- 1Integrative Biotechnology, University of Applied Sciences Kaiserslautern, Kaiserslautern, Germany
- 2Institute of Experimental Physics, Freie Universität Berlin, Berlin, Germany
- 3Dahlem Centre for Plant Sciences, Freie Universität Berlin, Berlin, Germany
- 4Department of Biotechnology, University of Verona, Verona, Italy
- 5Scientific coordinator of Astroland Agency, Logroño, Spain
- 6Parque Científico y Tecnológico de Cantabria, Santander, Spain
Subterranean environments on Earth serve as an analog for the study of microbes on other planets, which has become an active area of research. Although it might sound contradictory that photosynthetic cyanobacteria thrive in extreme low light environments, they are frequent inhabitants of caves on Earth. Throughout the phylum these cyanobacteria have developed unique adaptations that cannot only be used for biotechnological processes but also have implications for astrobiology. They can, for example, both accommodate for the low light conditions by producing specific pigments that allow photosynthesis in near-infrared (IR) radiation/far-red light, and they can synthesize bioplastic compounds and calcium carbonate sheaths which represent valuable resources during human colonization of other planets or rock bodies. This article will highlight the potential benefits of cave-inhabiting cyanobacteria and will present a suitable bioreactor technique for the utilization of these special microbes during future space missions.
1 Introduction
For human colonies to be installed on currently unhabitable planets preparation of the area of interest is crucial (Levchenko et al., 2021). Creating such an enclosed space where humans can operate under preferred conditions -also known as the biosphere- is likely not achievable on large scales, but will need to be achieved gradually. As an initial step, this includes a breathable atmosphere with a constant supply of oxygen, a moderate temperature range and protection from cosmic rays. At a later stage, resources are needed for the construction, maintenance of the colonies and food production. All of this must include a sustainable, circular low-waste concept, most preferably based on resources from the planet of interest (Ceylan, 2018; Santo, 2022). In the past, the concept of natural caves has been used as a test scenario for colonizing extra-terrestrial environments (Walden et al., 1998). Caves on Mars, for example, would not only be shielded from damaging ionizing and UV radiation, but also offer protection against small-scale meteorite impacts (e. g., Walden et al., 1998), and extreme weather conditions such as the dust storms that typically affect the Martian surface (Ryan and Sharman, 1981; Viúdez-Moreiras et al., 2019). These subterranean environments could protect astronauts during early human missions to other planets and could act as primary producers creating hospitable biospheres.
While Mars contains large and interconnected cave systems across the planet (Cushing et al., 2007; Sauro et al., 2020), the Earth’s Moon is well known for its craters and lunar tubes (Chappaz et al., 2017; Kaku et al., 2017). Such pit craters, formed from the collapsed ceilings of subsurface void spaces such as natural caves or lava tubes, have been detected on almost every rock body within the inner Solar System (Wyrick et al., 2004; Haruyama et al., 2009; Davey et al., 2013; Titus et al., 2021): so far, approximately 2,660 subsurface access points have been cataloged on Solar System bodies (Titus et al., 2021), and since the discovery of the first caves on Mars, the spacecraft NASA’s robotic Mars Odyssey Orbiter has helped identify over 1,000 probable gaps on the Red Planet that have been compiled into the Mars Global Cave Candidate Catalog, also known as the MGC (Cushing, 2017). Recently it has been shown that some of the lunar pits and caves provide a temperature around 17°C with a stable and safe thermal environment for long-term exploration and habitation of the Moon (Horvath et al., 2022; Rodeghiero et al., 2022). In addition, the ultraviolet radiation environment and shielding in pit craters and cave skylights on Mars can now be reliably modeled and demonstrated the protective character of such subterranean environments (Viúdez-Moreiras et al., 2021). Studying caves on Earth could thus support the prediction of conditions in cavities on other planets or rock bodies (Sauro et al., 2019; Sauro et al., 2020).
Lava tunnels and cave-like structures have been observed with the use of Mars satellite imaging and special measuring tools. They have been generally considered as suitable environments to search for life and biomarkers (Léveillé and Datta, 2010). However, photosynthetic bacteria such as cyanobacteria are one of the most promising cave-inhabitants in this context. They have evolved on Earth 3.5 mya (Sanchez-Baracaldo and Cardona, 2020; Hickman-Lewis et al., 2022) and are adapted to a wide range of environments, ranging from both hot and cold deserts (Friedmann, 1980; Wynn-Williams, 2000; Lacap-Bugler et al., 2017) with strong radiation (Rastogi et al., 2014) to high salinity habitats (Oren, 2015) and symbioses with lichens (Spribille et al., 2022) or plants (Rai et al., 2000). In addition, they have been proposed as suitable, multi-purpose microorganisms that are supportive during human missions to other planets including the selection of model strains (e.g., Ramalho et al., 2022). Researchers are able to not only isolate and grow them under artificial conditions, but also use them for large-scale industrial production of natural goods (Mutale-Joan et al., 2022). They are regularly found in high abundances and diversity in caves around the globe (Miscoe et al., 2016; Behrendt et al., 2020; Popović et al., 2020), possibly due to the relative constant conditions found in these habitats. In the following we review the suitability of cave-inhabiting cyanobacteria for colonization of other planets with a special emphasis on biotechnological aspects as demonstrated in Figures 1, 2.
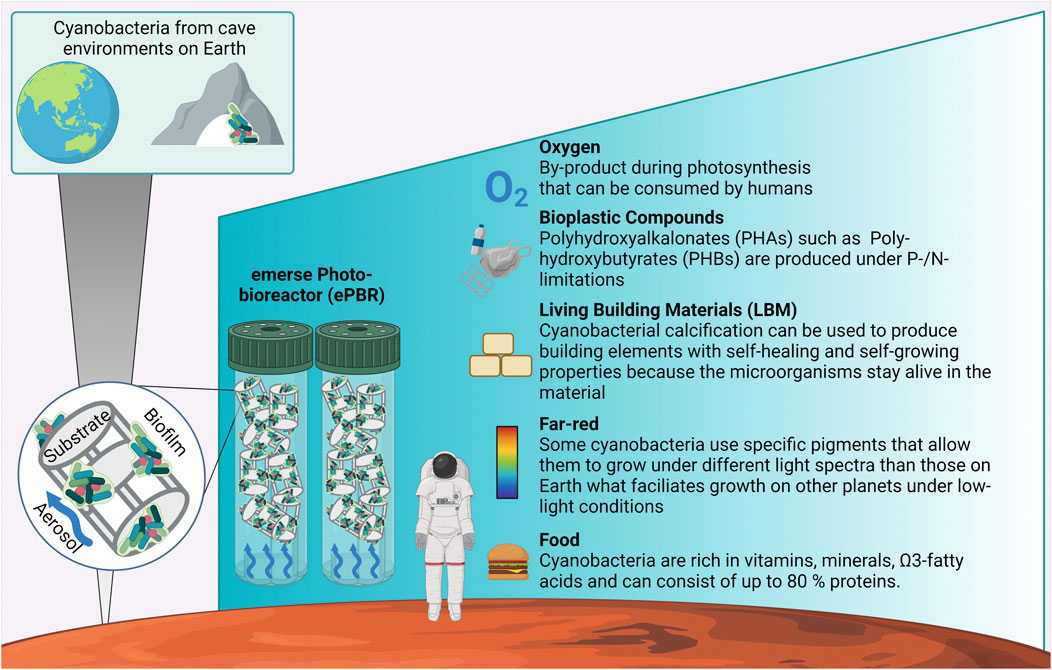
FIGURE 1. Potential of cave-inhabiting cyanobacteria as a model for astrobiology. Cyanobacteria from caves on Earth possess unique properties that make them great candidates for biotechnological applications during human colonization of other planets or the Earth’s Moon. Using minimum resources they can be grown in emerse photo-bioreactors (ePBR) that produce an aerosol directed over surfaces of biocarriers on which the cyanobacterial biofilm establishes. This technique allows the manufacturing of important and yet in this context ignored products such as living building materials or bioplastic compounds.
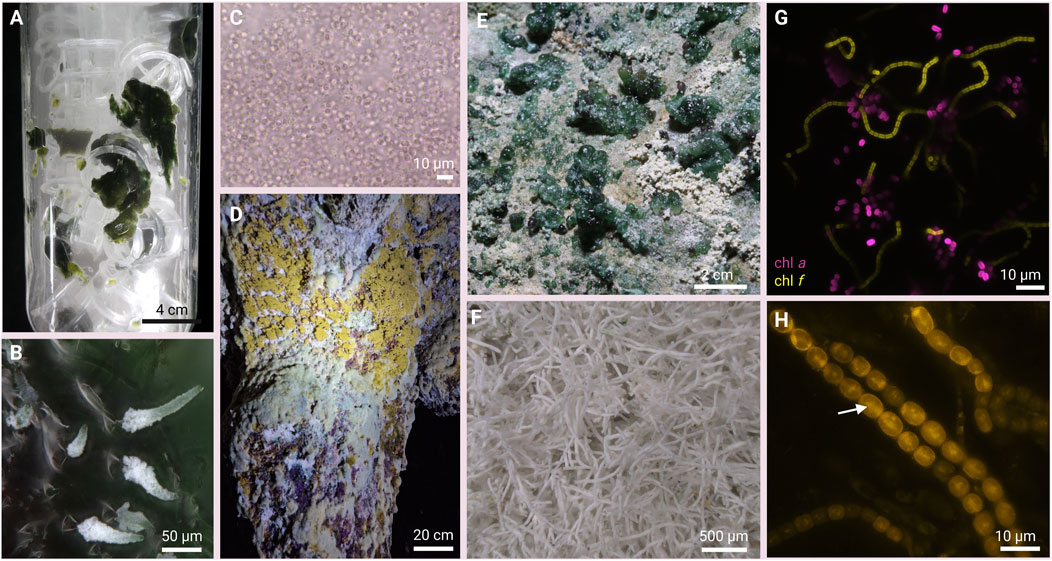
FIGURE 2. Cyanobacteria from the cave environment. (A) emerse photobioreactor (ePBR) filled with biocarriers as substrate on which cyanobacteria grow. The reactor is aerated with aerosol as the medium. (B) isolated Scytonema sp. with calcified tip filaments under laboratory conditions. (C) microscopy of Gloeobacter sp. (D, E) natural cyanobacteria dominated biofilms from caves. (F) calcified natural sheaths of Geitleria sp. (G) confocal laser scanning microscopy (CLSM) showing cyanobacteria with emission wavelengths characteristic for chl d/ f (yellow; 725–750 nm) and chl a and phycobilisomes (magenta; 650–700 nm). (H) Nile red staining and fluorescence of Nostoc sp. Isolated from the cave biofilms showing PHB-rich granules (arrow).
2 Current challenges and new solutions for the efficient cultivation of cyanobacteria
Before devising any new biotechnological application where cyanobacteria can be utilized -irrespectively whether on Earth or elsewhere- one needs to consider how cyanobacteria accumulate biomass and how these cultivation techniques need to be adapted. One of the major setbacks for cyanobacterial applications in industry as of today is the high amount of water used for submersed cultivations. During this process cyanobacterial biomass is, for example, cultivated in large open ponds filled with hundreds of liters of medium and stirred for several weeks under natural light conditions. To harvest biomass gained under these conditions, cells must be separated from the medium, resulting in poor biomass to water yields, usually of about <1% (w/v), and great amounts of wastewater that can hardly be recycled due to contaminations (Johnson et al., 2018). Moreover, most strains that are currently used in commercial approaches are aquatic cyanobacterial strains with a low acclimatization potential (e.g., artificial light and temperature regime in bioreactors) and from which only a few products can be derived, such as food supplements or pigments (Khalifa et al., 2021). However, there are also photobioreactors that were specifically designed to grow cyanobacteria as a life-support system on Mars (Verseux et al., 2021), but also these systems are based on submersed systems and were mostly tested for aquatic cyanobacterial strains.
A new biotechnological approach to cyanobacterial farming is the use of emerse -air exposed- biofilm photobioreactors (ePBRs; Figures 1, 2A), where only a little amount of aqueous medium is needed for aerosolization over cyanobacterial biofilms that grow immobilized on surfaces (Lakatos and Strieth, 2017; Scherer et al., 2022). This method is resource-friendly because the wetting of biofilms only with aerosols saves approximately 90% of water investment compared to submerse cultivation methods. Moreover, the energy consumption for dewatering in downstream processing can be up to 82 MJ per kg dry biomass (Chisti 2007; Jorquera et al., 2010; Ozkan et al., 2012). Consequently, the production of 1 kg biomass requires an energy input of 385 MJ kg−1 in a commercial tubular system and 9.18 MJ kg-1 in a raceway pond—both including mixing during cultivation (Chisti, 2007; Jorquera et al., 2010) while biofilm reactors only consume 4.7 MJ kg−1 because mixing and dewatering are no crucial process steps (Ozkan et al., 2012). In addition, in biofilm photobioreactors the cell density is up to 90 times higher in comparison to submerge technologies like open ponds or closed systems (Jorquera et al., 2010; Ozkan et al., 2012; Podola et al., 2017). Especially the ePBR-technology is suited for terrestrial cyanobacteria showing a much broader ecological spectrum in terms of light quantity and quality, pH, desiccation, temperature and other abiotic factors (Kuhne et al., 2014; Lakatos and Strieth 2017). Terrestrial cyanobacteria from e.g. biocrusts of arid deserts experience, for example, great temperature amplitudes as the soil heats up during the day and cools down at night and they need to be capable of using also short hydration events provided by, e.g., fog (Jung et al., 2019) while aquatic cyanobacteria constantly have a water supply and the water column has a constant temperature. Terrestrial cyanobacteria from caves could potentially not only photo-acclimatize towards the shifted light spectrum that we expect from other planets, but they could also be key to new products and materials created with the ePBR technology. Among these are for example induced calcification processes that result in so-called living building materials (LBMs) or bioplastic compounds among others (Figure 1).
3 Ecology and benefits of cave-inhabiting cyanobacteria for future applications
Cyanobacteria are the oldest living microorganisms capable of oxygenic photosynthesis on Earth and as a result, they are highly adapted to a wide range of environments (Chen et al., 2021). They are known to be pioneer organisms, capable of conquering new habitats and laying the foundation for whole ecosystems as primary producers and due to their ability of priming soils (Muñoz-Rojas et al., 2018; Chen et al., 2021). The Great Oxygenation Event during the Paleoproterozoic era demonstrated what photosynthetic microorganisms are capable of as this was the time interval when the Earth’s atmosphere and the shallow ocean first experienced a rise in the amount of oxygen (Knoll and Nowak, 2017). This injection of toxic oxygen into an anaerobic biosphere probably caused the extinction of many existing anaerobic species present on Earth at that time (Hodgskiss et al., 2019).
It has been predicted that extra-terrestrial photosynthesis might be possible where an Earth-like atmosphere is present and water can be supplied (Lingam and Loeb, 2020). On planetary surfaces, several factors could impair cyanobacterial colonization (Verseux et al., 2016), although some highly resistant strains have already been discovered (Billi et al., 2022). Especially cyanobacteria from extreme terrestrial habitats on Earth have already been shown to be suitable candidates to not only survive travelling through Space (Napoli et al., 2022), they could also grow under Space- and Mars-like conditions (de Vera et al., 2019), and on Martian regolith (Baqué et al., 2013). In particular, Chroococcidiopsis sp. CCMEE 029 from the Negev desert has been extensively studied and reported to withstand years of desiccation and the exposure to space and Mars-like stimulations (Billi, 2009; Billi et al., 2019). Their ability to withstand such extremes is due to a great amplitude of mechanisms such as the formation of extra cellular polymeric substances (EPS) that provide physical protection and prevent desiccation (Rossi and De Philippis, 2015) and also a variety of pigments that increases their resistance against UV irradiation (Sinha and Häder, 2008).
In contrast, caves represent a protected habitat with reduced abiotic stressors such as high irradiance, UV/gamma radiation, desiccation, different contaminants and fast temperature variations, at the cost of limited light availability (Billi et al., 2022). On Earth, caves show high (cyano)bacterial diversity (Hauer et al., 2015; Popović et al., 2020; Prescott et al., 2022) and could represent suitable growth environments on other planetary bodies featuring relatable conditions (Wynne et al., 2021). These conditions are represented by a stable temperature regime, rough surface textures and constant as well as continuous humidity supplies provided by runoff- or seepage water (Figures 2D, E). Karst areas are especially prone to form caves across the globe since the main component of limestone is calcium carbonate that easily gets dissolved by water. Such cave systems can be colonized by naturally occurring phototrophic microbial communities (Roldán and Hernández-Mariné, 2009) or their establishment can be induced by artificial lightning in touristic show caves, also known as Lampenflora (Mulec, 2019).
A common unicellular species of cyanobacteria in natural caves is Gloeobacter violaceus (Mareš et al., 2013; Figure 2C), representing one of the oldest lineages of modern cyanobacteria. The genus Gloeobacter is characterized by the lack of thylakoid membranes and the positioning of the photosynthetic machinery in the cytoplasmic membrane which makes it an interesting model for biotechnology and research on the origin of photosynthesis (Engqvist et al., 2015).
Other low-light adapted cyanobacteria from cave environments possess specialized chlorophylls, such as chlorophyll d and chlorophyll f, capable of absorbing far-red light in addition to visible light. The presence of far-red cyanobacteria as a consequence of the spectral shift towards longer wavelengths has been recently reported for cave systems (Behrendt et al., 2020). The ability to produce such pigments could allow the growth of cyanobacteria on planets and rock bodies exposed to different light spectra than Earth (Billi et al., 2022).
In addition, many filamentous cyanobacteria can be found in caves, some of which are well known to fix atmospheric nitrogen and to form calcium carbonate sheaths around their cells. Among the most prominent examples for such calcifying cyanobacteria are Geitleria spp. (Kilgore et al., 2018; Figure 2F) and Scytonema julianum (Couté and Bury, 1988; Figure 2B). Especially cells of the latter are encased with thick, well-developed calcified sheaths with external diameters of 11–25 μm, which develop through the sequential precipitation of amorphous CaCO3, acicular calcite crystals, triradiate calcite crystals, and dendrite calcite or aragonite crystals (Figure 2F; Jones and Peng, 2014) with a high degree of mineral variability (Hoffmann, 1992). Employing such cyanobacteria in the biomineralization of carbon dioxide by calcium carbonate precipitation has been suggested to offer self-sustaining strategies for point-source carbon capture and sequestration in biotechnological processes (Jansson and Northen, 2010; Phillips et al., 2013).
Interestingly, a high proportion of cave inhabiting cyanobacterial taxa have recently been shown to produce biocompatible and biodegradable bioplastics e.g., polyhydroxyalkanoates such as polyhydroxybutyrate (PHA / PHB; Figure 2H) with various application potential (Djebaili et al., 2022). Cyanobacteria have minimal nutrient requirements for growth and accumulate PHAs and PHBs through oxygenic photosynthesis under nutrient-limited conditions, such as nitrogen and/or phosphate starvation, where cells enter a quiescent state known as chlorosis (Koch et al., 2020a).
Next to the production of oxygen, dietary supplement, dyes, and food, the mentioned aspects such as far-red adaptations, PHB production, calcification, and biotechnological advantages make cave-inhabiting cyanobacteria suitable and multifunctional candidates to study the requirements for life on other planets.
4 Far-red light photosynthetic adaptations in Cyanobacteria
In limestone caves light forms a spectral gradient where, from the entrance to greater depths of the cavity, the visible component gradually becomes limiting while the far-red (FR, above 700 nm) portion of the spectrum is enriched (Behrendt et al., 2020). This is comparable to the Earth’s Moon, where features such as lava tube-related sinkhole chains present temperatures and radiance levels (Horvath et al., 2022; Rodeghiero et al., 2022). That could be compatible with cyanobacterial life. Due to the fact, that materials such as regolith and basalts show an increased reflectivity in the red/IR region (Anbazhagan and Arivazhagan, 2009; Gaier et al., 2012; Xu et al., 2021), a FR enrichment analogous to Earth caves could be expected. Similar features (Sauro et al., 2020) and materials (Mandon et al., 2022) can be found on Mars, whose caves might also present a more hospitable environment than the surface (Léveillé and Datta, 2010; Irwin and Schulze-Makuch, 2020). Further away from the Solar System, for habitable zone planetary conditions, the light spectrum emitted by M-stars (red dwarfs), albeit red-shifted compared to the Sun, could sustain oxygenic photosynthesis in cyanobacteria (Claudi et al., 2020), as could K-stars (Covone et al., 2021).
Cyanobacteria can modulate their photosynthetic activity according to light spectral distribution via several different processes designated chromatic acclimation (CA) (Sanfilippo et al., 2019). Some species, in response to FR light enriched exposure, show a CA called Far-Red Light Photoacclimation (FaRLiP). This process induces profound changes in the composition of photosystems, antennae and chlorophyll content, by synthesizing chl f and chl d (Figure 2G) in addition to chl a (Gan et al., 2014). Even if in FR acclimated cells chl f constitutes only about 12% of the total chlorophyll content, it is not only an accessory pigment, but it is instead present in both photosystems and it is directly involved in charge separation (Nürnberg et al., 2018). In contrast, in most species of the genus Acaryochloris where chl d represents the primary photopigment, photosynthetic use of FR light is a constitutive and more restrictive adaptation than FaRLiP (Wolf and Blankenship, 2019; Viola et al., 2022).
FR light adaptations allow cyanobacterial growth in widespread ecological niches, including caves, lakes, beach rock, deserts, hot spring mats, and subtropical forests (Antonaru et al., 2020). Cave light gradients can be considered, on a much bigger scale, comparable to vertical micro-gradients observed in biofilms, desert crusts or endolithic habitats, where the upper layers absorb the visible component, while FR light penetrates into deeper layers. Both cave- and micro gradient-induced FR light enrichment lead to a substantial increase in the growth of FR-adapted cyanobacteria (Behrendt et al., 2012; Behrendt et al., 2020; Kühl et al., 2020).
Far-red adaptations could then give cyanobacteria a crucial advantage in space biology applications, on the surface but especially in caves. For instance, direct light emitted by M-stars allows the growth of FaRLiP and chl d dependent cyanobacteria with little advantage over non-FR adapted strains (Ritchie et al., 2018; Claudi et al., 2020). In fact, while in those conditions Acaryochloris potential productivity estimate has been considered the highest among other commonly (or widely) used oxygenic phototrophs (e.g., 0.178 vs. 0.155 g C m−2 h−1 in Synechococcus sp. PCC 7942; Ritchie et al., 2018), a major drawback of FaRLiP strains is that they show reduced growth rates if they are cultivated exclusively under far-red light (Claudi et al., 2020). For Leptolyngbya sp. JSC-1, for example, it was shown that its doubling time is four times lower than in white (visible) light (Nien et al., 2022). Another FaRLiP strain, Halomicronema hongdechloris utilizes both chl a and chl f for photosynthesis under FR low light conditions (chl content per cell: 1.54 mg chl g−1 cell wet weight), nevertheless, and grows more rapidly than it does under WL (white light) low light conditions (chl content per cell: 1.0 mg chl g−1 cell wet weight; Li et al., 2014). Additionally, it has been shown that growth rates of some isolates can reach up to 80% by mimicking the field far-red light intensities of 6–8 μmol photons m−2 s−1 in comparison to those under the above described white light conditions, suggesting that chl f and chl d contributed effectively to cyanobacterial growth (Zhang et al., 2019). However, being in the presence of an already red-shifted spectrum, FR light adaptations could be vital for growing in further FR enriched environments as how expoplanet caves could be. Another prospect for space applications could be to genetically engineer non-FR-adapted cyanobacterial strains in order to allow the synthesis of red-shifted chlorophylls and extend the available light spectrum (Luan et al., 2020; Liu et al., 2021). Potential background strains could be chosen for improvements in biomass production (Verseux et al., 2016; Liu et al., 2021; Ramalho et al., 2022), mineral resources extraction (Olsson-Francis and Cockell, 2010), or resistance to extreme conditions (Billi et al., 2022). Preliminary studies to partially incorporate FaRLiP components have already been performed in cyanobacteria (Shen et al., 2019; Trinugroho et al., 2020; Tros et al., 2020), albeit so far, a full integration of the complete gene set has yet to be achieved. Such strains could be promising candidates for the cultivation in ePBRs on other planets as they effectively use the underlying natural light spectrum.
5 Cyanobacterial bioplastic production
Biodegradable and biocompatible materials such as PHAs/PHBs rapidly gained attention over the last decades (Anastas and Kirchhoff, 2002; Lenz and Marchessault, 2005). PHBs are microbially produced polymers (Figure 2H), which act as storage units for both energy and carbon (Williams et al., 1999; Steinbüchel and Lütke-Eversloh, 2003). Microbial PHB production depends on external triggers, such as nitrogen or phosphate starvation as well as an oversupply of carbon sources (Steinbüchel and Lütke-Eversloh, 2003). Industrial bio-based production solely relies on heterotrophic microorganisms, which require a carbon feedstock, mostly polysaccharides (Chavez et al., 2022). This production bears some disadvantages: firstly, the loss of these feedstocks as food-source and secondly, the high costs of the feedstocks.
Material properties of PHBs are comparable to petroleum-based polymers such as polypropylene or polyethylene in terms of elasticity module and tensile strength (Chee et al., 2018). PHBs are biodegradable and can be degraded enzymatically by soil microorganisms, which makes them even more appealing regarding plastic pollution (Madison and Huisman, 1999).
With cyanobacteria being able to produce a broad range of PHBs from CO2 and light, feedstock costs are low, making production partly attractive (Lee et al., 2021). Setbacks such as longer cultivation times have yet to be overcome (Das and Maiti, 2021; Lee et al., 2021). A case study about caves in Italy showed the capabilities of cave-inhabiting cyanobacteria to produce PHBs (Djebaili et al., 2022). Dark periods are somehow crucial for PHB production (e.g., Ansari and Fatma 2016), but why the ability for PHB biosynthesis in cave cyanobacteria is widespread remains unknown yet.
Compared to petroleum-based manufacturing processes, bio-based production requires fewer facilities: bioreactors with a subsequent extraction step are sufficient, which means that fewer materials would have to be deployed to other planets (Harding et al., 2007). Recent studies focused on blending PHBs with different materials or using chemical engineering to diversify material properties in order to further extend the application range (Chee et al., 2018; Turco et al., 2021). As of today, pilot scale projects are on the rise, using cyanobacteria firstly to reduce the carbon footprint of processes (power plants) and to produce PHBs, as started in 2011 by the Austrian producer of electricity EVN. Commercially available PHBs are mostly produced with bacteria; however, genetic engineering of cyanobacteria also makes them more and more appealing for industrial application (Koch et al., 2020b).
Based on these findings PHBs could be produced extra-terrestrially on-site as a platform compound with different post-productional blendings or chemical alterations to suit various applications in the biomedical, building or packaging sector. If combined with 3D printing technologies, product manufacturing could be fully automated and remotely supervised to install facilities before human colonization of other planets (Frone et al., 2019; Chiulan et al., 2021). The biodegradability of PHBs also possesses one more crucial advantage, the potential to establish a zero-waste circular economy that decreases the resource demands (Talan et al., 2022).
6 Cyanobacterial calcification and applications
Calcification, the process of biomineralization of CO2 by calcium carbonate (CaCO3) precipitation, is a widely occurring phenomenon in ecosystems and plays a crucial role in the biogeochemical carbon cycle (Gattuso and Buddemeier, 2000; Ridgwell and Zeebe, 2005). The first descriptions of entanglement between microorganisms and calcification were presented many years ago for the process of stromatolite formation (Logan et al., 1964).
Mostly, calcification is known for its role in deep-sea carbon storage, carried out by marine microorganisms, especially cyanobacteria and coccolithophores (Westbroek et al., 1984; Planavsky et al., 2009; Milner et al., 2016; Feng et al., 2017; Kalokora et al., 2020). However, calcification is not exclusively taking place in aquatic environments. It can also be observed in terrestrial habitats e.g. by aerophytic cyanobacteria (Hodač et al., 2015; Kamran et al., 2020). Here, a special emphasis should be given to cave-inhabiting cyanobacteria, since numerous studies found them capable of calcification when associated with a Ca2+ rich source, such as in karst caves (Figures 2B, F; Bourrelly and Dupuy, 1973; Arino et al., 1997). One theory states the potential for photon entrapment by distinct crystal structures, such as aragonite, which was shown for stone corals (Acropora sp.) and referred to as luminescent light collection (Neumann-Micheau and Tributsch, 2018). This theory could also be extrapolated to cave inhabiting cyanobacteria under low light conditions.
Although the mechanisms of this process remains largely unknown, biotechnological applications for calcification are on the rise. In terms of carbon-capturing processes, microbially produced CaCO3 sheaths represent an inorganic carbon sink (Jansson and Northen, 2010). The usage of cyanobacteria exhibits a double effect on the carbon utilization capacity, because firstly, they are fixing CO2 producing organic molecules during growth and photosynthesis and secondly capture inorganic carbon (CO2 / HCO3− / CO32−) during calcification. Biotechnology has experimented with calcifying microorganisms in the field of sustainable and eco-friendly building materials (De Muynck et al., 2010; Wang et al., 2012). A recent study by Heveran and colleagues presented a proof of concept where calcifying Synechococcus sp. PCC 7002 can be used to build actual concrete like-building blocks, which they referred to as living building materials (LBMs) (Williams et al., 2019; Heveran et al., 2020). While the use of cyanobacteria for manufacturing of concrete type building materials may seem far away, first steps have been taken for an industrial approach realization. The company Prometheus Materials (Colorado, United States), for example already developed an algae-based construction material with a low carbon footprint. Also, Minus Materials (Colorado, United States) aims for what they refer to as biogenic limestone to replace concrete as building material. The industrial application in this field rapidly increases leaving room for more exploration, even in Space.
When thinking about installing permanent infrastructure for human residence on another planet / Moon, building materials from local resources is a must-have to decrease the deployment of materials from Earth by expensive space launches. If calcium and CO2 were present in considerable amounts at the destined exo-planet, one could think about using cyanobacteria for manufacturing LBMs according to the published protocols of Heveran et al. (2020). Regarding the presence of lunar regolith, it might be possible to use these sands in a mixture with grown cyanobacterial biomass. According to NASA’s data, lunar regolith is mainly composed of silicon (45.4%) but also bears a considerable amount of calcium (11.8%) (Taylor, 1975). Notably, a study also analyzed the distribution of plagioclase (a calcium-rich feldspar) on the Moon, and showed a high abundance of this material (Ohtake et al., 2009; Baasch et al., 2021). Moon mining approaches, combined with the fabrication of LBMs and 3D printing technologies, could provide a low cost-solution for building infrastructure on Earth’s Moon and other planets (Cesaretti et al., 2014; Vítek et al., 2020).
Author contributions
PJ prepared the first draft of the manuscript and the figures. All authors contributed figures, revised and edited the manuscript and approved the final version.
Funding
The project is funded by the German Research Foundation (DFG) with the grant number JU 3228/1-1 to PJ and NU 421/1 to DJN. The joint project “Cyanolessinia” of FB is funded by the University of Verona (Italy) with the grant number JPVR19S9PT. ML is funded by the Ministry of Science and Health Rhineland-Palatinate (PhytoBioTech, 724-0116#2021/004-1501 15,405) and by the Federal Ministry of Education and Research (W2V-Strategy2Value, 03WIR4502A & Technology2Value 03WIR4504B). Moreover, ML and FH are supported by EU-HORIZON (Waste2BioComp ID: 101058654).
Acknowledgments
AG wants to thank Antonio Ordóñez, founder of Virtual Biodiversity (BV). All authors want to thank Astroland Agency and their astrobiology project within the framework of which this work was conducted.
Conflict of interest
The authors declare that the research was conducted in the absence of any commercial or financial relationships that could be construed as a potential conflict of interest.
Publisher’s note
All claims expressed in this article are solely those of the authors and do not necessarily represent those of their affiliated organizations, or those of the publisher, the editors and the reviewers. Any product that may be evaluated in this article, or claim that may be made by its manufacturer, is not guaranteed or endorsed by the publisher.
References
Anastas, P. T., and Kirchhoff, M. M. (2002). Origins, current status, and future challenges of green chemistry. Chem. Res. 35, 686–694. doi:10.1021/ar010065m
Anbazhagan, S., and Arivazhagan, S. (2009). Reflectance spectra of analog basalts; implications for remote sensing of lunar geology. Planet. Space Sci. 5712, 1346–1358. doi:10.1016/j.pss.2009.06.020
Ansari, S., and Fatma, T. (2016). Cyanobacterial polyhydroxybutyrate (PHB): Screening, optimization and characterization. PloS one 11 (6), e0158168. doi:10.1371/journal.pone.0158168
Antonaru, L. A., Cardona, T., Larkum, A. W., and Nürnberg, D. J. (2020). Global distribution of a chlorophyll f cyanobacterial marker. ISME J. 14 (9), 2275–2287. doi:10.1038/s41396-020-0670-y
Arino, X., Hernandez-Marine, M., and Saiz Jimenez, C. (1997). Colonization of Roman tombs by calcifying cyanobacteria. Physiologica 36, 366–373. doi:10.2216/i0031-8884-36-5-366.1
Baasch, J., Windisch, L., Koch, F., Linke, S., Stoll, E., and Schilde, C. (2021). Regolith as substitute mold material for aluminum casting on the Moon. Acta Astronaut. 182, 1–12. doi:10.1016/j.actaastro.2021.01.045
Baqué, M., de Vera, J. P., Rettberg, P., and Billi, D. (2013). The BOSS and BIOMEX space experiments on the EXPOSE-R2 mission: Endurance of the desert cyanobacterium Chroococcidiopsis under simulated space vacuum, Martian atmosphere, UVC radiation and temperature extremes. Acta Astronaut. 91, 180–186. doi:10.1016/j.actaastro.2013.05.015
Behrendt, L., Larkum, A. W., Trampe, E., Norman, A., Sørensen, S. J., and Kühl, M. (2012). Microbial diversity of biofilm communities in microniches associated with the didemnid ascidian Lissoclinum patella. ISME J. 66, 1222–1237. doi:10.1038/ismej.2011.181
Behrendt, L., Trampe, E. L., Nord, N. B., Nguyen, J., Kühl, M., Lonco, D., et al. (2020). Life in the dark: Far-red absorbing cyanobacteria extend photic zones deep into terrestrial caves. Environ. Microbiol. 22 (3), 952–963. doi:10.1111/1462-2920.14774
Billi, D., Napoli, A., Mosca, C., Fagliarone, C., De Carolis, R., Balbi, A., et al. (2022). Identification of far-red light acclimation in an endolithic Chroococcidiopsis strain and associated genomic features: Implications for oxygenic photosynthesis on exoplanets. Front. Microbiol. 13, 933404. doi:10.3389/fmicb.2022.933404
Billi, D. (2009). Subcellular integrities in Chroococcidiopsis sp. CCMEE 029 survivors after prolonged desiccation revealed by molecular probes and genome stability assays. Extremophiles 13, 49–57. doi:10.1007/s00792-008-0196-0
Billi, D., Verseux, C., Fagliarone, C., Napoli, A., Baqué, M., and de Vera, J.-P. (2019). A desert cyanobacterium under simulated mars-like conditions in low earth orbit: Implications for the habitability of Mars. Astrobiology 19 (2), 158–169. doi:10.1089/ast.2017.1807
Bourrelly, P., and Dupuy, P. (1973). Quelques stations françaises de Geitleria calcarea, Cyanophycée cavernicole. Schweiz. z. Hydrol. 35, 136–140. doi:10.1007/bf02502067
Cesaretti, G., Dini, E., Kestelier, X. D., Colla, V., and Pambaguian, L. (2014). Building components for an outpost on the Lunar soil by means of a novel 3D printing technology. Acta Astronaut. 93, 430–450. doi:10.1016/j.actaastro.2013.07.034
Ceylan, S. (2018). Space, architecture, and science fiction: An architectural interpretation of space colonization. Int. J. Constr. Environ. 9 (2), 1–17. doi:10.18848/2154-8587/cgp/v09i02/1-17
Chappaz, L., Sood, R., Melosh, H. J., Howell, K. C., Blair, D. M., Milbury, C., et al. (2017). Evidence of large empty lava tubes on the Moon using GRAIL gravity. Geophys. Res. Lett. 44 (1), 105–112. doi:10.1002/2016gl071588
Chavez, B. A., Raghavan, V., and Tartakovsky, B. (2022). A comparative analysis of biopolymer production by microbial and bioelectrochemical technologies. RSC Adv. 12, 16105–16118. doi:10.1039/d1ra08796g
Chee, J., Yeo, C., Muiruri, J. K., Thitsartarn, W., and Li, Z. (2018). Recent advances in the development of biodegradable PHB-based toughening materials: Approaches, advantages and applications. Mat. Sci. Eng. C 92, 1092–1116. doi:10.1016/j.msec.2017.11.006
Chen, M.-Y., Teng, W. K., Zhao, L., Hu, C. X., Zhou, Y. K., Han, B. P., et al. (2021). Comparative genomics reveals insights into cyanobacterial evolution and habitat adaptation. ISME J. 15, 211–227. doi:10.1038/s41396-020-00775-z
Chisti, Yusuf (2007). Biodiesel from microalgae. Biotechnol. Adv. 25 (3), 294–306. doi:10.1016/j.biotechadv.2007.02.001
Chiulan, I., Frone, A. N., Brandabur, C., Mihaela, D., and Id, P. (2021). Recent advances in 3D printing of aliphatic polyesters. Bioengineering 5, 2–18. doi:10.3390/bioengineering5010002
Claudi, R., Alei, E., Battistuzzi, M., Cocola, L., Erculiani, M. S., Pozzer, A. C., et al. (2020). Super-earths, M dwarfs, and photosynthetic organisms: Habitability in the lab. Life 1, 10. doi:10.3390/life11010010
Couté, A., and Bury, E. (1988). Ultrastructure d'une cyanophycée aérienne calcifiée cavernicole: Scytonema julianum (frank) richter (hormogonophycideae, nostocales, scytonemataceae). Hydrobiologia 160 (3), 219–239. doi:10.1007/bf00007137
Covone, G., Ienco, R. M., Cacciapuoti, L., and Inno, L. (2021). Efficiency of the oxygenic photosynthesis on Earth-like planets in the habitable zone. Mon. Notices R. Astronomical Soc. 5053, 3329–3335. doi:10.1093/mnras/stab1357
Cushing, G. E. (2017). “Mars global cave candidate catalog (MGC3),” in Astrobiology Science Conference 2017 (LPI Contrib. No. 1965).
Cushing, G. E., Titus, T. N., Wynne, J. J., and Christensen, P. R. (2007). THEMIS observes possible cave skylights on Mars. Geophys. Res. Lett. 34 (17), L17201. doi:10.1029/2007gl030709
Das, M., and Maiti, S. K. (2021). Recent progress and challenges in cyanobacterial autotrophic production of polyhydroxybutyrate (PHB), a bioplastic. J. Environ. Chem. Eng. 9 (4), 105379. doi:10.1016/j.jece.2021.105379
Davey, S. C., Ernst, R. E., Samson, C., and Grosfils, E. B. (2013). Hierarchical clustering of pit crater chains on Venus. Can. J. Earth Sci. 50 (1), 109–126. doi:10.1139/cjes-2012-0054
De Muynck, W., De Belie, N., and Verstraete, W. (2010). Microbial carbonate precipitation in construction materials: A review. Ecol. Eng. 36, 118–136. doi:10.1016/j.ecoleng.2009.02.006
De Vera, J. P., Alawi, M., Backhaus, T., Baqué, M., Billi, D., Böttger, U., et al. (2019). Limits of life and the habitability of Mars: The ESA space experiment BIOMEX on the ISS. Astrobiology 19 (2), 145–157. doi:10.1089/ast.2018.1897
Djebaili, R., Mignini, A., Vaccarelli, I., Pellegrini, M., Spera, D. M., Del Gallo, M., et al. (2022). Polyhydroxybutyrate-producing cyanobacteria from lampenflora: The case study of the “Stiffe” caves in Italy. Front. Microbiol. 13, 933398. doi:10.3389/fmicb.2022.933398
Engqvist, M. K., McIsaac, R. S., Dollinger, P., Flytzanis, N. C., Abrams, M., Schor, S., et al. (2015). Directed evolution of Gloeobacter violaceus rhodopsin spectral properties. J. Mol. Biol. 427 (1), 205–220. doi:10.1016/j.jmb.2014.06.015
Feng, Y., Roleda, M. Y., Armstrong, E., Boyd, P. W., and Hurd, C. L. (2017). Environmental controls on the growth, photosynthetic and calcification rates of a Southern Hemisphere strain of the coccolithophore Emiliania huxleyi. Limnol. Oceanogr. 62, 519–540. doi:10.1002/lno.10442
Friedmann, E. I. (1980). “Endolithic microbial life in hot and cold deserts,” in Limits of life (Dordrecht: Springer), 33–45.
Frone, A. N., Batalu, D., Chiulan, I., Oprea, M., Gabor, A. R., Nicolae, C. A., et al. (2019). Morpho-structural, thermal and mechanical properties of PLA/PHB/Cellulose biodegradable nanocomposites obtained by compression molding, extrusion, and 3d printing. Nanomaterials 10 (1), 51. doi:10.3390/nano10010051
Gaier, J. R., Ellis, S., and Hanks, N. (2012). Thermal optical properties of lunar dust simulants. J. Thermophys. heat Transf. 264, 573–580. doi:10.2514/1.t3838
Gan, F., Zhang, S., Rockwell, N. C., Martin, S. S., Lagarias, J. C., and Bryant, D. A. (2014). Extensive remodeling of a cyanobacterial photosynthetic apparatus in far-red light. Science 345, 62021312–62021317. doi:10.1126/science.1256963
Gattuso, J., and Buddemeier, R. W. (2000). Calcification and CO 2. Ocean. Biogeochem. 407, 311–313. doi:10.1038/35030280
Harding, K. G., Dennis, J. S., Blottnitz, H. Von, and Harrison, S. T. L. (2007). Environmental analysis of plastic production processes: Comparing petroleum-based polypropylene and polyethylene with biologically-based poly-β-hydroxybutyric acid using life cycle analysis. J. Bioeconomics 130, 57–66. doi:10.1016/j.jbiotec.2007.02.012
Haruyama, J., Hioki, K., Shirao, M., Morota, T., Hiesinger, H., van der Bogert, C. H., et al. (2009). Possible lunar lava tube skylight observed by SELENE cameras. Geophys. Res. Lett. 36 (21), L21206. doi:10.1029/2009gl040635
Hauer, T., Mühlsteinová, R., Bohunická, M., Kaštovskỳ, J., and Mareš, J. (2015). Diversity of cyanobacteria on rock surfaces. Biodivers. conservation 244, 759–779. doi:10.1007/s10531-015-0890-z
Heveran, C. M., Williams, S. L., Qiu, J., Artier, J., Hubler, M. H., Cook, S. M., and Srubar, W. V. (2020). Biomineralization and successive regeneration of engineered living building materials. Matter 2 (2), 481–494. doi:10.1016/j.matt.2019.11.016
Hickman-Lewis, K., Cavalazzi, B., Giannoukos, K., D'Amico, L., Vrbaski, S., Saccomano, G., et al. (2022). Advanced two- and three-dimensional insights into Earth’s oldest stromatolites (ca. 3.5 Ga): Prospects for the search for life on Mars. Geology 51 (1), 33–38. doi:10.1130/G50390.1
Hodač, L., Brinkmann, N., Mohr, K. I., Arp, G., Hallmann, C., Ramm, J., et al. (2015). Diversity of microscopic green algae (Chlorophyta) in calcifying biofilms of two karstic streams in GermanyVariability in the crystal morphology of calcified terrestrial Scytonema populations (Cyanobacteria, Cyanophyceae). Geomicrobiol. JournalGeomicrobiology J. 3210 (3-41), 27559–29064. doi:10.1080/01490459209377904
Hodgskiss, M. S., Crockford, P. W., Peng, Y., Wing, B. A., and Horner, T. J. (2019). A productivity collapse to end Earth’s Great Oxidation. Proc. Natl. Acad. Sci. 116 (35), 17207–17212. doi:10.1073/pnas.1900325116
Hoffmann, L. (1992). Variability in the crystal morphology of calcified terrestrial Scytonema populations (Cyanobacteria, Cyanophyceae). Geomicrobiol. J. 10 (1), 59–64.
Horvath, T., Hayne, P. O., and Paige, D. A. (2022). Thermal and illumination environments of lunar pits and caves: Models and observations from the diviner lunar radiometer experiment. Geophys. Res. Lett. 49, 1–9. doi:10.1029/2022gl099710
Irwin, L. N., and Schulze-Makuch, D. (2020). The astrobiology of alien worlds: Known and unknown forms of life. Universe 9, 130. doi:10.3390/universe6090130
Jansson, C., and Northen, T. (2010). Calcifying cyanobacteria—The potential of biomineralization for carbon capture and storage. Curr. Opin. Biotechnol. 21 (3), 365–371. doi:10.1016/j.copbio.2010.03.017
Johnson, T. J., Anderson, G. A., Gibbons, W. R., Gu, L., and Zhou, R. (2018). Photobioreactor cultivation strategies for microalgae and cyanobacteria. Am. Inst. Chem. Eng. 34, 811–827. doi:10.1002/btpr.2628
Jones, B., and Peng, X. (2014). Multiphase calcification associated with the atmophytic cyanobacterium Scytonema julianum. Sediment. Geol. 313, 91–104. doi:10.1016/j.sedgeo.2014.09.002
Jorquera, O., Kiperstok, A., Sales, E. A., Embiruçu, M., and Ghirardi, M. L. (2010). Comparative energy life-cycle analyses of microalgal biomass production in open ponds and photobioreactors. Bioresour. Technol. 101 (4), 1406–1413. doi:10.1016/j.biortech.2009.09.038
Jung, P., Schermer, M., Briegel-Williams, L., Baumann, K., Leinweber, P., Karsten, U., et al. (2019). Water availability shapes edaphic and lithic cyanobacterial communities in the Atacama Desert. J. Phycol. 55 (6), 1306–1318. doi:10.1111/jpy.12908
Kaku, T., Haruyama, J., Miyake, W., Kumamoto, A., Ishiyama, K., Nishibori, T., et al. (2017). Detection of intact lava tubes at marius hills on the moon by SELENE (kaguya) lunar radar sounder. Geophys. Res. Lett. 44 (20), 155–161. doi:10.1002/2017gl074998
Kalokora, O. J., Buriyo, A. S., Asplund, M. E., Gullström, M., Mtolera, M. S., and Björk, M. (2020). An experimental assessment of algal calcification as a potential source of atmospheric CO2. PloS one 15 (4), e0231971. doi:10.1371/journal.pone.0231971
Kamran, A., Sauter, K., Reimer, A., Wacker, T., Reitner, J., and Hoppert, M. (2020). Cyanobacterial mats in calcite-precipitating serpentinite-hosted alkaline springs of the Voltri Massif, Italy. Microorganisms 9 (1), 62. doi:10.3390/microorganisms9010062
Khalifa, S. A., Shedid, E. S., Saied, E. M., Jassbi, A. R., Jamebozorgi, F. H., Rateb, M. E., et al. (2021). Cyanobacteria—from the oceans to the potential biotechnological and biomedical applications. Mar. Drugs 19 (5), 241. doi:10.3390/md19050241
Kilgore, C., Johansen, J. R., Mai, T., Hauer, T., Casamata, D. A., and Sheil, C. (2018). Molecular characterization of Geitleria appalachiana sp. nov.(Nostocales, Cyanobacteria) and formation of Geitleriaceae fam. nov, Fottea 18(2):150–163. doi:10.5507/fot.2018.002
Knoll, A. H., and Nowak, M. A. (2017). The timetable of evolution. Sci. Adv. 3. Jg., Nr. 5, S., e1603076. doi:10.1126/sciadv.1603076
Koch, M., Berendzen, K. W., and Forchhammer, K. (2020b). On the role and production of polyhydroxybutyrate (PHB) in the cyanobacterium synechocystis sp. PCC 6803. Life 10, 47. doi:10.3390/life10040047
Koch, M., Bruckmoser, J., Scholl, J., Hauf, W., Rieger, B., and Forchhammer, K. (2020a). Maximizing PHB content in synechocystis sp. PCC 6803: A new metabolic engineering strategy based on the regulator PirC. Microb. Cell Factories 19, 231. doi:10.1186/s12934-020-01491-1
Kühl, M., Trampe, E., Mosshammer, M., Johnson, M., Larkum, A. W., Frigaard, N.-U., et al. (2020). Substantial near-infrared radiation-driven photosynthesis of chlorophyll f-containing cyanobacteria in a natural habitat. Elife 9, e50871. doi:10.7554/elife.50871
Kuhne, S., Strieth, D., Lakatos, M., Muffler, K., and Ulber, R. (2014). A new photobioreactor concept enabling the production of desiccation induced biotechnological products using terrestrial cyanobacteria. J. Biotechnol. 192, 28–33. doi:10.1016/j.jbiotec.2014.10.002
Lacap-Bugler, D. C., Lee, K. K., Archer, S., Gillman, L. N., Lau, M. C., Leuzinger, S., et al. (2017). Global diversity of desert hypolithic cyanobacteria. Front. Microbiol. 8, 867. doi:10.3389/fmicb.2017.00867
Lakatos, M., and Strieth, D. (2017). Terrestrial microalgae: Novel concepts for biotechnology and applications. Prog. Bot. 79, 269–312.
Lee, J., Park, H. J., Moon, M., Lee, J. S., and Min, K. (2021). Recent progress and challenges in microbial polyhydroxybutyrate (PHB) production from CO2 as a sustainable feedstock: A state-of-the-art review. Bioresour. Technol. 339, 125616. doi:10.1016/j.biortech.2021.125616
Lenz, R. W., and Marchessault, R. H. (2005). Bacterial polyesters: Biosynthesis, biodegradable plastics and biotechnology. Biomacromolecules 6 (1), 1–8. doi:10.1021/bm049700c
Levchenko, I., Xu, S., Mazouffre, S., Keidar, M., and Bazaka, K. (2021). “Mars colonization: Beyond getting there,” in Terraforming Mars. Editors M. Beech, J. Seckbach, and R. Gordon (Wiley). doi:10.1002/9781119761990
Léveillé, R. J., and Datta, S. (2010). Lava tubes and basaltic caves as astrobiological targets on earth and Mars: A review. Planet. Space Sci. 584, 592–598. doi:10.1016/j.pss.2009.06.004
Li, Y., Lin, Y., Loughlin, P. C., and Chen, M. (2014). Optimization and effects of different culture conditions on growth of Halomicronema hongdechloris–a filamentous cyanobacterium containing chlorophyll f. Front. plant Sci. 5, 67. doi:10.3389/fpls.2014.00067
Lingam, M., and Loeb, A. (2020). Photosynthesis on exoplanets and exomoons from reflected light. Int. J. Astrobiol. 19, 210–219. doi:10.1017/s1473550419000247
Liu, D., Liberton, M., Hendry, J. I., Aminian-Dehkordi, J., Maranas, C. D., and Pakrasi, H. B. (2021). Engineering biology approaches for food and nutrient production by cyanobacteria. Curr. Opin. Biotechnol. 67, 1–6. doi:10.1016/j.copbio.2020.09.011
Logan, B. W., Rezak, R., and Ginsburg, R. N. (1964). Classification and environmental significance of algal stromatolites. J. Geol. 72, 68–83. doi:10.1086/626965
Luan, G., Zhang, S., and Lu, X. (2020). Engineering cyanobacteria chassis cells toward more efficient photosynthesis. Curr. Opin. Biotechnol. 62, 1–6. doi:10.1016/j.copbio.2019.07.004
Madison, L. L., and Huisman, G. W. (1999). Metabolic engineering of poly (3-hydroxyalkanoates): From DNA to plastic. Microbiol. Mol. Biol. Rev. 63 (1), 21–53. doi:10.1128/mmbr.63.1.21-53.1999
Mandon, L., Beck, P., Quantin-Nataf, C., Dehouck, E., Thollot, P., Loizeau, D., et al. (2022). Roma: A database of rock reflectance spectra for martian in situ exploration. Earth Space Sci. 91, e2021EA001871. doi:10.1029/2021EA001871
Mareš, J., Hrouzek, P., Kaňa, R., Ventura, S., Strunecký, O., and Komárek, J. (2013). The primitive thylakoid-less cyanobacterium Gloeobacter is a common rock-dwelling organism. PLoS One 8 (6), e66323. doi:10.1371/journal.pone.0066323
Milner, S., Langer, G., Grelaud, M., and Ziveri, P. (2016). Ocean warming modulates the effects of acidification on Emiliania huxleyi calcification and sinking. Limnol. Oceanogr. 61, 1322–1336. doi:10.1002/lno.10292
Miscoe, L. H., Johansen, J. R., Kociolek, J. P., Lowe, R. L., Vaccarino, M. A., Pietrasiak, N., et al. (2016). The diatom flora and cyanobacteria from caves on Kauai, Hawaii. Acta Bot. Hung. 58, 3–4.
Muñoz-Rojas, M., Chilton, A., Liyanage, G. S., Erickson, T. E., Merritt, D. J., Neilan, B. A., et al. (2018). Effects of indigenous soil cyanobacteria on seed germination and seedling growth of arid species used in restoration. Plant Soil 429 (1), 91–100. doi:10.1007/s11104-018-3607-8
Mutale-Joan, C., Sbabou, L., and Hicham, E. A. (2022). Microalgae and cyanobacteria: How exploiting these microbial resources can address the underlying challenges related to food sources and sustainable agriculture: A review. J. Plant Growth Regul. 42, 1–20. doi:10.1007/s00344-021-10534-9
Napoli, A., Micheletti, D., Pindo, M., Larger, S., Cestaro, A., de Vera, J. P., et al. (2022). Absence of increased genomic variants in the cyanobacterium Chroococcidiopsis exposed to Mars-like conditions outside the space station. Sci. Rep. 12 (1), 8437–8439. doi:10.1038/s41598-022-12631-5
Neumann-Micheau, N., and Tributsch, H. (2018). Luminescence light collection technology in the aragonite of stone corals. Bioinspiration Biomimetics 13 (6), 066006. doi:10.1088/1748-3190/aae1bf
Nien, T. S., Bryant, D. A., and Ho, M. Y. (2022). Use of quartz sand columns to study far-red light photoacclimation (FaRLiP) in cyanobacteria. Appl. Environ. Microbiol. 88 (13), e0056222–22. doi:10.1128/aem.00562-22
Nürnberg, D. J., Morton, J., Santabarbara, S., Telfer, A., Joliot, P., Antonaru, L. A., et al. (2018). Photochemistry beyond the red limit in chlorophyll f–containing photosystems. Science 3606394, 1210–1213. doi:10.1126/science.aar8313
Ohtake, M., Matsunaga, T., Haruyama, J., Yokota, Y., Morota, T., Honda, C., et al. (2009). The global distribution of pure anorthosite on the MoonCyanobacteria in hypersaline environments: Biodiversity and physiological properties. NatureBiodiversity Conservation 46124 (72614), 236781–240798. doi:10.1007/s10531-015-0882-z
Olsson-Francis, K., and Cockell, C. S. (2010). Use of cyanobacteria for in-situ resource use in space applications. Planet. Space Sci. 5810, 1279–1285. doi:10.1016/j.pss.2010.05.005
Oren, A. (2015). Cyanobacteria in hypersaline environments: Biodiversity and physiological properties. Biodivers. Conserv. 24, 781–798.
Ozkan, A., Kinney, K., Katz, L., and Berberoglu, H. (2012). Reduction of water and energy requirement of algae cultivation using an algae biofilm photobioreactor. Bioresour. Technol. 114, 542–548. doi:10.1016/j.biortech.2012.03.055
Phillips, A. J., Gerlach, R., Lauchnor, E., Mitchell, A. C., Cunningham, A. B., and Spangler, L. (2013). Engineered applications of ureolytic biomineralization: A review. Biofouling 29 (6), 715–733. doi:10.1080/08927014.2013.796550
Planavsky, N., Reid, R. P., Lyons, T. W., Myshrall, K. L., and Visscher, P. T. (2009). Formation and diagenesis of modern marine calcified cyanobacteria. Geobiology 7, 566–576. doi:10.1111/j.1472-4669.2009.00216.x
Podola, B., Li, T., and Melkonian, M. (2017). Porous substrate bioreactors: A paradigm shift in microalgal biotechnology? Trends Biotechnol. 35 (2), 121–132. doi:10.1016/j.tibtech.2016.06.004
Popović, S., Krizmanić, J., Vidaković, D., Karadžić, V., Milovanović, Ž., Pećić, M., et al. (2020). Biofilms in caves: Easy method for the assessment of dominant phototrophic groups/taxa in situ. Environ. Monit. Assess. 192. Jg., S., 1–17. doi:10.1007/s10661-020-08686-4
Prescott, R. D., Zamkovaya, T., Donachie, S. P., Northup, D. E., Medley, J. J., Monsalve, N., et al. (2022). Islands within islands: Bacterial phylogenetic structure and consortia in Hawaiian lava caves and fumaroles. Front. Microbiol. 13, 934708. doi:10.3389/fmicb.2022.934708
Rai, A. N., Söderbäck, E., and Bergman, B. (2000). Tansley review No. 116. New Phytol. 147 (3), 449–481. doi:10.1046/j.1469-8137.2000.00720.x
Ramalho, T. P., Chopin, G., Pérez-Carrascal, O. M., Tromas, N., and Verseux, C. (2022). Selection of anabaena sp. PCC 7938 as a cyanobacterium model for biological ISRU on Mars. Appl. Environ. Microbiol. 88 (15), e0059422–22. doi:10.1128/aem.00594-22
Rastogi, R. P., Sinha, R. P., Moh, S. H., Lee, T. K., Kottuparambil, S., Kim, Y. J., and Han, T. (2014). Ultraviolet radiation and cyanobacteria. J. Photochem. Photobiol. B Biol. 141, 154–169. doi:10.1016/j.jphotobiol.2014.09.020
Ridgwell, A., and Zeebe, R. E. (2005). The role of the global carbonate cycle in the regulation and evolution of the Earth system. Earth Planet. Sci. Lett. 234, 299–315. doi:10.1016/j.epsl.2005.03.006
Ritchie, R. J., Larkum, A. W., and Ribas, I. (2018). Could photosynthesis function on Proxima Centauri b? Int. J. Astrobiol. 172, 147–176. doi:10.1017/s1473550417000167
Rodeghiero, G., Pernechele, C., Munari, M., Pozzobon, R., Pajola, M., Di Antonio, I., et al. (2022). Radiance values inside lunar caves and lava tubes. Space Telesc. Instrum. 2022 Opt. Infrared, Millim. Wave 12180, 1198–1210. SPIE.
Roldán, M., and Hernández-Mariné, M. (2009). Exploring the secrets of the three-dimensional architecture of phototrophic biofilms in caves. Int. J. Speleology 38 (1), 41–53. doi:10.5038/1827-806x.38.1.5
Rossi, F., and De Philippis, R. (2015). Role of cyanobacterial exopolysaccharides in phototrophic biofilms and in complex microbial mats. Life 5 (2), 1218–1238. doi:10.3390/life5021218
Ryan, J. A., and Sharman, R. D. (1981). Two major dust storms, one Mars year apart: Comparison from Viking data. J. Geophys. Res. Oceans 86 (C4), 3247–3254. doi:10.1029/jc086ic04p03247
Sánchez-Baracaldo, P., and Cardona, T. (2020). On the origin of oxygenic photosynthesis and Cyanobacteria. New Phytol. 225 (4), 1440–1446. doi:10.1111/nph.16249
Sanfilippo, J. E., Garczarek, L., Partensky, F., and Kehoe, D. M. (2019). Chromatic acclimation in cyanobacteria: A diverse and widespread process for optimizing photosynthesis. Annu. Rev. Microbiol. 73, 407–433. doi:10.1146/annurev-micro-020518-115738
Santo, L. (2022). Space sustainability, advanced materials and micro/nanotechnologies for future life in outer Space. Emergent Mater 5, 237–240. doi:10.1007/s42247-022-00373-z
Sauro, F., Pozzobon, R., Massironi, M., De Berardinis, P., Santagata, T., and De Waele, J. (2020). Lava tubes on earth, moon and Mars: A review on their size and morphology revealed by comparative planetology. Earth-Science Rev. 209, 103288. doi:10.1016/j.earscirev.2020.103288
Sauro, F., Pozzobon, R., Santagata, T., Tomasi, I., Tonello, M., Martı́nez-Frı́as, J., et al. (2019). “Volcanic caves of lanzarote: A natural laboratory for understanding volcano-speleogenetic processes and planetary caves,” in Lanzarote and chinijo islands geopark: From earth to space (Springer), 125–142.
Scherer, K., Soerjawinata, W., Schaefer, S., Kockler, I., Ulber, R., Lakatos, M., et al. (2022). Influence of wettability and surface design on the adhesion of terrestrial cyanobacteria to additive manufactured biocarriers. Bioprocess Biosyst. Eng. 45 (5), 931–941. doi:10.1007/s00449-022-02712-0
Shen, G., Canniffe, D. P., Ho, M.-Y., Kurashov, V., Est, A. v. d., Golbeck, J. H., et al. (2019). Characterization of chlorophyll f synthase heterologously produced in Synechococcus sp. PCC 7002. Photosynth. Res. 1401, 77–92. doi:10.1007/s11120-018-00610-9
Sinha, R. P., and Häder, D. P. (2008). UV-protectants in cyanobacteria. Plant Sci. 174 (3), 278–289. doi:10.1016/j.plantsci.2007.12.004
Spribille, T., Resl, P., Stanton, D. E., and Tagirdzhanova, G. (2022). Evolutionary biology of lichen symbioses. New Phytol. 234 (5), 1566–1582. doi:10.1111/nph.18048
Steinbüchel, A., and Lütke-Eversloh, T. (2003). Metabolic engineering and pathway construction for biotechnological production of relevant polyhydroxyalkanoates in microorganisms. Biochem. Eng. J. 16 (2), 81–96. doi:10.1016/s1369-703x(03)00036-6
Talan, A., Pokhrel, S., Tyagi, R. D., and Drogui, P. (2022). Biorefinery strategies for microbial bioplastics production: Sustainable pathway towards Circular Bioeconomy. Bioresour. Technol. Rep. 17, 100875. doi:10.1016/j.biteb.2021.100875
Titus, T. N., Wynne, J. J., Malaska, M. J., Agha-Mohammadi, A.-a., Buhler, P. B., Alexander, E. C., et al. (2021). A roadmap for planetary caves science and exploration. Nat. Astron. 56, 524–525. doi:10.1038/s41550-021-01385-1
Trinugroho, J. P., Bečková, M., Shao, S., Yu, J., Zhao, Z., Murray, J. W., et al. (2020). Chlorophyll f synthesis by a super-rogue photosystem II complex. Nat. Plants 6 (3), 238–244. doi:10.1038/s41477-020-0616-4
Tros, M., Bersanini, L., Shen, G., Ho, M.-Y., Stokkum, I. H. v., Bryant, D. A., et al. (2020). Harvesting far-red light: Functional integration of chlorophyll f into Photosystem I complexes of Synechococcus sp. PCC 7002. Biochimica Biophysica Acta (BBA)-Bioenergetics 18618, 148206. doi:10.1016/j.bbabio.2020.148206
Turco, R., Santagata, G., Corrado, I., Pezzella, C., and Di Serio, M. (2021). In vivo and post-synthesis strategies to enhance the properties of PHB-based materials: A review. Front. Bioeng. Biotechnol. 8, 619266. doi:10.3389/fbioe.2020.619266
Verseux, C., Baqué, M., Lehto, K., Vera, J.-P. P. d., Rothschild, L. J., and Billi, D. (2016). Sustainable life support on Mars–the potential roles of cyanobacteria. Int. J. Astrobiol. 151, 65–92. doi:10.1017/s147355041500021x
Verseux, C., Heinicke, C., Ramalho, T. P., Determann, J., Duckhorn, M., Smagin, M., et al. (2021). A low-pressure, N2/CO2 atmosphere is suitable for Cyanobacterium-based life-support systems on Mars. Front. Microbiol. 67, 611798. doi:10.3389/fmicb.2021.611798
Viola, S., Roseby, W., Santabarbara, S., Nürnberg, D., Assunção, R., Dau, H., et al. (2022). Impact of energy limitations on function and resilience in long-wavelength Photosystem II. Elife 11, e79890. doi:10.7554/elife.79890
Vítek, P., Ascaso, C., Artieda, O., Casero, M. C., and Wierzchos, J. (2020). Raman imaging of microbial colonization in rock — Some analytical aspects. Anal. Bioanal. Chem., 3717–3726. doi:10.1007/s00216-020-02622-8
Viúdez-Moreiras, D., Newman, C. E., De la Torre, M., Martínez, G., Guzewich, S., Lemmon, M., et al. (2019). Effects of the MY34/2018 global dust storm as measured by MSL REMS in Gale Crater. J. Geophys. Res. Planets 124 (7), 1899–1912. doi:10.1029/2019je005985
Walden, B. E., Billings, T. L., York, C. L., Gillett, S. L., and Herbert, M. V. (1998). Utility of lava tubes on other worlds. In Using in situ Resources for Construction of Planetary Outposts (p. 16).
Wang, J., Van Tittelboom, K., De Belie, N., and Verstraete, W. (2012). Use of silica gel or polyurethane immobilized bacteria for self-healing concrete. Constr. Build. Mater 26, 532–540. doi:10.1016/j.conbuildmat.2011.06.054
Westbroek, P., De Jong, E. W., Van der Wal, P., Borman, A. H., De Vrind, J. P. M., Kok, D., and Parker, S. B. (1984). Mechanism of calcification in the marine alga Emiliania huxleyi. Philosophical Trans. R. Soc. Lond. B, Biol. Sci. 304 (1121), 435–444.
Williams, S. F., Martin, D. P., Horowitz, D. M., and Peoples, O. P. (1999). PHA applications: Addressing the price performance issue. Int. J. Biol. Macromol. 25 (1-3), 111–121. doi:10.1016/s0141-8130(99)00022-7
Williams, S. L., Artier, J., Qiu, J., Heveran, C., Nagarajan, A., Hubler, M., et al. (2019): Regenerative hydrogel-based living microbial mortars: Investigation of viability and strength in successive material generations. 238–243. Pages/ Academic Journal of Civil Engineering, Vol 37 No 2 Special Issue - ICBBM 2019. doi:10.26168/ICBBM2019.34
Wolf, B. M., and Blankenship, R. E. (2019). Far-red light acclimation in diverse oxygenic photosynthetic organisms. Photosynth. Res. 1423, 349–359. doi:10.1007/s11120-019-00653-6
Wynn-Williams, D. D. (2000). “Cyanobacteria in deserts—Life at the limit?,” in The ecology of cyanobacteria (Dordrecht: Springer), 341–366.
Wynne, J. J., Titus, T. N., Agha-Mohammadi, A.-a., Azua-Bustos, A., Boston, P. J., León, P., et al. (2021). Fundamental science and engineering questions in planetary cave exploration. J. Geophys. Res. Planets, e2022JE007194. doi:10.1029/2022JE007194
Wyrick, D., Ferrill, D. A., Morris, A. P., Colton, S. L., and Sims, D. W. (2004). Distribution, morphology, and origins of Martian pit crater chains. J. Geophys. Res. Planets 109 (E6), E06005. doi:10.1029/2004je002240
Xu, J., Wang, M., Lin, H., Wang, R., Feng, Q., and Xu, X. (2021). Effect of lunar complex illumination on in situ measurements obtained using visible and near-infrared imaging spectrometer of Chang’E-4. Remote Sens. 1312, 2359. doi:10.3390/rs13122359
Keywords: emerse photobioreactor, polyhydroxybutyrate, biofilm bioreactor, far-red photosynthesis, calcification
Citation: Jung P, Harion F, Wu S, Nürnberg DJ, Bellamoli F, Guillen A, Leira M and Lakatos M (2023) Dark blue-green: Cave-inhabiting cyanobacteria as a model for astrobiology. Front. Astron. Space Sci. 10:1107371. doi: 10.3389/fspas.2023.1107371
Received: 24 November 2022; Accepted: 05 January 2023;
Published: 13 February 2023.
Edited by:
Alberto Fairén, Center for Astrobiology (CSIC), SpainReviewed by:
Lars Behrendt, Uppsala University, SwedenNicoletta La Rocca, University of Padua, Italy
Copyright © 2023 Jung, Harion, Wu, Nürnberg, Bellamoli, Guillen, Leira and Lakatos. This is an open-access article distributed under the terms of the Creative Commons Attribution License (CC BY). The use, distribution or reproduction in other forums is permitted, provided the original author(s) and the copyright owner(s) are credited and that the original publication in this journal is cited, in accordance with accepted academic practice. No use, distribution or reproduction is permitted which does not comply with these terms.
*Correspondence: Patrick Jung, cGF0cmlja19qdW5nOTBAd2ViLmRl