- 1Norfolk Institute, Norfolk, VA, United States
- 2School of Integrative Plant Science, Field of Soil and Crop Sciences, Cornell University, Ithaca, NY, United States
- 3Carl Sagan Institute, Cornell University, Ithaca, NY, United States
It seems to be an accepted assumption that human migration into space is inevitable. However, almost 60 years of scientific studies of the effects of space on Earth life suggest this is not a given. Life on Earth evolved in the context of conditions that are unique to Earth and are not duplicated anywhere else in our solar system. The science indicates that life-sustaining conditions on Earth could be the very things that inhibit our ability to live off-Earth. This paper combines 100 years of scientific development of a theory of ecological thermodynamics with classical mechanics theory and analytical models of self-restoring heat engines to explain how the Sun and Earth have evolved into islands of order in the entropy of space. An explanation is provided regarding how naturally occurring conservative force fields engage a diversity of natural resources in semi-reversible cycles that build a high-exergy ecosphere. The science infers that the ability to establish a human settlement in space without Earth-like self-restoring order, capacity, and organization will result in settlement sustainment challenges. Historical evidence of Earth settlements with disrupted ecosystems point to the following possibilities. Supply chains would disappear, market resources would be depleted, advancement in human pursuits would be disrupted, social and governance systems would falter or collapse, human population numbers would decline, genetic diversity in the human genome would be lost, average human individual biomass would decrease, and human knowledge and understanding would be forgotten. What does it mean to have a location in space outside of Earth be “like Earth?” The results of research are presented as a pancosmorio theory of human sustainability that is developed using the scientific philosophy methodology of abductive reasoning. Four analytical models of space ecosphere sustainability and five hypotheses with proposed tests for falsifiability are provided, including a theorem that suggests a limit to human expansion into space. A new quantitative method of human sustainability is developed from theories of network ecology, providing orthogonal properties of an ecosystem network stability function based upon an ecosystem network production function. Conclusions are made regarding the potential for sustainable development in space using balanced sustainability. Insights are provided regarding human endeavors on the Moon and Mars, as well as the Fermi paradox.
1 Introduction
It seems to be an accepted assumption that human migration into space is inevitable. Is this a valid assumption? Is it based on facts, or is it based upon a belief in human exceptionalism? Humans are migrators. The evidence is clear: humans are spread across Earth. Is there something about humans that would suggest that migrating into space is not much different than migrating out of Africa into Eurasia?
Humans are a species of Earth. We are connected to Earth through evolution. However, we are also connected to space through evolution. We are made of heavy elements generated by fusion in the cores of stars. We are warmed by the radiation of the closest star to us, the Sun. We exist because that star formed into a ball of fusing hydrogen in the local space under the influence of the gravitational force and the nuclear strong force, and because nearby star dust formed into a planet, Earth. Is it unreasonable to assume that human life had as part of its causal chain the formation of a star and a planet? The counterexample of Earth life evolving into existence without an actual Earth and without the power of the Sun suggests the stated assumption is a plausible initial causation.
What does the evolutionary connection of humans to a star and a planet mean for their ability to inhabit space? If human life is existentially dependent upon Earth, then we hypothesize that any human enterprise attempting to establish itself in space outside of Earth without an equivalent system will be unsustainable. We present a new theory and the supporting scientific research that human sustainability in space depends upon conditions that have been evolutionarily established within the Earth ecosphere1 by universal law.
To open the presented theory, the tools of analysis and abductive reasoning will be astrophysics initially, classical physics more broadly, and the burgeoning science of ecological thermodynamics theory (Nielsen et al., 2020). The proof begins with a cosmological review of the evolution of Earth and the Sun into islands of order in the entropy of an expanding space. All life evolved within the context of this order and a collection of evolving conditions. The sustainment of human life is dependent upon the sustainment of this order with its conditions. The level of detail provided in this Introduction section is to assist with understanding how materials acted on by conservative forces are captured in semi-reversible cycles that utilize energy from the Sun to build dissipative structures in the form of self-restoring heat engines. This will be contrasted with human-designed, technological systems that attempt to perform the same functions using non-self-restoring means. The objective is to understand what is required for sustainable human habitation in space. What will be revealed is that human sustainability is about more than just diversity and energy efficiency; it is about self-restoring order, capacity, and organization.
1.1 Self-restoring heat engines provide a basis for sustainable growth
The basis condition of the ecosphere from which all life evolves is the existence of Earth and the Sun. Earth and the Sun form because of the conservative gravitational and nuclear strong forces that are universal laws described by physics theory. They are conservative in that the energy contained by the interaction between properties of matter and the fields of these forces is conserved. No irreversible entropy is generated by the interaction of conservative force fields and matter. The gravitational force results in particles of matter being pulled together through inelastic collisions into collections of greater density to form Earth and the Sun. The nuclear strong force results in atoms of hydrogen being pulled together through inelastic collisions to fuse into helium within the Sun as it collapses under the gravitational force. Calculations of the changes in entropy of the matter that collapses from a cloud of hydrogen to form the Sun and the matter that collapses from a debris field of heavier elements to form Earth reveal that the collapsing matter has a net negative change in entropy.2 As the matter collapses, the heat that is generated and expelled from Earth and the Sun spreads into space and results in a larger net positive change in entropy in the surrounding space than the net negative change in entropy of the matter that forms Earth and the Sun into relative islands of order, thus not violating the second law of thermodynamics (Wallace, 2010; de Souza et al., 2015; Greene, 2021, page 63 and footnote 14).
The generation of heat and the net positive change in entropy correlates with the conservation of momentum theorem that dictates that when inelastic collisions occur, mechanical energy that is typically conserved in the interactions between matter and the conservative gravitational and nuclear strong force fields is lost to heat-generating mechanisms in a proportion that conserves the momentum of matter (see Supplementary Presentation 1: Pancosmorio Theory Classical Mechanics Clarifications for discussion of Conservation of Momentum and Entropy). If the process ends here, the result is the Sun at a high temperature, the Earth at a lower temperature, and surrounding space at an even lower temperature, with heat passing from the Sun to Earth and space and from Earth to space. This simplest form of the movement of energy in the form of heat from a high temperature source to a low temperature sink produces maximum specific entropy3 (Figure 1A).
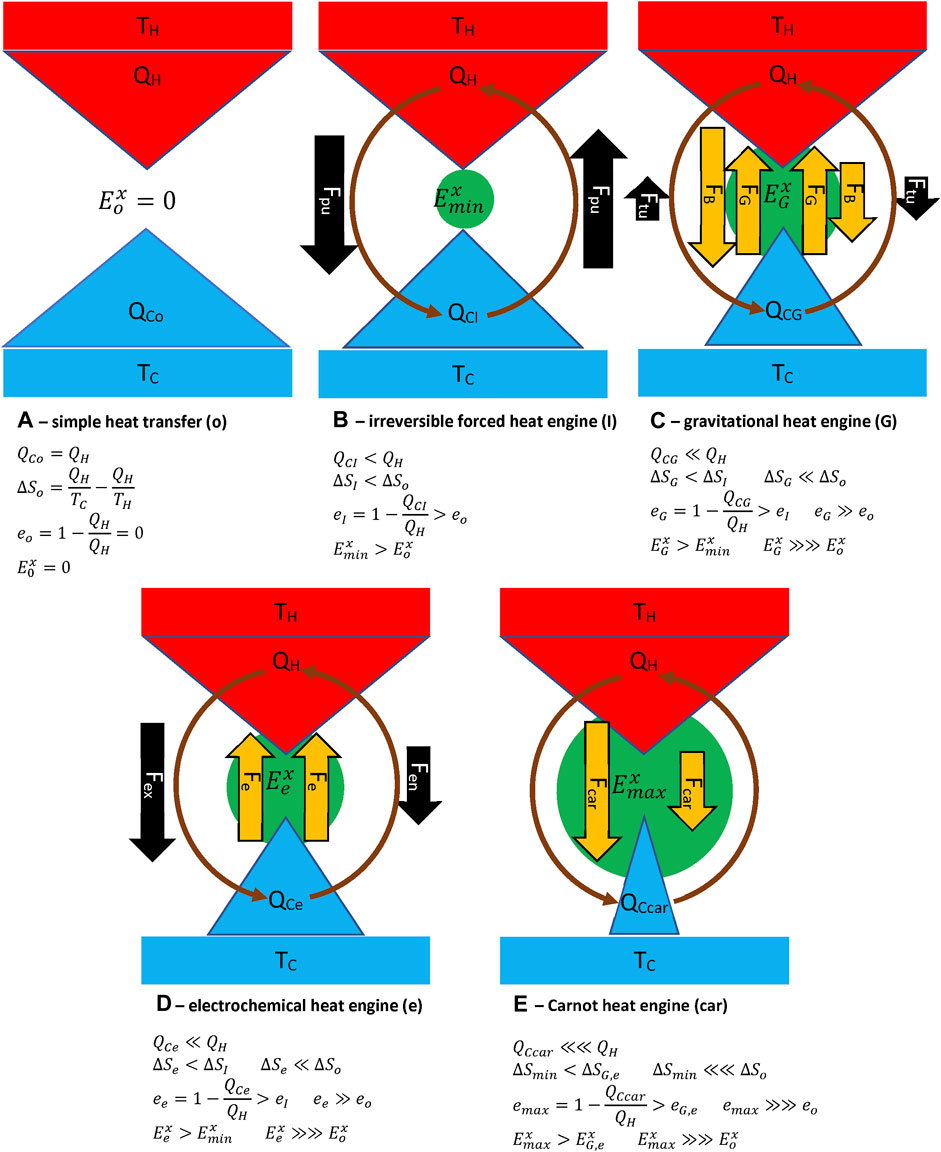
FIGURE 1. Heat engine exergy diagrams indicate useful work in the form of exergy buildup. They are arranged in order ((A) through (E)) of increasing exergy, decreasing heat loss to the heat sink, and decreasing specific entropy and net (universal) entropy generation. They present ecological thermodynamic parameters in terms of a heat reservoir (red rectangle, top, at temperature TH), a heat sink (blue rectangle, bottom, at temperature TC), heat transfer into material out of a heat reservoir (red triangles, with heat input QH), heat transfer out of material into a heat sink (blue triangles, with heat output QC) material displacement captured in heat engine cycles (brown counterclockwise circle arrows), and exergy (green circles). Larger bases of triangles indicate greater heat transfer. Larger circles indicate greater exergy. Gold force arrows inside of material displacement circle are conservative forces and do not generate additional heat; FG is gravity, FB is buoyancy (see Supplementary Presentation 1: Pancosmorio Theory Classical Mechanics Clarifications for discussion of Buoyancy as a Conservative Force), Fe is electrical/electrochemical, and Fcar is the adiabatic and isothermal expansion and contraction of an ideal gas of a theoretical Carnot cycle. Black force arrows outside of material displacement circle are non-conservative forces due to inelastic collisions and increase heat output; Fpu is pumping force, Ftu is air friction/turbulence, Fex is exothermic/debonding explosive force, and Fen is endothermic/bonding collision force. Relative directions of force arrows align with relative directions of material displacement circle, force arrows left of center matching to material displacement toward the heat release portion of the cycle and force arrows right of center matching to material displacement toward the heat absorption part of the cycle. Longer arrows indicate greater force, being additive according to Newton’s Second Law with the sum either supporting or resisting the momentum of material displacement.
However, the process does not end there. The result of the formation of Earth is a gravity well. This gravity well has a spherical rock lithosphere and core at its center with a hydrosphere of water and an atmosphere of gases pulled against it. The force of gravity pulling against the water and gases results in normal forces of the lithosphere pushing back against the hydrosphere and atmosphere; these normal forces fundamentally exist because of the conservative electrical force of repulsion between atoms that exceeds the force of the gravitational field packing the atoms together. It is the pull of gravity and the push of such normal forces that result in the phenomenon of the apparent force known as weight (see Supplementary Presentation 1: Pancosmorio Theory Classical Mechanics Clarifications for discussion of The Apparent Force of Weight).
Weight resulting from the combination of gravity and normal forces produces an important effect. The weight of layers of water and atmosphere piled from the lithosphere to the top of the hydrosphere and atmosphere result in a gradient of depth pressures, with depths closer to the lithosphere having greater pressures. These material spheres, their depth pressures, and the solar insolation falling on the interfaces between the material spheres result in convective forces driven by temperature differences and in the latent heat of evaporation of water from the hydrosphere. Solar power is used to overcome gravity through the gravitationally driven conservative buoyancy force lifting masses of air and evaporated water through the pressure gradient and higher into the atmosphere (see Supplementary Presentation 1: Pancosmorio Theory Classical Mechanics Clarifications for discussion of Buoyancy as a Conservative Force). Gravitational potential energy of the rising air and water vapor increase, such that when the air and water vapor expel heat in the upper atmosphere (and positive specific entropy) into space, the gravitational potential energy generates the power to return each unit of mass to its elevation of origin.
The solar insolation and gravity power sources, the air pressure gradient, and the conservative gravitational and buoyant forces result in a gravitational heat engine (Figure 1C) that drives the rising and falling actions to produce the orderly gravitational dissipative structures of the water cycle and barometric weather cells. Forming the material trapped in the gravity well of Earth into the orderly structures of heat engines results in the material having another net negative specific entropy change. This moves Earth further away from thermodynamic equilibrium. However, the orderly structures are also dissipative structures in that they move heat through the Earth ecosphere and out into space. If the gravitational heat engines only involved an ideal gas atmosphere as a working medium, then the net change in the entropy of the heat captured from solar insolation and then expelled to the space heat sink would be zero, as with a Carnot cycle (Figure 1E). However, considering the atmosphere is not an ideal gas, there are inelastic collisions (i.e., friction) occurring between particles as gravitational potential energy converts to kinetic energy, and kinetic energy is subsequently lost to heat through the conservation of momentum in inelastic collisions. This inefficiency steals some of the heat input from solar insolation away from performing useful work, resulting in the generation of extra heat that is lost to space. It is this extra heat loss that results in a net positive entropy change for the heat transfer of solar heat passing through the material of Earth and out into space that adds up over time and exceeds the net negative change of entropy for the material formed into orderly dissipative structures on Earth. There is a total positive change in entropy for the overall Sun-Earth-space system.
We conceive that the conservative gravitational field acts as an ideal pump (Figure 2A) that neither depletes an external energy source nor generates entropy with its pumping action that uses a conservative force. We postulate that the action of the ideal pump of the gravitational heat engine results in a semi-reversible cycle. In effect, this means that the gravitational heat engine is self-restoring even as the working medium used by the heat engine loses kinetic energy to heat during non-conservative inelastic collisions between the non-ideal-gas particles of the atmosphere.
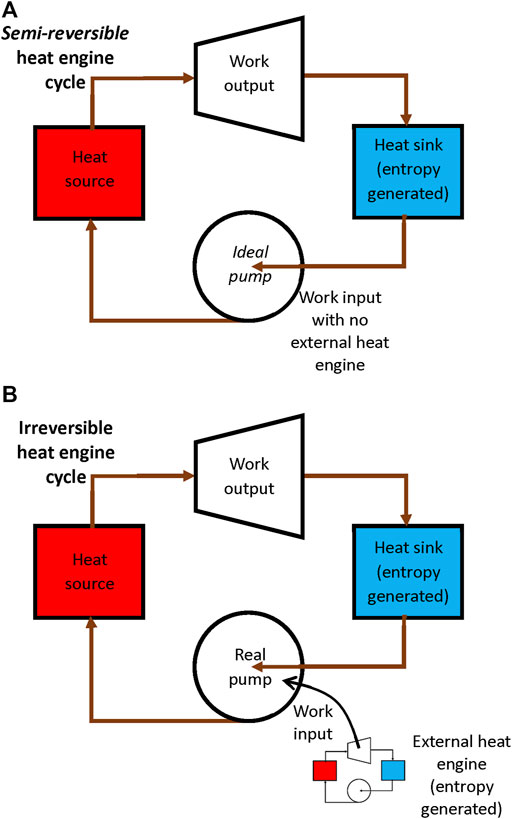
FIGURE 2. Comparison of two heat engine cycles. (A) The semi-reversible heat engine cycle has an ideal pump in the form of a conservative force that requires no external heat engine, and therefore requires no maintenance and never fails. (B) The irreversible heat engine cycle requires an external heat engine to drive the real pump, requires maintenance due to wear and tear, and suffers from ultimate failure.
As time passes, Earth becomes dominated by geophysical and biogeochemical cycles capturing available resources into gravitational and electrochemical dissipative structures that are more orderly and result in a net negative specific entropy change for Earth, moving it further from thermodynamic equilibrium. Electrochemical interactions require proximity of constituents and availability of heat, so the development of electrochemical heat engines proceeds and grows as the right combinations of elements and molecules are brought together by the geophysical cycles involving gravity, water, weather, and heat from solar and geothermal power. We postulate that electrochemical heat engines are pairs of irreversible endothermic and exothermic chemical reactions that sustain each other in a semi-reversible cycle (Figure 1D) with the conservative electrical force acting as an ideal pump. The irreversibility of the chemical reactions is referring to the heat that is required to initiate the reactions and the mechanical energy that is lost to inelastic collisions when atoms are kinetically pulled together (i.e., chemically bonded) or kinetically separated (i.e., bond breaking). Whereas an endothermic chemical reaction combines various atoms and molecules into a larger molecule, an exothermic chemical reaction breaks that larger molecule back down into the original atoms and molecules, thus making that material re-available for the recurrence of the endothermic chemical reaction and the semi-reversible cycle. The electric field is conserved in this cycle, with its full energy continuing to be available to govern the cycle, just as with the gravitational field.
Defining gravitational heat engine cycles and electrochemical heat engine cycles as being semi-reversible is to differentiate them from both a completely reversible ideal Carnot cycle (Figure 1E) and a completely irreversible heat engine cycle in which all applied forces are non-conservative (Figure 1B). The implication of the first and second laws of thermodynamics is that naturally occurring self-restoring heat engines are more efficient than irreversible forced heat engines, resulting in a semi-reversible cycle that generates the entropy of heat transfer at a lower rate than an irreversible cycle relative to equivalent useful work performance. Self-restoring heat engines utilize ideal pumps, described earlier. They do not require an external heat engine to drive them as is required by the pump of an irreversible heat engine cycle (Figure 2B). They also do not require maintenance due to wear, nor do they suffer from ultimate failure. The irreversible heat engine’s pumping force, the pump external power source, and the pump’s wear and failure are more entropic than the semi-reversible cycles of self-restoring heat engines.
The transition away from thermodynamic equilibrium is explained in Table 1 as an exergy-building process of the Universe. As entropy generation rate of the solar heat transfer through the material of Earth and out into space drops with the capture of Earth material into gravitational and electrochemical dissipative structures, the additional energy available for work results in more exergy captured in the building of more dissipative structures. The exergy captured as useful work in upstream dissipative structures is utilized as available work energy to build the heat engines of new downstream dissipative structures. The buildup of lengthening networks of self-restoring heat engines as more Earth materials are captured into cycles results in an increase in the net negative change in specific entropy of Earth as it transitions further away from thermodynamic equilibrium. This forms the basis of sustainable growth of an ecosphere on Earth.
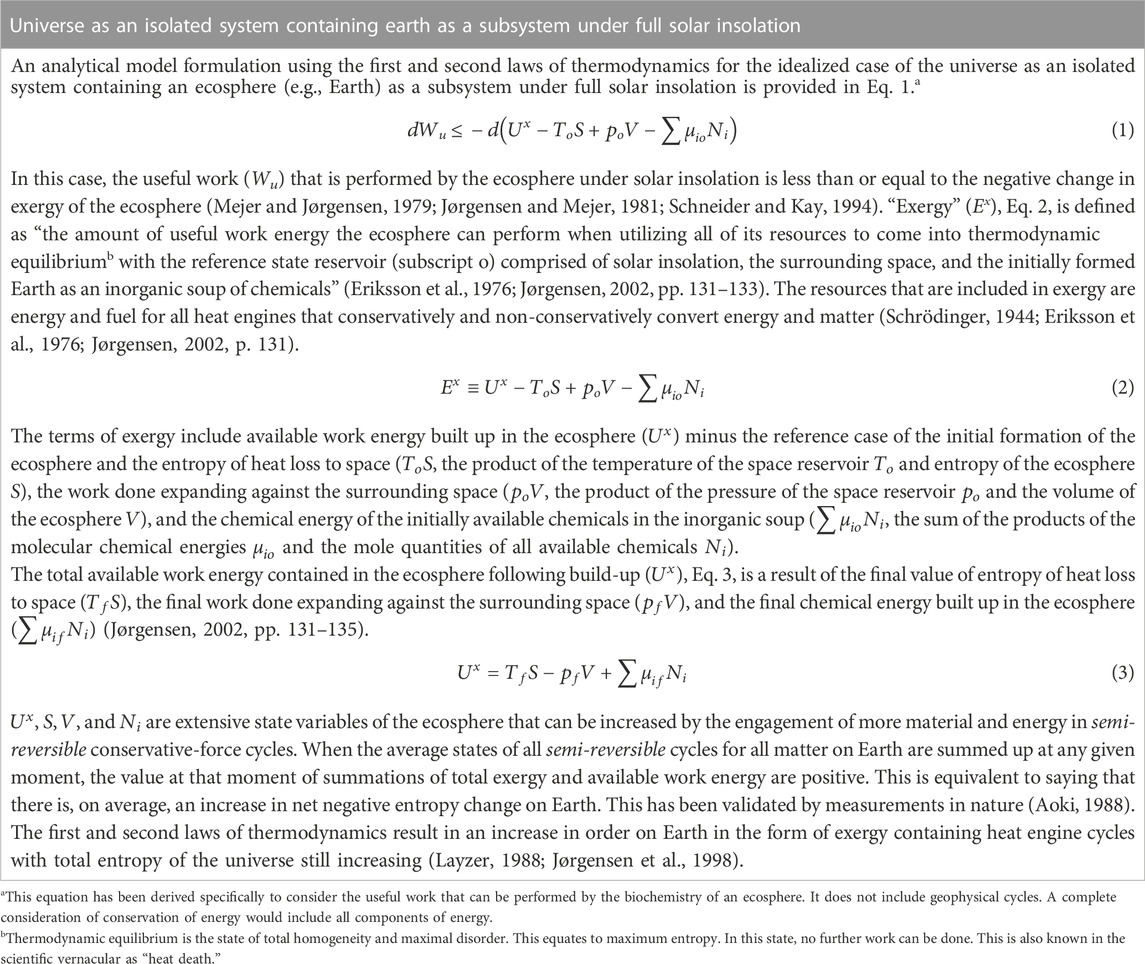
TABLE 1. Universe as an isolated system containing Earth as a subsystem under full solar insolation.
The process of solar insolation power pouring down over a long period of time on all the material present in the gravity well of Earth results in five exergy-building phenomena that engage increasingly more material and energy in adding to available work energy, summarized in Table 2. We postulate the Earth ecosphere builds in a manner that maximizes three traits: self-restoring order characterized by dissipative structures conditions present in the prebiotic Earth, capacity characterized by solar power conditions pouring down on the surface area conditions of Earth, and organization characterized by network vitality conditions and functional diversity conditions of the resulting biotic ecosystem. These phenomena and the empirical data collected for real ecosystems have been proposed to be observational evidence for a central hypothesis of ecological thermodynamics theory. This hypothesis was first formulated at a workshop in Møn, Denmark, August 1993, by J. J. Kay, S. E. Jørgensen, H. F. Mejer, S. N. Nielsen, and E. D. Schneider as a proposal for a fourth law of thermodynamics (Jørgensen, 2002, p. 164). Jørgensen et al. (2016, p. 58) states the latest form of the hypothesis:
“A system that receives a throughflow of work energy will try to utilize the work energy flow to move away from thermodynamic equilibrium (more biomass, more structure, and more information), and if more combinations of components and processes are offered to utilize the work energy flow, the system will select the organization that gives the system as much work energy content (storage) as possible.”
Jørgensen et al. (2016, p. 54) identifies three biological growth forms of ecosystem development under the ecological thermodynamics hypothesis, summarized in Table 3: increasing the amount of available work energy as stored biomass Eq. 4, stored genetic information (Eqs. 5 through 7), and total system throughflow in the growth of the network as whole (Eq. 8). We describe these as three basal growth forms that enable the increase in self-restoring order and capacity of a basal ecosystem through the natural selection of the organization that moves the entire ecosystem further from thermodynamic equilibrium. Basal human populations in the form of hunter-gatherers are a result of and are sustained by this basal ecosystem. The basal ecosystem provides the minimum human life support.
Humans capitalize on these types of growth forms to access basal exergy sources within the ecosphere to augment human society above what is basally produced by the ecosphere under basal dissipative structures conditions, power conditions, network vitality conditions, functional diversity conditions, and area conditions. By so doing, humans have utilized what we describe as three augmentational growth forms, a known and observed human activity, that are corollary to the basal growth forms described by Jørgensen et al.4 This corollary is provided in Table 3. Using the basal ecosystem, humans have built their augmentational ecosystem under augmentational power conditions, network vitality conditions, functional diversity conditions, and area conditions. The self-restoring order, capacity, and organization of the basal ecosystem provides a fundamental basis for sustaining the capacity and organization of the human augmentational ecosystem.
1.2 Consequences for human migration into space
If the current state of human civilization on Earth were to be placed in an ecosphere with lesser basal self-restoring order, capacity, and organization than present on Earth, then humans would have access to less exergy in an ecosphere that is closer than Earth to thermodynamic equilibrium. It would be like expecting your car to run with a cell phone battery. The consequences that can be drawn from the preceding discussion are that human civilization would decline to a level of basal ecosphere self-restoring order, capacity, and organization that is sustainable by the available exergy. The implication in terms of the three augmentational growth forms in Table 3 is that decay of exergy in the human augmentational ecosystem would result. Over time and in an uncontrollable and unpreventable way, supply chains would disappear, market resources would be depleted, advancement in human pursuits would be disrupted, social and governance systems would falter or collapse, human population numbers would decline, genetic diversity in the human genome would be lost, average human individual biomass would decrease, and human knowledge and understanding would be forgotten.
The answer to sustaining the human enterprise as it tries to expand into space has at its heart an understanding that such expansion cannot be in such a way that humans decline in the long term. In the short term, such a reversal of growth would appear as failed settlements. A failed settlement could be an outpost where the humans have lost Earth support, forced to survive on an insufficient production of basal ecosystem exergy. Over time, such an isolated settlement of humans could deteriorate in ways discussed in the previous paragraph. The answer of how to sustain humans in space requires an understanding of the basal ecosystem of Earth and an ability to duplicate the conditions of that basal ecosystem in space. This should be the objective of environmental control and life support (ECLS) systems used in space.
The approach to date that has been used for providing ECLS for humans in space has been completely augmentational. The volume of spacecraft containing humans is minimized to reduce the size of the space that must be controlled. Technology generates the resources that humans need. Such technology is also necessarily limited in size and uses irreversible forced heat engines designed by humans that have high power densities resulting from the concentrated consumption of energy and a concentration of non-conservative forces that are added to drive cycles (e.g., real pumps). Compared to the basal self-restoring heat engines on Earth that provide the basal human life support, the use of technology for life support results in a greater entropy generated per unit of energy consumed to drive the cycles. It also results in the need for maintenance against wear, as well as the eventual failure of the technology due to entropy. Augmentational human technology is ultimately non-self-restoring. Such augmentational means of life support are inherently unsustainable by themselves. A basal ecosystem must be actively producing exergy that an augmentational ecosystem needs to operate. An augmentational ecosystem in space requires the exergy production of Earth—or someplace like Earth.
To better comprehend the difference between augmentational systems and basal systems beyond the theoretical discussion of conservative versus non-conservative forces, entropy, and exergy, consider everything on Earth that does not require human work or input. As an example, breathing is a passive activity for humans. The production of oxygen by the Earth ecosphere is performed without active human involvement. Basal life support on Earth requires no effort by humans for it to be available to humans. Now consider the amount of human effort required to make a technological (i.e., augmentational) system operable and indefinitely available off planet Earth, where humans would not have a basal ecosphere from which to passively draw oxygen. Generating air to breath becomes an active working effort to operate and maintain the augmentational systems required to create the oxygen and scrub the air of the exhaled carbon dioxide. The maintenance of such systems includes a support infrastructure of industry and knowledgeable humans on Earth to continue to provide repair parts and handle issues of malfunction, part and technology obsolescence, and supplier market changes as they occur. The operation also requires power technology and possibly fuel, both of which come with their own support infrastructures. All the oxygen generation technology, power technology, human societal effort, and industry that would be needed to just breath in space would be new in our lives as such. How much extra, non-passive support would be required?
And that is only the oxygen.
The nature of non-self-restoring augmentational systems that utilize irreversible cycles is that they require self-restoring basal systems that utilize semi-reversible cycles to be sustained. This is known as strong sustainability (Pelenc et al., 2015; Maiwald, 2023). The International Space Station would not be able to function without ground support from Earth and the basal ecosystems of Earth to support all of it. Moving further out into space, ground support from Earth becomes more difficult, more costly, riskier. This suggests that the weak sustainability of technofixes (Huesemann and Huesemann, 2011) as has been the to-date approach for deep-space missions is risky for human life. However, solutions that maximize self-restoring elements in a basal ecosystem approach, even if they do not have all that are present on Earth, might reduce the risk of failure.
These considerations suggest what Pirni (2016) calls an “anthropology of limit” to the human endeavor of migration into and habitation of space. Holy-Luczaj and Blok (2019) recognize the need of human life to be conative to the natural environment and the natural environment to be responsive to human life to sustain the integrity of human function on Earth. They raise the question of whether technology, biomimetic technology, or hybrids can be used to improve such conativity and responsiveness. Nielsen (2006) posits that eco-mimetic technology rather than biomimetic technology is needed to achieve sustainable production in human society, but that more studies are needed to understand how to use the basal properties of ecosystems in societal systems. The attempt to move life into space, effectively presenting life with a more extreme environment than on Earth, extends these ontic and ontological questions to space. The evidence suggests that the ability of humans and Earth-life to adapt to space is problematic, with the use of technology to simulate basal Earth life support also having challenges. The trade-off of trying to establish a basal life support system in space is that it would effectively need to be like Earth. The question is whether that is possible? Before we consider the possibility, we need to understand what it means to have a location in space outside of Earth be “like Earth.” The ideas presented in this paper extend the science of ecological thermodynamics theory into space.
1.3 Thesis
The results of research into the beforementioned questions are presented in Section 2 as a pancosmorio theory of human sustainability. The theory is developed based on the science of ecological thermodynamics theory using the methodology of abductive reasoning (Pfister, 2022). A description of the theory (Section 2.1) is provided that has as its factual consequent: i) there are conditions from which human life has evolved, ii) such conditions are required to sustain human life at its current level of growth, and iii) the availability of such conditions to humans defines the limit of their world. This is followed by two propositional antecedents and nine conditionals that can be abductively reasoned from this factual consequent in support of the propositional antecedents. Four analytical models of levels of sustainability in space (Section 2.2) and five testable pancosmorio hypotheses (Section 2.3) are provided. Section 3 contains a discussion of the elements of the theory, referring to the supporting body of research in the physical, ecological, and social sciences. It also contains a new quantitative method of human sustainability that is used in the theory. The methodology builds on and formalizes the proposals in the perspective presented by Irons and Irons (2021), presenting a method for quantifying the human sustainability of any context in which humans are a part, including on Earth or in space. Conclusions are drawn in Section 4 on the application to sustainable development and the potential for the use of a balanced sustainability approach to solve the challenges presented by the theory. Conclusions are also drawn regarding implications for the Fermi paradox.
2 Theory
2.1 Pancosmorio theory of human sustainability in space
The pancosmorio5 theory of human sustainability, hereinafter referred to as the pancosmorio theory, has one factual consequent that comes from ecological thermodynamics theory. It is supported by two propositional antecedents and nine conditionals, described in Sections 2.1.2 and 2.1.3. Discussion of the supporting science for each element of the theory is presented in Section 3.1 through Section 3.4.
2.1.1 Earth ecosphere factual consequent
There are conditions from which human life has evolved. Such conditions are required to sustain human life at its current level of growth. The availability of such conditions to humans defines the limit of their world.
The following propositional antecedents are new to ecological thermodynamics theory in that they expand the science from Earth into the realm of space.
2.1.2 Equivalent basal growth propositional antecedent
The first propositional antecedent is new to ecological thermodynamics theory, is based on the basal growth hypothesis, and infers the factual consequent: basal life activity can be sustained in any space location that has self-restoring order, capacity, and organization equivalent to the basal ecosphere in which such life evolved on its home planet. For humans, the base or settlement in space would need to be supported by ecosystems like what humans evolved with on Earth.
The following conditionals are written in the context of human life to connect the general form of the propositional antecedent to the specific factual consequent of human life on Earth.
2.1.2.1 Basal dissipative structures conditional
The first conditional is new to ecological thermodynamics theory, is based on the basal growth hypothesis, and implies the first propositional antecedent: basal self-restoring order in space requires dissipative structures conditions equivalent to those provided by Earth, Gravitational dissipative structures include geophysical and biogeochemical cycles driven by gravity and gradient air pressure, heating of the lithosphere provided by latent heat of formation, seasons and tidal forces driven by the Sun-Moon-Earth gravi-dynamic system, radiation protection provided by the electromagnetic dynamo of Earth. These gravitational dissipative structures are also necessary for the continuous function of the electrochemical dissipative structures of the biochemical and biophysiological cycles that make up Earth life.
2.1.2.2 Basal power conditional
The second conditional is new to ecological thermodynamics theory, is based on the basal growth hypothesis, and implies the first propositional antecedent: basal capacity in space requires basal power conditions available to match the power in quality and quantity that is basally available to the ecosphere of Earth. This equivalence is necessary to power the growth in total system throughflow exergy. The major contributors to this power are solar insolation at 239 W/m2 and gravity at 95 W/m2, for a total minimum requirement of 334 W/m2. of the area comprising both the basal and augmentational areas of the base or settlement. Production of power by the other gravitational dissipative structures (e.g., the radiation and solar wind shielding provided by the electromagnetic field of Earth) are factors, though the power levels are relatively minimal relative to solar insolation and gravity. Such contributors of power must be considered in the functional services they provide on Earth that are required at the location in space (e.g., the function of providing shielding against radiation).
2.1.2.3 Basal network vitality conditional
The third conditional is existing science in ecological thermodynamics theory, is based on the basal growth hypothesis, and implies the first propositional antecedent: basal organization in space requires basal network vitality conditions of a network that is robust to enable system persistence under nominal loads. Network robustness is characterized by a window of vitality that frames network effective connectivity between the values of one and three and effective number of roles between 2 and 4.5. The network is to provide equivalent production to a basal network on Earth.
2.1.2.4 Basal functional diversity conditional
The fourth conditional is existing science in ecological thermodynamics theory, is based on the basal growth hypothesis, and implies the first propositional antecedent: basal organization in space requires basal functional diversity conditions of abiotic and biotic resources that are sufficiently reliable to enable basal ecosystem resilience under disruptions. Functional diversity is characterized by a normalized ascendency between 36% and 44% and a normalized reserve of 56%–64%. Functional diversity is to provide ecosystem functions and services equivalent to the basal ecosphere on Earth.
2.1.2.5 Basal area conditional
The fifth conditional is new to ecological thermodynamics theory, is based on the basal growth hypothesis, and implies the first propositional antecedent: basal capacity and basal organization in space require basal area conditions of a geographic area sufficiently large to capture and route necessary levels of total system throughflow exergy to support network growth to enable basal network vitality conditional and the basal functional diversity conditional for basal ecosystem persistence and resilience. Based upon the size and population of Earth, the lower limit value is 60,500 m2 per capita of the human population on location in space.
2.1.3 Equivalent augmentational growth propositional antecedent
The second propositional antecedent is new to ecological thermodynamics theory, is based on the augmentational growth corollary, and infers the factual consequent: augmentational life activity can be sustained in any space location that has basal self-restoring order, capacity, and organization equivalent to the home planet, as well as augmentational capacity and organization equivalent to civilization of that life on its home planet. For humans, the base or settlement in space would need to be supported by a basal ecosystem and the same human infrastructure, technology, and society as on Earth, human systems that would need to be sustained by exergy throughflow of in-situ resources from the basal ecosystem, augmentational power systems, and in-situ agricultural production and manufacturing capabilities for all utilized food and technology.
2.1.3.1 Augmentational power conditional
The sixth conditional is new to ecological thermodynamics theory, is based on the augmentational growth corollary, and implies this second propositional antecedent: augmentational capacity in space requires additional augmentational power conditions equivalent to the level of augmentational power consumption utilized by humans on Earth at their current level of growth. This is necessary to power human society and the systems and technology that society runs on. The power requirement is estimated at 1900 W per capita of the human population on location in space.
2.1.3.2 Augmentational network vitality conditional
The seventh conditional is new to ecological thermodynamics theory, is based on the augmentational growth corollary, and implies the second propositional antecedent: augmentational organization in space requires augmentational network vitality conditions (i.e., infrastructure, technology, and society that produce all of the human products, services, knowledge, and information) sufficiently robust to enable system persistence under nominal augmentational loads. The augmentational network is aggradated with the basal network in a way that does not contribute to the effective connectivity and the effective number of roles and quantities of throughflow that do not push the combined network outside the window of vitality. The augmentational network is to provide equivalent production to an augmentational ecosystem on Earth.
2.1.3.3 Augmentational functional diversity conditional
The eighth conditional is new to ecological thermodynamics theory, is based on the augmentational growth corollary, and implies this second propositional antecedent: augmentational organization in space requires augmentational functional diversity conditions of abiotic and biotic resources that are sufficiently reliable to enable augmentational ecosystem resilience under disruptions. The augmentational functional diversity when combined with the basal functional diversity does not push the combined functional diversity outside of and, ideally, keeps it within the standard deviation of normalized ascendency between 36% and 44% and a normalized reserve of 56%–64%. The functional diversity is to contain ecosystem functions equivalent to the contemporary augmentational ecosystem on Earth.
2.1.3.4 Augmentational area conditional
The ninth conditional is new to ecological thermodynamics theory, is based on the augmentational growth corollary, and implies the second propositional antecedent: basal capacity and basal organization in space requires augmentational area conditions of a geographic area sufficiently large to support network growth to enable the augmentational network vitality and functional diversity conditionals for augmentational ecosystem persistence and resilience. The lower limit value for a human society based upon the United States of America is 4,600 m2 per capita of the human population on location in space and includes land area for augmentational habitation and production zones (i.e., for agriculture and industry).
2.2 Analytical models
The conditionals of the theory are interpreted as conditions that an ecosphere separate from Earth must have for a human base or settlement to be sustainable. Any such ecosphere that has these conditions to a limited extent will have limited human sustainability. There are four levels of sustainability that are analytically modeled from the pancosmorio theory. Table 4 summarizes the application of the conditionals to the analytical models. Discussion of the supporting science for each model is presented in Section 3.5 and in Supplementary Presentation 3: Pancosmorio Theory Sustainability Analytical Models.
2.2.1 Level 1 sustainability analytical model
Level 1 sustainability is met by the basal dissipative structures conditional providing self-restoring order, the basal and augmentational power conditionals and area conditionals providing ecosystem capacity, and the basal and augmentational network vitality conditionals and functional diversity conditionals providing ecosystem organization (i.e., within the window of vitality with ascendency and reserve in proper ratios to capacity). This is the level of an advanced human society in space that has solved its human sustainability problems. This is the minimum recommended level for human space bases and settlements that do not maintain a supply chain back to Earth.
2.2.2 Level 2 sustainability analytical model
Level 2 sustainability is met when self-restoring order and capacity are sufficient with dissipative structures, power, and area conditionals being met, but with insufficient organization resulting in a brittle network that is subject to blight and diversity loss under disturbances. Either the basal ecosystem is not sufficiently organized or the augmentational ecosystem excessively loads an organized basal ecosystem, resulting in the combined network vitality conditions and functional diversity conditions not meeting sustainable organization criteria. This is the level that provides humans with a familiar basal and augmentational environmental context that enables innovation and adaptation, with a minimal supply chain to replace depleted resources and specialized technology.
2.2.3 Level 3 sustainability analytical model
Level 3 sustainability is met when self-restoring order is established, but capacity does not sufficiently meet the power and area conditionals to sustain enough of a basal network vitality and functional diversity needed to ensure human adaptability. This results in sufficient capacity to build up enough basal network vitality and functional diversity for human life support, though there is insufficient organization of the combined basal and augmentational ecosystem to mitigate cascade failure following disturbances. This is only recommended as an early stage for a human space base or settlement that is supported by an supplemental supply chain6 while working on transitioning to a higher level of sustainability.
2.2.4 Level 4 sustainability analytical model
Level 4 sustainability is the default when none of the conditionals are met. This results in insufficient self-restoring order, capacity, and organization to be able to support human life without Earth support. The augmentational system provides for ECLS system function and a supporting supply chain from Earth. This is only recommended for locations within Earth orbit that maintain an umbilical supply chain.7 This level is recommended only for staffed missions in near-Earth orbit and unstaffed missions of any lengthy duration beyond Earth orbit.
2.3 Hypotheses
Out of this theory, we provide five testable hypotheses. These hypotheses will be testable by the results of human missions, bases, and settlements as they migrate out into the Solar System and experience unplanned events of loss of productivity, blight, diversity loss, and cascade failure. Alternatively, failure mode tests can be designed and terraformed test platforms built in extreme environments on Earth or in space to test these hypotheses without putting life at risk. The tests for falsifiability of these hypotheses are summarized in Table 5. The tests use the quantitative method of human sustainability. Discussion of the supporting science for each hypothesis and this method are presented in Section 3.6.
2.3.1 The growth hypothesis
Any human base or settlement in space operating at level 1 sustainability can experience basal and augmentational growth that sustains all planned loads robustly without resulting in load-driven disruptions and with a minimum of time to reliably adapt in efficiency to unplanned loads and disruptions. This would appear as no drops in productivity under planned loads and temporary drops in productivity as basal and augmentational networks adapt in response to an unplanned load or disruption, taking time to recover. The result can be an improved ecosphere with improved human sustainability.
2.3.2 The blight hypothesis
Any human base or settlement in space operating at level 2 sustainability or lower can experience basal and augmentational networks faltering under planned loads but without any subsequent disruption. This lack of robustness would appear as ecosystem blight and a limited loss of basal and augmentational production. The result can be a loss of ability to sustain planned loads, requiring corrective and preventative changes to restore production.
2.3.3 The diversity loss hypothesis
Any human base or settlement in space operating at level 2 sustainability or lower can experience disruptions driven by planned loads and lack of full recovery from any disruption. This lack of reliability would appear as a limited loss of functional diversity from basal and augmentational ecosystems. The result can be local extinctions of species and loss of technological capability.
2.3.4 The cascade failure hypothesis
Any human base or settlement in space operating at level 3 sustainability or lower can experience disruptions without recovery. This would appear as a cascading loss of both production and functional diversity of both basal and augmentational ecosystems. A cascading loss would appear as a resource becoming fully depleted with no further production of that resource occurring, and a chain reaction risking total loss of other resources. The result would be a need to establish emergency supply chain support from another location or abandon the location to save life.
2.3.5 The pancosmorio theorem hypothesis
There is a maximum number of astronomical units from the Sun out to which a terraformed system is basally sustainable (assuming dissipative structures, and area conditionals are met) based upon meeting the basal power conditional with solar power panel area in use and the availability of non-solar power. Eq. 9 provides the mathematical form of the pancosmorio theorem. See Supplementary Presentation 2: Pancosmorio Theorem Proof for the development of the proof. The pancosmorio theorem is used to determine the combination of power from solar panels and power from non-solar-power sources that is needed to meet the basal power conditional to enable achieving and maintaining the organization necessary for level 1 sustainability at the distance it is planned to be from the Sun. See Table 6 for definitions of the variables.
3 Discussion
3.1 Theoretical basis
The conditions required to sustain humans are empirically and factually associated with Earth where life evolved and can be determined in contrast to observable space where human life does not exist. Life on Earth is a factual consequence of the existence of these conditions. This is the fundamental basis of ecological thermodynamics theory. The pancosmorio theory expands upon the ecological thermodynamics theory by explaining what this means for human life and other Earth life to exist in any location in space.
The pancosmorio theory is developed using scientific philosophy and the theory of abduction (Pfister, 2022) (Figure 3). Abductive reasoning is necessary to infer a broader explanation of the existence of Earth and its ecosphere. When proven inductively by hypotheses and experiments, such a broader explanation can then be used deductively to apply to any location where humans attempt to establish an ecosphere outside of Earth. Risk to human life in the application of this theory is best mitigated by use of analog test platforms either on Earth or in space that do not involve human life or that have safety measures to protect humans. Such analogs could then be used as inductive experimental proofs of the pancosmorio hypotheses.
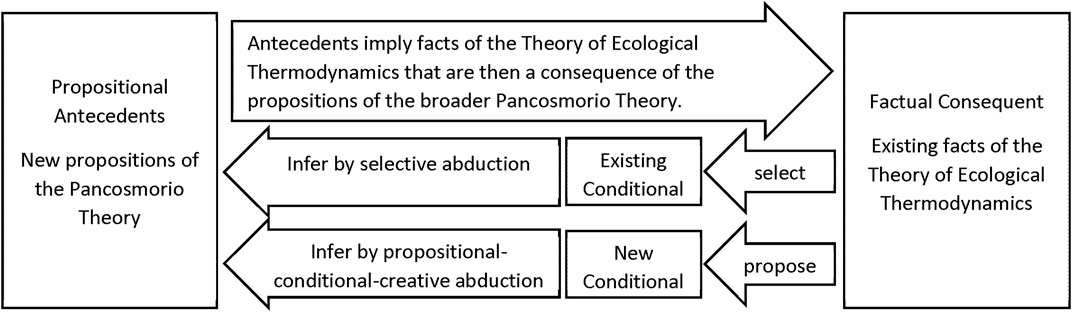
FIGURE 3. Abductive reasoning of the pancosmorio theory. The theory of abduction allows for the expanding of a fact (i.e., the factual consequent) to infer broader propositions (i.e., the propositional antecedents). The propositions, if proven true, establish a more encompassing theory (i.e., antecedence), of which the narrow, understood fact is one implied phenomenon (i.e., consequence). Science must then prove the broader propositional antecedents inductively by hypotheses and experiments to establish a more complete theory. The pancosmorio theory has one factual consequent as existing facts of ecological thermodynamics theory, two propositional antecedents that are new propositions to science as developed in the pancosmorio theory, two conditionals that are known existing conditions of ecological thermodynamics theory and infer one proposition of the pancosmorio theory by selective abduction, and seven conditionals that are newly proposed for ecological thermodynamics theory and infer the propositions of the pancosmorio theory by propositional-conditional-creative abduction. The propositional antecedents imply facts of ecological thermodynamics theory.
3.2 Dissipative structures and power conditionals
Research on Earth’s energy budget (Kiehl and Trenberth, 1997; Loeb et al., 2009; Trenberth et al., 2009) has determined that the average amount of power going into Earth’s atmosphere from solar insolation is 341 W/m2. Of this amount, 102 W/m2 is reflected from clouds, atmosphere, and snow and ice back into space. This means that 239 W/m2 from solar insolation enters the ecosphere network and becomes exergy. Of the 239 W/m2, 175 W/m2 is converted into direct heating of the atmosphere, evaporation and entrainment of water in the atmosphere, convection, and turbulence. Except for the amount that is used for the latent heat of evaporation of water, 80 W/m2, this power is used to overcome gravity, with gravitational potential energy stored in the gravitational field generating the power to return the air mass and water to its elevation of origin. Thus, the rate of gravitational energy input to the ecosphere through self-restoring gravitational heat engines is (175–80) W/m2, or 95 W/m2, equivalent to the amount of power from solar insolation that is required to lift the air and generate the gravitational potential energy. Thus, we arrive at an estimate of energy input rate per unit area of (239 + 95) W/m2, or 334 W/m2.
There are other functional systems of note that generate power and provide vital functions to Earth life. The rotation and the resulting diurnal warming and cooling of the Earth, water, and air result in weather patterns that generally move west to east. An annual revolution of Earth around the Sun, a tilt of the Earth axis relative to its plane of revolution, and the revolution of the Moon around Earth also result in seasons and tidal forces. The lithosphere is heated by latent heat of the original gravitational collapse of Earth as well as ongoing energy input to the lithosphere from the tidal forces. Earth is protected by a magnetosphere from the solar wind that would otherwise generate high energy radiation on Earth and strip away water and gases from the atmosphere and hydrosphere. The magnetosphere is generated by an electromagnetic dynamo of circulating and electrically charged molten liquid matter in the core of Earth also formed out of the original gravitational collapse. Earth rotates on an axis, resulting in a diurnal variation of solar insolation. All of these dissipative structures are a result of the gravitational formation of Earth in orbit of the Sun. They are necessary to sustain life and must be provided for life when outside of Earth.
Gravity is also needed to sustain the biochemical and biophysiological function of humans and other biotic lifeforms. The mostly anecdotal and observational results of life-science research in gravitational orbit around Earth (e.g., see example reviews provided by Kandarpa et al., 2019; Kordyum and Hasenstein, 2021; Bijlani et al., 2021) provide evidence that living things do not function in the same way or at the same level of performance and efficiency in an inertial free-fall reference frame where there is gravity without apparent weight and centripetal force without apparent centrifugal force. Even with a space capsule pressurized to the same level of atmospheric pressure that exists at altitudes where humans live on Earth, the lack of weight and centrifugal force means that there is no fluid pressure gradient within the biotic organism body (see Supplementary Presentation 1: Pancosmorio Theory Classical Mechanics Clarifications for discussion of Forces on the Human Body in the Free-Fall of Space), which is a likely factor in the dysfunction of the human body and other organisms in space.
Our era of the Anthropocene is characterized by a contribution of humans to the total system throughflow of the Earth ecosphere in the burning of fossil fuels that are the stored exergy of past solar insolation being rerouted through the ecosphere. Based on the research of Syvitski et al. (2020), this augmentational power conditional is estimated to be 1900 W per capita of the human population on Earth. This is power that is in addition to the basal power conditional. Compared to the basal power required just to establish and maintain the natural part of the ecosphere, this might seem like a minor amount. However, it adds up quickly as the local population rises.
3.3 Network vitality and functional diversity conditionals
The objective of organization is a network that matches that of an equivalent Earth ecosystem in persistence and resilience to achieve human sustainability. As noted in Section 3.2, one element of persistence and resilience is an ongoing supply of sufficient power. That power must then be utilized by the ecosphere. The intuitive thinking has been that maximized biodiversity and maximized thermodynamic efficiency are requirements for sustainable power utilization. Solid theoretical justification is ambiguous at best for a positive relationship between biodiversity and sustainability (McCann, 2000; Loreau et al., 2001; Loreau and de Mazancourt, 2013). Ulanowicz (1997, Ulanowicz, 2002, Ulanowicz et al., 2008, Ulanowicz, 2009, Ulanowicz et al., 2014, Ulanowicz, 2018, Ulanowicz, 2020) has established by theoretical development and correlative, empirical evidence of Earth ecosystem network data that there is a network variety (
Functional diversity of the ecosystem is characterized by a normalized ascendency (a; a measure of the degree of organization of the network) between the values of 36% and 46% and a normalized reserve (
The normalized ascendency enables resilience that presents as a network robustness and is further characterized by a window of vitality (Ulanowicz, 2009; Ulanowicz, 2020). The window of vitality frames network effective connectivity (c), a measure of the weighted average number of links through which the throughflow is passing, between the values of one and three and the network effective number of roles (r), a measure of the weighted average number of trophic levels through which the throughflow is passing, between the values of 2 and 4.5 (Ulanowicz et al., 2014; Ulanowicz, 2020). The relationship between them is characterized by Eqs 16, 17.
The assumption is that there must be a diversity of abiotic and biotic resources equivalent to a matching Earth ecosystem. Modeling and comparison to real ecosystem networks provides key parameters to consider in building the network. The goal is to achieve a quantitative capacity (
How network vitality and functional diversity are used to build an ecosystem matter. Experiments performed to-date of human-designed ecosystems that are closed to a complete or partial (quasi-closed) extent from the ecosphere of Earth result in local ecosystem dysfunction, even on Earth. Biosphere 2 (Allen et al., 2003; Gitelson et al., 2003, page 52) was an example of attempting to contain a high diversity within a closed system without proper network organization; biomes requiring different environmental conditions were juxtaposed in close proximity due to insufficient area, likely resulting in disturbances of proper throughflows of the biomes' networks. Biosphere 2 also failed to consider the impact of augmentational human systems and technology and their integration into the basal network.
The establishment of a local ecosystem in space likely requires the formulation of an ecosphere network in a managed stepwise fashion. Irons (2018) suggests a boot-strapping effort to establish three zones (i.e., a habitation zone, an agricultural zone, and an ecological buffer zone) using expedited primary succession, competitive redundancy, and ecological service reservoirs. This might be sufficient to get things started but would eventually have to proceed to a proportional fullness of network as exists in the Earth ecosphere. The key shown in Figure 4 is to aggradate the augmentational network with the basal network in a way that does not result in excessive contribution of the augmentational network to the effective connectivity and the effective number of roles. The augmentational network contribution to the combined network must also keep the combined normalized reserve between 56% and 64%.
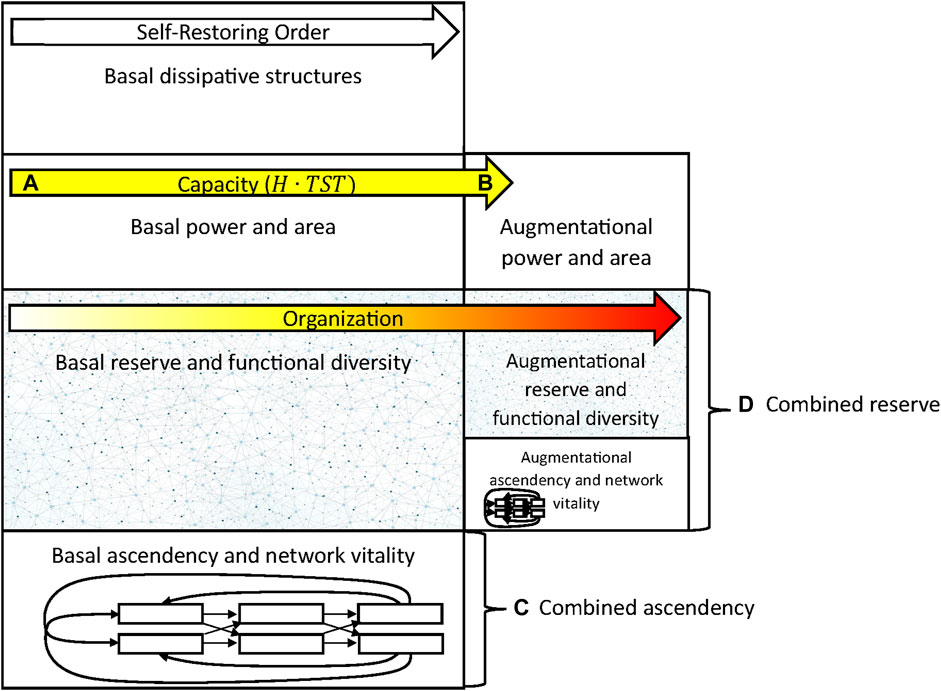
FIGURE 4. Visual of an ecosphere that meets all conditionals. The end objectives are: (A) Basal ecosystem carries most of the total system throughflow (TST). (B) Augmentational capacity is a small percentage of the total system throughflow (TST) coming from the basal ecosystem. (C) The basal ecosystem provides all of the combined ascendency at 36%–44% of combined capacity. The ascendency ensures the combined network has an effective connectivity and an effective number of roles within the window of vitality. Shown is an example effective network with two links per node and three roles. Each link in the augmentational network, including the links that most contribute to augmentational ascendency, must have an amount of throughflow that is relatively minor compared to the throughflow going through the links of the basal network that most contribute to the effective connectivity and the effective number of roles. (D) Combined reserve is made up of a less than 56%–64% of the capacity of the basal network combined with all of the capacity (i.e., reserve and ascendency) of the augmentational network, making up 56%–64% of the combined capacity, such that it does not pull the combined network outside of the window of vitality. The augmentational ascendency must mostly contribute to the combined reserve. Minimizing the impact of the augmentational network is also accomplished by aggradating (i.e., recycling) waste material of the augmentational network back into the basal network.
The effort required to set up the full set of basal and augmentational ecosystems with optimum network vitality and functional diversity will involve a detailed level of environmental management to establish patches and populations of biotic species and communities of biotic interactions within local, regional, and biome-wide ecosystem architectures of abiotic accumulations and flows. This must be done in a way that leverages the established dissipative structures. Once all abiotic and biotic resources are established in appropriate locations and quantities, the sufficient levels of temperature, atmospheric pressure gradient, apparent weight, and physical and biogeochemical cycles provided under the dissipative structures conditional will tend to naturally drive interactions between producers and consumers as evidenced by their function in the Earth ecosphere. These interactions create nodes and links of the network. And as more interactions engage, the network expands across the landscape and builds. Competition with sufficient management should drive the ecosystem into the window of vitality with the proper balance of the capacity between the ascendency and the reserve.
3.4 Area conditionals
The area conditionals are at the crux of the relationship of all the conditionals. The power and area conditionals together determine the ecosphere capacity. The power conditional is in terms of power per unit area, therefore the total area condition of the ecosphere location in space will drive the total power condition. Diamond (1975) notes that when the area of an island or isolated ecosystem is not sufficiently large to support the diversity of biotic species, there will be local species extinctions that occur naturally. The body of work following Diamond’s 1975 paper has confirmed this phenomenon (e.g., Connor and McCoy, 1979; Saunders et al., 1991; Caughley, 1994; Debinski and Holt, 2000; Margules and Pressey, 2000; Sayer et al., 2013). Thus, the area conditional also impacts functional diversity and the ecosphere organization.
The lower limit for the basal area conditional of 60,500 m2 per capita ensures the current basal evolutionary and adaptive state of humans can be supported by the capacity and organization of the ecosphere. It is based upon the surface area (including water) of Earth of 196.9 million mi2 divided by the current population of Earth of 7.837 billion equaling 65,100 m2 per capita, followed by subtracting the augmentational area conditional of 4,600 m2 per capita.
The lower limit for the augmentational area conditional of 4,600 m2 per capita is based upon the population of the United States of 331.9 million and the combined urban and agricultural area of the United States of 63,400 mi2 and 530,400 mi2, respectively (National Land Cover Database, 2016). Agricultural land is included with urban land in the augmentational area conditional considering that human agriculture is augmentational. Human hunting and gathering for food are basal and supported by the basal area conditionals, allowing for human survivability when augmentational systems fail.
The combined area required to support one person is the size of a square 255 m on a side. The per capita land area might seem excessive. It is assumed that the level of human augmentation at any point in the history of Earth is efficiently adapted to the available basal and augmentational surface area on Earth based upon that time’s human adaptation and technological advancement. Human civilization on Earth today utilizes the entire Earth to basally support human augmentational adaptation. A human settlement in space must be basally and augmentationally similar to a developed nation on Earth to be fully sustainable because that is what contemporary humans are adapted to.
3.5 Analytical models
The analytical models for levels of sustainability are based upon the way Earth evolved into an island of order. The steps that Earth proceeded through in its evolution of order are the building blocks of a functioning and sustainable ecosphere. Self-restoring order formed the early lithosphere, hydrosphere, and atmosphere using the gravitational dissipative structures. Power from the Sun poured down on the surface area of Earth to start the buildup of capacity with geophysical cycles and biogeochemical cycles. Ultimately life formed and, through patterns of growth of electrochemical dissipative structures, increased in capacity through the buildup of organization by way of network vitality and functional diversity. See Supplementary Presentation 3: Pancosmorio Theory Sustainability Analytical Models for further explanation of the levels of sustainability.
3.6 Hypotheses and terraform sustainability
The first four pancosmorio hypotheses utilize four parameters: terraform threshold load consistence, terraform threshold disruption resistance, terraform threshold load persistence, and terraform threshold disruption resilience. These are based on an approach to measuring human sustainability derived from stability properties of an ecosystem as first proposed by Irons and Irons (2021). Section 3.6.1 provides a refinement of the original framework into a quantitative method of human sustainability. The first four hypotheses are then explained in Section 3.6.2 in the context of the refined quantitative method of human sustainability.
Section 3.6.3 explains the pancosmorio theorem hypothesis as a limit to the human sustainability of a terraformed ecosphere. The implications are that humans will be limited in how far away from their home Sun they can terraform their environment to an ecosphere of level 1 sustainability. Any attempt to terraform a human base or settlement beyond the distance calculated by the pancosmorio theorem will likely undergo the problems predicted by the cascade failure hypothesis due to not meeting the basal power conditional.
3.6.1 Quantitative method of human sustainability
The pancosmorio theory uses a formalized quantitative method of human sustainability developed from the original terraform sustainability assessment framework proposed by Irons and Irons (2021). Human sustainability is defined by Irons and Irons for a bioregenerative life support system as a form of engineered ecosphere. Human sustainability as refined herein is for application to an entire human base or settlement in space. Table 7 compares the classification of disturbances in the original framework to the new method. The new method utilizes threshold loads and threshold disruptions, an approach to defining disturbances that enables the use of orthogonal measures of stability for quantifying human sustainability. See the Supplementary Presentation 4: Pancosmorio Theory Orthogonal Stability Measures Analytical Model Discussion for the development of the orthogonal stability measures of threshold load stability and threshold disruption stability. Using this refined classification system with the pancosmorio theory allows for testing of the hypotheses of Section 2.3.
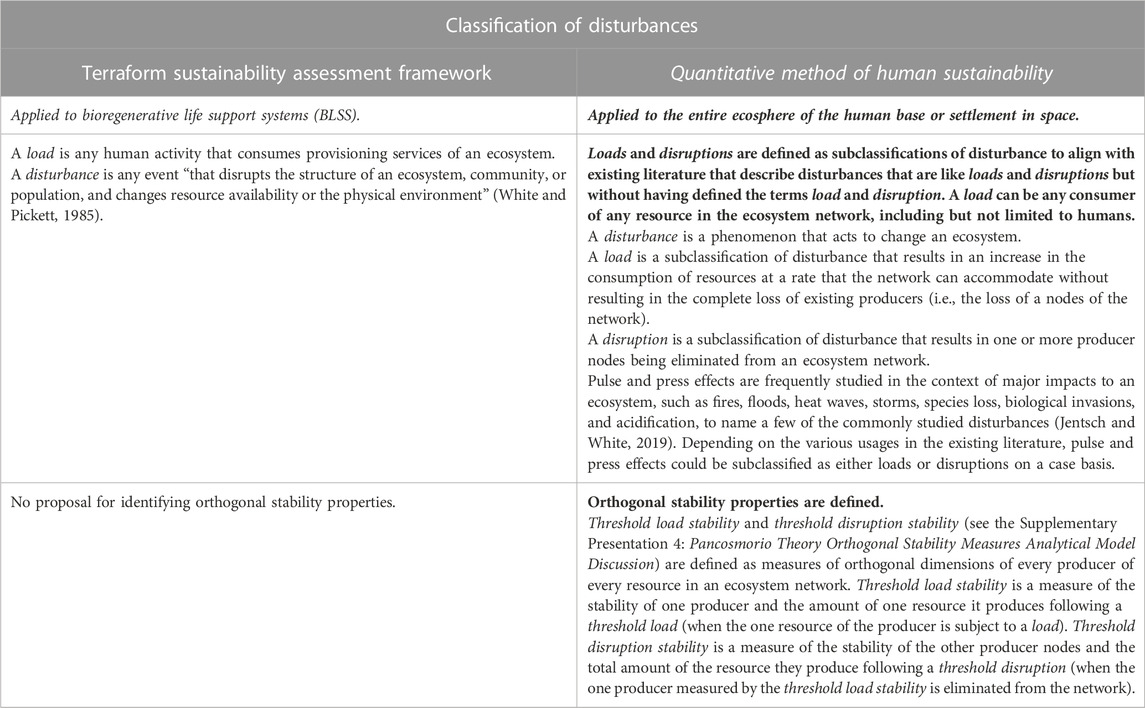
TABLE 7. Classification of disturbances. Comparison of terraform sustainability assessment framework (Irons and Irons, 2021) and quantitative method of human sustainability.
Table 8 compares the original framework and the new method regarding the calculating of stability properties, explaining how the new disturbance classification system provides a means for calculating ecosphere stability. The threshold load stability of Figure P4.4 of Supplementary Presentation 4 is put in terms of threshold load consistence (
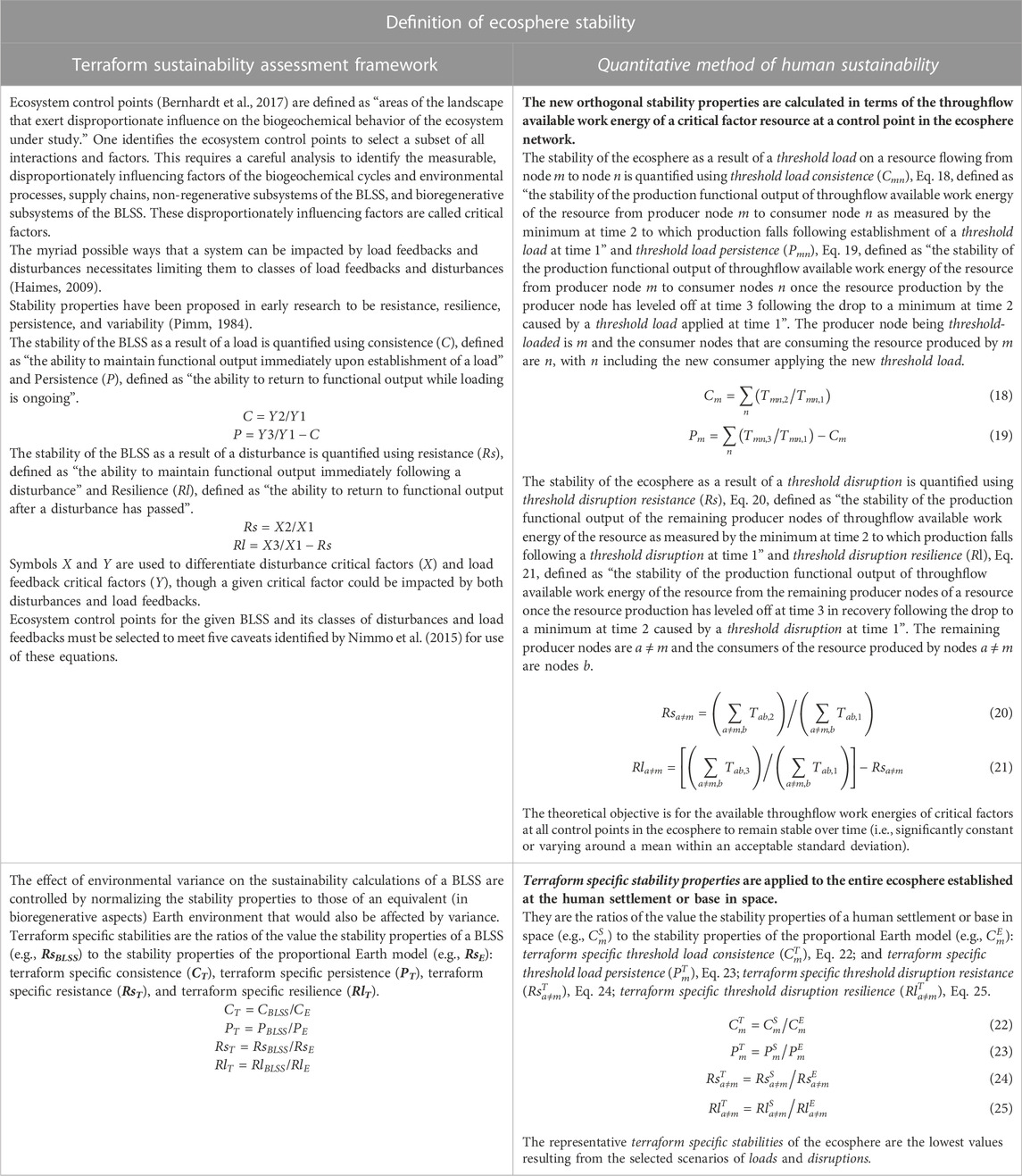
TABLE 8. Definition of ecosphere stability. Comparison of terraform sustainability assessment framework (Irons and Irons, 2021) and quantitative method of human sustainability.
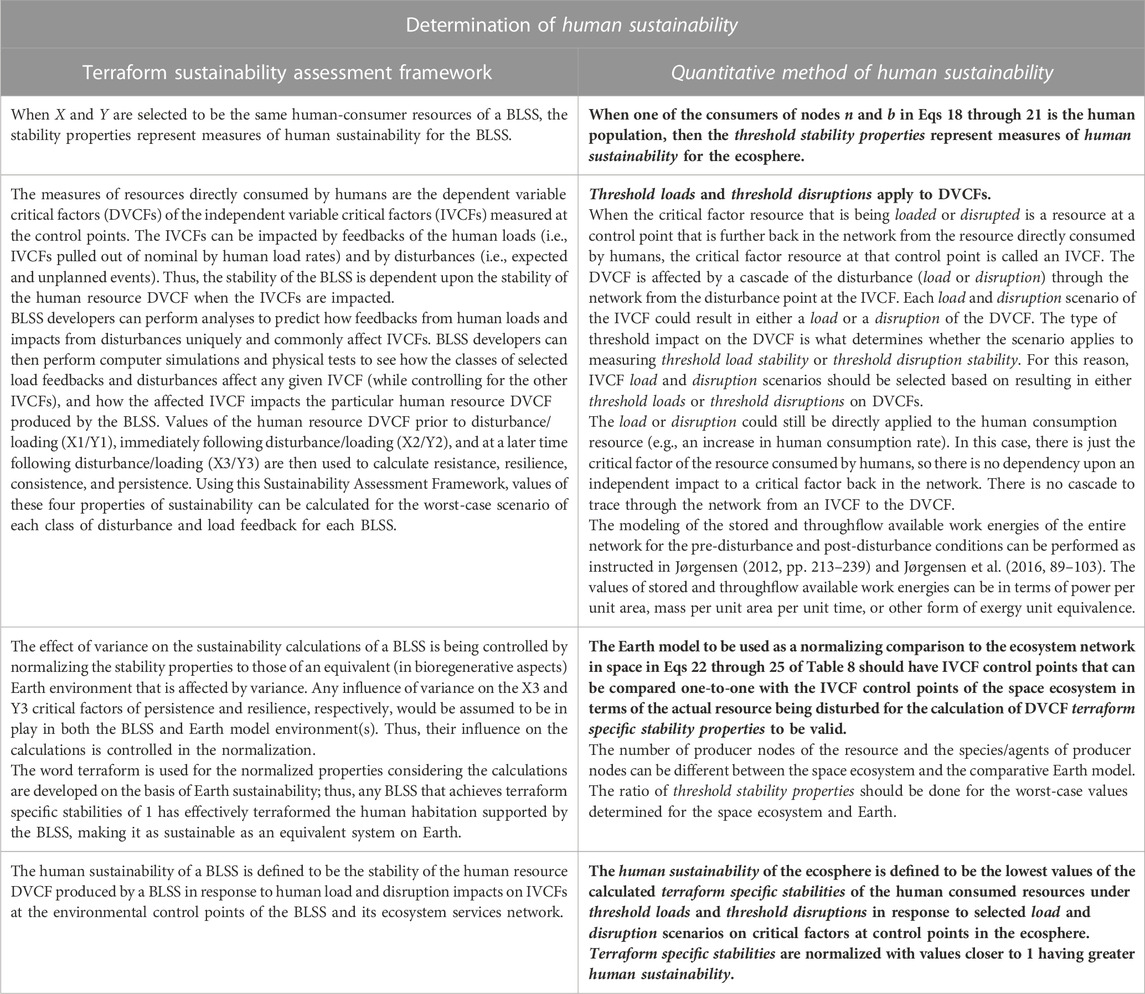
TABLE 9. Determination of human sustainability. Comparison of terraform sustainability assessment framework (Irons and Irons, 2021) and quantitative method of human sustainability.
3.6.2 Growth, blight, diversity loss, and cascade failure hypotheses
The growth hypothesis predicts a state of continued human sustainability of an ecosphere. The basal ecosystem is complete and the augmentational ecosystem is not excessive or wasteful but does support a complete human civilization and all of its technology. This results in terraform specific threshold load persistence and terraform specific threshold disruption resilience equal to one.
The blight hypothesis and the diversity loss hypothesis are both based on level 2 sustainability. For the former hypothesis, blight is a result of a lack of robustness when the network has excessive effective connectivity (i.e., too many links are carrying too much load) and/or excessive effective number of roles (i.e., too many effective number of flows in series pulling too much of a load). The result is a drop in terraform specific threshold load consistence and terraform specific threshold load persistence of throughflows in the network. For the latter hypothesis, diversity loss is a result of a lack of reliability when the network has insufficient normalized reserve (i.e., not enough functional diversity) to fill the gap in ascendency left by the diversity loss while also recovering in reserve. The result is a drop in terraform specific threshold disruption resistance and terraform specific threshold disruption resilience of throughflows in the network. This equates to the current sustainability struggle documented in human cities on Earth (e.g., Morello et al., 2000; Pope et al., 2009; Bergman et al., 2022; Zucaro et al., 2022). If we do things the same way in space as we have done on Earth, the struggle will be similar.
The cascade failure hypothesis is based on level 3 sustainability. This starts out like the diversity loss hypothesis, but results in a cascade of diversity losses due to weakness of the network vitality and functional diversity, resulting in terraform specific threshold disruption resilience dropping to zero. Just as there is evidence in existing populations of humans having successfully migrated all over Earth, there is evidence in the archeological and anthropological record of colony and society ecological collapse. It has even been the topic of popular science writing. Some claim it is human overconsumption of natural resources that drives collapse. Others critique this and say that collapse is frequently driven by other nations invading and enslaving a society and pillaging its lands to the point of its collapse. Still others point to natural cycles and catastrophes on Earth that can result in periodic disruption of human societies. Researchers who might disagree as to the cause of the ecological collapse of societies do agree on at least two points: 1) there is a record of societies collapsing and 2) each collapse correlates with disruptions (de Menocal et al., 2005; Diamond, 2005; Peiser, 2005; Hunt and Lipo, 2006; Diamond, 2011; Flexner, 2011; Butzer, 2012; Gause, 2014).
3.6.3 Pancosmorio theorem hypothesis
The development of Eq. 9 is provided in the Supplementary Presentation 2: Pancosmorio Theorem Proof. Eq. 9 is presented in a form that enables terraform engineers and designers to determine the quantities of solar power panels and non-renewable power generation needed to support the basal portion of the ecosphere based upon the distance from the Sun planned for the base or settlement. The examples of mixed-power settlements in Table 10 have a relative abundance of in-situ materials that can be used as sources of non-solar power (e.g., methane on Titan) to meet the power conditional. In each of these examples, the area of solar power panels used is equal to the total land area used for the human base or settlement. The efficiency of the solar power panel technology being used is 0.33, based upon current maximum efficiency of solar power technology being 0.342 (France et al., 2022). The results provide the level of non-solar power generation (i.e., PNS = NNSP × 334 W/m2) required for powering basal growth at level 1 sustainability. It is important to remember that, to achieve level 1 sustainability, 1900 W per capita of additional solar and non-solar power is also required for powering augmentational growth.
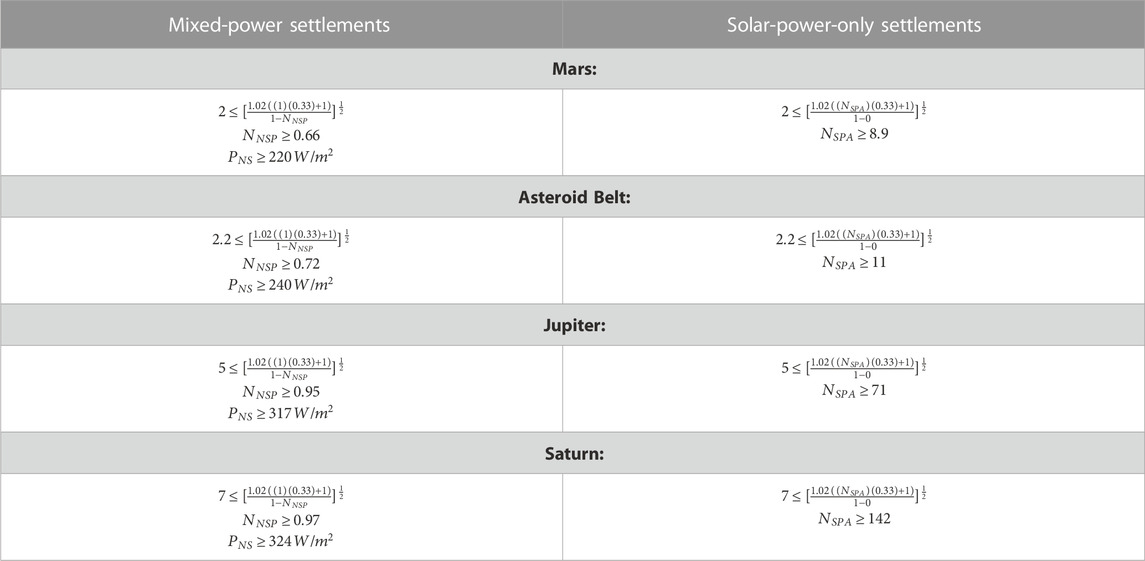
TABLE 10. Comparison of settlement types at four Solar System locations. For mixed-power settlements, NNSP (the ratio of non-solar power availability per unit area of terraformed area to the natural power available to Earth ecosphere per unit area) increases toward the natural power available to Earth ecosphere per unit area (334 W/m2) when there is only as much area of solar power panels as there is land area under human use (NSPA = 1). Non-solar power becomes much more vital as distance from the Sun increases. For solar-power-only settlements, NSPA (the ratio of solar power panel area to land area under human use) increases exponentially with distance from the Sun in cases where there is no non-solar power available for use.
For a human base or settlement that is only powered by solar insolation, NNSP would be zero. The implication is that the area of solar panels in use will need to mathematically square as the distance from the Sun to capture the same amount of power. Table 10 provides examples of the same four locations but for solar-power-only settlements, providing the ratio of solar power panel area to land area under use. These examples reflect that it becomes exponentially more difficult to sustain a human base or settlement with just solar insolation further out from the Sun.
There comes a point of unsustainability where the required area of solar power panels is not feasible. At such a point, non-solar power generation as provided in the first examples would be needed. Non-solar power generation would be limited to geothermal, biofuel, chemical fuel, or nuclear fuel. With the exception of geothermal that requires a geologically active planetoid, the need to refuel would require local in-situ resources or a supply chain. The availability of in-situ materials in space is discrete and limited. Calculations indicate there is insufficient hydrogen available to collect with a scoop while underway to maintain power by nuclear fusion (Mauldin, 1992, Section 4.9) once efficient fusion power technology is available. Human bases and settlements would be limited to locations at planets, dwarf planets, asteroids, and Kuiper Belt objects. Travel and supply chain shipments between such locations would be at great risk.
4 Conclusion
The theory and the scientific development that backs the theory point to the need for a self-restoring basal ecosystem for human sustainability. The theory and the scientific development also point to the characteristics of an augmentational ecosystem that require the basal system to be developed sustainably. Both propositional antecedents and the nine conditionals of the theory provide a basis for a definition of sustainable development in space. A consistent definition of “sustainable development” with a comprehensive set of indicators and analysis tools are currently lacking in the space industry (Maiwald, 2023). Stated simply, sustainable development of a human settlement requires a basal ecosystem to be present on location with self-restoring order, capacity, and organization equivalent to Earth. The sustainable development of the human systems then becomes an augmentational activity that must proceed in a manner that does not produce unrecoverable instability in either the basal or augmentational portions of the total ecosphere. This follows the arguments for strong sustainability (Pelenc et al., 2015; Maiwald, 2023).
Establishing a settlement in a location with pre-existing self-restoring order and capacity equivalent to Earth appears to be impossible. For the dissipative structures conditional required for self-restoring order, 1) there is not a known planetary body in our Solar System that has a 1-g gravitational field equivalent to Earth and 2) humans are unable to artificially generate a conservative gravitational force field to establish self-restoring gravitational heat engines anywhere in space. Keep in mind, the other functions of gravitational dissipative structures needed for a basal ecosystem equivalent to Earth would also need to be naturally present (e.g., radiation protection). There is also research that suggests that even with a 1-g gravitational field, the very specific gravi-dynamics of the Earth-Moon-Sun gravitational system might be required for the biochemical and biophysiological metabolic processes of Earth life to function properly (Thorne, 2021). For the power conditional required for capacity, 1) there is no planetary body on our Solar System that has the diurnal cycle equivalent to one Earth-day and simultaneously 2) has a level of direct solar insolation equivalent to Earth.
These challenges suggest that even level 3 sustainability as modeled by the theory is not achievable. However, the solution might be in using balanced sustainability (Maiwald, 2023), an approach of partially replacing the non-critical functions of natural capital with technology. In an Earth-based context, replacing gravity and the magnetic field of Earth that both provide critical functions with technology would not even be considered. In the space context, the need noted in the previous paragraph is to replace elements of the basal dissipative structures conditional with technology and enhance the basal power conditional with technology, all considered as critical functions. However, certain configurations of ecosphere design in space might allow such a technological approach and still achieve level 1 sustainability. The science behind the theory suggests the key. An entire cross section of the ecosphere at some point in the exergy throughflow must be self-restoring.
Consider first what would be required to replace the Earth functions provided by gravitational dissipative structures with technology. Humans would need to develop augmentational technological systems to emulate Earth gravity and the diurnal solar insolation of Earth. The current methods under consideration in the literature are by using rotation to emulate gravity and solar power collection cells to collect solar power and then direct this power to LED lighting and control systems that emulate Earth-levels of solar insolation in a diurnal cycle. It is possible to emulate the apparent-weight effects of gravity using technology such as an O’Neill cylinder or a McKendree cylinder. What could be more challenging than designing a rotating cylinder that is the size and scale of a biome-sized land area on Earth? Designing technology that would generate the atmospheric pressure gradient as exists on Earth, the phenomenal effect of gravity and the normal force of the lithosphere on the atmosphere.
The spin of a cylinder around an enclosed atmosphere suspended in the free fall of space would not produce this pressure gradient without something to impart an angular velocity to the air suspended within the cylinder. One might imagine a complex system made up of air-columnizing structures that generate the needed angular velocity and a resulting apparent centrifugal force to push the air toward the outer ring of the cylinder as the cylinder rotates, creating an apparent weight effect on the air and resulting in an air pressure gradient. Such an internal structure would result in internal air friction on the spin of the cylinder, which would require energy input to keep the cylinder spinning at the required angular velocity to maintain the apparent centrifugal force equivalent to Earth gravity.
Herein lies the challenge: the technology used to generate the rotation, and therefore apparent weight and the air pressure gradient, is non-self-restoring. It is driven by augmentational technology in the form of irreversible forced heat engines. As noted earlier, high-power-density, forced-cycle heat engines are much less efficient than basal self-restoring heat engines driven by basal gravity and electrochemistry. In addition, the spinning system and its drive engine would be subject to maintenance, wear, and ultimate failure, requiring repair and replacement. Similar considerations apply to the technology to emulate the latent heat of formation of Earth for minimum ground temperature maintenance and tidal, the gravi-dynamic tidal forces of the Sun and the Moon on Earth, and the Earth magnetosphere for shielding the ecosphere from the solar wind. The use of material shielding solutions in space would not necessarily require a power supply. However, if an electromagnetic shielding system is used in space, this power requirement would need to be included.
What remains to be considered for providing the self-restoring anchor are the electrochemical dissipative structures of the biosphere portion of the ecosphere. Theory suggests that the self-restoring cross section of the dissipative structure network might be shifted to the basal ecosystem biological cycles that are driven by self-restoring electrochemical heat engines, Figure 5. The concept is that the gravitational dissipative structures being provided by technology become an extension of the augmentational systems that humans maintain, driven by the self-restoring electrochemical dissipative structures of the biosphere.
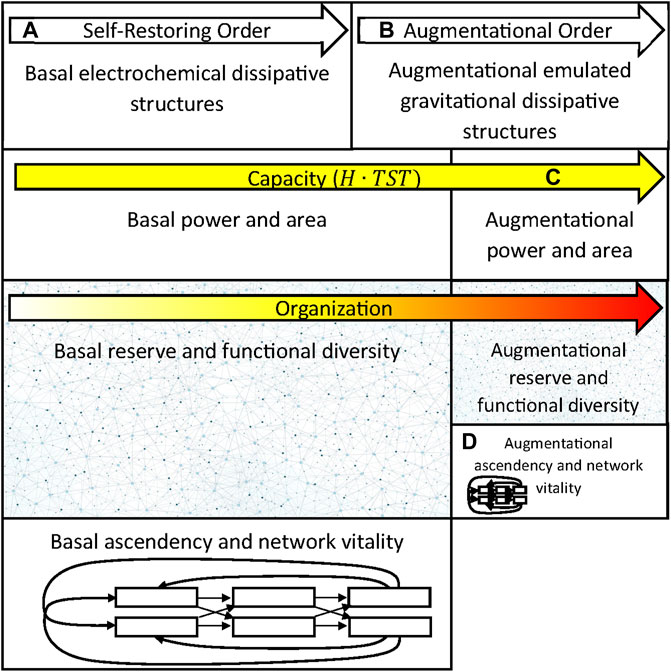
FIGURE 5. A balanced sustainability approach. (A) The self-restoring electrochemical dissipating structures of the basal biosphere are effectively shifted to start the dissipative structure network. (B) The augmentational ecosystem is then used to maintain the non-self-restoring technology needed to emulate the gravitational dissipative structures at the end of the dissipative structure network. Resources generated from the basal ecosystem must be utilized by ISRU and manufacture by ISMF into consumables, replacements parts, and full replacements units as needed to sustain the operation of the augmentational gravitational dissipative structures indefinitely. (C) More augmentational capacity is provided with technology being used to convert the available solar power at location as well as the exergy of biofuel and nuclear fuel into emulated Earth-level diurnal solar insolation and emulated gravitational power. (D) The concern is that if excessive biofuels are used for power generation, this could contribute to augmentational ascendency to an extent that it pulls the combined network outside of the window of vitality, resulting in excessive combined ascendency.
There is also the challenge suggested by the theory of an inability to maintain basal and augmentational functional diversity on location in space due to area limitations, thus limiting such an area-limited space ecosphere to level 3 sustainability. Such a result can be mitigated by utilizing a supplemental supply chain for an ongoing managed migration of new species and material from Earth (and other locations in space) to replace the ones that locally go extinct (depleted). This includes non-renewable energy resources (e.g., methane for chemical power; uranium and transuranic minerals for nuclear fission power; hydrogen, helium, and lithium for nuclear fusion power) needed to supplement the renewable resources when required to meet the basal power conditional and technologically replace functions of Earth gravitational dissipative structures. However, achieving higher levels of sustainability is not possible with such an ongoing supply-chain influx of species and material unless transportation between the settlement, Earth, and other locations in space that can provide resources becomes commoditized and ubiquitous, such that single-point disruptions of transportation do not shut down all migration and commerce. Such a wide-area network of intra-supportive ecospheres with a commoditized space supply-chain market could effectively solve the area problem. Ultimately, the question is whether a location in space can be sufficiently bootstrapped until it reaches the point of being internally self-restoring.
The theory provides interesting insights into ongoing human endeavors in space. The first concerns the plans of the member states of the International Space Exploration Coordination Group (ISECG) for the planned permanent human presence on the Moon (ISECG, 2020; Landgraf, 2021; NASA, 2021). The plans achieve less human sustainability than a space station in LEO. With a greater distance to cover for emergency egress, the escape plans are no better than those for a space station in LEO. The plans are heavily dependent upon a very limited augmentational supply-chain support system with effectively no augmentational reserve elements. Any attempt to use bio-mimetic or eco-mimetic technologies to provide human life support with bioregenerative capability that would be weakly self-restoring outside of Earth gravity only increases the supply-chain support needs due to the increased maintenance requirements of technology that integrates biotic elements. ECLS systems with redundancy somewhat mitigate the risk, but an augmentational reserve as a backup to the main and redundant ECLS systems would provide mitigation against the risk of redundancy failure, which is known to happen with augmentational systems (e.g., Krisher and Koenig, 2023). This is an understandable first step toward developing greater levels of sustainability over time. What is hopeful is that longer-term plans will be developed that consider incorporating more elements toward achieving human sustainability.
The second insight is that establishing a human settlement on Mars would be challenging to achieve any level of sustainability. Level 4 sustainability is ruled out by the distance of Mars from Earth. Level 3 sustainability and above is challenging due to the difficulty with a balanced-sustainability approach of developing a technological system to augmentationally produce the apparent weight and atmospheric pressure gradient needed to generate1-g gravitational dissipative structures. Even if one could develop and build the technology, the functional operation of a settlement on a city-size centrifuge table is difficult to conceive.
The third insight is that abductive, ontic, and ontological reasoning regarding our search for other life in the universe suggests that this theory is applicable to any life evolved anywhere in the universe driven by universal laws. It suggests one of many possible answers to the Fermi paradox. Perhaps we have not yet been visited by alien life due to the challenges any evolved life form would have associated with sustainability of that life far from its home star.
Data availability statement
The original contributions presented in the study are included in the article/Supplementary Material, further inquiries can be directed to the corresponding authors.
Author contributions
All authors listed have made a substantial, direct, and intellectual contribution to the work and approved it for publication.
Funding
MI was supported by a National Science Foundation Graduate Research Fellowship. This material is based upon work supported by the National Science Foundation Graduate Research Fellowship Program under Grant No. DGE-1650441.
Acknowledgments
We thank Cyprien Verseux (Center of Applied Space Technology and Microgravity), Nigel Hywel-Jones (Zhejiang BioAsia Institute of Life Sciences), José Alexandre Matelli (São Paulo State University), and Claude Boullevraye de Passillé (Ordre des architectes du Québec and Ordre des architectes de France) for early review and feedback on the manuscript; Nathan Briggs, Leo Stiles, Alexis Hochheiser, and Mahia Qureshi (The Penn State University Renewable Energy and Sustainability Systems Program) for practical application review and feedback; and the National Science Foundation for providing funding to MI through its Graduate Research Fellowship Program.
Conflict of interest
Author LI is on the Board of Directors of Norfolk Institute LLC which operates as a non-profit entity.
The remaining author declares that the research was conducted in the absence of any commercial or financial relationships that could be construed as a potential conflict of interest.
Publisher’s note
All claims expressed in this article are solely those of the authors and do not necessarily represent those of their affiliated organizations, or those of the publisher, the editors and the reviewers. Any product that may be evaluated in this article, or claim that may be made by its manufacturer, is not guaranteed or endorsed by the publisher.
Author disclaimer
Any opinions, findings, and conclusions or recommendations expressed in this material are those of the authors and do not necessarily reflect the views of the National Science Foundation.
Supplementary material
The Supplementary Material for this article can be found online at: https://www.frontiersin.org/articles/10.3389/fspas.2023.1081340/full#supplementary-material
Footnotes
1This paper uses the term “ecosphere” instead of the commonly used term “biosphere.” “Ecosphere” is defined by the Collins English Dictionary (Ecosphere, 2023) as “the planetary ecosystem, including all the earth’s living organisms and their physical environment.” It relates to the study of ecology as compared to the study of biology. Biology is the study of life, whereas ecology is the study of how life interacts with its physical environment. Use of the term “biosphere” will be limited to the sum of all biotic organisms of an ecosphere.
2The major contributions to the net negative entropy change are that the atoms take up less space and form into new atoms that are fewer in number.
3“Specific entropy” is the amount of entropy generated per unit mass. Speaking in terms of specific entropy allows for comparison of the varying states of an open system that might increase or decrease in quantity of matter over time, the open system in this case being Earth.
4The terms basal and augmentational growth forms are first defined in the present paper and are used in preference to the words “natural” and “artificial” to avoid implying a human externalism, exceptionalism, or supernaturalism by the distinction. Other biotic species can and do have augmentational growth forms when they modify their ecosystem for functional use. However, the questions being addressed are related to human sustainability and, thus, are focused on human activity.
5Pancosmorio is a protologism combining the Greek root words “pan” (neuter form of “pas”) meaning “all”, “kosmos” meaning “world”, and “orio” meaning “limit”.
6Supplemental supply chain support can be defined as a supply chain that provides all augmentational resources for the augmentational ecosystem to provide human life support and to support the building of the basal ecosystem.
7Umbilical supply chain support is defined as a supply chain that incorporates a space location into the Earth basal ecosystem and provides all of the augmentational resources for human life support.
References
Allen, J. P., Nelson, M., and Alling, A. (2003). The legacy of biosphere 2 for the study of biospherics and closed ecological systems. Advances in Space Research 31 (7), 1629–1639. doi:10.1016/S0273-1177(03)00103-0
Aoki, I. (1988). Entropy flows and entropy productions in the Earth’s surface and in the Earth’s atmosphere. J. Phys. Soc. Jpn. 57, 3262–3269. doi:10.1143/JPSJ.57.3262
Autio, W. R., Greene, D. W., Cooley, D. R., and Schupp, J. R. (1991). Improving the growth of newly planted apple trees. HortScience 26 (7), 840–843. doi:10.21273/HORTSCI.26.7.840
Bergman, F., Anderberg, S., Krook, J., and Svensson, N. (2022). A critical review of the sustainability of multi-utility tunnels for colocation of subsurface infrastructure. Front. Sustain. Cities 4, 847819. doi:10.3389/frsc.2022.847819
Bernhardt, E., Blaszczak, J., Ficken, C., Fork, M., Kaiser, K., and Seybold, E. (2017). Control points in ecosystems: Moving beyond the hot spot hot moment concept. Ecosystems 20, 665–682. doi:10.1007/s10021-016-0103-y
Bijlani, S., Stephens, E., Singh, N. K., Venkateswaran, K., and Wang, C. C. C. (2021). Advances in space microbiology. Science 24 (5), 102395. doi:10.1016/j.isci.2021.102395
Boltzmann, L. (1905). The second law of thermodynamics (Populare schriften. Essay no. 3 (Address to Imperial Academy of Science in 1886)). Reprinted in English in Theoretical physics and philosophical problems, selected writings of L. Boltzmann. Dordrecht, Netherlands: D. Riedel.
Butzer, K. W. (2012). Collapse, environment, and society. Proc. Natl. Acad. Sci. 109 (10), 3632–3639. doi:10.1073/pnas.1114845109
Caughley, G. (1994). Directions in conservation biology. J. Animal Ecol. 63 (2), 215–244. doi:10.2307/5542
Christensen, V. (1992). “Network analysis of trophic interactions in aquatic ecosystems,” in Eco targets, goal, and orientors (Berlin, Germany: Springer), 243–254.
Connor, E. F., and McCoy, E. D. (1979). The statistics and biology of the species-area relationship. Am. Nat. 113 (6), 791–833. doi:10.1086/283438
de Menocal, P. B., Cook, E. R., Demeritt, D., Hornborg, A., Kirch, P. V., Mc Elreath, R., et al. (2005). Perspectives on Diamond’s collapse: How societies choose to fail or succeed. Curr. Anthropol. 46 (S5), S91–S99. doi:10.1086/497663
de Souza, R., de Avellar, M., and Horvath, J. (2015). Evolution of stellar entropy. Astron. Nachrichten 336 (8-9), 840–844. doi:10.1002/asna.201512234
Debinski, D. M., and Holt, R. D. (2000). A survey and overview of habitat fragmentation experiments. Conserv. Biol. 14 (2), 342–355. doi:10.1046/j.1523-1739.2000.98081.x
Diamond, J. M. (1975). The island dilemma: Lessons of modern biogeographic studies for the design of natural reserves. Biol. Conserv. 7 (2), 129–146. doi:10.1016/0006-3207(75)90052-X
Engelman, R., Cincotta, R. P., Dye, B., Gardner-Outlaw, T., and Wisnewski, J. (2000). People in the balance: Population and natural resources at the turn of the millenium. Washington D.C.: Population Action International.
Eriksson, B., Eriksson, K. E., and Wall, G. (1976). Basic thermodynamics of energy conversions and energy use. Goteborg, Sweden: Institute of Theoretical Physics.
Flexner, J. L. (2011). Questioning Collapse: Human resilience, ecological vulnerability, and the aftermath of empire. Pac. Aff. 84 (4).
France, R. M., Geisz, J. F., Song, T., Olavarria, W., Young, M., Kibbler, A., et al. (2022). Triple-junction solar cells with 39.5% terrestrial and 34.2% space efficiency enabled by thick quantum well superlattices. Joule 6 (5), 1121–1135. doi:10.1016/j.joule.2022.04.024
Gause, E. (2014). A critique: Jared Diamond’s Collapse put in perspective. Pap. Inst. Archaeol. 24 (1).
Gitelson, I. I., Lisovsky, G. M., and MacElray, R. D. (2003). in Manmade closed ecological systems. Editors P. Kleber, and J. Greenleaf (New York: Taylor and Francis).
Greene, B. (2021). Until the end of time: Mind, matter, and our search for meaning in an evolving universe. New York: Vintage Books.
Haimes, Y. Y. (2009). On the definition of resilience in systems. Risk Anal. 29 (4), 498–501. doi:10.1111/j.1539-6924.2009.01216.x
Hoły-Łuczaj, M., and Blok, V. (2019). How to deal with hybrids in the anthropocene? Towards a philosophy of technology and environmental philosophy 2.0. Environ. Values 28 (3), 325–345. doi:10.3197/096327119X15519764179818
Huesemann, M., and Huesemann, J. (2011). Techno-Fix: Why technology won’t save us or the environment. Gabriola Island, Canada: New Society Publishers.
Hunt, T. L., and Lipo, C. P. (2006). Late colonization of easter island. Science 311 (5767), 1603–1606. doi:10.1126/science.1121879
International Space Exploration Coordination Group (2020). Global exploration roadmap supplement. Washington DC: National Aeronautics and Space Administration.
Irons, M. A. (2018). “Ecological system model for a self-sustaining and resilient human habitation on the Moon and Mars and for food security and climate change mitigation anywhere on Earth,” in U.S. Patent No. 9,970,208 B2 (Washington, D.C: U.S. Patent and Trademark Office).
Irons, M. A., and Irons, L. G. (2021). Terraform sustainability assessment framework for bioregenerative life support systems. Front. Astronomy Space Sci. 8, 789563. doi:10.3389/fspas.2021.789563
Jentsch, A., and White, P. (2019). A theory of pulse dynamics and disturbance in ecology. Ecology 100 (7), e02734. doi:10.1002/ecy.2734
Johnson, R. D., and Holbrow, C. (1975). Space settlements: A design study. Washington D.C.: National Aeronautics and Space Administration.
Jørgensen, S. E. (2007). Ecological network theory. Ecol. Model. 208 (1), 1–2. doi:10.1016/j.ecolmodel.2007.04.018
Jørgensen, S. E. (2002). Integration of ecosystem theories: A pattern. 3rd ed. Dordrecht, Netherlands: Kluwer Academic Publishers.
Jørgensen, S. E. (2012). Introduction to systems ecology. Editor S. E. Jørgensen (Boca Raton: CRC Press).
Jørgensen, S. E., and Mejer, H. F. (1981). “Exergy as key function in ecological models,” in Energy and ecological modelling. Editors W. J. Mitsch, R. W. Bosserman, and J. M. Klopatek (Amsterdam: Elsevier Scientific Pub. Co.), 839.
Jørgensen, S. E., and Nielsen, S. N. (2014). Use of eco-exergy in ecological networks. Ecol. Model. 293, 202–209. doi:10.1016/j.ecolmodel.2014.05.007
Jørgensen, S. E., and Ulanowicz, R. (2009). Network calculations and ascendency based on eco-exergy. Ecol. Model. 220 (16), 1893–1896. doi:10.1016/j.ecolmodel.2009.04.032
Jørgensen, S. E., Marques, J. C., and Nielsen, S. N. (2016). in Integrated environmental management: A transdisciplinary approach. Editor S. E. Jørgensen (Boca Raton: CRC Press).
Jørgensen, S. E., Mejer, H., Nielsen, S. N., and Teuber, J. (1998). The evolution of the thermodynamic equilibrium in the expanding universe. Phys. Scr. 58 (5), 543–544. doi:10.1088/0031-8949/58/5/021
Jørgensen, S. E., Patten, B. C., and Straskraba, M. (2000). Ecosystems emerging:. Ecol. Model. 126 (2-3), 249–284. doi:10.1016/s0304-3800(00)00268-4
Kandarpa, K., Schneider, V., and Ganapathy, K. (2019). Human health during space travel: An overview. Neurol. India 67 (8), 176–181. doi:10.4103/0028-3886.259123
Kay, J. J., and Schneider, E. D. (1994). Embracing complexity: The challenge of the ecosystem approach. Alternatives 20, 32–39.
Kiehl, J. T., and Trenberth, K. E. (1997). Earth’s annual global mean energy budget. Bull. Am. Meteorological Soc. 78 (2), 197–208. doi:10.1175/1520-0477(1997)078<0197:EAGMEB>2.0.CO;2
Kordyum, E., and Hasenstein, K. H. (2021). Plant biology for space exploration – building on the past, preparing for the future. Life Sci. Space Res. 29, 1–7. doi:10.1016/j.lssr.2021.01.003
Krisher, T., and Koenig, D. (2023). Explainer: How NOTAM caused widespread flight disruptions. New York: The Associated Press.
Landgraf, M. (2021). Pathways to sustainability in lunar exploration architectures. J. Spacecr. Rockets 58 (6), 1681–1693. doi:10.2514/1.A35019
Layzer, D. (1988). “Growth of order in the universe,” in Entropy, information and evolution: New perspectives on physical and biological evolution. Editors B. H. Weber, D. J. Depew, and J. D. Smith (Cambridge, Massachusetts, and London: The MIT Press), 23–24.
Loeb, N. G., Wielicki, B. A., Doelling, D. R., Smith, G. L., Keyes, D. F., Kato, S., et al. (2009). Toward optimal closure of the Earth’s top-of-atmosphere radiation budget. J. Clim. 22 (3), 748–766. doi:10.1175/2008JCLI2637.1
Loreau, M., and de Mazancourt, C. (2013). Biodiversity and ecosystem stability: A synthesis of underlying mechanisms. Ecol. Lett. 16, 106–115. doi:10.1111/ele.12073
Loreau, M., Naeem, S., Inchausti, P., Bengtsson, J., Grime, J. P., Hector, A., et al. (2001). Biodiversity and ecosystem functioning: Current knowledge and future challenges. Science 294, 804–808. doi:10.1126/science.1064088
Maiwald, V. (2023). Frameworks of sustainability and sustainable development in a spaceflight context: A systematic review and critical analysis. Acta Astronaut. 204, 455–465. doi:10.1016/j.actaastro.2023.01.023
Margules, C. R., and Pressey, R. L. (2000). Systematic conservation planning. Nature 405 (6783), 243–253. doi:10.1038/35012251
Mauldin, J. H. (1992). “Interstellar ramjets,” in Prospects for interstellar travel. Editors H. Jacobs, and R. H. Jacobs (San Diego, California: Kluwer American Astronautical Society), 110–116.
Mejer, H. F., and Jørgensen, S. E. (1979). Exergy and ecological buffer capacity. Proc. Conf. Ecol. Model. 829, 829–846. doi:10.1016/B978-0-08-023443-4.50042-7
Morello, J., Buzai, G. D., Baxendale, C. A., Rodriguez, A. F., Matteucci, S. D., Godagnone, R. E., et al. (2000). Urbanization and the consumption of fertile land and other ecological changes: The case of buenos aires. Environ. Urbanization 12, 119–131. doi:10.1177/095624780001200210
National Land Cover Database (2016). Editor Multi Resolution Land Characteristics Consortium. Sioux Falls, South Dakota: U. S. Geological Survey.
Nicolis, G., and Prigogine, I. (1971). Fluctuations in nonequilibrium systems. Proc. Natl. Acad. Sci. 68, 2102–2107. doi:10.1073/pnas.68.9.2102
Nielsen, S. N. (2019). Reductions in ecology and thermodynamics. On the problems arising when shifting the concept of exergy to other hierarchical levels and domains. Ecol. Indic. 100, 118–134. doi:10.1016/j.ecolind.2018.04.062
Nielsen, S. N. (2006). What has modern ecosystem theory to offer to cleaner production, industrial ecology and society? The views of an ecologist. J. Clean. Prod. 15 (17), 1639–1653. doi:10.1016/j.jclepro.2006.08.008
Nielsen, S. N., and Ulanowicz, R. E. (2000). On the consistency between thermodynamical and network approaches to ecosystems. Ecol. Model. 132, 23–31. doi:10.1016/S0304-3800(00)00302-1
Nielsen, S. N., Müller, F., Marques, J., Bastianoni, S., and Jørgensen, S. E. (2020). Thermodynamics in ecology – an introductory review. Entropy 22, 820. doi:10.3390/e22080820
Nimmo, D. G., Mac Nally, R., Cunningham, S. C., Haslem, A., and Bennett, A. F. (2015). Vive la Resistance: Reviving resistance for 21st century conservation. Trends Ecol. Evol. 30, 516–523. doi:10.1016/j.tree.2015.07.008
Nowak, D. J., Hoehn, R., and Crane, D. E. (2007). Oxygen production by urban trees in the United States. Arboric. Urban For. 33 (3), 220–226. doi:10.48044/jauf.2007.026
Odum, E. P. (1969). The strategy of ecosystem development. Science 164 (3877), 262–270. doi:10.1126/science.164.3877.262
Patten, B. C. (1991). “Network ecology: Indirect determination of the life–environment relationship in ecosystems,” in Theoretical ecosystem ecology: The network perspective. Editors M. Higashi, and T. P. Burns (London, Cambridge: University Press), 288–351.
Peiser, B. (2005). From genocide to ecocide: The rape of Rapa Nui. Energy and Environ. 16 (3), 513–539. doi:10.1260/0958305054672385
Pelenc, J., Ballet, J., and Dedeurwaerdere, T. (2015). Brief for GSDR 2015 – weak sustainability versus strong sustainability. New York: United Nations Department of Economic and Social Affairs. Available at: https://sdgs.un.org/documents/brief-gsdr-2015-weak-sustainability-versus-s-20782.
Pfister, R. (2022). Towards a theory of abduction based on conditionals, 200. Synthese. doi:10.1007/s11229-022-03581-6
Pimm, S. L. (1984). The complexity and stability of ecosystems. Nature 307, 321–326. doi:10.1038/307321a0
Pirni, A. (2016). Space and anthropology of limit: A philosophical perspective. Front. Astronomy Space Sci. 3, 22. doi:10.3389/fspas.2016.00022
Pope, C. A., Ezzati, M., and Dockery, D. W. (2009). Fine-particulate air pollution and life expectancy in the United States. N. Engl. J. Med. 360, 376–386. doi:10.1056/NEJMsa0805646
Prigogine, I., and Wiame, J. M. (1946). Biologie et thermodynamique des phénomènes irréversibles. Experientia 2, 451–453. doi:10.1007/BF02153597
Salomonsen, J. (1992). Examination of properties of exergy, power and ascendency along a eutrophication gradient. Ecol. Model. 62, 171–181. doi:10.1016/0304-3800(92)90089-W
Saunders, D. A., Hobbs, R. J., and Margules, C. R. (1991). Biological consequences of ecosystem fragmentation: A review. Conserv. Biol. 5 (1), 18–32. doi:10.1111/j.1523-1739.1991.tb00384.x
Sayer, J., Sunderland, T., Ghazoul, J., Pfund, J. L., Sheil, D., Meijaard, E., et al. (2013). Ten principles for a landscape approach to reconciling agriculture, conservation, and other competing land uses. Proc. Natl. Acad. Sci. 110 (21), 8349–8356. doi:10.1073/pnas.1210595110
Schneider, E. D., and Kay, J. J. (1994). Life as a manifestation of the second law of thermodynamics. Math. Comput. Model. 19, 25–48. doi:10.1016/0895-7177(94)90188-0
Syvitski, J., Waters, C.N., Day, J., Milliman, J. D., Summerhayes, C., Steffen, W., et al. (2020). Extraordinary human energy consumption and resultant geological impacts beginning around 1950 CE initiated the proposed Anthropocene Epoch. Commun. Earth Environ. 1 (32), doi:10.1038/s43247-020-00029-y
Thorne, S. (2021). Modeling the role of gravitation in metabolic processes. Commun. Integr. Biol. 14 (1), 115–135. doi:10.1080/19420889.2021.1914913
Trenberth, K. E., Fasullo, J. T., and Kiehl, J. (2009). Earth’s global energy budget. Bull. Am. Meteorological Soc. 90 (3), 311–324. doi:10.1175/2008BAMS2634.1
Ulanowicz, R. E. (2018). Biodiversity, functional redundancy and system stability: Subtle connections. J. R. Soc. Interface 15, 20180367. doi:10.1098/rsif.2018.0367
Ulanowicz, R. E. (1997). Ecology: The ascendent perspective. Editors T. F. H. Allen, and D. W. Roberts (New York: Columbia University Press).
Ulanowicz, R. E. (1986). Growth and development—ecosystems phenomenology. Berlin/Heidelberg: Springer.
Ulanowicz, R. E. (2020). Quantifying sustainable balance in ecosystem configurations. Curr. Res. Environ. Sustain. 1, 1–6. doi:10.1016/j.crsust.2019.09.001
Ulanowicz, R. E. (2002). The balance between adaptability and adaptation. Biosystems 64, 13–22. doi:10.1016/S0303-2647(01)00170-8
Ulanowicz, R. E. (2009). The dual nature of ecosystem dynamics. Ecol. Model. 220 (16), 1886–1892. doi:10.1016/j.ecolmodel.2009.04.015
Ulanowicz, R. E., Goerner, S. J., Lietaer, B., and Gomez, R. (2008). Quantifying sustainability: Resilience, efficiency and the return of information theory. Ecol. Complex. 6, 27–36. doi:10.1016/j.ecocom.2008.10.005
Ulanowicz, R. E., Holt, R. D., and Barfield, M. (2014). Limits on ecosystem trophic complexity: Insights from ecological network analysis. Ecol. Lett. 17, 127–136. doi:10.1111/ele.12216
Wallace, D. (2010). Gravity, entropy, and cosmology: In search of clarity. Br. J. Philosophy Sci. 61 (3), 513–540. doi:10.1093/bjps/axp048
White, P. S., and Pickett, S. T. A. (1985). “Natural disturbance and patch dynamics: An introduction,” in The ecology of natural disturbance and patch dynamics. Editors S. T. A. Pickett, and P. S. White (New York, NY: Academic Press), 3–13. doi:10.1016/b978-0-08-050495-7.50006-5
Keywords: sustainability, resilience, terraform, sustainable development, ecology, thermodynamics, space habitation, Fermi paradox
Citation: Irons LG and Irons MA (2023) Pancosmorio (world limit) theory of the sustainability of human migration and settlement in space. Front. Astron. Space Sci. 10:1081340. doi: 10.3389/fspas.2023.1081340
Received: 27 October 2022; Accepted: 07 February 2023;
Published: 06 March 2023.
Edited by:
Andreas Losch, University of Bern, SwitzerlandReviewed by:
Søren Nors Nielsen, Aalborg University, DenmarkErich Schienke, The Pennsylvania State University (PSU), United States
Copyright © 2023 Irons and Irons. This is an open-access article distributed under the terms of the Creative Commons Attribution License (CC BY). The use, distribution or reproduction in other forums is permitted, provided the original author(s) and the copyright owner(s) are credited and that the original publication in this journal is cited, in accordance with accepted academic practice. No use, distribution or reproduction is permitted which does not comply with these terms.
*Correspondence: Lee G. Irons, bGVlQG5vcmZvbGtpbnN0aXR1dGUub3Jn; Morgan A. Irons, bWFpNTdAY29ybmVsbC5lZHU=