institute for Health and Bioeconomy, Instituto Superior Técnico, Universidade de Lisboa, Lisboa, Portugal
- 5Spin.Works, Lisboa, Portugal
- 6CFisUC, Departamento de Física, Universidade de Coimbra, Coimbra, Portugal
- 7IMCCE, Observatoire de Paris, PSL Université, Paris, France
Phobos, a satellite of Mars, was successfully studied by flyby, orbiter, and landing missions to the Red Planet, but several questions remain about its origin, composition, and relationship to Mars. It is suggested that Phobos is either a captured body from the asteroid belt or the outer Solar System (capture scenario), or a consequence of re-accreted ejecta from Mars (in situ formation/giant impact). So far, Phobos has been characterized by its two spectral units - blue and red - with different compositional restrains. The red unit represents most of the surface, while the blue unit is focused on the Stickney crater and surroundings. In the absence of samples returned from this satellite, simulant regolith must be studied to infer various proprieties, and complement in situ studies. To date, there are three simulants of this satellite: Phobos-1C, Phobos Captured Asteroid-1 (PCA-1), and Phobos Giant Impact-1 (PGI-1). Since Phobos may have a Mars-like composition, terrestrial analogues of Mars should also be analysed. The data retrieved from the various assays performed with these planetary field analogues may be used as a database to complement future space missions to Phobos, but, ultimately, the composition of Phobos will have to be analysed by a sample-return mission.
1 Introduction
The formation and evolution of the Solar System began around 4.56 billion years ago, due to the gravitational collapse of a molecular cloud. Rocky planets such as Mercury, Venus, Earth, and Mars formed in distinct stages (Chambers, 2004). Mars appears to have partly similar characteristics to Earth, however, its satellite system comprises two small moons (Phobos and Deimos). Phobos is the biggest Martian Moon, and it orbits closer to the primary body than any other Moon in the Solar System.
Many questions about Phobos remain unanswered, including: What is the composition and origin of Phobos? What is the relationship between Phobos and Deimos? What is the internal structure of these moons and the mineralogy of the surface? Will the composition of Phobos help to tell the story of the Martian past? Are there biosignatures on the surface ofPhobos? The origin of Phobos remains unresolved (Craddock, 2011; Rosenblatt, 2011; Canup and Salmon, 2018), and may provide insights into the formation of the terrestrial planets. The above questions ultimately rely on the acquisition of regolith specimens, and state-of-the-art laboratory equipment, with future sample return missions presenting growing interest. The preparation and analysis of specimens in terrestrial laboratories allows the optimization for a particular study, which can sometimes be impossible to do in situ. Terrestrial analyses can also be replicated many times, and verified by diverse and independent analytical methods, that can be unaffordable or impossible for remote or robotic techniques (Murchie et al., 2014). In the meantime, and in the absence of samples of regolith returned from Phobos to the terrestrial laboratories, analogous or simulant regolith are fundamental to prepare future sample return missions.
Finally, the study of Phobos may also provide an opportunity for future human exploration of the Mars system (Murchie et al., 2014), as the exploration of Phobos is not only driven by planetary science or the quest to understand its origin. Phobos also holds strategic importance in developing a forward base for human exploration on Mars (Muscatello et al., 2012). With the increasing interest in taking humans to Mars, scientists and engineers are also considering using Phobos as a staging area for a trip to the Red Planet, or even a teleoperation centre for robots on the surface of Mars, to avoid long-latency (Nallapu et al., 2020). Ultimately, Phobos ensures the continuity of the exploration program to Mars.
In this review, the different reasons why it is relevant to study Phobos will be covered. The origin of the Martian moons will be covered in Section 2 while Section 3 describes how the Mars-Phobos system may inform about the geological history of Mars. Section 4 will cover the space exploration of Phobos, including past, present, and future space missions and how these will inform on the characterization of Phobos. Finally, Section 5 will describe the fundamental role and mineral composition of different regolith simulants of Phobos.
2 Origin of the Martian moons
After years of exploring Mars, the origin and evolution of Phobos and Deimos remain unknown. Understanding their origin may provide constraints on the formation and early dynamical environment of Mars (see, e.g., Ramsley and Head, 2021). To explain the origin of the Martian Moon system, several hypothesis have been presented: the recycling hypothesis, the hypothesis that Phobos and Deimos were once a single large Moon that later split, the hypothesis in which the moons are captured small bodies from the asteroid belt or outer Solar System (capture scenario), or the hypothesis in which they are reaccreted impact ejecta from Mars (in situ formation or giant impact scenario).
Hesselbrock and Minton (2017) proposed the recycling hypothesis, in which Phobos and Deimos would have accreted from a ring of debris, with the orbit of Phobos slowly decaying and eventually split up to form a new ring of debris around Mars. This new ring would deposit most debris onto Mars, and the leftover ring material would accrete into a new Moon. This recycling hypothesis was challenged by Liang and Hyodo (2023), which showed that particles of rp ∼10 cm may be removed by the Poynting–Robertson (PR) effect over 109 years, and smaller particles would have been removed faster. Liang and Hyodo (2023) indicated that the PR effect alone would have not been able to remove the particles in the disk, and another process would have been needed. Without that, the hypothesis of Hesselbrock and Minton (2017) would not be accurate.
Bagheri et al. (2021) used geophysical and tidal-evolution modelling of a Mars–satellite system to propose another hypothesis, in which Phobos and Deimos were once a single large Moon that later disintegrated into two. This hypothesis was challenged by Hyodo et al. (2022), who indicated that those authors did not study the impact itself, and therefore the outcome was not demonstrated (i.e., that a single Moon splits only into two fragments), and that the gravitational interactions and collisions during a moon–moon close encounter were neglected.
The capture scenario proposes that the moons were asteroids from the main belt, subsequently captured, under suitable conditions, by the gravitational attraction of Mars. This hypothesis arises from remote-sensing observations of the moons that present similarities with numerous low-albedo and small-sized asteroids (Burns, 1978; Pollack et al., 1979; Rosenblatt, 2011). Phobos presents numerous grooves, which also recall some asteroids, and are claimed to have been formed during the capture by Mars (Pollack and Burns, 1977; Hunten, 1979; Thomas et al., 1979). To note that recently, Cheng et al. (2022) have proposed a tidal fracturing model to explain the numerous grooves, with their analysis supporting a layered heterogeneous structure for Phobos with possible underlying failure-induced fractures. However, significant areas showing absences and anomalies were observed, which were not consistent with the tidal fracturing model. Escaping ejecta imprinted Phobos with linear, low-velocity crater chains (sesquinary catenae) matching the morphology of prominent features (grooves) that do not match a tidal model (Murray and Heggie, 2014; Nayak and Asphaug, 2016; Cheng et al., 2022).
Further support for the capture scenario, the resemblance between the surface spectra of Phobos and D-type carbonaceous material points to the condensation of this material in the solar nebula at a distance far away from Mars (Murchie et al., 1991; Yamamoto et al., 2018), which later moved inwards to the inner Solar System (Rosenblatt, 2011). Nevertheless, this scenario is weakened by some ambiguities in the interpretation of the reflectance spectra and requires high tidal dissipation rates inside the moons to account for their current orbits around Mars (Rosenblatt, 2011). According to Fraeman et al. (2014), in this scenario, a possible composition of the surface implies the presence of primitive phyllosilicate-rich carbonaceous chondrites or anhydrous, olivine- and pyroxene-dominated carbonaceous chondrites.
In recent years, the idea that Phobos may result from a collision between an asteroid and Mars has gained popularity. In this scenario, the collision would have formed a disc of debris around the planet, and it has been proposed that these moons formed from the outer edge of such disk, while massive inner moons spiralled inward and were lost (Ronnet et al., 2016; Rosenblatt et al., 2016; Canup and Salmon, 2018; Changela et al., 2022). It is thought that the Martian surface was heated to 3,000–6,000 K immediately after the massive impact, while the building blocks of Phobos, including volatile-rich vapor, were heated to 2,000 K, depleting the present Moon from volatiles (Hyodo et al., 2017). Like the Earth, Mars has too much angular momentum to be explained by the accretion of many small bodies (Dones and Tremaine, 1993a). Studies suggest that a planetesimal with 0.02 Mars masses must have collided with Mars early in its history (Dones and Tremaine, 1993b). This collision would have been able to produce the largest basins on Mars (Borealis, Elysium, Utopia, and Hellas) (Craddock, 2011; Canup and Salmon, 2018), and may have been significant enough to impact Mars at a velocity to vaporize rock, ejecting large amounts of material into orbit (Craddock, 2011). Other lines of evidence point to the low mass and high porosity of Phobos that may be explained by a composition of loosely aggregated material from an accretion disk (Craddock, 2011; Rosenblatt, 2011). Due to the inferred presence of phyllosilicates on Phobos, which are probably derived from Mars, the silica composition also supports this hypothesis (Giuranna et al., 2011). It is considered that Mars-derived phyllosilicates are probably significant sources of phyllosilicate intake on the Moon. The physical properties (orbital eccentricity and inclination) of the satellites, which previous scenarios could not explain, are the natural result of accretion from a circum-planetary disk (Craddock, 2011; Ronnet et al., 2016). In this rationale, disk material would be heated to dehydrate OH-bearing minerals (Lange and Ahrens, 1982), possibly indicating a dry endogenic composition. If a significant impact occurred, the source materials would be mostly Martian, and the refractory elemental composition of Phobos would be Mars-like (Fraeman et al., 2014). Notwithstanding, since the composition of the debris may also come from the impactor, which could be of carbonaceous composition, the in situ formation hypothesis also supports the carbonaceous composition of Phobos (Rosenblatt, 2011). As also discussed by Pignatale et al. (2018), and Ronnet et al. (2016), the spectral properties of Phobos are in accordance with an impact-generated accretion disk formation. A more conclusive answer to the origin of Phobos will come from more in situ measurements or sample return missions, in which state-of-the-art technology will help constrain the various scenarios (see Section 4.3).
3 Martian past
As one of the major terrestrial planets in our the Solar System, Mars contributes to our understanding of the evolution of habitable planets. Throughout its history, Mars has experienced numerous asteroidal impacts from various locations, producing ejecta from its surface. A fraction of this debris is delivered to Earth as meteorites, but another fraction is delivered to Phobos and Deimos and mixed within the surface regolith. Hyodo et al. (2019) reported that the materials delivered to Phobos are physically and chemically different from the Martian meteorites retrieved on Earth, as they are less shocked (<5 GPa) and more fragile. Impacts on the surface of Mars were frequent, and it was already suggested that the regolith of Phobos might hold up to 250 ppm of this material (Ramsley and Head, 2013). As Phobos has been receiving this ejecta representative of the surface of Mars over geological time, Phobos may hold remarking information of the entire history of the planet that would have been altered by the changing geological processes on Mars. If life ever existed on Mars, biosignatures could be carried to Phobos. This could be investigated in the future by the Mars Sample Return (MSR) mission led by NASA-ESA, which will return Mars material from the Jezero crater, and the Japan Aerospace Exploration Agency (JAXA)-led Martian Moons eXploration (MMX) mission, which will collect material from the Martian Moon Phobos (Hyodo and Usui, 2021).
Besides asteroidal impacts in the Mars-Phobos system, atoms and molecules that escape the planetary atmosphere can also be embedded and preserved inside the surface of the satellite (Nénon et al., 2021). Although the age of the surface of Phobos may be uncertain (Schmedemann et al., 2014; Ramsley and Head, 2017; Hu et al., 2020), it is believed that atmospheric ions may have bombarded some regolith during the pre-Noachian epoch of Mars, where water is thought to have existed (Fassett and Head, 2008; Warner et al., 2010). Phobos currently shows its nearside to Mars, but it is likely that the Stickney Crater impact desynchronized the rotation of Phobos (Ramsley and Head, 2017). After the pre-impact tidal lock was restored, Phobos either returned to the original pre-impact longitude or was reoriented into a tidal lock ∼180°. Therefore, atmospheric flux embedded on the top of Phobos regolith may reflect the different periods of time of the geological history after the Stickney Crater impact. Due to its orbit and based on in situ ion observations, Phobos presents a space-weathering dichotomy between the nearside and farside (Nénon et al., 2021). The bombardment by Martian ions can lead to the transformation of an initially crystalline material at a given depth into an amorphous material, creating amorphous rims on regolith grains (Nénon et al., 2021). Sampling the Phobos regolith would provide information about the atmospheric composition of Mars, just like Martian meteorites have (Bogard and Garrison, 1998; Filiberto and Schwenzer, 2019).
4 Observing and exploring Phobos
Phobos was discovered by the astronomer Asaph Hall in 1877, with the collaboration of his wife and mentor, Angeline Stickney Hall (Hall, 1877). However, the first mission to observe this Moon from a closer distance was almost 100 years later. Since the first photograph, taken in 1969 (Masursky et al., 1972), various missions, including failed ones, attempted to study Phobos. Although the majority was aimed for Mars, and there are still no samples from the regolith, several questions have been answered over the past 50 years. Flyby, orbiter, and lander missions that studied Mars were also able to characterize Phobos successfully (Figure 1). In recent years, due to the possibility of having humans on Mars in the 2040s (Deutsch et al., 2018), Phobos has been viewed as a potential staging area for future human exploration of Mars. In this section, a brief history of the exploration of Phobos, including past and present missions, along with the main findings essential for the characterization of Phobos, will be reported. The future of the research concerning Phobos will also be discussed.
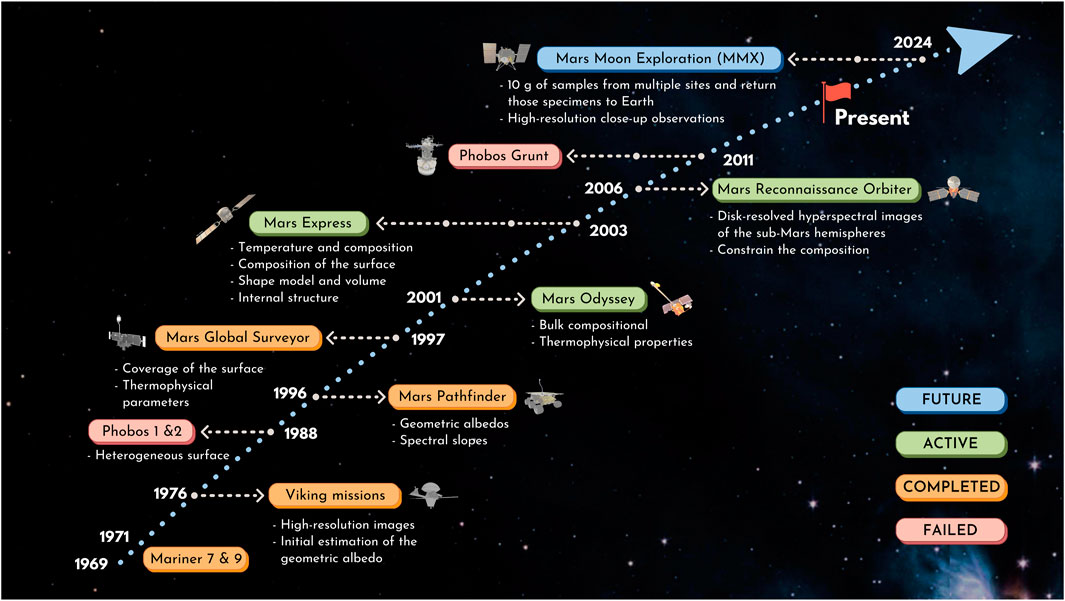
FIGURE 1. Timeline of the exploration of Phobos with corresponding achievements. Failed missions are represented in red, completed missions in orange, still active missions in green, and future mission in blue. ©Image by Catarina Miranda.
4.1 Past and present space missions
To address the distinct hypotheses and other aspects of its history, Phobos has been studied via spacecraft-based thermal, imaging, spectroscopy, and gravity measurements. Most missions that observed Phobos were flyby, orbiter, or lander/rover destined to study Mars (Siddiqi, 2018 and Figure 1). Nevertheless, as the interest in exploring Phobos grew, missions were explicitly developed for investigating this satellite (e.g., Marov et al., 2004; Nakamura et al., 2021).
Phobos was first photographed by some early probes to Mars as flyby targets of opportunity, beginning with the Mariner 7 in 1969, followed by Mariner 9 spacecraft, launched in 1971 (Masursky et al., 1972). Although the observation of Phobos was not initially scheduled, the final proposed orbits for the Mariner 9 mission were sufficiently close to use the high-resolution B-camera (Pollack et al., 1972), infrared (IR) radiometer (Gatley et al., 1974), and ultraviolet (UV) spectrometer onboard (Chase and Hat, 1972). A total of 214 images of Phobos and Deimos, some as close as 1,200 km were recovered (Duxbury et al., 2014). The subsequent missions to explore Phobos were the Viking missions, launched in 1975, which placed two orbiters around Mars and two landers on the Martian surface in 1976. During the Viking primary mission, from June to November 1976, the cameras on the two orbiters took high-resolution images (<500 m) of Phobos, completing its surface coverage and leading to the discovery of a few unusual surface features, including a global network of grooves (Duxbury and Veverka, 1977; Veverka and Duxbury, 1977). In the extended mission phase, close flybys within ∼100 km were performed. The infrared thermal mappers (IRTMs) onboard the orbiters carried extensive thermal infrared observations of the Martian satellite at various ranges, observing geometries, and wavelengths, allowing the deduction of the near-surface thermophysical properties (Lunine et al., 1982). A sequence of pictures taken from the narrow-band blue and red diodes, and one of the three infrared imaging (in this case IR2) diodes onboard the Viking lander also enabled an initial estimation of the geometric albedo of Phobos (Pollack et al., 1978). Named after their target, Phobos 1 and Phobos 2 missions, consisting of an orbiter and lander spacecraft, were sent in 1988 and lost prematurely, with Phobos 1 lost on the way to Mars. Nevertheless, before losing contact nearly an hour before it was due for a touch-down, Phobos 2 observed parts of the surface with three multispectral sensors, covering the wavelength range 0.33–3.16 μm (Murchie and Erard, 1996). Thirty-seven images during three (700 km) encounters were retrieved, showing the presence of more grooves (Avanesov et al., 1989) and a heterogeneous surface (Murchie and Erard, 1996), consisting of two spectral units (see Section 3.3 Characterization of Phobos).
Almost 10 years later, on the surface of Mars, the 1996 Mars Pathfinder Rover was also able to make multispectral measurements of Phobos, using the Imager to Mars Pathfinder (IMP) onboard, showing consistent results with previous data (Murchie et al., 1999; Thomas et al., 1999). The satellite was observed on two occasions (Murchie et al., 1999), covering the wavelength range from 440 nm to 1 μm. The spectra retrieved were used to derive the geometric albedos and spectral slopes of the objects (Thomas et al., 1999). The IMP data also contributed to tantalizing but inconclusive evidence for the presence of some type of Fe mineral having an absorption near 700 nm (Murchie et al., 1999).
Over 350 resolved observations of Phobos were performed by the 1997 Mars Global Surveyor (MGS). As MGS went into the orbit of Mars, close flybys of Phobos were targeted. Data retrieved from the scientific remote sensing payload: Thermal Emission Spectrometer (TES), Mars Orbiter Camera (MOC), and Mars Orbiter Laser Altimeter (MOLA), provided coverage of the surface of Phobos (Duxbury et al., 2014), allowing insights into the variations of thermophysical parameters across the surface (Smith et al., 2018). In the following year, the first Japanese planetary spacecraft (Ichiro Kawaguchi, 1999) named NOZOMI was launched. This mission was expected to take pictures of Mars and its moons. However, the spacecraft did not reach Mars, and therefore did not return any data about Mars and/or its moons, only providing valuable data about the deep space environment (Siddiqi, 2002). In October 2001, the 2001 Mars Odyssey spacecraft performed a low, circular orbit around Mars and is still operating Clique ou toque aqui para introduzir texto. Although it was not possible to observe Phobos right away, the Thermal Emission Imaging System (THEMIS) was able to study the satellite in the following months. THEMIS enabled the characterization and mapping of the bulk compositional and thermophysical properties of Phobos. This instrument collected images in the thermal infrared (TIR) and visible (VIS) wavelengths, observing the shadow of Phobos in February 2012 (Duxbury et al., 2014), and the full Moon near the closest approach on 19 September 2017 (Bandfield et al., 2018). The retrieved TIR images revealed new spectral and thermophysical properties, possibly indicating significant compositional heterogeneity (see Section 3.3 Characterization of Phobos) (Bandfield et al., 2018).
The Mars Express (MEx) mission has been orbiting Mars since 24 December 2003, approaching Phobos at distances less than1000 km, at about every 500 orbits (5 months) (Witasse et al., 2014). MEx has an orbit that extends beyond Phobos, providing data for the side that is not facing Mars (Duxbury et al., 2014). The instrumentation onboard comprises the High-Resolution Stereo Camera (HRSC), imaging spectrometer Observatoire pour la Minéralogie, l'Eau, les Glaces et l'Activité (OMEGA), Planetary Fourier Spectrometer (PFS), UV-IR spectrometer Spectroscopy for the Investigation of the Characteristics of the Atmosphere of Mars (SPICAM), radar instrument Mars Advanced Radar for Subsurface and Ionosphere Sounding (MARSIS), plasma package Analyzer of Space Plasmas and EneRgetic Atoms (ASPERA-3), and the Mars Radio Science experiment (MaRS) (Witasse et al., 2014). These instruments were designed to study Mars but are very well suited for the observation of Phobos. In fact, MEx mapped 90% of the surface, allowing images in 3-D and colour to be taken, including high-resolution images of the far side of Phobos, adding substantially to the science of Phobos. Other achievements include the study of the temperature and composition of the surface with the IR and UV-VIS-IR spectrometers, respectively, the investigation of the internal structure of the satellite and detection of backscattered solar wind protons from the surface of Phobos, enlightening the regolith-solar wind interaction, and the refinement of the ephemerides (Witasse et al., 2014). Data retrieved also improved the shape model and volume of Phobos, constrained the composition of the surface, allowed new geologic interpretation for the grooves, and contributed to the still debatable dating of the surface age of Phobos (Witasse et al., 2014). Several authors have attempted to age-dating Phobos by counting craters (see, e.g., Schmedemann et al., 2014). However, the overprinting of secondary craters inside the Stickney crater does not allow a feasible crater age-dating (Ramsley and Head, 2017). This gives an indication of a history of several impacts on Phobos, but it does not allow to obtain information on the present-day surface age.
Another still operating spacecraft is the Mars Reconnaissance Orbiter (MRO), which went into its low, near-circular orbit in August 2006. For calibration purposes, Phobos and Deimos were observed as point sources against star fields by the Navigation Camera during the approach (Thomas et al., 2011). The hyperspectral infrared Compact Reconnaissance Imaging Spectrometer for Mars (CRISM) (Murchie et al., 2007; Fraeman et al., 2012; Pajola et al., 2018) and the High-Resolution Imaging Science Experiment (HiRISE) (Thomas et al., 2011) onboard were able to image Phobos (see Figure 2). These instruments are expected to add new observations of the satellite periodically. CRISM has two high spatial resolution spectrometers with a spectral resolution of 6.55 nm/channel, whereby one channel is in the VIS/NIR region (from 0.4 to 1.0 mm), and the other in the IR region (1.0–3.9 mm) (Murchie et al., 2007). This instrument has acquired disk-resolved hyperspectral images of the sub-Mars hemispheres of Phobos, and along HiRISE, helped to constrain the knowledge on the composition of Phobos (Murchie et al., 2007; Thomas et al., 2011; Pajola et al., 2018).
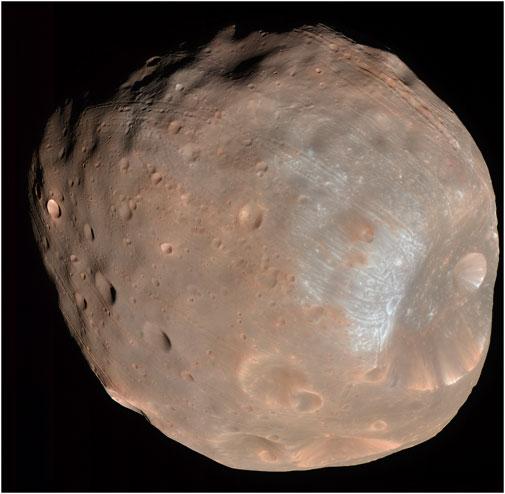
FIGURE 2. VIS/NIR colour composite images of Phobos from HiRISE instrument onboard MRO. The biggest crater, Stickney is on the left edge of the image. The image is in the HiRISE catalog as PSP_007769_9010_IR. Credit NASA/JPL-Caltech/UArizona; https://www.uahirise.org/releases/phobos/.
The last mission sent with the ability to study in detail Phobos was the follow-up to Phobos 2 mission, named Phobos-Grunt. This spacecraft was launched on 9 November 2011 and intended to return up to 200 g of regolith to Earth (Galimov, 2010; Murchie et al., 2014). However, soon after launch, the spacecraft failed, and the probe re-entered the atmosphere of the Earth in January 2012 (Murchie et al., 2014). Presently, the investigation of Phobos relies on the still operating orbiter missions (Mars Odyssey, MEx, and MRO) and surface observations during the solar transits by the Mars Surface Laboratory Curiosity, and Interior Exploration using Seismic Investigations, Geodesy and Heat Transport (InSight) mission (Duxbury et al., 2014; Stähler et al., 2020).
4.2 Future exploration of Phobos
Various missions already had the opportunity to observe Phobos, while others remain at the mission concept stage. While missions that remain at the concept stage were never launched, information is still gained: a team of scientists and engineers develop their concept around a question and idea, and their proposal is evaluated for scientific merit and feasibility, including among others, power consumption, mass, operations to be carried out and instruments to be used. At this point, the concept of the proposal is fine-tuned, so even when a space mission concept is not selected and approved, the information that is gained in this process will inform efforts in other future space projects, in terms of science, payload and engineering, therefore reducing risk and guarantying a cost-effective proposal. The proposed Phobos mission concepts can be divided into three different goals: sample return, imaging and spectroscopy, and high-resolution imaging. Sample return missions include the low-cost Discovery mission Aladdin (Mueller et al., 2003), Martian Moon Sample Return (MMSR) (Michel et al., 2011), PHOOTPRINT (Galimov, 2010), and HALL (Lee et al., 2010) missions. On the other hand, Mars Phobos and Deimos Survey (M-PADS) (Ball et al., 2009), Phobos Close Rendevous Observation Sensing Satellite (PCROSS) (Bellerose and Andrews, 2012), Phobos Surveyor (Lang et al., 2012), PANDORA (Raymond et al., 2015), and CubeSat missions Low Orbit Geology Imaging CubeSat (LOGIC) (Thangavelautham et al., 2017), and Perseus (Nallapu et al., 2020) were proposed as imaging and spectroscopy missions, and NASA Discovery mission Phobos And Deimos and Mars Environment (PADME) (Lee et al., 2014) as a high-resolution mission.
Currently, the only approved mission targeting Phobos is the JAXA mission named MMX (see Figure 1), which is planned for launch in 2024 (Ulamec et al., 2019). MMX focuses on revealing the origin of the Martian moons, and making progress in the study of planetary system formation and primordial material transport around the border between the inner- and outer parts of the early Solar System. In addition, MMX aims to understand the physical processes in the circum-Martian environment and atmosphere of Mars, improving the knowledge about the evolution of the Martian moons and the surface environmental transition of the Red Planet (Kuramoto et al., 2018). As such, the main spacecraft will make high-resolution close-up observations of both moons from orbit, and a small rover will be delivered to the surface of Phobos. More than 10 g of samples from multiple sites will be collected by the main lander, and the MMX spacecraft will later return those specimens to Earth. Once in the laboratory, scientists will analyse the regolith, measure the mineralogical composition of the surface, and determine the thermal properties of the surface material (Kuramoto et al., 2018). The mineralogical composition of the specimens will be scrutinized with chronological analysis, unravelling the origin and cosmochemical nature of Phobos in the context of planet formation and the evolution of the Martian system. The operation period will be shorter than 2.5 years (Hyodo et al., 2019), where rover operations are foreseen for at least 100 days in 2026. This mission is supposed to return in 2028, arriving on Earth in 2029 (Kuramoto et al., 2018).
MMX will be crucial to finally determine the origin of Phobos, combining close-up spectroscopic observations of the mineralogical and chemical composition of bedrock material of Phobos, with sample analyses, such as petrological, chemical and isotopic characterization (Kuramoto et al., 2022). A capture origin will be supported if the Phobos bedrock material is enriched in hydrous minerals and organic matter, therefore being similar to carbonaceous meteorites. The capture scenario is further supported if a sign of internal ice is detected (either through water molecules emerging from the surface of Phobos and/or internal density heterogeneity). Alternatively, if the Phobos samples are volatile-poor, and igneous characteristics are found, then a giant impact is supported (Kuramoto et al., 2022).
4.3 Characterization of Phobos
The Martian Moon Phobos is an irregularly shaped and low-density body (Pätzold et al., 2014), whose surface can be characterized as an arena for various geologic processes. The significant landforms detectable on the surface are craters and grooves (Basilevsky et al., 2014). From available spectroscopic data, Phobos features two distinct units (e.g., (Thomas et al., 1999); (Murchie and Erard, 1996); (Thomas et al., 2011): the “blue unit”, which follows Stickney crater and continues eastward for about 5–7 km (proximal ejecta), and the “red unit”, corresponding to the remaining surface (Pieters et al., 2014). UV-VIS albedo patterns, the relationship between color and albedo, thermal IR spectra, and the UV continuum slope spectral properties can distinguish both units, possibly constraining the mineralogy of the surface materials. VIS to NIR spectra acquired are commonly used to establish, or at least confine, the mineral composition of Solar System bodies through the identification of highly diagnostic mineral absorption bands (Pieters et al., 2014).
As a result of its proximity with Mars and slightly eccentric orbit, Phobos experiences downslope movements, altering the global distribution of dynamic slopes (Crites et al., 2018). This mass movements play an important role in surface modification by exposing fresh sub-surface material. In fact, there is a correlation between mass wasting characteristics and regions where the dynamic slope increased because of the tidal action (Shi et al., 2016). On the “blue unit”, on the eastern ridge of Stickney (Shingareva and Kuzmin, 2001; Shi et al., 2016; Crites et al., 2018).
Thermal infrared measurements performed by the PFS/MEx (Giuranna et al., 2011), TES/MGS (Giuranna et al., 2011; Ebel and Brook, 2015), THEMIS/Mars Odyssey (Bandfield et al., 2018) and CRISM/MRO (Fraeman et al., 2014) indicated the presence of phyllosilicates (which are the primary phase in carbonaceous chondrite meteorites) on parts of Phobos. Although it was initially interpreted to be a signature associated with the “blue unit” (Giuranna et al., 2011), the visual correlation of the footprints of the spectra of phyllosilicates with CRISM colour variations indicates a better match with the “red unit” (Fraeman et al., 2014). According to (Fraeman et al., 2014), the “blue unit” is spectrally featureless in the thermal infrared, as it is in the near-infrared. Spectral analyses from PFS and TES also point to the presence of feldspars/feldspathoids. Notwithstanding, there are differences compared to the spectra observed in the laboratory, and classes of chondrites are excluded, as they do not present a good agreement with the spectral features observed (Giuranna et al., 2011). The THEMIS continuum-removed spectra hit for the presence of material with identical SiO2 content to pyroxene minerals or basaltic rocks, as opposed to more silicic or ultramafic compositions (Bandfield et al., 2018).
Two focal absorption features in the VIS/NIR region could be differentiated at 0.65 μm and 2.8 μm. The broad absorption centered at 0.5 μm was highlighted by CRISM but reexamined observations from IMP/Pathfinder (Murchie et al., 1999), Phobos-2 (Murchie and Erard, 1996), and OMEGA/MEx (Fraeman et al., 2012) are also in agreement. Cronstedtite (the most common phyllosilicate in CM chondrites) and nontronite (Fe-bearing phyllosilicate) were intended to match this feature but proved inadequate. Moreover, Fe2+ electronic absorptions diagnostic of olivine and pyroxene were not detected. However, a mixture of iron particles ranging in size from a few nanometres to a few micrometres provided an alternative spectral match (Fraeman et al., 2014). Without the thermally emitted component, a shallower metal-OH absorption band in the “blue unit” compared to the “red unit” was detected at 2.8 μm. The strength, position, and shape of both features are similar to characteristics seen on red-sloped, low-albedo primitive asteroids. Therefore, evidence that desiccated phyllosilicates may be present on the surface of Phobos. As an alternative to explaining the presence of highly desiccated Fe-phyllosilicate minerals, the idea that space weathering-related processes may create Fe and OH on the surface was also suggested to justify the features observed by CRISM (Fraeman et al., 2014).
The studies already performed helped constrain the possible mineral composition of Phobos, pointing to the plausible presence of phyllosilicates, specifically desiccated Fe-phyllosilicates (e.g., Nontronite), feldspars/feldspathoids, pyroxene minerals, or basaltic rocks. The future investigation should depend on samples from the regolith and comparison with the already mentioned minerals.
5 Simulant regolith and mineralogical composition
In the absence of specimens from the regolith of Phobos here on Earth, analogous or simulant regolith are an useful solution for inferring properties and complement in situ studies in the future. Composition, size, shape distribution, and packing density are the four major characteristics needed to specify a simulant (Rickman et al., 2013). Since there are no specimens from Phobos, none of the characteristics are explicitly known. As such, specifications for a Phobos simulant must be based on spectroscopy and thermophysical properties or other measurements and informed judgment. So far, three Phobos regolith simulants have been developed: Phobos Captured Asteroid-1 (PCA-1), Phobos Giant Impact-1 (PGI-1) (Landsman et al., 2021), and Phobos-1C (Rickman et al., 2016). It is still not clear which hypothesis, i.e., either a capture scenario or in situ formation/giant impact explains the origin of Phobos. Furthermore, in this last scenario the composition of Phobos would result from reaccreted impact ejecta from Mars, therefore it is important to study both Phobos and Mars analogues (for example, JSC Mars-1). There are still various questions regarding the Mars-Phobos system, and by studying both regoliths, one can complement this intriguing riddle. The composition of Phobos-1C, PCA-1, PGI-1, and JSC Mars-1 analogues are described in Table 1.
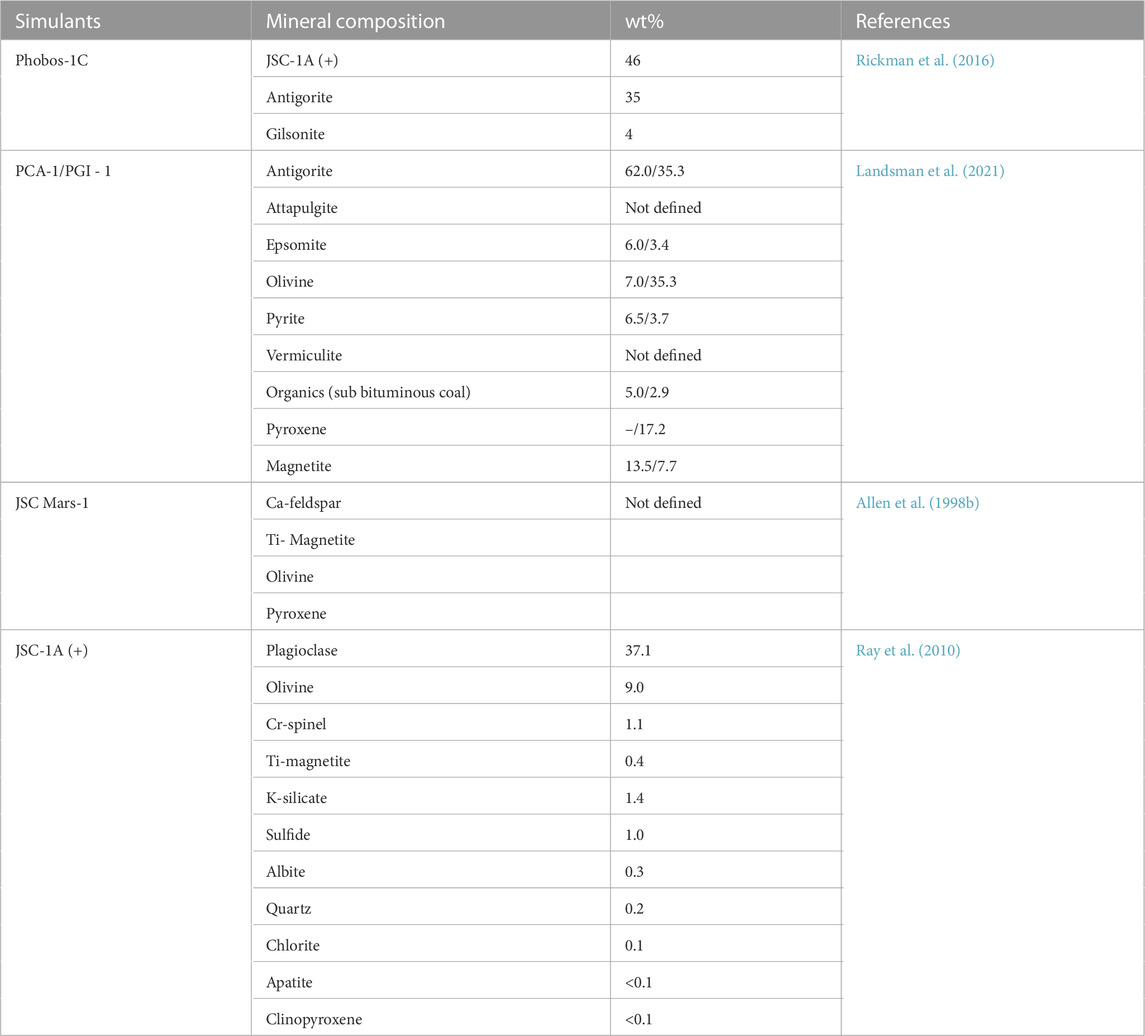
TABLE 1. Mineral composition of the different simulant of Phobos (Phobos Captured Asteroid-1 (PCA-1), Phobos Giant Impact-1 (PGI-1), and Phobos-1C), and Mars (JSC Mars-1). JSC-1A (+) is a component of Phobos-1C.
Phobos-1C is a compositional simulant, for which the Tagish Lake meteorite was taken as an estimation for the target composition, customized for the effects of space weathering at the surface. This simulant is composed of the lunar soil simulant JSC-1A (Ray et al., 2010), pseudo-agglutinate, antigorite, and gilsonite. The first two components provide glass with microcrystals and mineral grains, while antigorite was used to represent phyllosilicates found in the meteorite and gilsonite to mimic the complex organic constituents (Rickman et al., 2016). PCA-1 and PGI-1 are also compositional simulants based on the inferred mineralogy of Phobos (Landsman et al., 2021). These regoliths manifest both hypotheses for the formation of Phobos. PCA-1 is based on the scenario that Phobos is a captured primitive asteroid, where the primitive carbonaceous CI meteorite Orgueil (Metzger et al., 2019) was used as a reference material. PGI-1 was developed under the assumption that Phobos is probably a mixture of the upper mantle of Mars and a compositionally primitive impactor. In fact, numerical simulations suggest that the Martian crust could have also been mixed because of a giant impact (Hyodo et al., 2017; Pignatale et al., 2018). As such, the composition of PGI-1 is 43% simplified Mars upper mantle material (olivine and pyroxene), and 57% CI simulant (Landsman et al., 2021). Both simulants have already been studied, specifically their geotechnical properties, and tested in the context of spacecraft hardware and mission operations for the upcoming MMX mission. In the latter studies, the regoliths help constraining the designs regarding the sampling and landing mechanisms (D’Amore et al., 2019; Miyamoto et al., 2018). Using the same recipe as PCA-1 and PGI-1, but sourced in Japan, Miyamoto et al. (2018) developed the “University of Tokyo Phobos Simulant-Impact Based” (UTPS-IB) and the “University of Tokyo Phobos Simulant-Tagish Based” (UTPS-TB).
JSC Mars-1 is a Hawaiian altered volcanic soil consisting of a mixture of ash particles (Allen et al., 1998a). This regolith has been used in various Mars-oriented research studies (Sternovsky et al., 2002; Kral et al., 2004; Garry et al., 2006). Fortunately, this analogue has already been well characterized by Allen et al. (1998a) and Moroz et al. (2009). According to these authors, a relatively featureless ferric absorption edge can be detected in the visible. There is also an indication of a ferric absorption band in the 800–1,000 nm region and a relatively flat absorption in the near-IR spectrum. X-Ray diffraction (XRD) spectra of JSC Mars-1 are dominated by peaks corresponding to Ca-feldspar and minor magnetite (Ti-magnetite), but olivine and pyroxene could also be evidenced. Nevertheless, the spectra from the JSC Mars-1 do not present evidence for a composition rich in phyllosilicates (Allen et al., 1998).
6 Conclusion
After more than 50 years of studying Phobos, this Moon remains a mystery. Intriguing questions regarding the origin, composition and relation with the history of Mars are still unanswered. From the two main hypothesis that prevail, capture scenario or giant impact, the latter gained popularity in recent years. As such, in the absence of regolith from this satellite, and taking into consideration that the composition of Phobos may be similar to Mars, it is also important to consider Martian analogues in different regolith studies. Analogue regoliths from Phobos have already been developed and are key elements to complement in situ studies in the future. Phobos simulants may be used to support future space missions to test protocols and payload instrumentation, as well as to establish and better understand Phobos properties. The Phobos analogues PCA-1, PGI-1, and Phobos-1C, along the Martian analogues (i.e., JSC-1) may be crucial to infer properties for future space missions, such as the MMX. The JAXA mission will study Phobos, and hopefully will return regolith samples back to Earth by 2029. These samples will later be studied by state-of-the-art technology, and will finally provide detailed information on the physical and chemical properties of the Phobos regolith, and its origin.
Author contributions
AC, ZM, TH, and MB-S contributed to conception and design of the study. CM wrote the first draft of the manuscript, with contribution by BP and ZM. All authors contributed to the article and approved the submitted version.
Funding
CM was financed by Fundo Europeu de Desenvolvimento Regional (FEDER) funds through the COMPETE 2020 - Operational Programme for Competitiveness and Internationalisation (POCI), and by Portuguese funds through FCT—Fundação para a Ciência e a Tecnologia in the framework of the project POCI-01-0145-FEDER-029932 (PTDC/FIS-AST/29932/2017). Centro de Química Estrutural (CQE) acknowledges the financial support of FCT—Fundação para a Ciência e Tecnologia through grants UIDB/00100/2020 and UIDP/00100/2020. Institute of Molecular Sciences acknowledges the financial support of FCT—Fundação para a Ciência e Tecnologia through grant LA/P/0056/2020. Institute for Bioengineering and Biosciences (iBB) acknowledges the financial support of FCT—Fundação para a Ciência e Tecnologia through grants UIDB/04565/2020 and UIDP/04565/2020. The Associate Laboratory Institute for Health and Bioeconomy (i4HB) acknowledges the financial support of FCT—Fundação para a Ciência e Tecnologia through grant LA/P/0140/2020. Centro de Física da Universidade de Coimbra (CFisUC) acknowledges the financial support of FCT—Fundação para a Ciência e Tecnologia through grants UIDB/04564/2020 and UIDP/04564/2020. The Open University acknowledges funding from the UK Space Agency through grants ST/V005332/1 and ST/V002295/1.
Acknowledgments
We are grateful to three referees for helpful comments.
Conflict of interest
TH was employed by Spin.Works.
The remaining authors declare that the research was conducted in the absence of any commercial or financial relationships that could be construed as a potential conflict of interest.
Publisher’s note
All claims expressed in this article are solely those of the authors and do not necessarily represent those of their affiliated organizations, or those of the publisher, the editors and the reviewers. Any product that may be evaluated in this article, or claim that may be made by its manufacturer, is not guaranteed or endorsed by the publisher.
References
Allen, C. C., Jager, K. M., Morris, R. v., Lindstrom, D. J., Lindtsrom, M. M., and Lockwood, J. P. (1998a). Martian soil stimulant available for scientific, educational study. Eos, Trans. Am. Geophys. Union79 (34), 405–409. doi:10.1029/98EO00309
Allen, C. C., Morris, R. V., Jager, K. M., Golden, D. C., Lindstrom, D. J., Lindstrom, M. M., et al. (1998b). “Martian regolith simulant JSC MARS-1,” in 29th Annual Lunar and Planetary Science Conference, Houston, TX,, March 16-20, 1998.
Avanesov, G. A., Bonev, B. I., Kempe, F., Bazilevsky, A. T., Boycheva, V., Chikov, K. N., et al. (1989). Television observations of Phobos. Nature341 (6243), 585–587. doi:10.1038/341585a0
Bagheri, A., Khan, A., Efroimsky, M., Kruglyakov, M., and Giardini, D. (2021). Dynamical evidence for Phobos and Deimos as remnants of a disrupted common progenitor. Nat. Astron5, 539–543. doi:10.1038/s41550-021-01306-2
Ball, A. J., Price, M. E., Walker, R. J., Dando, G. C., Wells, N. S., and Zarnecki, J. C. (2009). Mars Phobos and Deimos Survey (M-PADS) - a martian moons orbiter and Phobos lander. Adv. Space Res.43 (1), 120–127. doi:10.1016/j.asr.2008.04.007
Bandfield, J. L., Piqueux, S., Glotch, T. D., Shirley, K. A., Duxbury, T. C., and Edwards, C. S. (2018). “Mars Odyssey themis observations of Phobos: New spectral and thermophysical measurements,” in 49th Lunar and Planetary Science Conference, Woodlands, TX, March 19-23, 2018, 7–8.
Basilevsky, A. T., Lorenz, C. A., Shingareva, T. V., Head, J. W., Ramsley, K. R., and Zubarev, A. E. (2014). The surface geology and geomorphology of Phobos. Planet. Space Sci.102 (C), 95–118. doi:10.1016/J.PSS.2014.04.013
Bellerose, J., and Andrews, D. (2012). Phobos close rendevous observation sensing satellite A introduction: PCROSS is a robotic pre-cursor mission. Concepts Approaches MarsExploration1679, 4180.
Bogard, D. D., and Garrison, D. H. (1998). Relative abundances of argon, krypton, and xenon in the Martian atmosphere as measured in Martian meteorites. Geochimica Cosmochimica Acta62 (10), 1829–1835. doi:10.1016/S0016-7037(98)00116-1
Burns, J. A. (1978). The dynamical evolution and origin of the Martian moons. Vistas Astronomy22 (2), 193–210. doi:10.1016/0083-6656(78)90015-6
Canup, R., and Salmon, J. (2018). Origin of Phobos and Deimos by the impact of a vesta-to-ceres sized body with Mars. Sci. Adv.4 (4), eaar6887. doi:10.1126/sciadv.aar6887
Chambers, J. E. (2004). Planetary accretion in the inner solar system. Earth Planet. Sci. Lett.223 (3–4), 241–252. doi:10.1016/J.EPSL.2004.04.031
Changela, H. G., Chatzitheodoridis, E., Antunes, A., Beaty, D., Bouw, K., Bridges, J. C., et al. (2022). Mars: New insights and unresolved questions. Int. J. Astrobiol.20 (6), 394–426. doi:10.1017/S1473550421000276S
Chase, J. R., Hat, H., Stewart, A. I., and Lane, A. L. (1972). Mariner 9 ultraviolet spectrometer experiment: Initial results. Science175 (4019), 309–312. doi:10.1126/science.175.4019.309
Cheng, B., Asphaug, E., Ballouz, R.-L., Yu, Y., and Baoyin, H. (2022). Numerical simulations of drainage grooves in response to extensional fracturing: Testing the Phobos groove formation model. Planet. Sci. J.3 (11). doi:10.3847/PSJ/ac8c33
Craddock, R. A. (2011). Are Phobos and Deimos the result of a giant impact?Icarus211 (2), 1150–1161. doi:10.1016/j.icarus.2010.10.023
Crites, S., Kawakatsu, Y., Fujimoto, M., Ballouz, R.-L., and Baresi, N. (2018). The shifting sands of Phobos: Space weathering and mass wasting on Mars’ moon. Cosp42, B1.1-62–18. doi:10.1016/J.PSS.2014.02.008KALUNA
D’Amore, M., Maturilli, A., Miyamoto, H., Niihara, T., Grott, M., Knollenberg, J., et al. (2019). “Phobos regolith simulant for MMX mission: Spectral measurement for remote target identification and deconvolution system training,” in 50th Lunar and Planetary Science Conference, Woodlands, TX, March 18-22, 2019.
Deutsch, A. N., Head, J. W., Ramsley, K. R., Pieters, C. M., Potter, R. W. K., Palumbo, A. M., et al. (2018). Science exploration architecture for Phobos and Deimos: The role of Phobos and Deimos in the future exploration of Mars. Adv. Space Res.62 (8), 2174–2186. doi:10.1016/j.asr.2017.12.017
Dones, L., and Tremaine, S. (1993a). On the origin of planetary spins. Icarus103 (1), 67–92. doi:10.1006/icar.1993.1059
Dones, L., and Tremaine, S. (1993b). Why does the Earth spin forward?Science259 (5093), 350–354. doi:10.1126/science.259.5093.350
Duxbury, T. C., and Veverka, J. (1977). Viking imaging of Phobos and Deimos: An overview of the primary mission. J. Geophys. Res.82 (28), 4203–4211. doi:10.1029/JS082i028p04203
Duxbury, T. C., Zakharov, A. V., Hoffmann, H., and Guinness, E. A. (2014). Spacecraft exploration of Phobos and Deimos. Planet. Space Sci.102 (C), 9–17. doi:10.1016/j.pss.2013.12.008
Ebel, D. S., and Brook, S. (2015). “Spectral properties of Phobos from the Mars global surveyor thermal emission spectrometer: Evidence for water and carbonate,” in 46th Lunar and Planetary Science Conference, Woodlands, Texas, March 16–20, 2015, 5–6.
Fassett, C. I., and Head, J. W. (2008). Valley network-fed, open-basin lakes on Mars: Distribution and implications for Noachian surface and subsurface hydrology. Icarus198 (1), 37–56. doi:10.1016/j.icarus.2008.06.016
Filiberto, J., and Schwenzer, S. P. (2019). “Introduction to volatiles in the martian crust,” in Volatiles in the martian crust (Amsterdam, Netherlands: Elsevier), 1–12.
Fraeman, A. A., Arvidson, R. E., Murchie, S. L., Rivkin, A., Bibring, J. P., Choo, T. H., et al. (2012). Analysis of disk-resolved OMEGA and CRISM spectral observations of Phobos and Deimos. J. Geophys. Res. E Planets117 (10), 1–11. doi:10.1029/2012JE004137
Fraeman, A. A., Murchie, S. L., Arvidson, R. E., Clark, R. N., Morris, R. v., Rivkin, A. S., et al. (2014). Spectral absorptions on Phobos and Deimos in the visible/near infrared wavelengths and their compositional constraints. Icarus229, 196–205. doi:10.1016/j.icarus.2013.11.021
Galimov, E. M. (2010). Phobos sample return mission: Scientific substantiation. Sol. Syst. Res.44 (1), 5–14. doi:10.1134/S0038094610010028
Garry, J. R. C., Loes ten Kate, I., Martins, Z., Nørnberg, P., and Ehrenfreund, P. (2006). Analysis and survival of amino acids in Martian regolith analogs. Meteorit. Planet. Sci.41 (3), 391–405. doi:10.1111/j.1945-5100.2006.tb00470.x
Gatley, I., Kieffer, H., Miner, E., and Neugebauer, G. (1974). Infrared observations of PHOBOS from mariner 9. Astrophysical J.190, 497–503. doi:10.1086/152902
Giuranna, M., Roush, T. L., Duxbury, T., Hogan, R. C., Carli, C., Geminale, A., et al. (2011). Compositional interpretation of PFS/MEx and TES/MGS thermal infrared spectra of Phobos. Planet. Space Sci.59 (13), 1308–1325. doi:10.1016/J.PSS.2011.01.019
Hesselbrock, A., and Minton, D. (2017). An ongoing satellite–ring cycle of Mars and the origins of Phobos and Deimos. Nat. Geosci.10, 266–269. doi:10.1038/ngeo2916
Hu, X., Oberst, J., and Willner, K. (2020). Equipotential figure of Phobos suggests its late accretion near 3.3 Mars radii. Geophys. Res. Lett.47, 1–7. doi:10.1029/2019GL085958
Hunten, D. M. (1979). Capture of Phobos and Deimos by photoatmospheric drag. Icarus37 (1), 113–123. doi:10.1016/0019-1035(79)90119-2
Hyodo, R., Genda, H., Charnoz, S., and Rosenblatt, P. (2017). On the impact origin of Phobos and Deimos. I. Thermodynamic and physical aspects. Astrophysical J.845 (2), 125. doi:10.3847/1538-4357/AA81C4
Hyodo, R., Genda, H., Sekiguchi, R., Madeira, G., and Charnoz, S. (2022). Challenges in forming Phobos and Deimos directly from a splitting of an ancestral single moon. Planet. Sci. J.3, 204. doi:10.3847/PSJ/ac88d2
Hyodo, R., Kurosawa, K., Genda, H., Usui, T., and Kazuhisa, F. (2019). Challenges in forming Phobos and Deimos directly from a splitting of an ancestral single moon. Planet. Sci. J.3, 204–206. doi:10.3847/PSJ/ac88d2
Hyodo, R., and Usui, T. (2021). Searching for life on Mars and its moons: Sample-return missions will look for extraterrestrial life and biomarkers on Mars and Phobos. Science373 (6556), 742. doi:10.1126/science.abj1512
Ichiro Kawaguchi, J. (1999). “On the lunar and heliocentric gravity assist experienced in the planet-B (“NOZOMI”),” in Fourth International Symposium on Space Flight Dynamics, Foz do Iguaçu, Brazil, February 8–12, 1999.
Kral, T. A., Bekkum, C. R., and McKay, C. P. (2004). Growth of methanogens on a Mars soil simulant. Orig. Life Evol. Biosphere34 (6), 615–626. doi:10.1023/B:ORIG.0000043129.68196.5F
Kuramoto, K., Kawakatsu, Y., Fujimoto, M., Araya, A., Barucci, M. A., Genda, H., et al. (2022). Martian moons exploration MMX: Sample return mission to Phobos elucidating formation processes of habitable planets. Earth Planets Space74 (12), 12–31. doi:10.1186/s40623-021-01545-7
Kuramoto, K., Kawakatsu, Y., Fujimoto, M., Genda, H., Imamura, T., Kameda, S., et al. (2018). “Martian moons exploration (MMX) conceptual study update,” in 49th Lunar and Planetary Science Conference, Woodlands, TX, March 19-23, 2018, 1–2.
Landsman, Z. A., Schultz, C. D., Britt, D. T., Peppin, M., Kobrick, R. L., Metzger, P. T., et al. (2021). Phobos regolith simulants PGI-1 and PCA-1. Adv. Space Res.67 (10), 3308–3327. doi:10.1016/j.asr.2021.01.024
Lang, J. J., Baker, J. D., Castillo-Rogez, J. C., Mcelrath, T. P., Piacentine, J. S., and Snyder, J. S. (2012). Phobos surveyor - Phobos exploration using two small solar electric propulsion spacecraft. Pasadena, CA: Jet Propulsion Laboratory, National Aeronautics and Space Administration.
Lange, M. A., and Ahrens, T. J. (1982). The evolution of an impact-generated atmosphere. Icarus51 (1), 96–120. doi:10.1016/0019-1035(82)90031-8
Lee, P., Benna, M., Britt, D., Colaprete, A., Davis, W., Delory, G., et al. (2014). PADME (Phobos and Deimos and Mars environment): A proposed NASA discovery mission to investigate the two moons of Mars. Available at: https://ntrs.nasa.gov/api/citations/20150001913/downloads/20150001913.pdf.
Lee, P., Veverka, J., Bel-Lerose, J., Boucher, M., Boynton, J., Braham, S., et al. (2010). “Hall: A Phobos and Deimos sample return mission,” in 41st Lunar and Planetary Science Conference, Woodlands, TX, March 1-5, 2010.
Liang, Y., and Hyodo, R. (2023). Giga-year dynamical evolution of particles around Mars. Icarus391, 115335. doi:10.1016/j.icarus.2022.115335
Lunine, J. I., Neugebauer, G., and Jakosky, B. M. (1982). Infrared observations of Phobos and Deimos from viking. J. Geophys. Res.87 (12), 10297. doi:10.1029/jb087ib12p10297
Marov, M. Y., Avduevsky, V. S., Akim, E. L., Eneev, T. M., Kremnev, R. S., Kulikov, S. D., et al. (2004). Phobos-grunt: Russian sample return mission. Adv. Space Res.33 (12), 2276–2280. doi:10.1016/S0273-1177(03)00515-5
Masursky, H., Batson, R. M., Mccauley, J. F., Soderblom, L. A., Wildey, R. L., Carr, M. H., et al. (1972). Mariner 9 television reconnaissance of Mars and its satellites: Preliminary results. Science175 (4019), 294–305. doi:10.1126/science.175.4019.294
Metzger, P. T., Britt, D. T., Covey, S., Schultz, C., Cannon, K. M., Grossman, K. D., et al. (2019). Measuring the fidelity of asteroid regolith and cobble simulants. Icarus321, 632–646. doi:10.1016/J.ICARUS.2018.12.019
Michel, P., Agnolon, D., Brucato, J., Gondet, B., Korablev, O., Koschny, D., et al. (2011). “Mmsr - a study for a martian moon sample return mission,” in EPSC-DPS Joint Meeting 2011, Nantes, France, 2-7 October 2011, 849.
Miyamoto, H., Niihara, T., Wada, K., Ogawa, K., Baresi, N., Abell, P., et al. (2018). “Phobos environment model and regolith simulant for MMX mission,” in 49th Lunar and Planetary Science Conference, Woodlands, TX, March 19-23, 2018, 1–2.
Moroz, L. v., Basilevsky, A. T., Hiroi, T., Rout, S. S., Baither, D., van der Bogert, C. H., et al. (2009). Spectral properties of simulated impact glasses produced from martian soil analogue JSC Mars-1. Icarus202 (1), 336–353. doi:10.1016/j.icarus.2009.02.007
Mueller, J. T., Guo, Y., Von Mehlem, U. I., and Cheng, A. F. (2003). Aladdin mission concept. Acta Astronaut.52 (2–6), 211–218. doi:10.1016/S0094-5765(02)00159-5
Murchie, S., Arvidson, R., Bedini, P., Beisser, K., Bibring, J. P., Bishop, J., et al. (2007). Compact reconnaissance imaging spectrometer for Mars (CRISM) on Mars reconnaissance orbiter (MRO). J. Geophys. Res. E Planets112 (5), E05S03–57. doi:10.1029/2006JE002682
Murchie, S., and Erard, S. (1996). Spectral properties and heterogeneity of Phobos from measurements byPhobos 2. Icarus123 (1), 63–86. doi:10.1006/icar.1996.0142
Murchie, S. L., Britt, D. T., Head, J. W., Pratt, S. F., Fisher, P. C., Zhukov, B. S., et al. (1991). Color heterogeneity of the surface of Phobos: Relationships to geologic features and comparison to meteorite analogs. J. Geophys. Res. Solid Earth96 (4), 5925–5945. doi:10.1029/90JB02354
Murchie, S. L., Britt, D. T., and Pieters, C. M. (2014). The value of Phobos sample return. Planet. Space Sci.102, 176–182. doi:10.1016/j.pss.2014.04.014
Murchie, S., Thomas, N., Britt, D., Herkenhoff, K., and Bell, J. F. (1999). Mars pathfinder spectral measurements of Phobos and Deimos: Comparison with previous data. J. Geophys. Res. E Planets104 (E4), 9069–9079. doi:10.1029/98JE02248
Murray, J. B., and Heggie, D. C. (2014). Character and origin of Phobos’ grooves. Planet. Space Sci.102, 119–143. doi:10.1016/J.PSS.2014.03.001
Muscatello, A. C., Mueller, R., et al. Muscatello, A. C., Mueller, R., Sanders, G. B., and Larson, W. E. (2012). “Phobos and Deimos sample collection and prospecting missions for science and ISRU,” in Concepts and Approaches for Mars Exploration, Houston, TX, June 12-14, 2012, 4296.
Nakamura, T., Ikeda, H., Kouyama, T., Nakagawa, H., Kusano, H., Senshu, H., et al. (2021). Science operation plan of Phobos and Deimos from the MMX spacecraft. Earth, Planets Space 73, 227–253. doi:10.21203/RS.3.RS-414828/V1
Nallapu, R. T., Dektor, G., Kenia, N., Uglietta, J., Ichikawa, S., Herreras-Martinez, M., et al. (2020). Trajectory design of Perseus: A CubeSat mission concept to Phobos. Aerospace7 (12), 179. doi:10.3390/AEROSPACE7120179
Nayak, M., and Asphaug, E. (2016). Sesquinary catenae on the Martian satellite Phobos from reaccretion of escaping ejecta. Nat. Commun.7 (1), 12591–12598. doi:10.1038/ncomms12591
Nénon, Q., Poppe, A. R., Rahmati, A., and McFadden, J. P. (2021). Implantation of Martian atmospheric ions within the regolith of Phobos. Nat. Geosci.14 (2), 61–66. doi:10.1038/s41561-020-00682-0
Pajola, M., Roush, T., Dalle Ore, C., Marzo, G. A., and Simioni, E. (2018). Phobos MRO/CRISM visible and near-infrared (0.5–2.5 μm) spectral modeling. Planet. Space Sci.154, 63–71. doi:10.1016/j.pss.2018.02.016
Pätzold, M., Andert, T., Jacobson, R., Rosenblatt, P., and Dehant, V. (2014). Phobos: Observed bulk properties. Planet. Space Sci.102 (C), 86–94. doi:10.1016/j.pss.2014.01.004
Pieters, C. M., Murchie, S., Thomas, N., and Britt, D. (2014). Composition of surface materials on the moons of Mars. Planet. Space Sci.102 (C), 144–151. doi:10.1016/j.pss.2014.02.008
Pignatale, F. C., Charnoz, S., Rosenblatt, P., Hyodo, R., Nakamura, T., and Genda, H. (2018). On the impact origin of Phobos and Deimos. III. Resulting composition from different impactors. Astrophysical J.853, 118. doi:10.3847/1538-4357/aaa23e
Pollack, J. B., and Burns, J. A. (1977). An origin by capture for the martian satellites?Bull. Am. Astron. Soc.9, 518–519.
Pollack, J. B., Burns, J. A., and Tauber, M. E. (1979). Gas drag in primordial circumplanetary envelopes: A mechanism for satellite capture. Icarus37 (3), 587–611. doi:10.1016/0019-1035(79)90016-2
Pollack, J. B., Veverka, J., Noland, M., Sagan, C., Hartmann, W. K., Duxbury, T. C., et al. (1972). Mariner 9 television observations of Phobos and Deimos. Icarus17 (2), 394–407. doi:10.1016/0019-1035(72)90007-3
Pollack, J. B., Veverka, J., Pang, K., Colburn, D., Lane, A. L., and Ajello, J. M. (1978). Multicolor observations of Phobos with the viking lander cameras: Evidence for a carbonaceous chondritic composition. Science199, 66–69. doi:10.1126/science.199.4324.66
Ramsley, K. R., and Head, J. W. (2013). Mars impact ejecta in the regolith of Phobos: Bulk concentration and distribution. Planet. Space Sci.87, 115–129. doi:10.1016/J.PSS.2013.09.005
Ramsley, K. R., and Head, J. W. (2021). The origins and geological histories of Deimos and Phobos: Hypotheses and open questions. Space Sci. Rev.217, 86. doi:10.1007/s11214-021-00864-1
Ramsley, K. R., and Head, J. W. (2017). The Stickney Crater ejecta secondary impact crater spike on Phobos: Implications for the age of Stickney and the surface of Phobos. Planet. Space Sci.138, 7–24. doi:10.1016/J.PSS.2017.02.004
Ray, C. S., Reis, S. T., Sen, S., and O’Dell, J. S. (2010). JSC-1A lunar soil simulant: Characterization, glass formation, and selected glass properties. J. Non-Crystalline Solids356 (44–49), 2369–2374. doi:10.1016/j.jnoncrysol.2010.04.049
Raymond, C. A., Diniega, S., and Prettyman, T. H. (2015). “Pandora - discovering the origin of the moons of Mars (a proposed Discovery mission),” in AGU Fall Meeting Abstracts, San Francisco, December 14-18, 2015, 11B–2101B.
Rickman, D., Edmunson, J., and McLemore, C. (2013). Functional comparison of lunar regoliths and their simulants. J. Aerosp. Eng.26 (1), 176–182. doi:10.1061/(ASCE)AS.1943-5525.0000223
Rickman, D., Patel, M. R., Pearson, V. K., Wilson, S., and Edmunson, J. (2016). A carbonaceous chondrite based simulant of Phobos. Earth Space, 576–583. doi:10.1061/9780784479971.054
Ronnet, T., Vernazza, P., Mousis, O., Brugger, B., Beck, P., Devouard, B., et al. (2016). Reconciling the orbital and physical properties of the martian moons. Astrophysical J.828, 109. doi:10.3847/0004-637X/828/2/109
Rosenblatt, P., Charnoz, S., Dunseath, K. M., Terao-Dunseath, M., Trinh, A., Hyodo, R., et al. (2016). Accretion of Phobos and Deimos in an extended debris disc stirred by transient moons. Nat. Geosci.9 (8), 581–583. doi:10.1038/ngeo2742
Rosenblatt, P. (2011). The origin of the Martian moons revisited. Astronomy Astrophysics Rev.19 (1), 44. doi:10.1007/s00159-011-0044-6
Schmedemann, N., Michael, G. G., Ivanov, B. A., Murray, J. B., and Neukum, G. (2014). The age of Phobos and its largest crater, Stickney. Planet. Space Sci.102 (C), 152–163. doi:10.1016/j.pss.2014.04.009
Shi, X., Oberst, J., Willner, K., Shi, X., Oberst, J., and Willner, K. (2016). Mass wasting on Phobos triggered by an evolving tidal environment. GeoRL43 (24), 371–412. doi:10.1002/2016GL071650
Shingareva, T. V., and Kuzmin, R. O. (2001). Mass-wasting processes on the surface of Phobos. SoSyR35 (6), 431–443. doi:10.1023/a:1013082711274
Siddiqi, A. A. (2018). Beyond Earth: A chronicle of deep space exploration. National aeronautics and space administration (NASA), office of communications, NASA history division. Available at: https://www.nasa.gov/connect/ebooks/beyond_earth_detail.html (Accessed September 28, 2018).
Siddiqi, A. A. (2002). Deep space chronicle A chronology of deep space and planetary probes 1958-2000. Monogr. Aerosp. Hist.24, 173–176.
Smith, N. M., Edwards, C. S., Mommert, M., Trilling, D. E., and Glotch, T. D. (2018). “Mapping the thermal inertia of Phobos using MGS-TES observations and thermophysical modeling,” in 49th Lunar and Planetary Science Conference, Woodlands, TX, March 19-23, 2018, 1–2.
Stähler, S. C., Widmer-Schnidrig, R., Scholz, J.-R., Driel, M. V., Mittelholz, A., Hurst, K., et al. (2020). Geophysical observations of Phobos transits by InSight. Geophys. Res. Lett.47 (19), e2020GL089099. doi:10.1029/2020GL089099
Sternovsky, Z., Robertson, S., Sickafoose, A., Colwell, J., and Horányi, M. (2002). Contact charging of lunar and Martian dust simulants. J. Geophys. Res. Planets107 (11), 15-1–15-8. doi:10.1029/2002JE001897
Thangavelautham, J., Asphaug, E., Dektor, G., Kenia, N., Uglietta, J., Ichikawa, S., et al. (2017). “An interplanetary CubeSat mission to Phobos,” in Lunar and Planetary Science Conference, Woodlands, TX, March 20-24, 2017.
Thomas, N., Britt, D. T., Herkenhoff, K. E., Murchie, S. L., Semenov, B., Keller, H. U., et al. (1999). Observations of Phobos, Deimos, and bright stars with the imager for Mars pathfinder. J. Geophys. Res. E Planets104 (4), 9055–9068. doi:10.1029/98JE02555
Thomas, N., Stelter, R., Ivanov, A., Bridges, N. T., Herkenhoff, K. E., and McEwen, A. S. (2011). Spectral heterogeneity on Phobos and Deimos: HiRISE observations and comparisons to Mars pathfinder results. Planet. Space Sci.59 (13), 1281–1292. doi:10.1016/j.pss.2010.04.018
Thomas, P. C., Veverka, J., Bloom, A., and Duxbury, T. C. (1979). Grooves on Phobos: Their distribution, morphology and possible origin. J. Geophys. Res.84, 8457–8477. doi:10.1029/JB084iB14p08457
Ulamec, S., Michel, P., Grott, M., Böttger, U., Hübers, H., Murdoch, N., et al. (2019). “A rover for the JAXA MMS mission to Phobos,” in 70th International Astronautical Congress, Mmx, Washington, DC, October 21 – 2, 2019, 1–8.
Veverka, J., and Duxbury, T. C. (1977). Viking observations of Phobos and Deimos: Preliminary results. J. Geophys. Res.82 (28), 4213–4223. doi:10.1029/JS082i028p04213
Warner, N., Gupta, S., Lin, S.-Y., Kim, J.-R., Muller, J.-P., and Morley, J. (2010). Late Noachian to Hesperian climate change on Mars: Evidence of episodic warming from transient crater lakes near Ares Vallis. J. Geophys. Res. Planets115 (6), E06013. doi:10.1029/2009JE003522
Witasse, O., Duxbury, T., Chicarro, A., Altobelli, N., Andert, T., Aronica, A., et al. (2014). Mars express investigations of Phobos and Deimos. Planet. Space Sci.102, 18–34. doi:10.1016/j.pss.2013.08.002
Keywords: Mars, Phobos, regolith, minerals, space missions
Citation: Miranda C, Patel M, Berberan-Santos MN, Hormigo T, Correia ACM, Pedras B and Martins Z (2023) The importance of Phobos simulants: a review on our current knowledge. Front. Astron. Space Sci. 10:1130743. doi: 10.3389/fspas.2023.1130743
Received: 23 December 2022; Accepted: 09 June 2023;
Published: 19 June 2023.
Edited by:
Konstantinos Dialynas, Academy of Athens, GreeceReviewed by:
Ryuki Hyodo, Japan Aerospace Exploration Agency (JAXA), JapanAkos Kereszturi, Hungarian Academy of Sciences (MTA), Hungary
Copyright © 2023 Miranda, Patel, Berberan-Santos, Hormigo, Correia, Pedras and Martins. This is an open-access article distributed under the terms of the Creative Commons Attribution License (CC BY). The use, distribution or reproduction in other forums is permitted, provided the original author(s) and the copyright owner(s) are credited and that the original publication in this journal is cited, in accordance with accepted academic practice. No use, distribution or reproduction is permitted which does not comply with these terms.
*Correspondence: Zita Martins, eml0YS5tYXJ0aW5zQHRlY25pY28udWxpc2JvYS5wdA==
†These authors share senior authorship