- 1NASA, Marshall Space Flight Center, Huntsville, AL, United States
- 2Center for Space Plasma and Aeronomic Research, University of Alabama in Huntsville, Huntsville, AL, United States
- 3Lockheed Martin Solar and Astrophysics Laboratory, Palo Alto, CA, United States
- 4Bay Area Environmental Research Institute, NASA Research Park, Petaluma, CA, United States
- 5Indian Institute of Astrophysics, Koramangala, Bangalore, India
Solar coronal jets are frequently occurring collimated ejections of solar plasma, originating from magnetically mixed polarity locations on the Sun of size scale comparable to that of a supergranule. Many, if not most, coronal jets are produced by eruptions of small-scale filaments, or minifilaments, whose magnetic field reconnects both with itself and also with surrounding coronal field. There is evidence that minifilament eruptions are a scaled-down version of typical filament eruptions that produce solar flares and coronal mass ejections (CMEs). Moreover, the magnetic processes building up to and triggering minifilament eruptions, which is often flux cancelation, might similarly build up and trigger the larger filaments to erupt. Thus, detailed study of coronal jets will inform us of the physics leading to, triggering, and driving the larger eruptions. Additionally, such studies potentially can inform us of smaller-scale coronal-jet-like features, such as jetlets and perhaps some spicules, that might work the same way as coronal jets. We propose a high-resolution (
1 Introduction
Solar coronal jets are transient phenomena that originate near the solar surface and extend into the corona in the form of long and narrow spires. They are visible at soft X-ray (SXR) and EUV wavelengths, and occur in coronal holes, quiet Sun, and the periphery of active regions. While there were some scattered earlier observations, coronal jets were first observed in detail in SXRs with the Yohkoh satellite (Shibata et al., 1992), and they have since been observed with several instruments in different wavelengths. (Surges, which traditionally have been observed in the chromosphere, share some properties with coronal jets, and in some cases accompany coronal jets; e.g., Canfield et al., 1996; Moore et al., 2010; 2013; Sterling et al., 2016.) In coronal images, coronal jets consist of a spire that emanates from a bright base region and extends into the corona. From a study of SXR coronal jets in polar coronal holes (Savcheva et al., 2007), coronal jets live for tens of minutes, and have an occurrence rate of about 60/day in the two polar coronal holes, which translates to about one per hour in a given polar coronal hole. That same study reported that the coronal-jet spires reach ∼50,000 km, and have widths ∼10,000 km. Coronal jets seem to have two speeds, or two components of different speeds; a slower speed of ∼200 km s−1, which is close to the local sound speed, and a faster speed of ∼1,000 km s−1, which is near the Alfvén speed (Cirtain et al., 2007). It had been pointed out much earlier (Shibata et al., 1992) that the base region of the coronal jet often has a particularly bright spot in the coronal-jet base, and that brightening is offset to one side of the base. Several general summaries and reviews of coronal jets have come out during different eras of coronal-jet studies (Shimojo and Shibata, 2000; Shibata and Magara, 2011; Raouafi et al., 2016; Hinode Review Team et al., 2019; Shen, 2021; Sterling, 2021; Schmieder, 2022).
It has been argued by Sterling et al. (2015) that most or all coronal jets result from the eruption of small-scale filaments, or minifilaments (being several times to orders-of-magnitude smaller than “typical” large-scale filaments, that erupt to make typical solar flares and coronal mass ejections), based on their study of 20 polar coronal hole jets, and building on several earlier studies of coronal jets (Nisticò et al., 2009; Moore et al., 2010, 2013; Hong et al., 2011; Huang et al., 2012; Shen et al., 2012; Adams et al., 2014). Sterling et al. (2015) proposed that the minifilament eruption and resulting coronal jet are scaled-down versions of typical filament eruptions that produce solar flares and coronal mass ejections (CMEs).
Figure 1 shows an example of a coronal jet, occurring in the south polar coronal hole. Panel 1 (a) shows the coronal jet when it is well developed, in a SXR image from the Hinode spacecraft. The blue arrow points to the spire, and the green arrow points to the base brightening. We call the latter a JBP for the “jet bright point” in the coronal-jet’s base (this terminology is from Sterling et al., 2015). Panels 1 (b)—1 (d) show the same location in EUV images from the SDO/AIA instrument’s 193 Å channel. Panel 1(b) shows the situation prior to the start of the coronal jet. Panel 1 (c) is from about the same time as the SXR image in 1 (a). It shows the spire less prominently than in SXRs at that moment, but it also shows a minifilament (in absorption) in the process of erupting outward (yellow arrow). These erupting minifilaments usually are, at best, only hinted at in SXR images, but are often clearly visible in at least one and often in several AIA EUV channels (Sterling and Moore, 2023). In 1 (d), this minifilament has continued to erupt, with portions of it leaking out into the bright spire.
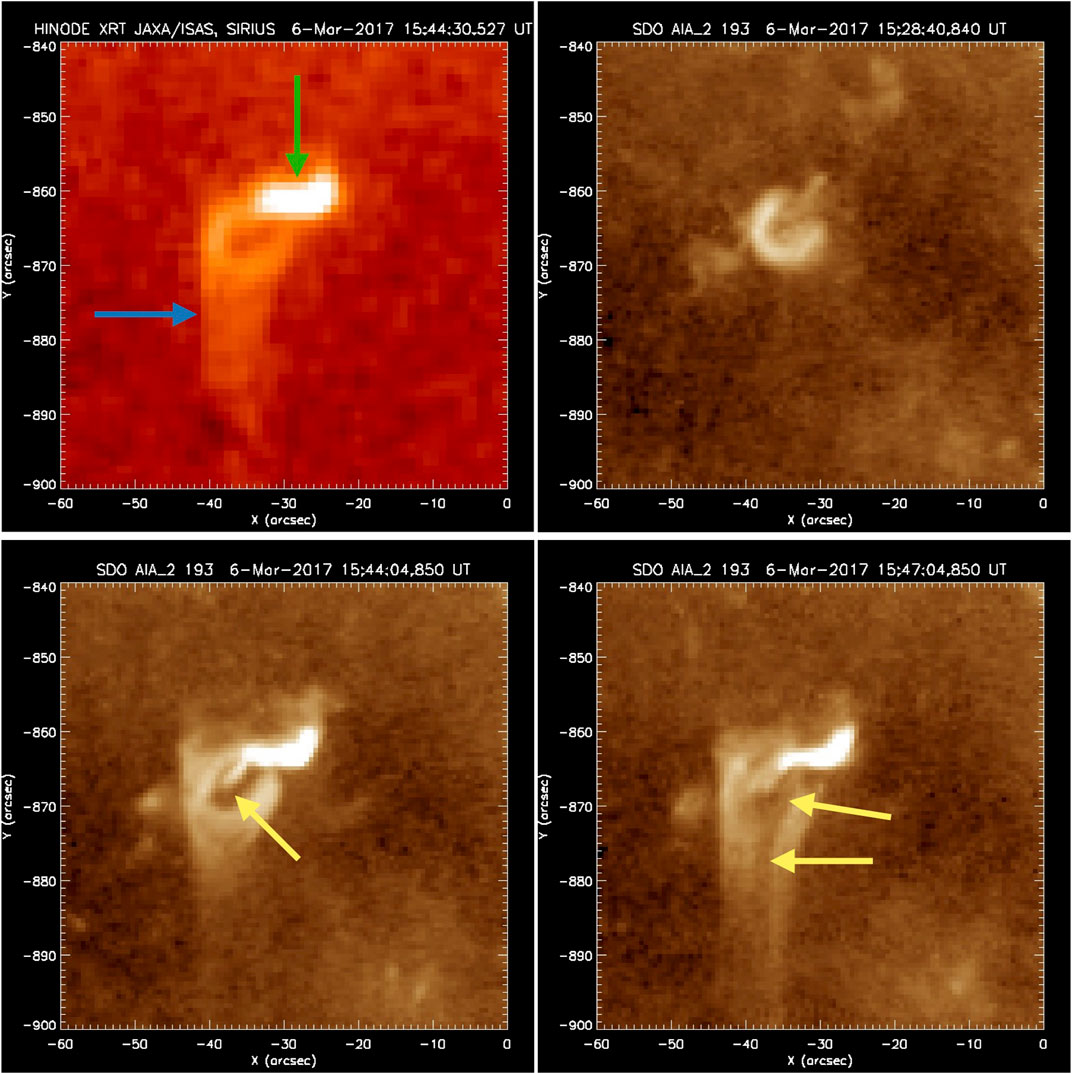
FIGURE 1. Example of a coronal jet, occurring on 2017 March 6. (a) Hinode/XRT soft X-ray image of the coronal-jet spire (blue arrow) and the jet bright point (“JBP”) brightening in the coronal-jet’s base (green arrow). North is upward and west is to the right in this and all solar images in this paper. This coronal jet is in the south polar region, and hence the spire is pointing downward.
Figure 2 shows the scenario proposed by Sterling et al. (2015) to explain coronal jets. This shows a coronal-hole region: most of the photospheric magnetic flux has the same polarity (negative in this case), and the ambient coronal field is open. While this drawing is tailored for a coronal hole region, the same description holds where the ambient coronal field is a long loop (compared to the size of the base region) instead, which would be common in quiet Sun and active regions. A positive-polarity patch is present in the region, and this forms an anemone-type structure (Shibata et al., 2007), with flux emanating out of the positive patch and closing down in negative flux surrounding the positive patch in 3-D, where Figure 2A shows a 2-D cross-section of this structure. One lobe of the anemone - the smaller lobe on the right-hand side (i.e., the closed-field region between B and C in Figure 2) in this depiction - contains non-potential (sheared and twisted) field, and holds a minifilament. The adjacent lobe on the left side is larger and contains more-nearly potential field. Figure 2B shows the minifiament, and its enveloping flux-rope field, erupting. This results in two magnetic reconnections. One reconnection occurs where the erupting-minifilament’s field encounters the ambient coronal field above the larger lobe. This adds new, heated loops to the large lobe, and creates new open field, along which heated material can flow out and create the coronal-jet spire. The second reconnection occurs among the leg field of the erupting flux-rope-enveloping field. This causes a miniature flare to occur, in a fashion analogous to how typical solar flares are formed (e.g., Hirayama, 1974; Shibata et al., 1995; Moore et al., 2001). This miniature flare is what appears as the aforementioned JBP. In Figure 2C, the erupting field has reconnected far enough into the open-field region for the cool minifilament material in the flux-rope core of the erupting field to leak out onto the open field, where it flows away as part of the spire.
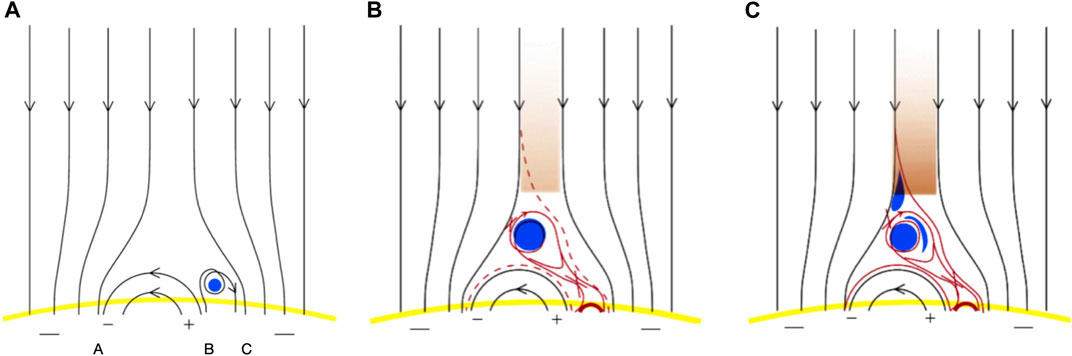
FIGURE 2. Schematic showing the minifilament-eruption mechanism for coronal jets, from Sterling et al. (2015) (this slightly modified version of the original figure appeared in Sterling et al., 2018). This is a two-dimensional cross-sectional cut of the jetting region, with the yellow curve representing the Sun’s limb. Black lines represent magnetic field lines prior to reconnection, with the arrow heads and the “+” and “−” signs indicating polarities. Red lines indicate reconnected field lines, with the dotted red lines indicating that the field lines are newly reconnected. The blue circle indicates cool minifilament material, with the circular field surrounding it being the minifilament’s field. Panel (A) shows the situation prior to eruption. “A,” “B,” and “C” indicate locations for reference. In (B) the minifiament’s field is erupting, carrying with it the cool minifilament material. Red X-es show reconnections, with the one below the erupting minifilament being flare-like reconnection (called “internal” reconnection, being internal to the erupting flux-tube’s lobe field; Sterling et al., 2015) resulting in the jet bright point (JBP), represented by the thick-red simicircle between the B and C locations. The reconnection in front of (to the left and slightly above the center of) the erupting minifilament (“external” reconnection) results in new open field, along which heated plasma flows to form the coronal jet’s spire (shaded region). This reconnection also adds new heated closed field above the magnetic lobe between locations A and B. In (C) the higher-height reconnection has proceeded enough that some of the cool minifilament material is flowing out onto the spire on newly reconnected open field.
Studies of coronal jets occurring on the solar disk have provided insight into the magnetic origins of the coronal-jet-producing minifilament eruptions. Panesar et al. (2016b) found that magnetic cancelation occurred at the coronal-jet location before and during the coronal jet in 10 quiet Sun jets, and in a similar study Panesar et al. (2018a) found magnetic cancelation occurring near the start of 13 coronal hole jets. Additional multiple-coronal-jet studies support these findings, including McGlasson et al. (2019) and Muglach (2021). A study of (Kumar et al., 2019) argues that “shearing and/or rotational photospheric motions” are more important than cancelation in producing coronal-hole jets that they studied; they do find, however, evidence that minifilament eruptions produce about two-thirds of the coronal jets, and that small-scale eruptions without cool-minfilament material cause the remaining ones. Magnetic cancelation has also been found to accompany many active region jets (Sterling et al., 2016; Sterling et al., 2017; Mulay et al., 2016 state that cancelation, flux emergence, or cancelation-plus-emergence produce the active-region coronal jets they observe). Single-event studies have also found cancelation to accompany coronal jets in many cases. There are several other observational examples of magnetic flux cancelation leading to minifilament eruptions that produce coronal jets (e.g., Mazumder, 2019; Solanki et al., 2019; Yang et al., 2019). See the above-mentioned reviews for additional citations.
Observations support that flux cancelation at the neutral line labeled “B” in Figure 2A often triggers the minifilament to erupt. Similarly, magnetic flux cancelation also likely often builds the minifilament prior to eruption, in some cases hours to ∼two days prior to its eruption (Panesar et al., 2017). If the minifilament has twist on it, perhaps supplied when canceling magnetic elements themselves contain shear derived from photospheric motions, that twist can be supplied to the coronal-jet’s spire upon eruption of that minifilament and its reconnection with the coronal field, explaining why many coronal-jet spires display a spinning motion during their evolution (e.g., Moore et al., 2015).
2 Some coronal-jet-observing instruments
We briefly introduce instruments often used for coronal-jet studies over recent decades. Here we describe those instruments that are referred to most in this paper. See the reviews listed in §1 for discussions of other instruments used in coronal jet observations.
In SXRs, coronal jets were first extensively observed with the Yohkoh/Soft X-ray Telescope (SXT; Tsuneta et al., 1991), which operated from 1991 to 2001. It had a detector with square pixels of width 2″. 455, and variable cadence with the fastest being about 2 s, although it often ran with much coarser cadence. Its followup was the X-ray Telescope (Golub et al., 2007) on the Hinode satellite, launched in 2006, and still operating as of this writing. It has pixels of width 1″. 02, and—for observations most appropriate for coronal jets—operated with a cadence of ∼30 s. Both SXT and XRT imaged with variable field of view (FOV), although for cadences sufficient to observe coronal jets of ∼10-min lifetime both instruments used a FOV smaller than that of the full solar disk.
With Yohkoh/SXT, a large percentage of observed coronal jets occurred in active regions, with very few seen in polar regions (Shimojo et al., 1996). Koutchmy et al. (1997) did see some polar coronal hole jets with SXT, but only with relatively long exposures of 15 and 30 s. In contrast, coronal jets are very prominent and common in polar regions in Hinode/XRT observations (Cirtain et al., 2007). As discussed in Hinode Review Team et al. (2019) (in the subsection on coronal jets), this difference in visibility between the two SXR-imaging instruments can be understood because the filters that see the coolest SXR plasma with Yohkoh/SXT had sensitivity that dropped off sharply below about 2 MK, while the coolest filters of Hinode/XRT have good sensitivity to plasmas of down to just under 1 MK. From filter-ratio temperature studies, Nisticò et al. (2011), Pucci et al. (2013), and Paraschiv et al. (2015) determined that polar-coronal-jet spires have temperatures of ∼1—2 MK. Therefore this could explain why they are easily visible in images from the cooler-temperature-detecting Hinode/XRT, but much-less visible or invisible in images from the hotter-temperature-detecting Yohkoh/SXT.
For EUV observations, although there were some earlier useful observations with the EUV Imager (EUVI) telescope on the STEREO spacecraft (Nisticò et al., 2009, 2010), the results discussed in the present paper largely derive from the Atmospheric Imaging Assembly (AIA; Lemen et al., 2012) on the Solar Dynamics Observatory (SDO) satellite, which has been operational from 2010, and is still operational as of this writing. It has a detector of 0″. 6 pixels, and regularly observes the entire solar disk with 12 s cadence in seven EUV channels centered at 304, 171, 193, 211, 131, 335, 94 Å, roughly in order from detectability of the coolest to the hottest plasmas for non-flaring situations (the details of the ordering depend on the distribution of temperatures in the emitting plasmas, and also some channels have good response in multiple temperature ranges; Lemen et al., 2012 gives the AIA response curves and principle ions contributing to each wavelength band). Sterling et al. (2015) found that polar coronal hole jets are best visible in the first four of these channels, and that the hotter channels of 131, 335, and 94 Å added little new information. Active region jets, which tend to be hotter than the polar coronal hole jets, generally are well seen over a broader range of AIA channels. Shimojo and Shibata (2000) found active region jets to have temperatures 3—8 MK, based on SXT filter-ratio methods, and later studies have also found active region jet temperatures in this range (Paraschiv et al., 2022).
Several papers by Mulay et al. (2016, 2017b,a) use the EUV Imaging Spectrometer (EIS) on Hinode to undertake spectroscopic studies of coronal jets, and survey a broader temperature range than that of the SXR filter-ratio methods of the just-mentioned studies. They report the bulk of the emission of active-region-jet spires to be of temperatures ≲1—2MK. This is substantially lower than the active-region-jet temperatures from the filter ratios mentioned above, e.g., the 3—8 MK of Shimojo and Shibata (2000). But this difference is likely due to the nature of the plasmas that the respective instruments can detect. EIS, being an EUV spectrometer, has spectral coverage of substantially cooler spectral lines than those contributing to the SXR emission. Thus it is likely that there is a wide distribution of plasma temperatures in coronal jets, and–not surprisingly–the SXR telescopes preferentially detect the hotter plasmas in those coronal jet spires, and therefore yield higher temperatures for coronal jets than the bulk of the coronal-jet plasmas detected by EIS.
Spectroscopy in the UV from IRIS has provided valuable insight into coronal jets. This includes studies finding rotational (spinning) motion in coronal jets (e.g., Cheung et al., 2015; Liu et al., 2018; Ruan et al., 2019; Schmieder et al., 2022), confirming indications of such rotation from earlier observations in EUV (Pike and Mason, 1998). These spectra also provide information on densities in coronal jets (e.g., Cheung et al., 2015; Mulay et al., 2017b; Panesar et al., 2022). Moreover, the high resolution of the IRIS slitjaw images can complement the EUV and SXR coronal-jet observations in, for example, zeroing in on the fine-scale structure and dynamics at the coronal-jet magnetic-source location in the photosphere (Sterling et al., 2017).
Magnetograms from the SDO Helioseismic and Magnetic Imager (HMI; Scherrer et al., 2012) are frequently used to study the photospheric magnetic flux values and changes around the base of coronal jets. HMI has pixels of 0″. 5, and takes a line-of-sight magnetogram of the full solar disk once every 45 s.
3 Coronal jets and jet-like activity on different size scales
3.1 Coronal-jet physics on large scales
Coronal jets appear to be small-scale versions of larger eruptions, with the eruptive process that results in a minifilament eruption that produces a coronal-jet spire and a JBP corresponding to large-scale eruptions that result in filament eruptions and typical solar flares (Sterling et al., 2015). That is, just as “typical” solar filaments erupt (in what we are here calling “large-scale eruptions”) to make long-observed “typical” solar flares and that sometimes expel coronal mass ejections into the heliosphere, coronal-jets appear to be made by a minifilament eruption (a scaled-down version of a large-scale filament eruption) that leaves in its wake a JBP (a scaled-down version of a typical solar flare), and to result in material and magnetic disturbances that flow out along a spire and that sometimes flow into the heliosphere.
If coronal jets are indeed a scaled-down version of larger “standard flare model” solar eruptions, then we would expect other aspects of the smaller-scale eruptions that cause coronal jets and JBPs to have counterparts in the larger-scale eruptions that cause CMEs. Here we discuss examining large-scale eruptions based on what has been found in coronal jets.
A characteristic of coronal jets is the anemone magnetic setup, similar to that shown in Figure 2A. There are many examples of flares occurring from anemone active regions (Asai et al., 2009; Lugaz et al., 2011; Kumar and Manoharan, 2013; Devi et al., 2020). Joshi et al. (2017) showed that the setup for a large-scale eruption matched that of the coronal-jet minifilament-eruption picture, and that the dynamic motions of the eruption matched closely that of an erupting minifilament producing a coronal jet. A similar schematic was in fact drawn to explain a series of recurring solar eruptions much earlier (Sterling and Moore, 2001a, Sterling and Moore, 2001b; Sterling et al. 2001; these schematics in fact helped inspire the Figure 2 schematic of Sterling et al., 2015). These setups show that the same type of magnetic setup appears to be capable of generating similar solar expulsions both on the coronal-jet size scale, and on the size scale of typical solar eruptions. Whether a coronal jet results or a CME results depends on how much of the erupting minifilament/flux-rope lobe remains after the external reconnection in Figures 2B, C. If the flux rope is robust enough to survive that reconnection (that is, if only the outer portion of the flux-rope lobe is eroded away by external reconnection), then the remaining lobe and flux rope can escape to form a CME that carries a magnetic flux rope in its core region. If on the other hand the external reconnection totally reconnects the flux rope, so that the field lines that were previously closed in a flux rope all become open, then the feature becomes a coronal jet instead of a CME.
An anemone setup appears to be necessary for coronal-jet formation, and formation of coronal jets in such a setup is supported by numerical simulations (Wyper et al., 2017, 2018a; b; Wyper et al., 2019; Doyle et al., 2019). But large-scale eruptions also occur outside of an anemone setup, and so we might ask whether the eruptions of minifilaments that cause coronal jets might also have similarities to larger-scale eruptions, independent of whether those larger-scale eruptions occur in an anemone configuration. One possible such similarity is in the manner in which the minifilament eruptions and the large-scale eruptions are triggered to erupt. We have seen above that coronal-jet-producing erupting minifilaments are apparently often built-up and triggered to erupt by magnetic-flux cancelation. What about larger-scale eruptions?
To investigate whether large-scale eruptions are built up and triggered to erupt in a manner similar to coronal-jet-producing minifilament eruptions, Sterling et al. (2018) studied how large-scale eruptions evolve toward eruption. In the case of coronal jets, the magnetic elements taking part in the cancelation typically converge toward each other over the hours prior to the eruption onset, as discussed in several papers (Panesar et al., 2016b, 2017; 2018a; Sterling et al., 2017), and as exemplified by Figure 3C. This time period is short enough for those elements to have relatively little interaction with surrounding flux elements. In contrast, large-scale eruptions often occur in active regions that develop for many days, or even weeks, prior to expelling an eruption (complex regions, such as delta regions, can evolve faster than this, but the objective of Sterling et al., 2018 was to compare with more standard eruptions). Thus, in order to follow the region from the time of flux emergence through to the time of the eruption, it was necessary to look at regions that were small enough for this evolution to occur during a single disk passage of the region. Sterling et al. (2018) presented two examples of this class. In both cases the active regions were comparatively small bipolar active regions (total flux in each ≲ 1021 Mx). Also in both cases the eruptions occurred about five days after emergence, and those eruptions produced CMEs observed in coronagraphs. One of the regions remained almost completely isolated from any surrounding substantial flux over this period. And the second (shown in Figure 4) was largely isolated from surrounding flux, although one of its polarities did have some interaction with nearby pre-exisiting opposite-polarity flux.
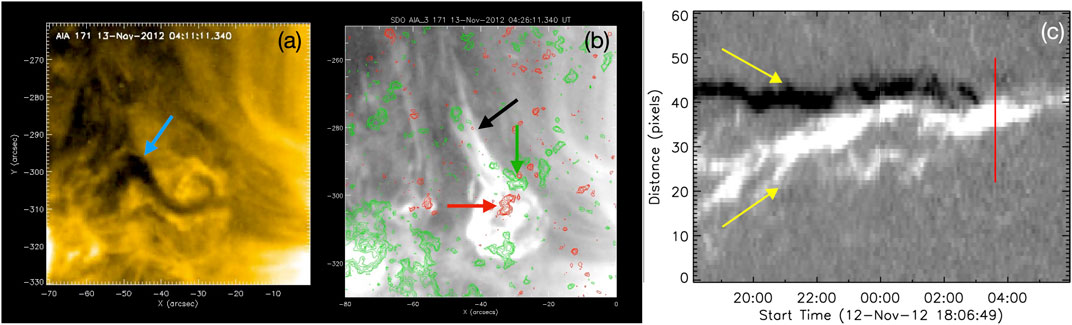
FIGURE 3. Observations of a coronal jet originating from an on-disk location, on 2012 November 13, from Panesar et al. (2016b). Panel (A) is an SDO/AIA 193 Å image, showing a minifilament (blue arrow) starting to erupt to produce the coronal jet. Panel (B) shows an AIA 193 Å image (grey scale) 15 min later, where the minifilament has erupted and generated the coronal jet, with the black arrow pointing to the spire. Overlaid is magnetic flux from an SDO/HMI magnetogram, with red/green contours indicating positive/negative polarities. Red and green arrows point to two polarity elements highlighted in the next panel. Panel (C) shows the time evolution of those positive-polarity (white) and negative-polarity (black) fluxes in a time-distance map, with the vertical red line indicating the time of coronal-jet occurrence. This shows the polarities converging and undergoing cancelation near the time of the coronal-jet’s onset.
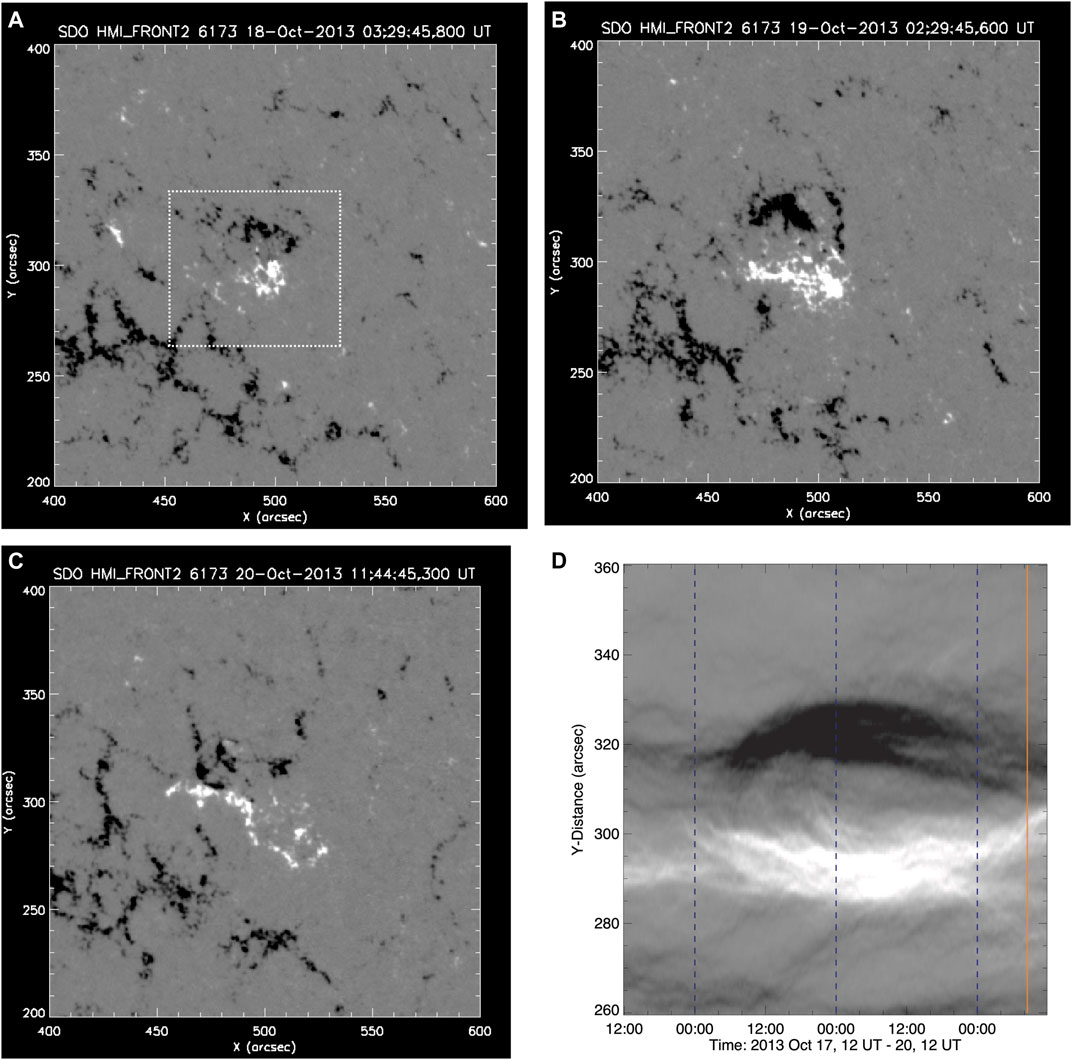
FIGURE 4. SDO/HMI magnetograms showing the evolution of a small-sized active region, NOAA AR 11868, which started emerging on 2013 October 16, and which produced a C-class flare and expelled a CME four days later on October 20. (A) The boxed region shows the emerging bipolar region about two days after the start of emergence. Black/white indicate negative/positive magnetic polarities. (B) The main negative and positive patches of the emerging region continue to grow and spread apart. (C) Portions of the opposite-polarity patches have converged on each other, and mangnetic cancelation is occurring between them. (D) A time-distance plot showing evolution of the two polarity patches of the region. This plot is formed by summing fluxes in the horizontal direction across the box in (A), and the y-axis of this panel is a sub-portion of the y-axis of panels (A–C). (From Sterling et al., 2018.)
Both regions displayed similar evolution. Figure 4 shows the evolution of one of these regions. The boxed region in (a) shows a bipolar active region that is still emerging in this frame. In panel (b) the emergence is continuing, with centroids of the main positive-polarity (white) and negative-polarity (black) patches further separated from their central neutral line than they were in (a). By the time of (c) however, they are no longer separating, and some of the opposite-polarity portions of the region have converged toward the central neutral line. Panel (d) shows a time-distance map of this region, analogous to that in Figure 3C. This shows that the polarities initially separate for about one day following their initial emergence. Their mutual directions then reverse, and the polarities start to converge. The orange line shows on this plot the time of the CME-producing eruption; this did not occur until after the polarities had converged on each other, and were undergoing flux cancelation along their central neutral line. There were no CME-producing eruptions from this region prior to this time. SDO/AIA observations show that the bright centroid of the resulting GOES C-class flare was on that neutral line. Thus, similar to the situation with coronal jets, a flux rope eruption occurs along a cancelation neutral line. In this case, the region evolved for about four days with essentially no activity, and then had an eruption only after that cancelation started taking place.
A second region examined in Sterling et al. (2018), which also was substantially isolated magnetically from surrounding structures, began with flux emergence, underwent flux-polarity separation, and then had flux convergence and apparent cancelation along its central neutral line among some portion of its two opposite-polarity patches. This resulted in a eruption that produced a GOES B-class flare on the region’s central neutral line (although in this case a second, weaker, eruption also occurred on a neutral line formed from one of the emerging polarities and a pre-existing opposite polarity patch), and in the expulsion of a CME.
Chintzoglou et al. (2019), studying more complex magnetic situations involving multiple active regions, also found that eruptions occurred at flux-cancelation neutral lines.
Returning to the discussion of the size scale of the erupting minifilaments that can cause coronal jets, Moore et al. (2022) examined the evolution of 10 bipolar ephemeral active regions (BEARs) in a manner similar to Sterling et al. (2018), but where they tracked their regions from emergence to disappearance. These 10 regions produced 43 small-scale eruptions in total, and all of these eruptions occurred at a neutral line in which apparent flux cancelation was taking place. This again supports that the physics that causes eruptions on the coronal-jet size scale is essentially the same as that which causes large-scale eruptions that produce typical solar flares and CMEs.
These observations strongly support that flux cancelation is often essential in the magnetic build-up and triggering of both smaller-scale eruptions that cause coronal jets, and larger-scale eruptions that cause flares and CMEs. This is fully consistent with the mechanism for the build-up of the non-potential energy required for eruption via flux cancelation, as suggested by van Ballegooijen and Martens (1989). Observations of these processes occurring on faster time scales in the smaller-size-scale jets, however, helps to elucidate strategies for investigating the processes in the more-slowly developing larger active regions.
3.2 Possible extensions to smaller scales
From the preceding discussions, we have presented evidence that the same basic process leading to eruptions occurs on two size scales–that of large-scale eruptions and that of coronal jets. Sterling and Moore (2016) considered a possible extended relationship to smaller size scales, by plotting the size of a typical filament or filament-like structure that erupts on one axis, and, on the other axis, a measure of the number of the respective eruptive events occurring at any given time on the Sun. Their motivation was to see whether a substantial number of similar features might occur on a spicule size scale, assuming that the coronal-jet mechanism continues to scale downward to sufficiently small sizes. If so, then it might be that at least some spicules (and perhaps many or most spicules) result from erupting filament-like features (erupting flux ropes) of that size scale. (See §3.4 for a summary of spicule properties).
The larger of the two size scales of eruptive events that have observed filament eruptions are the “typical” filament eruptions that have solar flares occurring beneath them, and–in the case of ejective eruptions–expulsion of a CME. Sterling and Moore (2016) took a typical eruptive filament size to be 70,000 km, with an appropriate scatter based on observed values for a large number of filaments by Bernasconi et al. (2005). For the number of large-scale eruptions, they took observed CME rates of from less than one to a few per day (Yashiro et al., 2004; Chen, 2011). For coronal jets, they estimated corresponding numbers for the size of erupting minifilaments based on measurements in Sterling et al. (2015), which on average was just over 5,000 km for the erupting-minifilament lengths. For the frequency of eruptions they extrapolated rates given in Savcheva et al. (2007), which yields about a few hundred per day over the entire Sun.
In order to compare with spicule occurrence rates, Sterling and Moore (2016) converted the occurrence rates for the large-scale eruptions and coronal-jet-size-scale minifilament eruptions to the expected number of events occurring on the Sun at any random given time. The motivation for these units was to utilize the historical studies of spicule counts, which were sometimes expressed as the total number of spicules on the Sun seen at a given time. Those resulting values vary substantially (Athay, 1959; Lynch et al., 1973), but overall they are roughly in the neighborhood of 106 spicules on the Sun at a given time (Judge and Carlsson, 2010 estimate about a factor of ten higher; see Sterling et al., 2020a).
In order to complete the comparison with the larger erupting features, a value for the typical size of the erupting filament-like flux rope that would produce a spicule is required. No such cool-material eruptions have been convincingly observed to date, and therefore spicules being formed by the coronal-jet-producing minifilament-eruption-type mechanism is wholly speculative at this point. But because coronal-jet-spire widths are similar to the measured lengths of the erupting minifilaments that produce them, by analogy, Sterling and Moore (2016) hypothesized that there might be erupting microfilaments (erupting micro flux ropes) of lengths comparable to the width of spicules (a few hundred km), that produces some spicules.
Figure 5 shows the resulting plot. When a linear extension is made from the large-scale eruptions through the coronal-jet-sized eruptions, and then extended down to the size scale of the putative erupting microfilaments, the ordinate’s value for their occurrence rate falls near the lower end of the estimated spicule-number counts. This implies that at least some portion of spicules might be scaled-down versions of coronal jets, formed by eruptions of microfilaments, or it could be that multiple spicules are produced by a single such eruption.
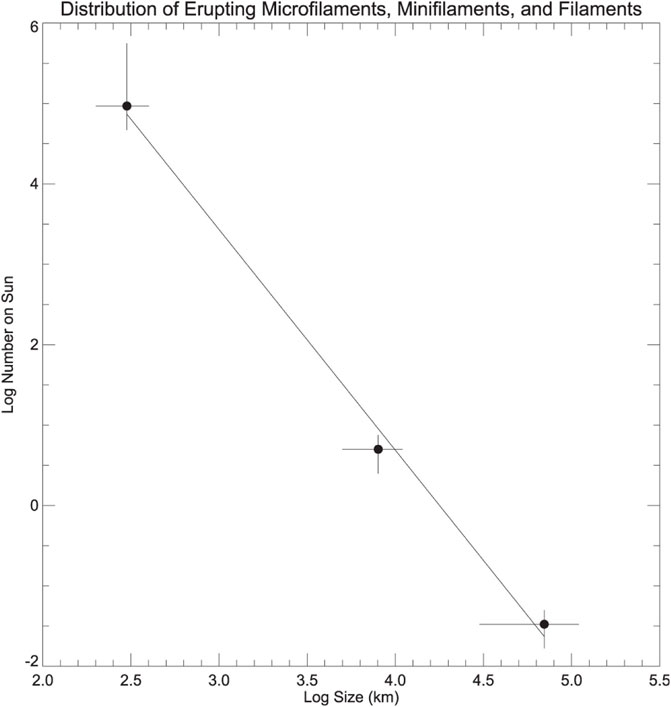
FIGURE 5. Plot showing the instantaneous number distribution of filament-like eruptive events on the Sun, where those eruptions plausibly all operate by the same general eruptive mechanism as that for coronal jets pictured in Figure 2. The horizontal axis is the size of the erupting cool-material feature, and the vertical axis shows an estimate of the number of eruptions of those features occurring on the Sun at any given time. There are only three points plotted. The right-most point represents large-scale eruptions of typical-sized filaments that produce solar flares, and CMEs in the case of ejective eruptions. The middle point represents coronal jets, such as shown in Figures 1, 3, and which are made by minifilament eruptions as depicted in Figure 2. The leftmost point is for speculative “erupting microfilaments” (erupting microfilament-carrying flux ropes) of the size scale of spicules, and which might produce spicules or spicule-like features in the manner of Figure 2. “Error” bars represent uncertainties in the observational parameters. This plot demonstrates the plausibility that spicules might fit on the same power-law distribution as large-scale eruptions and the minifilament eruptions that make jets, but much more evidence is required before a firm conclusion can be make regarding whether some or most spicules are generated in a manner analogous to how coronal jets are made. This plot is from Sterling and Moore (2016); see that paper for further details.
We next consider from an observational standpoint the suggestion that the coronal-jet-producing mechanism operates on size scales smaller than that of coronal jets.
3.3 Jetlets
Raouafi and Stenborg (2014) studied long and narrow transient features of a scale smaller than coronal jets, using SDO/AIA images and HMI magentograms. They called these features jetlets, due to their similarity to coronal jets, except having a smaller size (both width and length). Jetlets are smaller than coronal jets, but larger than chromospheric spicules. Raouafi and Stenborg (2014) found the jetlets to occur at the base of coronal plumes, and they suggest that they (along with transient base brightenings) are the result of “quasi-random cancellations of fragmented and diffuse minority magnetic polarity.” Therefore, these features seem to be smaller versions of coronal jets. (“Plumes” are long and narrow features–first noticed during total eclipses–extending out to several solar radii in polar regions. Compared to jet-like features, plumes are long-lasting, persisting for ∼day. See, e.g., Poletto, 2015).
Panesar et al. (2018b) examined jetlets with IRIS UV and AIA EUV images, and HMI magnetograms. They studied ten jetlets, and found them to have lengths ∼27,000 km, spire widths ∼3,000 km, base size of ∼4,000 km and speed ∼70 km s−1. They argued that jetlets are a more general solar feature than presented in Raouafi and Stenborg (2014), occurring at the edges of chromospheric network both inside and outside of plumes. In agreement with Raouafi and Stenborg (2014), they found that magnetic flux cancelation was the likely cause of the jetlets, just as it often leads to coronal jets. Furthermore, their jetlets were accompanied by brightenings analogous to the JBP seen at the base of coronal jets. Based on these and other characteristics, Panesar et al. (2018b) concluded that the jetlets are likely scaled-down version of coronal jets, and that they are consistent with the erupting-minifilament scenario for their production. They did not, however, observe a clear indication of the existence of an actual cool-material erupting minifilament at the base of their jetlets.
Panesar et al. (2019) extended these studies to even smaller-sized jetlets, using EUV 172 Å (Fe ix/Fe x) images from the Hi-C 2.1 rocket flight. This instrument had pixels of width 0″. 129, and cadence of 4.4 s; see Rachmeler et al. (2019) for details. Six events were identified from the data from Hi-C 2.1’s 5-min flight. As with the Panesar et al. (2018b) jetlet study, these events also occurred at the edges of network cells. On average they had spire lengths of ∼9,000 km, widths of 600 km, and speeds of ∼60 km s−1. At least four of these events seemed consistent with being small-scale coronal jets following the erupting-minifilament mechanism, although once again there were no direct observations of erupting cool-material minifilaments.
3.4 Spicule-sized features
Spicules are chromospheric features that are extremely common, have lengths ∼5,000–10,000 km, widths of a few hundred km, lifetimes of a few minutes, and cluster around the magnetic network (e.g., Beckers, 1968, 1972). Although many ideas exist, their generation mechanism is still unknown; see discussions in various review works (e.g., Beckers, 1968, 1972; Sterling, 2000; Tsiropoula et al., 2012; Hinode Review Team et al., 2019; Sterling, 2021).
As mentioned in §3.2, erupting microfilament flux ropes have not been observed in spicules. They may, however, be present, but hard to observe for a variety of reasons. Similarly, a bright point that corresponds to a JBP has not been convincingly observed at the base of spicules. These details are not consistent with an erupting-microfilament mechanism for spicules. Nonetheless, these absences are not definitive evidence that these features do not exist in spicules; they may exist but be hard to detect, as discussed in Sterling et al. (2020a).
Moreover, there are several observations that are consistent with a microfilament-eruption mechanism for spicules. One of these is the observation of mixed polarity elements at the base of many spicules. Fresh evidence for this is presented in Samanta et al. (2019), obtained using state-of-the-art ground-based observations in the pre-DIKIST era. This work presents evidence that spicules result from dynamic activity at the base of spicules, which could be due to flux cancelation and/or emergence. As discussed above, there is extensive evidence that many coronal jets result from flux-cancelation episodes. Ideas for coronal-jet production from flux emergence has also been presented (Yokoyama and Shibata, 1995).
Spicules also display characteristics of spinning motions (Pasachoff et al., 1968; De Pontieu et al., 2012; Sterling et al., 2020b). We have noted that the minifilament-eruption idea offers an explanation for the spinning of coronal jets, when an erupting twisted minifilament transfers its twist to the coronal-jet-spire’s coronal field via external reconnection. Thus the same mechanism acting on speculative erupting microfilaments might explain this spicule spinning as magnetic untwisting.
There remains, however, the possibility that spicules are driven by any of a number of other suggested mechanisms (see the above-cited reviews), and many of these ideas cannot yet be ruled out. Spicule-sized features that work via the coronal-jet-production mechanism may instead drive other features of that size, such as the UV network jets described by Tian et al. (2014) (some of which are UV observable in EUV, at least in AIA 171 Å images; Tian et al., 2014), or the “chromospheric anemone jets” observed in active regions, or similar jet-like features observed in plages (De Pontieu et al., 2004; Sterling et al., 2020a).
4 The importance of coronal jets
Coronal jets are important for solar physics in a number of ways. One of these is to gain insight into the buildup and onset of large-scale eruptions. Studies of these eruptions has importance in a variety of areas, ranging from the understanding of key inputs to Space Weather to gaining insight into fundamental astrophysical processes.
One of the key unknowns of solar physics is the details of the mechanism leading up to and causing large-scale solar eruptions that produce typical-sized-filament eruptions, solar flares, and CMEs. The revelation that many coronal jets are scaled-down versions of large eruptions has important implications for resolving these questions. Not only are coronal jets of a smaller size scale than those large eruptions, but also the pre-coronal-jet evolution time scale of coronal jets is substantially shorter than that for large eruptions: Large eruptions from bipolar active regions usually require at least many days to build up the magnetic circumstances that result in the eruption (e.g., Sterling et al., 2018), while in contrast, for a sample of quiet-region jets the corresponding time scale was found to be of the order of hours or a couple of days (Panesar et al., 2017). Additionally, it is difficult to find examples of magnetically isolated large-scale regions that produce large eruptions for many days while they remain on the Earth-facing side of the Sun, and this exacerbates the difficulty in unraveling the fundamental processes that lead to eruptions. On the other hand, it is often easy to find and follow coronal-jet-producing regions on the Sun, by working backwards from the time of coronal-jet occurrence. As discussed in §3.1, Sterling et al. (2018) used these points to learn about regions leading to flares and CMEs in two small active regions, largely based on lessons learned from coronal-jet studies. These studies and comparisons are, however, still vastly incomplete. Careful study of the details of eruptions happening on the coronal-jet-sized scale are necessary to understand fully the coronal-jet-production process. And then the findings can be used as starting input for improved studies of large-scale events.
And as demonstrated in §3.2, coronal jets almost certainly can provide insight into the operation of some smaller-scale events. This is in particular true for those smaller objects with the most apparent coronal-jet-like qualities; this includes jetlets, at least some of which must be scaled-down versions of coronal jets. It will be important to see how far the obvious similarities continue down in size scale by using long-term high-resolution and high-cadence observations at appropriate wavelengths. This will help determine whether the similarities continue down to the size of the UV network jets, which are nearing spicule size. Because the solar wind appears to originate from most if not all locations on the Sun (in particular, the fast solar wind originates from open-field areas, while the slow wind comes from closed-field regions), the solar-surface events that drive it are likely distributed almost uniformly over the surface. An understanding of jetlets and UV network jets will provide insight into whether these events plausibly power the solar wind. Careful additional coronal-jet studies are required to guide and assist observational investigations of these yet smaller-scale features.
Coronal-jet physics may have even broader implications beyond the most obvious looking coronal-jet-like or solar-eruption counterparts. For example, Katsukawa et al. (2007) have examined “penumbral jets,” rooted along the filament-like low-lying magnetic loops (the penumbral fibrils) that spread radially from sunspot umbra and form the penumbra (Tiwari et al., 2013). Tiwari et al. (2016, 2018) also studied penumbral jets, and found that large ones originated from the end of the penumbral filament rooted farthest away from the umbra, and that these regions had mixed magnetic polarities that underwent magnetic cancelation near the time of penumbral-jet generation. Moreover, using IRIS spectra, they found Doppler evidence that the penumbral jets are undergoing spinning motion. Although these penumbral jets are ∼100 times smaller than typical SXR coronal jets, their properties of magnetic cancelation at their bases, long (compared to their width) spires, and spin, are similar to what has been found in numerous coronal jets. This suggests that the basic physical mechanism creating these penumbral jets might be essentially the same as that which produces coronal jets.
“Campfires” are features recently discovered in high-resolution EUV images from the Extreme Ultraviolet Imager (EUI) on the Solar Orbiter spacecraft, appearing as small localized brighteings of size scales of a few 100—few 1,000 km and lasting 10–200 s (Berghmans et al., 2021). By comparing a selection of campfires with SDO/HMI magentograms, Panesar et al. (2021) present evidence that they occur on canceling magnetic neutral lines, similar to how coronal-jet-producing erupting minifilaments and accompanying base brightenings also frequently occur on canceling neutral lines. This suggests that the same process that makes coronal jets (small-scale-filament/flux rope eruptions) might also make campfires.
If the same processes indeed make features as varied as large-scale solar eruptions, coronal jets, jetlets, some spicules, UV network jets, campfires, penumbral jets, and perhaps other features, then it is important for heliophysics to clarify which magnetic and physical circumstances produce which feature in what situations.
Coronal jets also have influence far out into the heliosphere. White-light coronagraph images show that some CMEs are relatively narrow, with angular extents ≲ 5°; these features have been called both “narrow CMEs” and “white-light jets” (See discussion and references in Sterling, 2018). Wang et al. (1998) have shown that these features often originate from jetting activity at the solar surface, and a mechanism for producing these features has been presented, based on the minifilament-eruption picture, by Panesar et al. (2016a). Sterling and Moore (2020) present observations of material expelled from coronal jets extending out to tens of solar radii in images from the STEREO “Hi1” Heliospheric Imager. There is also evidence of in situ detection of coronal-jet material in the solar wind (Yu et al., 2014, 2016). Both (Sterling and Moore, 2020), and a followup work (Neugebauer and Sterling, 2021), suggest that coronal jets, and/or smaller jet-like features that work via the minifilament-eruption mechanism, might propagate out to the heliospheric locations of the Parker Solar Probe satellite, and be detected as magnetic Alfvénic kinks in the field that are known as “switchbacks” (Bale et al., 2019; Schwadron and McComas, 2021). The idea is that the erupting minifilaments might carry twist, and external reconnection with the ambient coronal field could transfer that twist to the field, as described by Shibata and Uchida (1986). That twist could convert to swaying of inner coronal field (Moore et al., 2015), and then steepen into an Alfvénic kink–forming the switchback–due to variations of the Alfvén speed in the solar wind (Sterling and Moore, 2020). Switchbacks are extremely common in the solar wind at distances of a few tens of solar radii (Bale et al., 2019). They also appear to carry the imprint of size scales at the Sun corresponding to supergranules (Bale et al., 2021; Fargette et al., 2021), and even of granules (Fargette et al., 2021). The minifilaments that erupts to make coronal jets (∼10,000—few ×10,000 km; e.g., Sterling et al., 2015; Panesar et al., 2016b) are not so different from supergranule scales (∼40,000 km), while the width of spicules and similar features (few 100 km or so) is not too different from the size scale of granules (∼1,000 km). Better observations of coronal jets can help determine whether this switchback-production idea matches detailed observations, or whether a different mechanism might be responsible for the switchbacks, such as “interchange reconnection” ideas (e.g., Owens et al., 2020; Zank et al., 2020; Drake et al., 2021; Schwadron and McComas, 2021), or any of several ideas for generating the switchbacks in the solar wind (see citations in Fargette et al., 2022), while keeping in mind that the mechanism should explain the observations of a supergranule- and/or granule-size-scale dependence of switchback size scales.
These points illustrate the value to solar physics–and beyond–of understanding the nature of the processes that lead up to and produce coronal jets. To this end, we suggest a new instrument focused on observing coronal-jet-sized features in the low corona. We first note, however, that another reason for further observations of coronal jets is to learn more about the nature of coronal jets themselves. Even though there is strong evidence that the minifilament eruption model explains many coronal jets, it is still to be confirmed that the model holds up to close scrutiny under improved observations. An alternative mechanism, which was originally proposed along with the earliest detailed coronal-jet observations (Shibata et al., 1992), is that they result when emerging magnetic flux reconnects (external reconnection) with surrounding coronal field. As mentioned in §1, many observations fit the minifilament-eruption model and find that coronal jets frequently occur as the result of minifilament (or flux rope) eruptions on canceling magnetic neutral lines. Other observations of coronal jets–such as the direction of motion of coronal-jet spires horizontal to the solar surface–are also consistent wtih the minifilament-eruption mechanism and not with the emerging-flux mechanism (Baikie et al., 2022). So questions are: do coronal jets ever form via the emerging-flux mechanism, and if so, are there any special characteristics of those coronal jets compared to those that we have described in §1? Another question is, if coronal jets do not occur frequently via the emerging-flux mechanism, then why not? After all, numerical simulations indicate that flux emerging into open surrounding coronal field should produce a coronal jet (e.g., Yokoyama and Shibata, 1995; Nishizuka et al., 2008). So if that process does not produce coronal jets on the Sun in reality, then it is important to understand why the reconnection resulting from flux emergence (which inevitably must happen) does not produce actual coronal jets. These questions emphasize the importance of understanding physics on the size scale of coronal jets.
5 The “SEIM” instrument to observe coronal jets and jet-like structures
We propose a new instrument for the next-generation, under the provisional name of the “Solar Explosions IMager” (SEIM). This instrument would be tuned to observe features of size scales of coronal jets at EUV wavelengths, with a spatial resolution and cadence similar to that of the Hi-C2.1 instrument. The Hi-C flights, however, were on sounding rockets, and so of short duration (∼5 min). The Solar Orbiter/EUI instrument can also achieve high resolution comparable to that of Hi-C, but such high resolution is only available for a few days around Solar Orbiter’s perihelion. Our idea is for SEIM to be on a satellite, allowing for long-term high-resolution observations. We now outline the instrument’s desired characteristics.
5.1 Wavelength coverage
As pointed out in §2, coronal jets in polar coronal holes such that the spires are generally seen in the SDO/AIA channels of 304, 171, 193, and/or 211 Å. Among these, 171, 193, and 211 are all coronal lines. It would be best to include all of these channels, as sometimes the spire tends to be better seen in one than the other. Nonetheless, if it is visible in one of these three channels, it is usually at least detectable in the other two, based on observations of 41 coronal jets in Sterling et al. (2015) and Sterling and Moore (2023). Therefore, as a minimum, one of these three channels should be included in a minimal mission. The 304 Å channel shows a mixture of what might be called “upper”-chromospheric and transition region plasmas. It sometimes shows features of coronal jets detected in SXRs that are not apparent in the other three cool-coronal (171, 193, 211 Å) channels (Sterling and Moore, 2023), and therefore that channel would be essential to include in a SEIM mission.
Brighter coronal jets, such as those occurring at the periphery of active regions, are often visible in all AIA EUV channels. Therefore, an instrument designed to see coronal-jet-like features in coronal holes (and quiet Sun) well would also be able to see coronal jets and similar features in active regions.
A channel that shows photospheric emissions, such as AIA’s 1600 Å channel, would be essential to facilitate comparisons with other instruments such as DKIST. This channel also shows ribbon-like flare emission at the base of some active-region jets (Sterling et al., 2016).
Given these considerations, a minimal wavelength-coverage package for a SEIM instrument could be 304, 171 or 193, 94, and 1600 Å.
5.2 Resolution, cadence, and field of view
In active regions, some strands of erupting minifilaments are substantially thinner than the width of erupting minifilaments in coronal holes or quiet regions, having widths of ≲ 2″ (Sterling et al., 2016). Hi-C, either in its original incarnation (Kobayashi et al., 2014) or the Hi-C2.1 version discussed above (§3.3), would be able to resolve many of these, and in general sees features at the limit of or beyond what is readily detectable in AIA (e.g., Brooks et al., 2013; Tiwari et al., 2016; Panesar et al., 2019; Tiwari et al., 2019; Sterling et al., 2020a). Based on this, we are confident that a resolution comparable to that of Hi-C (0″. 1 pixels) will be adequate for revolutionary breakthroughs in the study of coronal jets and similar-sized phenomena.
For coronal-jet studies, the 12-s cadence of AIA has been adequate. Smaller-scale jet-like features can have lifetimes shorter than the ∼tens of minutes of coronal jets, including ∼one minute for small jetlets (Panesar et al., 2018b) and UV network jets. Therefore a faster-than-AIA time cadence comparable to that of Hi-C, about 5 s, would be preferred in order to sample these objects well.
A FOV of about 6′ × 6′, would be acceptable for an initial mission. This would be slightly larger than the 4′.4 × 4′.4 Hi-C2.1 FOV (Rachmeler et al., 2019). SDO/AIA’s detector is a circle of diameter 41′ (Lemen et al., 2012), and so our proposed detector would have a FOV of about one-sixth that of AIA’s. Thus we could obtain our goal of 0″. 1 with a FOV one-sixth that of AIA’s, by using an AIA-sized detector (4096 × 4096 pixels2). Advances in technology might make it feasible to improve upon this, allowing for increased FOV and/or higher resolution, but these minimal criteria would allow for substantial advancements in our understanding of coronal jets and jet-like features. Figure 6 shows a sample image from XRT with a FOV similar to that being discussed (that image’s FOV is only slightly larger, at 6′.67 × 6′.67), with much of the northern polar coronal hole and several X-ray coronal jets visible.
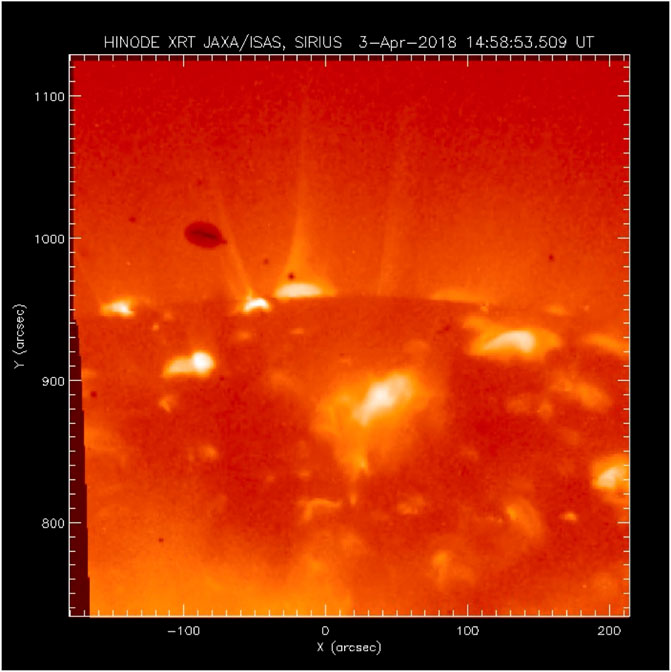
FIGURE 6. Hinode/XRT image in SXRs (from Sterling and Moore, 2023) of the northern polar coronal hole region, showing several coronal jets. The field of view (FOV) here is 6′.67 × 6′.67. This is a little larger than the minimum FOV (6′.0 × 6′.0) that would be desired for the suggested SEIM mission. (The dark oval near (−100, 1,000) and similar smaller dark spots are imaging artifacts).
5.3 Orbit, accompanying instrumentation, and operations planning
Ideally, a mission carrying a SEIM instrument would have extended, uninterrupted views of the Sun. Accordingly, a Sun-synchronous orbit, such as that of Hinode (Kosugi et al., 2007) or IRIS (De Pontieu et al., 2014) would be appropriate, allowing for ∼nine mouths of uninterrupted viewing, and ∼three months with orbits that include spacecraft nights while still allowing ∼one hour of solar observing per orbit. Longer periods of uninterrupted viewing would be possible from L1 or a similar location, but that might be more appropriate for a more extensive followup mission.
An imaging-only mission plan would be of limited value for advancing the science of jet-like features. As a minimum, systematic corresponding line-of-sight magnetograms would be essential to complement these observations. This could be included on the same spacecraft, in which case a magnetogram FOV comparable to that of the EUV instrument would be acceptable. Alternatively, it would be possible to use synoptic full-disk magnetograms from elsewhere if appropriate ones are available; for example, it would be fully acceptable to rely on magnetograms from SDO/HMI or a similar instrument on a different satellite that is operational at the time of a SEIM mission. In either case, the time cadence should be about ∼1 min, comparable to that of SDO/HMI (45 s). Spatial resolution of HMI’s level would be the minimum desired, but would be adequate for an initial mission. For special programs, coordinated observations with DKIST or other ground-based instruments (such as BBSO; e.g., Samanta et al., 2019) would allow for much higher-resolution magnetograms. It will also be extremely valuable to have spectroscopic observations at UV and/or EUV, or even SXR, wavelengths, to obtain diagnostic information on the observed objects. These spectra should have sufficient spatial and spectral resolution and high-enough cadence to address questions such as whether jetlet, UV network jet, and even spicule-sized objects routinely display characteristics of jets, such as spinning motion of their spires.
We know that coronal jets are common in polar coronal holes, and they are observed in on-disk coronal holes also. Therefore, a basic minimal-maintenance plan would be to observe (with tracking) an on-disk coronal hole when one is available. This would allow for coordination with line-of-sight magentograms. A second low-latitude target would be active regions. The frequency of typical coronal jets from active regions is not yet known, but they are not uncommon. Even in the absence of such coronal jets, there are smaller-scale penumbral jets that are ubiquitous in active regions, and it would be desirable to have high-resolution, high-cadence observations of other active-region activity, and of course it would be highly desirable for the instrument to observe large-scale eruptions from active regions. In the absence of on-disk coronal holes and active regions (or if there are only active regions showing essentially no substantial activity), one of the two polar regions (preferably one with a prominent coronal hole) would be the standard default target.
5.4 Extensions
The instrument proposed here could act as a proving ground for a more elaborate mission that features a full-disk FOV and wider wavelength coverage. This would be analogous to how the TRACE mission (Handy et al., 1999) preceded AIA on SDO. It would be fully appropriate for such a more-extensive mission to operate from L1 or similar location, with uninterrupted solar viewing. Such an instrument would ideally be accompanied by a complementary magnetograph, and perhaps other instruments, on the same spacecraft.
The Appendix (in Supplementary Material) provides a summary of properties of coronal jets and jet-like features that will be either observed directly with SEIM, or to which SEIM will provide valuable supplementary observations, for refining our understanding of all of these features.
Even in the simplest form however, the SEIM instrument suggested above would be far more than just a “solar-jets telescope.” As we have argued above, solar jets can be viewed as a proxy for one of the many types of possibly similar solar features, on both larger and smaller size scales, that could be observed and studied in detail with such as instrument. Therefore, SEIM would provide a new, high-resolution, high-time-cadence window into an understanding of fundamental explosive phenomena that occur on multiple size scales in the lower solar atmosphere, and that possibly power the heliosphere as well.
Data availability statement
No new data were analyzed for this summary-type presentation. All background material presented in the figures is available in the references given in the corresponding figure captions.
Author contributions
AS collected all materials and authored the bulk of the manuscript. RM consulted on all aspects of the content. NP contributed consultations and input into coronal jet and jetlet details. TS contributed consultations and input into spicule observation and analysis details. ST contributed consultations and input into sunspot and penumbral jet details. SS critiqued the manuscript and provided valuable feedback.
Funding
ACS, RLM, and NKP were supported with funding from the Heliophysics Division of NASA’s Science Mission Directorate through the Heliophysics Supporting Research (HSR, grant No. 20-HSR20_2–0124) Program, and the Heliophysics Guest Investigators program. ACS and RLM were also supported through the Heliophysics System Observatory Connect (HSOC, grant No. 80NSSC20K1285) Program. ACS received additional support through the MSFC Hinode Project, and NKP received additional support through a NASA SDO/AIA grant. SKT gratefully acknowledges support by NASA HGI (80NSSC21K0520) and HSR (80NSSC23K0093) grants, and NASA contract NNM07AA01C (Hinode).
Acknowledgments
This paper expands upon material presented previously in a white paper submitted to the National Academies of Sciences, Engineering, and Medicine for the U.S. Solar and Space Physics (Heliophysics) Decadal Survey, for Heliophysics 2050 (Sterling and Moore, 2023). Hinode is a Japanese mission developed and launched by ISAS/JAXA, with NAOJ as domestic partner and NASA and UKSA as international partners. It is operated by these agencies in co-operation with ESA and NSC (Norway). We acknowledge the use of data from AIA and HMI data, both of which are instruments onboard SDO, a mission of NASA’s Living with a Star program.
Conflict of interest
The authors declare that the research was conducted in the absence of any commercial or financial relationships that could be construed as a potential conflict of interest.
Publisher’s note
All claims expressed in this article are solely those of the authors and do not necessarily represent those of their affiliated organizations, or those of the publisher, the editors and the reviewers. Any product that may be evaluated in this article, or claim that may be made by its manufacturer, is not guaranteed or endorsed by the publisher.
Supplementary material
The Supplementary Material for this article can be found online at: https://www.frontiersin.org/articles/10.3389/fspas.2023.1117870/full#supplementary-material
References
Adams, M., Sterling, A. C., Moore, R. L., and Gary, G. A. (2014). A small-scale eruption leading to a blowout macrospicule jet in an on-disk coronal hole. Astrophysical J. 783, 11. doi:10.1088/0004-637X/783/1/11
Asai, A., Shibata, K., Ishii, T. T., Oka, M., Kataoka, R., Fujiki, K., et al. (2009). Evolution of the anemone AR NOAA 10798 and the related geo-effective flares and CMEs. J. Geophys. Res. (Space Phys. 114, A00A21. doi:10.1029/2008JA013291
Athay, R. G. (1959). The number of spicules in the middle chromosphere. Astrophysical J. 129, 164. doi:10.1086/146603
Baikie, T. K., Sterling, A. C., Moore, R. L., Alexander, A. M., Falconer, D. A., Savcheva, A., et al. (2022). Further evidence for the minifilament-eruption scenario for solar polar coronal jets. arXiv e-prints , arXiv:2201.08882.
Bale, S. D., Badman, S. T., Bonnell, J. W., Bowen, T. A., Burgess, D., Case, A. W., et al. (2019). Highly structured slow solar wind emerging from an equatorial coronal hole. Nature 576, 237–242. doi:10.1038/s41586-019-1818-7
Bale, S. D., Horbury, T. S., Velli, M., Desai, M. I., Halekas, J. S., McManus, M. D., et al. (2021). A solar source of alfvénic magnetic field switchbacks: In situ remnants of magnetic funnels on supergranulation scales. ApJ 923, 174. doi:10.3847/1538-4357/ac2d8c
Beckers, J. M. (1972). Solar spicules. Annu. Rev. Astronomy Astrophysics 10, 73–100. doi:10.1146/annurev.aa.10.090172.000445
Beckers, J. M. (1968). Solar spicules (invited review paper). Sol. Phys. 3, 367. doi:10.1007/BF00171614
Berghmans, D., Auchère, F., Long, D. M., Soubrié, E., Mierla, M., Zhukov, A. N., et al. (2021). Extreme-UV quiet sun brightenings observed by the solar orbiter/EUI. Astron. & Astrophys. 656, L4. doi:10.1051/0004-6361/202140380
Bernasconi, P. N., Rust, D. M., and Hakim, D. (2005). Advanced automated solar filament detection and characterization code: Description, performance, and results. Sol. Phys. 228, 97–117. doi:10.1007/s11207-005-2766-y
Brooks, D. H., Warren, H. P., Ugarte-Urra, I., and Winebarger, A. R. (2013). High spatial resolution observations of loops in the solar corona. ApJ Lett. 772, L19. doi:10.1088/2041-8205/772/2/L19
Canfield, R. C., Reardon, K. P., Leka, K. D., Shibata, K., Yokoyama, T., and Shimojo, M. (1996). H-alpha surges and x-ray jets in ar 7260. Astrophysical J. 464, 1016. doi:10.1086/177389
Chen, P. F. (2011). Coronal mass ejections: Models and their observational basis. Living Rev. Sol. Phys. 8, 1. doi:10.12942/lrsp-2011-1
Cheung, M. C. M., De Pontieu, B., Tarbell, T. D., Fu, Y., Tian, H., Testa, P., et al. (2015). Homologous helical jets: Observations by IRIS, SDO, and hinode and magnetic modeling with data-driven simulations. ApJ 801, 83. doi:10.1088/0004-637X/801/2/83
Chintzoglou, G., Zhang, J., Cheung, M. C. M., and Kazachenko, M. (2019). The origin of major solar activity: Collisional shearing between nonconjugated polarities of multiple bipoles emerging within active regions. ApJ 871, 67. doi:10.3847/1538-4357/aaef30
Cirtain, J. W., Golub, L., Lundquist, L., van Ballegooijen, A., Savcheva, A., Shimojo, M., et al. (2007). Evidence for alfvén waves in solar x-ray jets. Science 318, 1580–1582. doi:10.1126/science.1147050
De Pontieu, B., Carlsson, M., Rouppe van der Voort, L. H. M., Rutten, R. J., Hansteen, V. H., and Watanabe, H. (2012). Ubiquitous torsional motions in type ii spicules. Astrophysical J. 752L, 12. doi:10.1088/2041-8205/752/1/L12
De Pontieu, B., Erdélyi, R., and James, S. P. (2004). Solar chromospheric spicules from the leakage of photospheric oscillations and flows. Nature 430, 536–539. doi:10.1038/nature02749
De Pontieu, B., McIntosh, S., Hansteen, V. H., Carlsson, M., Schrijver, C. J., Tarbell, T. D., et al. (2007). A tale of two spicules: The impact of spicules on the magnetic chromosphere. Publ. Astron. Soc. Jpn. 59, 655. doi:10.1093/pasj/59.sp3.S655
De Pontieu, B., Title, A. M., Lemen, J. R., Kushner, G. D., Akin, D. J., Allard, B., et al. (2014). The interface region imaging spectrograph (iris). Sol. Phys. 289, 2733–2779. doi:10.1007/s11207-014-0485-y
Devi, P., Joshi, B., Chandra, R., Mitra, P. K., Veronig, A. M., and Joshi, R. (2020). Development of a confined circular-cum-parallel ribbon flare and associated pre-flare activity. Sol. Phys. 295, 75. doi:10.1007/s11207-020-01642-y
Doyle, L., Wyper, P. F., Scullion, E., McLaughlin, J. A., Ramsay, G., and Doyle, J. G. (2019). Observations and 3D magnetohydrodynamic modeling of a confined helical jet launched by a filament eruption. ApJ 887, 246. doi:10.3847/1538-4357/ab5d39
Drake, J. F., Agapitov, O., Swisdak, M., Badman, S. T., Bale, S. D., Horbury, T. S., et al. (2021). Switchbacks as signatures of magnetic flux ropes generated by interchange reconnection in the corona. Astron. & Astrophys. 650, A2. A2. doi:10.1051/0004-6361/202039432
Fargette, N., Lavraud, B., Rouillard, A. P., Réville, V., Bale, S. D., and Kasper, J. (2022). The preferential orientation of magnetic switchbacks and its implications for solar magnetic flux transport. Astron. & Astrophys. 663, A109. doi:10.1051/0004-6361/202243537
Fargette, N., Lavraud, B., Rouillard, A. P., Réville, V., Dudok De Wit, T., Froment, C., et al. (2021). Characteristic scales of magnetic switchback patches near the sun and their possible association with solar supergranulation and granulation. ApJ 919, 96. doi:10.3847/1538-4357/ac1112
Foukal, P. (2013). Solar astrophysics. third, revised edition. Weinheim, Germany: WILEY-VCH Verlag GmbH & KGaA.
Golub, L., Deluca, E., Austin, G., Bookbinder, J., Caldwell, D., Cheimets, P., et al. (2007). The x-ray telescope (xrt) for the hinode mission. Sol. Phys. 243, 63–86. doi:10.1007/s11207-007-0182-1
Golub, L., Krieger, A. S., Silk, J. K., Timothy, A. F., and Vaiana, G. S. (1974). Solar X-ray bright points. ApJ Lett. 189, L93. doi:10.1086/181472
Handy, B. N., Acton, L. W., Kankelborg, C. C., Wolfson, C. J., Akin, D. J., Bruner, M. E., et al. (1999). The transition region and coronal explorer. Sol. Phys. 187, 229–260. doi:10.1023/A:1005166902804
Hinode Review Team, Al-Janabi, K., Antolin, P., Baker, D., Bellot Rubio, L. R., Bradley, L., et al. (2019). Achievements of hinode in the first eleven years. Publ. Astron. Soc. Jpn. 71 (5). doi:10.1093/pasj/psz084
Hirayama, T. (1974). Theoretical model of flares and prominences i evaporating flare model. Sol. Phys. 34, 323–338. doi:10.1007/BF00153671
Hong, J., Jiang, Y., Zheng, R., Yang, J., Bi, Y., and Yang, B. (2011). A micro coronal mass ejection associated blowout extreme-ultraviolet jet. Astrophysical J. 738L, 20. doi:10.1088/2041-8205/738/2/L20
Huang, M., Doyle, J. G., and Lamb, D. A. (2012). Coronal hole boundaries at small scales. Astronomy Astrophysics 548, 62. doi:10.1051/0004-6361/201220079
Joshi, B., Thalmann, J. K., Mitra, P. K., Chandra, R., and Veronig, A. M. (2017). Observational and model analysis of a two-ribbon flare possibly induced by a neighboring blowout jet. Astrophysical J. 851, 29. doi:10.3847/1538-4357/aa9564
Judge, P. G., and Carlsson, M. (2010). On the solar chromosphere observed at the limb with hinode. Astrophysical J. 719, 469–473. doi:10.1088/0004-637X/719/1/469
Katsukawa, Y., Berger, T. E., Ichimoto, K., Lites, B. W., Nagata, S., Shimizu, T., et al. (2007). Small-scale jetlike features in penumbral chromospheres. Science 318, 1594–1597. doi:10.1126/science.1146046
Kobayashi, K., Cirtain, J., Winebarger, A. R., Korreck, K., Golub, L., Walsh, R. W., et al. (2014). The high-resolution coronal imager (hi-C). Sol. Phys. 289, 4393–4412. doi:10.1007/s11207-014-0544-4
Kosugi, T., Matsuzaki, K., Sakao, T., Shimizu, T., Sone, Y., Tachikawa, S., et al. (2007). The hinode (solar-b) mission: An overview. Sol. Phys. 243, 3–17. doi:10.1007/s11207-007-9014-6
Koutchmy, S., Hara, H., Suematsu, Y., and Reardon, K. (1997). Sxr coronal flashes. Astronomy Astrophysics 320L, 33.
Kumar, P., Karpen, J. T., Antiochos, S. K., Wyper, P. F., DeVore, C. R., and DeForest, C. E. (2019). Multiwavelength study of equatorial coronal-hole jets. Astrophysical J. 873, 93. doi:10.3847/1538-4357/ab04af
Kumar, P., and Manoharan, P. K. (2013). Eruption of a plasma blob, associated M-class flare, and large-scale extreme-ultraviolet wave observed by SDO. Astron. & Astrophys. 553, A109. doi:10.1051/0004-6361/201220283
Lemen, J. R., Title, A. M., Akin, D. J., Boerner, P. F., Chou, C., Drake, J. F., et al. (2012). The atmospheric imaging assembly (aia) on the solar dynamics observatory (sdo). Sol. Phys. 275, 17–40. doi:10.1007/s11207-011-9776-8
Liu, J., Erdélyi, R., Wang, Y., and Liu, R. (2018). Untwisting jets related to magnetic flux cancellation. Astrophysical J. 852, 10. doi:10.3847/1538-4357/aa992d
Lugaz, N., Downs, C., Shibata, K., Roussev, I. I., Asai, A., and Gombosi, T. I. (2011). Numerical investigation of a coronal mass ejection from an anemone active region: Reconnection and deflection of the 2005 august 22 eruption. ApJ 738, 127. doi:10.1088/0004-637X/738/2/127
Lynch, D. K., Beckers, J. M., and Dunn, R. B. (1973). A morphological study of solar spicules. Sol. Phys. 30, 63L–70L. doi:10.1007/BF00156173
Mazumder, R. (2019). Solar quiet region jet by eruption of minifilament and associated change in magnetic flux. Astron. Rep. 63, 404–408. doi:10.1134/S1063772919050044
McGlasson, R. A., Panesar, N. K., Sterling, A. C., and Moore, R. L. (2019). Magnetic flux cancellation as the trigger mechanism of solar coronal jets. ApJ 882, 16. doi:10.3847/1538-4357/ab2fe3
Moore, R. L., Cirtain, J. W., Sterling, A. C., and Falconer, D. A. (2010). Dichotomy of solar coronal jets: Standard jets and blowout jets. Astrophysical J. 720, 757–770. doi:10.1088/0004-637X/720/1/757
Moore, R. L., Panesar, N. K., Sterling, A. C., and Tiwari, S. K. (2022). Bipolar ephemeral active regions, magnetic flux cancellation, and solar magnetic Explosions. ApJ 933, 12. doi:10.3847/1538-4357/ac6181
Moore, R. L., Sterling, A. C., Falconer, D. A., and Robe, D. (2013). The cool component and the dichotomy, lateral expansion, and axial rotation of solar x-ray jets. Astrophysical J. 769, 134. doi:10.1088/0004-637X/769/2/134
Moore, R. L., Sterling, A. C., Hudson, H. S., and Lemen, J. R. (2001). Onset of the magnetic explosion in solar flares and coronal mass ejections. Astrophysical J. 552, 833–848. doi:10.1086/320559
Moore, R. L., Sterling, R. L., and Falconer, D. A. (2015). Magnetic untwisting in solar jets that go into the outer corona in polar coronal holes. Astrophysical J. 806, 11. doi:10.1088/0004-637X/806/1/11
Muglach, K. (2021). The photospheric footpoints of solar coronal hole jets. ApJ 909, 133. doi:10.3847/1538-4357/abd5ad
Mulay, S. M., Del Zanna, G., and Mason, H. (2017a). Cool and hot emission in a recurring active region jet. Astronomy Astrophysics 606, 4. doi:10.1051/0004-6361/201730429
Mulay, S. M., Del Zanna, G., and Mason, H. (2017b). Temperature and density structure of a recurring active region jet. Astronomy Astrophysics 598, 11. doi:10.1051/0004-6361/201628796
Mulay, S. M., Tripathi, D. T., Del Zanna, G., and Mason, H. (2016). Multiwavelength study of 20 jets that emanate from the periphery of active regions. Astronomy Astrophysics 589, A79. doi:10.1051/0004-6361/201527473
Neugebauer, M., and Sterling, A. C. (2021). Relation of microstreams in the polar solar wind to switchbacks and coronal X-ray jets. ApJ Lett. 920, L31. doi:10.3847/2041-8213/ac2945
Nishizuka, N., Shimizu, M., Nakamura, T., Otsuji, K., Okamoto, T. J., Katsukawa, Y., et al. (2008). Giant chromospheric anemone jet observed with hinode and comparison with magnetohydrodynamic simulations: Evidence of propagating alfvén waves and magnetic reconnection. Astrophysical J. 683, 83–L86. doi:10.1086/591445
Nisticò, G., Bothmer, V., Patsourakos, S., and Zimbardo, G. (2009). Characteristics of euv coronal jets observed with stereo/secchi. Sol. Phys. 259, 87–108. doi:10.1007/s11207-009-9424-8
Nisticò, G., Bothmer, V., Patsourakos, S., and Zimbardo, G. (2010). Observational features of equatorial coronal hole jets. Ann. Geophys. 28, 687–696. doi:10.5194/angeo-28-687-2010
Nisticò, G., Patsourakos, S., Bothmer, V., and Zimbardo, G. (2011). Determination of temperature maps of EUV coronal hole jets. Adv. Space Res. 48, 1490–1498. doi:10.1016/j.asr.2011.07.003
Owens, M., Lockwood, M., Macneil, A., and Stansby, D. (2020). Signatures of coronal loop opening via interchange reconnection in the slow solar wind at 1 AU. Sol. Phys. 295, 37. doi:10.1007/s11207-020-01601-7
Panesar, N. K., Sterling, A. C., Moore, R. L., and Chakrapani, P. (2016b). Magnetic flux cancelation as the trigger of solar quiet-region coronal jets. Astrophysical J. 832L, 7. doi:10.3847/2041-8205/832/1/L7
Panesar, N. K., Sterling, A. C., and Moore, R. L. (2016a). Homologous jet-driven coronal mass ejections from solar active region 12192. Astrophysical J. 822L, L23. doi:10.3847/2041-8205/822/2/L23
Panesar, N. K., Sterling, A. C., and Moore, R. L. (2018a). Magnetic flux cancelation as the trigger of solar coronal jets in coronal holes. Astrophysical J. 853, 189. doi:10.3847/1538-4357/aaa3e9
Panesar, N. K., Sterling, A. C., and Moore, R. L. (2017). Magnetic flux cancellation as the origin of solar quiet-region pre-jet minifilaments. Astrophysical J. 844, 131. doi:10.3847/1538-4357/aa7b77
Panesar, N. K., Sterling, A. C., Moore, R. L., Tiwari, r. S. K., De Pontieu, B., and Norton, A. A. (2018b). Iris and sdo observations of solar jetlets resulting from network-edge flux cancelation. Astrophysical J. 868L, 27. doi:10.3847/2041-8213/aaef37
Panesar, N. K., Sterling, A. C., Moore, R. L., Winebarger, A. R., Tiwari, S. K., Savage, S. L., et al. (2019). Hi-C 2.1 observations of jetlet-like events at edges of solar magnetic network lanes. ApJ Lett. 887, L8. doi:10.3847/2041-8213/ab594a
Panesar, N. K., Tiwari, S. K., Berghmans, D., Cheung, M. C. M., Müller, D., Auchere, F., et al. (2021). The magnetic origin of solar campfires. ApJ Lett. 921, L20. doi:10.3847/2041-8213/ac3007
Panesar, N. K., Tiwari, S. K., Moore, R. L., Sterling, A. C., and De Pontieu, B. (2022). Genesis and coronal-jet-generating eruption of a solar minifilament captured by IRIS slit-raster spectra. ApJ 939, 25. doi:10.3847/1538-4357/ac8d65
Paraschiv, A. R., Bemporad, A., and Sterling, A. C. (2015). Physical properties of solar polar jets. A Stat. study Hinode XRT data, Astronomy Astrophysics 579, 96. doi:10.1051/0004-6361/201525671
Paraschiv, A. R., Donea, A. C., and Judge, P. G. (2022). Thermal and non-thermal properties of active region recurrent coronal jets. ApJ 935, 172. doi:10.3847/1538-4357/ac80fb
Pasachoff, J. M., Noyes, R. W., and Beckers, J. M. (1968). Spectral observations of spicules at two heights in the solar chromosphere. Sol. Phys. 5, 131–158. doi:10.1007/BF00147962
Pike, C. D., and Mason, H. E. (1998). Rotating transition region features observed with the soho coronal diagnostic spectrometer. Sol. Phys. 182, 333–348. doi:10.1023/A:1005065704108
Pucci, S., Poletto, G., Sterling, A. C., and Romoli, M. (2013). Physical parameters of standard and blowout jets. Astrophysical J. 776, 16. doi:10.1088/0004-637X/776/1/16
Rachmeler, L. A., Winebarger, A. R., Savage, S. L., Golub, L., Kobayashi, K., Vigil, G. D., et al. (2019). The high-resolution coronal imager, flight 2.1. Sol. Phys. 294, 174. doi:10.1007/s11207-019-1551-2
Raouafi, N. E., Patsourakos, S., Pariat, E., Young, P. R., Sterling, A. C., Savcheva, A., et al. (2016). Solar coronal jets: Observations, theory, and modeling. Space Sci. Rev. 201, 1–53. doi:10.1007/s11214-016-0260-5
Raouafi, N. E., and Stenborg, G. (2014). Role of transients in the sustainability of solar coronal plumes. Astrophysical J. 787, 118. doi:10.1088/0004-637X/787/2/118
Ruan, G., Schmieder, B., Masson, S., Mein, P., Mein, N., Aulanier, G., et al. (2019). Bidirectional reconnection outflows in an active region. ApJ 883, 52. doi:10.3847/1538-4357/ab3657
Samanta, T., Tian, H., Yurchyshyn, V., Peter, H., Cao, W., Sterling, A., et al. (2019). Generation of solar spicules and subsequent atmospheric heating. Science 366, 890–894. doi:10.1126/science.aaw2796
Savcheva, A., Cirtain, J., Deluca, E. E., Lundquist, L. L., Golub, L., Weber, M., et al. (2007). A study of polar jet parameters based on hinode xrt observations. Publ. Astron. Soc. Jpn. 59, 771. doi:10.1093/pasj/59.sp3.S771
Scherrer, P. H., Schou, J., Bush, R. I., Kosovichev, A. G., Bogart, R. S., Hoeksema, J. T., et al. (2012). The helioseismic and magnetic imager (hmi) investigation for the solar dynamics observatory (sdo). Sol. Phys. 275, 207–227. doi:10.1007/s11207-011-9834-2
Schmieder, B., Joshi, R., and Chandra, R. (2022). Solar jets observed with the interface region imaging spectrograph (IRIS). Adv. Space Res. 70, 1580–1591. doi:10.1016/j.asr.2021.12.013
Schmieder, B. (2022). Solar jets: SDO and IRIS observations in the perspective of new MHD simulations. arXiv e-printsarXiv:2201.11541.
Schwadron, N. A., and McComas, D. J. (2021). Switchbacks explained: Super-parker fields—the other side of the sub-parker spiral. ApJ 909, 95. doi:10.3847/1538-4357/abd4e6
Shen, Y., Liu, Y., Su, J., and Deng, Y. (2012). On a coronal blowout jet: The first observation of a simultaneously produced bubble-like cme and a jet-like cme in a solar event. Astrophysical J. 745, 164. doi:10.1088/0004-637X/745/2/164
Shen, Y. (2021). Observation and modelling of solar jets. Proc. R. Soc. Lond. Ser. A 477, 20200217. doi:10.1098/rspa.2020.0217
Shibata, K., Ishido, Y., Acton, L. W., Strong, K. T., Hirayama, T., Uchida, Y., et al. (1992). Observations of x-ray jets with the yohkoh soft x-ray telescope. Publ. Astron. Soc. Jpn. 44, L173.
Shibata, K., and Magara, T. (2011). Solar flares: Magnetohydrodynamic processes. LRSP 8, 6. doi:10.12942/lrsp-2011-6
Shibata, K., Masuda, S., Shimojo, M., Hara, H., Yokoyama, T., Tsuneta, S., et al. (1995). Hot-plasma ejections associated with compact-loop solar flares. ApJ Lett. 451, L83. doi:10.1086/309688
Shibata, K., Nakamura, T., Matsumoto, T., Otsuji, K., Okamoto, T. J., Nishizuka, N., et al. (2007). Chromospheric anemone jets as evidence of ubiquitous reconnection. Science 318, 1591–1594. doi:10.1126/science.1146708
Shibata, K., and Uchida, Y. (1986). A magnetodynamic mechanism for the formation of astrophysical jets. ii - dynamical processes in the accretion of magnetized mass in rotation. Sol. Phys. 178, 379.
Shimojo, M., Hashimoto, S., Shibata, K., Hirayama, T., Hudson, H. S., and Acton, L. W. (1996). Statistical study of solar x-ray jets observed with the yohkoh soft x-ray telescope. Publ. Astron. Soc. Jpn. 48, 123. doi:10.1093/pasj/48.1.123
Shimojo, M., and Shibata, K. (2000). Physical parameters of solar x-ray jets. Astrophysical J. 542, 1100–1108. doi:10.1086/317024
Solanki, R., Srivastava, A. K., Rao, Y. K., and Dwivedi, B. N. (2019). Twin cme launched by a blowout jet originated from the eruption of a quiet-sun mini-filament. Sol. Phys. 294, 68. doi:10.1007/s11207-019-1453-3
Sterling, A. C. (2018). Coronal jets, and the jet-CME connection. J. Phys. Conf. Ser. 1100, 012024. doi:10.1088/1742-6596/1100/1/012024
Sterling, A. C. (2021). “Fine-scale features of the Sun’s atmosphere: Spicules and jets,” in Solar physics and solar wind. Editors N. E. Raouafi, and A. Vourlidas, 1, 221. doi:10.1002/9781119815600.ch6
Sterling, A. C. (2000). Solar spicules: A review of recent models and targets for future observations - (invited review). Sol. Phys. 196, 79–111. doi:10.1023/A:1005213923962
Sterling, A. C., and Moore, R. L. (2016). A microfilament-eruption mechanism for solar spicules. Astrophysical J. 828, L9. doi:10.3847/2041-8205/828/1/L9
Sterling, A. C., and Moore, R. L. (2020). Coronal-jet-producing minifilament eruptions as a possible source of parker solar Probe switchbacks. ApJ Lett. 896, L18. doi:10.3847/2041-8213/ab96be
Sterling, A. C., and Moore, R. L. (2001a). Eit crinkles as evidence for the breakout model of solar eruptions. Astrophysical J. 560, 1045–1057. doi:10.1086/322241
Sterling, A. C., and Moore, R. L. (2001b). Internal and external reconnection in a series of homologous solar flares. JGR 106, 25227–25238. doi:10.1029/2000JA004001
Sterling, A. C., and Moore, R. L. (2023). Future high-resolution and high-cadence observations for unraveling eruptive solar features. Bullet. Amer. Astron. Soc. Available at: http://surveygizmoresponseuploads.s3.amazonaws.com/fileuploads%2F623127%2F6920789%2F44-ad8196fa57ed847241efefcfd0a78fcd_SterlingAlphonseC.pdf. (in press).
Sterling, A. C., Moore, R. L., Falconer, D. A., and Adams, M. (2015). Small-scale filament eruptions as the driver of x-ray jets in solar coronal holes. Nature 523, 437–440. doi:10.1038/nature14556
Sterling, A. C., Moore, R. L., Falconer, D. A., Panesar, N. K., Akiyama, S., Yashiro, S., et al. (2016). Minifilament eruptions that drive coronal jets in a solar active region. Astrophysical J. 821, 100. doi:10.3847/0004-637X/821/2/100
Sterling, A. C., Moore, R. L., Falconer, D. A., Panesar, N. K., and Martinez, F. (2017). Solar active region coronal jets. ii. triggering and evolution of violent jets. Astrophysical J. 844, 28. doi:10.3847/1538-4357/aa7945
Sterling, A. C., Moore, R. L., and Panesar, N. K. (2022). Another look at erupting minifilaments at the base of solar X-ray polar coronal “standard” and “blowout” jets. ApJ 927, 127. doi:10.3847/1538-4357/ac473f
Sterling, A. C., Moore, R. L., and Panesar, N. K. (2018). Magnetic flux cancelation as the buildup and trigger mechanism for cme-producing eruptions in two small active regions. Astrophysical J. 864, 68. doi:10.3847/1538-4357/aad550
Sterling, A. C., Moore, R. L., Panesar, N. K., Reardon, K. P., Molnar, M., Rachmeler, L. A., et al. (2020a). Hi-C 2.1 observations of small-scale miniature-filament-eruption-like cool ejections in an active region plage. ApJ 889, 187. doi:10.3847/1538-4357/ab5dcc
Sterling, A. C., Moore, R. L., Qiu, J., and Wang, H. (2001). Hα proxies for EIT crinkles: Further evidence for preflare “breakout”-type activity in an ejective solar eruption. ApJ 561, 1116–1126. doi:10.1086/323374
Sterling, A. C., Moore, R. L., Samanta, T., and Yurchyshyn, V. (2020b). Possible production of solar spicules by microfilament eruptions. ApJ Lett. 893, L45. doi:10.3847/2041-8213/ab86a5
Tandberg-Hanssen, E. (1995). The nature of solar prominences, 199. Kluwer Academic Publishers. doi:10.1007/978-94-017-3396-0
Tian, H., DeLuca, E. E., Cranmer, S. R., De Pontieu, B., Peter, H., Martínez-Sykora, J., et al. (2014). Prevalence of small-scale jets from the networks of the solar transition region and chromosphere. Science 346, 1255711. doi:10.1126/science.1255711
Tiwari, S. K., Moore, R. L., De Pontieu, B., Tarbell, T. D., Panesar, N. K., Winebarger, A. R., et al. (2018). Evidence of twisting and mixed-polarity solar photospheric magnetic field in large penumbral jets: Iris and hinode observations. Astrophysical J. 869, 147. doi:10.3847/1538-4357/aaf1b8
Tiwari, S. K., Moore, R. L., Winebarger, A. R., and Alpert, S. E. (2016). Transition-region/coronal signatures and magnetic setting of sunspot penumbral jets: Hinode (sot/fg), hi-c, and sdo/aia observations. Astrophysical J. 816, 92. doi:10.3847/0004-637X/816/2/92
Tiwari, S. K., Panesar, N. K., Moore, R. L., De Pontieu, B., Winebarger, A. R., Golub, L., et al. (2019). Fine-scale explosive energy release at sites of prospective magnetic flux cancellation in the core of the solar active region observed by hi-C 2.1, IRIS, and SDO. ApJ 887, 56. doi:10.3847/1538-4357/ab54c1
Tiwari, S. K., van Noort, M., Lagg, A., and Solanki, S. K. (2013). Structure of sunspot penumbral filaments: A remarkable uniformity of properties. Astron. & Astrophys 557, A25. doi:10.1051/0004-6361/201321391
Tsiropoula, G., Tziotziou, K., Kontogiannis, I., Madjarska, M. S., Doyle, J. G., and Suematsu, Y. (2012). Solar fine-scale structures. i. spicules and other small-scale, jet-like events at the chromospheric level: Observations and physical parameters. Space Sci. Rev. 169, 181–244. doi:10.1007/s11214-012-9920-2
Tsuneta, S., Acton, L., Bruner, M., Lemen, J., Brown, W., Caravalho, R., et al. (1991). The soft x-ray telescope for the solar-a mission. Sol. Phys. 136, 37–67. doi:10.1007/BF00151694
van Ballegooijen, A. A., and Martens, P. C. H. (1989). Formation and eruption of solar prominences. Astrophysical J. 343, 971. doi:10.1086/167766
Wang, Y.-M., Sheeley, N. R., Socker, G., D., Howard, R. A., Brueckner, G. E., Michels, D. J., et al. (1998). Observations of correlated white-light and extreme-ultraviolet jets from polar coronal holes. Astrophysical J. 508, 899–907. doi:10.1086/306450
Wyper, P. F., Antiochos, S. K., and DeVore, C. R. (2017). A universal model for solar eruptions. Nature 544, 452–455. doi:10.1038/nature22050
Wyper, P. F., DeVore, C. R., and Antiochos, S. K. (2018a). A breakout model for solar coronal jets with filaments. Astrophysical J. 852, 98. doi:10.3847/1538-4357/aa9ffc
Wyper, P. F., DeVore, C. R., and Antiochos, S. K. (2019). Numerical simulation of helical jets at active region peripheries. Mon. Not. R. Astro. Soc. 490, 3679–3690. doi:10.1093/mnras/stz2674
Wyper, P. F., DeVore, C. R., Karpen, J. T., Antiochos, S. K., and Yeates, A. R. (2018b). A model for coronal hole bright points and jets due to moving magnetic elements. Astrophysical J. 864, 165. doi:10.3847/1538-4357/aad9f7
Yang, B., Yang, J., Bi, Y., Xu, Z., Hong, J., Li, H., et al. (2019). Recurrent two-sided loop jets caused by magnetic reconnection between erupting minifilaments and a nearby large filament. ApJ 887, 220. doi:10.3847/1538-4357/ab557e
Yashiro, S., Gopalswamy, N., Michalek, G., Cyr, St.O. C., Plunkett, S. P., Rich, N. B., et al. (2004). A catalog of white light coronal mass ejections observed by the SOHO spacecraft. J. Geophys. Res. (Space Phys. 109, A07105. doi:10.1029/2003JA010282
Yokoyama, T., and Shibata, K. (1995). Magnetic reconnection as the origin of x-ray jets and hα surges on the sun. Nature 375, 42–44. doi:10.1038/375042a0
Yu, H.-S., Jackson, B. V., Buffington, A., Hick, P. P., Shimojo, M., and Sako, N. (2014). The three-dimensional analysis of hinode polar jets using images from lasco c2, the stereo cor2 coronagraphs, and smei. Astrophysical J. 784, 166. doi:10.1088/0004-637X/784/2/166
Yu, H.-S., Jackson, B. V., Yang, Y. H., Chen, N. H., Buffington, A., and Hick, P. P. (2016). A 17 june 2011 polar jet and its presence in the background solar wind. J. Geophys. Res. Space Phys. 121, 4985–4997. doi:10.1002/2016JA022503
Keywords: solar filament eruptions, solar corona, solar X-ray emission, solar extreme ultraviolet emission, solar coronal jets, solar magnetic activity
Citation: Sterling AC, Moore RL, Panesar NK, Samanta T, Tiwari SK and Savage SL (2023) Future high-resolution and high-cadence observations for unraveling small-scale explosive solar features. Front. Astron. Space Sci. 10:1117870. doi: 10.3389/fspas.2023.1117870
Received: 07 December 2022; Accepted: 13 February 2023;
Published: 01 March 2023.
Edited by:
Luca Sorriso-Valvo, Institute for Space Physics, SwedenReviewed by:
Jiajia Liu, University of Science and Technology of China, ChinaMarkus Aschwanden, Lockheed Martin, United States
Copyright © 2023 Sterling, Moore, Panesar, Samanta, Tiwari and Savage. This is an open-access article distributed under the terms of the Creative Commons Attribution License (CC BY). The use, distribution or reproduction in other forums is permitted, provided the original author(s) and the copyright owner(s) are credited and that the original publication in this journal is cited, in accordance with accepted academic practice. No use, distribution or reproduction is permitted which does not comply with these terms.
*Correspondence: Alphonse C. Sterling, YWxwaG9uc2Uuc3RlcmxpbmdAbmFzYS5nb3Y=