- Birbal Sahni Institute of Palaeosciences, Lucknow, India
This review paper summarizes the literature on the organic matter detection by various Mars lander/rover missions, in order to understand the progress towards dealing with methodological challenges in the analysis of the Martian regolith and drilled mudstone samples. This paper shows that Martian missions are so far successful in detecting simple and some complex organic molecules, but their origin i.e., whether sourced by cosmic dust, meteoric bombardment, geochemical reduction of inorganic carbon during hydrothermal or igneous activity, or produced biologically in the ancient habitable Martian deposition environment, remains unknown. The preservation of organic matter in the Martian depositional realm has also been found as one of the biggest hurdles in its search. Therefore, upcoming ExoMars mission has been equipped with the instruments that would be able to drill and retrieve 2 m subsurface cores for organic matter analysis, with the assumption that the subsurface samples would have better chances of preserving original organic matter from the disintegration by ultraviolet (UV) radiation, galactic cosmic rays, and solar energetic particles. In addition to the method used for organic matter detection in previous missions [simple pyrolysis-GCMS and the use of combination of thermal combustion and derivatization (thermochemolysis)-GCMS], other alternative organic matter detection methodologies i.e., Raman spectroscopy (laser 523) plus deep resonant Raman and fluorescence spectroscopy are used in Mars 2020 Perseverance rover and will be used in ExoMars mission as well. Learning from the past and upcoming Mars missions will help in developing strategies and tools for the future Martian missions with goal to better understand it is ancient habitability.
Introduction
There is compelling evidence that during the first half-billion years (4 to 3.5 Ga), Mars was wetter i.e., its surface and shallow subsurface hosted liquid water (Nisbet and Sleep, 2001; Baker, 2006; Greenwood et al., 2008). As a result, Mars has become the most promising target for astrobiological studies. However, the major question is how long this water must persist for life to evolve. Based on the Earth’s geological record the estimated upper limit for the origins of life is considered 500 million years (Davis and McKay, 1996). Using a simple climate model, Davis and McKay (1996) determined that liquid water habitats on Mars could have survived for up to 500 million years under relatively thin ice covers with an atmospheric temperature below freezing point. Thus, the timeframe for liquid water on early Mars appears to be equivalent to the maximum timeframe for the emergence of life on Earth (Davis and McKay, 1996). In the last 20 years, the scientific search for life on Mars has been taken on two major aspects: 1) exploring the Martial geological records for probable evidences of extinct life, 2) looking for signs of extant microbial life on Mars surface and subsurface environments. Progress has been made in both the directions. However, the search for extinct life on Mars has received more attention (Carrier et al., 2020) mainly due to the fact that early Martian condition was relatively more hospitable for life (Fairén et al., 2010).
There is a general consensus that life, if it exists elsewhere, is based on carbon chemistry, needs nitrogen, highly information-rich organic compounds, energy, substrates that allow for the synthesis of the organic compounds, and liquid water (Marais and Walter, 1999; Schulze-Makuch et al., 2002; Stern and Jedrzejas, 2008). Therefore, understanding how organic substances are synthesized abiogenically throughout the universe also piques our intrinsic curiosity (Oparin, 1965; Lemmon, 1970; Becker et al., 1999; Sephton and Hazen, 2013). Organic molecule signatures of early life on Earth are largely destroyed by tectonic recycling. Such tectonic recycling of early biotic or pre-biotic organic signatures on Mars seems unlikely as no evidence of past tectonic activity has been recorded on this planet (Mahaffy, 2008). However, the presence of superoxide radicals (Yen et al., 1999; Yen et al., 2000), oxidized halides (Zent and McKay, 1994; Hecht et al., 2009), and radiation (Kminek and Bada, 2006) on the Martian surface may have transformed organic molecules, reducing the diversity of such compounds from earlier periods and limiting our ability to describe their origin. It is nonetheless important to note that the type of organic material found on Mars has a direct bearing on two most important scientific questions: 1) how life arose on Earth and, 2) how life in the universe may have originated. For this reason, several missions have been sent or are under preparation to Mars for the detection of organic compounds in surface/subsurface sediments and the atmosphere.
Several Martian meteorites and Martian surface sediments have been found to contain organic carbon, but their origin remains unclear (Biemann et al., 1977; Mazur et al., 1978; Steele et al., 2012; Glavin et al., 2013; Freissinet et al., 2015; Eigenbrode et al., 2018; Franz et al., 2020; Millan et al., 2022a; Millan et al., 2022b; Stern et al., 2022). There have been a number of sources proposed for the detected organic compounds: 1) terrestrial contamination (Biemann et al., 1977; Mazur et al., 1978; Jull et al., 1998); 2) chondritic meteoric inputs (Becker et al., 1999); 3) direct precipitation from aqueous fluids (Steele et al., 2007; Ming et al., 2014; Eigenbrode et al., 2018; Franz et al., 2020; House et al., 2022; Stern et al., 2022); and, 4) biotic remains (McKay et al., 1996). By confirming and understanding the origin and formation of organic carbon on Mars, we will be able to improve our understanding of its putative carbon cycle, the availability of carbon for biotic chemistry, life detection, and how to detect organic compounds on future extraterrestrial missions (McKay et al., 1996). This review article is focused on the published results of Martian hydrocarbon detection missions: Vikings, Phoenix, Curiosity, and Perseverance, highlighting the challenges in detecting indigenous organic carbon on Martian surface, determining their origin, and developing strategy for the future missions.
Existence of water and its role in the evolution and persistence of life on ancient Mars
In contrast to contemporary Mars, which is an aeolian planet containing intermittent liquid water on its surface (likely brines), ancient Mars appears to have had localized habitats that were compatible with the needs of putative early life similar to that existed on Earth (Grotzinger et al., 2014; Grotzinger et al., 2015). Based on crater counts, water channels on the Martian surface are estimated to have formed intermittently between 0.5 and 3.5 billion years ago (Malin, 1976; Masursky et al., 1977). This ancient fluvial areas are unlikely to have been relevant to the current life but might have played a significant role in the evolution of organic compounds and the possible existence of past life. However, the existence of liquid water on the Martian surface was probably sporadic and might have been too brief to allow life to emerge and flourish. So even if a large quantity of water might have flowed during these episodes, it would have vanished in a matter of weeks due to high evaporation rates (Masursky et al., 1977). However, the observations do not rule out the possibility that, in local oases, a steady production of liquid water could have counterbalanced the high evaporation rates and permitted the long-term existence of liquid water (Mazur et al., 1978; Grotzinger et al., 2015).
Findings from Martian meteorites
The presence of organics in meteorites apparently ejected from Mars has been investigated in several studies (McSween Jr, 1994; McKay et al., 1996; Sephton et al., 2002; Steele et al., 2007; Steele et al., 2012; Steele et al., 2016; Eigenbrode et al., 2018). These include reports of organic volatile substances such as benzene, toluene, C2 alkylbenzene, and benzonitrile found by pyrolysis GCMS in Nakhla (Sephton et al., 2002) and polycyclic aromatic hydrocarbons (PAHs) in the Antarctic Martian meteorite ALH 84001 (McKay et al., 1996). Sephton et al. (2002) identified no organic pyrolysis products in their samples of ALH 84001, although they did discover aromatic organics in EET A79001. In this type of study, the amount of terrestrial contribution to Martian meteorites’ organic matter during their residence on Earth (102–104 years) was a primary concern. For PAHs (Becker et al., 1997), amino acids (Bada et al., 1998), and acid-insoluble organic material (Jull et al., 2000), this issue has received considerable attention. Nevertheless, the extent of terrestrial contamination can be assessed by analyzing the environment from which the meteorite was collected, looking for molecules that appear to have been produced abiotically, and measuring carbon and hydrogen isotopes accurately (Mahaffy, 2008; Elsila et al., 2011; Aponte et al., 2014; Elsila et al., 2016; Glavin et al., 2021).
There has been evidence of macromolecular carbon (MMC) in Martian meteorites dating back from 4.2 Ga to 190 Ma (Borg and Draper, 2003; Lapen et al., 2010). Hirschmann and Withers (2008) postulated that carbon cycling in the Martian environment was dominated by its consumption in the reduced mantle which would have limited the release of CO2 to the Martian atmosphere. The presence of MMC species in reduced igneous rocks (Steele et al., 2012) supported the central tenet of Hirschmann and Withers (2008). In contrast, the absence of MMC in inclusions in the most oxidized sample (Nakhla) illustrates the possibility that the redox state of the Martian magmas might affect the preservation of these carbon species during crystallization (Sephton et al., 2002). This has implications for the distribution of MMC on Mars surface and within its crust (Michalski and Niles, 2010; Morris et al., 2010).
No evidence of CH4 or CO2 has been reported from igneous inclusions of Martian Meteorites (Steele et al., 2012). Although there is some skepticism about its existence at all (Zahnle et al., 2011), CH4 has been found in the Martian atmosphere and has been explained by both abiotic and biotic processes (Atreya et al., 2007; Mumma et al., 2009; Webster et al., 2018). According to Steele et al. (2012), occurrence of MMC in igneous rocks could be used as a supporting evidence for abiotic generation of CH4 (Atreya et al., 2007; Mumma et al., 2009). The discovery of ∼190 million years old MMC-containing Martian meteorite, suggests that reduced organic carbon production on Mars has been active in the recent past and it is possible that the Martian carbon cycle may still be active (Steele et al., 2012). Furthermore, according to Steele et al. (2012), primary organic carbon is almost always present in Martian basaltic rocks. It was transported to the surface over the majority of Martian geologic history, as recently as ∼190 million years ago, through igneous mechanisms rather than biological ones. Photolysis of organic carbon (MMC) in the surface sediments or rocks can be a possible abiotic source of CH4 in Martian atmosphere (Keppler et al., 2012; Schuerger et al., 2012).
Findings from various Martian missions
Viking mission
The main goal of the Viking mission was to look for signs of current or past life on Mars (Biemann et al., 1977; Mazur et al., 1978). Viking found clear evidence of the presence of water vapour and water ice, as well as strong inferences that there was once a significant amount of underlying permafrost on Mars. But it found no indication of existing liquid water with the high chemical potential necessary for the survival of terrestrial life, which is in line with the known pressure-temperature relations on the planet’s surface (Mazur et al., 1978). The elemental analysis of the atmosphere and regolith revealed that the chemical elements typically thought to be necessary for terrestrial life are present (Mazur et al., 1978). Viking’s GCMS studies carried by Biemann et al. (1977) and Mazur et al. (1978) found no unambiguous indigenous organic compound. According to Biemann et al. (1977) and Mazur et al. (1978), minor traces of volatile chloro-hydrocarbons detected in Martian regolith samples (Table 1) by the Viking’s GCMS (Figure 1) was a result of terrestrial contamination. Furthermore, they stated that if volatile organic compounds were present in the regolith samples, they were either limited to substances like methane, which were undetectable or only detectable at low sensitivity levels, or they were present in concentrations below the detection limit of the instrument.
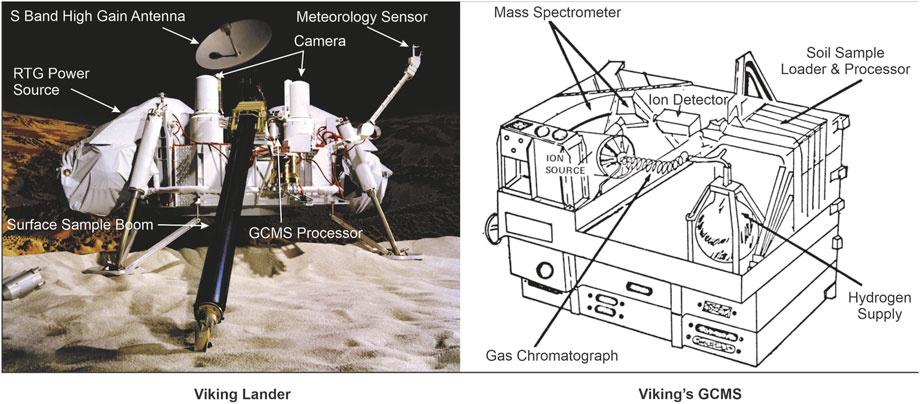
FIGURE 1. Viking Lander and GCMS instruments [sources: Huidobro et al. (2022) and NASA (2010)].
The reasons for the negative results of Viking’s GCMS in detecting the presence of indigenous organic compounds were debated in subsequent studies (Benner et al., 2000; Glavin et al., 2013). According to Benner et al. (2000) and Glavin et al. (2013) the Viking’s GCMS design was not optimized for the detection of certain potential hydrocarbons found in Martian meteorites (Sephton et al., 2002) which could have been present in the Martian samples. Benner et al. (2000) re-examined the Viking’s GCMS results in light of what is known about the oxidation of organic compounds more generally as well as the makeup of organics that are likely to arrive on Mars via meteorite. They came to the conclusion that non-volatile salts of benzenecarboxylic acids, as well as possibly oxalic and acetic acid, should be metastable intermediates of meteoritic hydrocarbons under oxidizing conditions. For the Viking’s GCMS, the salts of these non-volatile organic acids would have been largely undetectable, as the organic molecule must first go through a GC in order to access the MS, which is only possible for volatile molecules. Under pyrolysis, oxalic acid produces carbon dioxide, carbon monoxide, and water (Lewis et al., 2021). In fact, all of these were detected in the Viking’s GCMS, but all these molecules are also present in the Martian atmosphere. Thus, Benner et al. (2000) and Mahaffy (2008) presented three possible reason for the failure of Viking’s GCMS in detecting indigenous organic matter in the Martian regolith’s: 1) the design of the gas-processing system that safeguarded the vacuum ion pumps decreased the GCMS’s sensitivity for light organic compounds by a factor of 1,000; 2) the Viking GC column would not have transmitted numerous kinds of polar organic compounds, such as carboxylic acids, which are probably oxidation products of aliphatic and aromatic hydrocarbons; 3) instead of less permeable materials that would have been better protected from oxidants, the Viking sample-acquisition device could only collect loosely consolidated particles samples.
After the discovery of perchlorate in the Martian arctic soils by the Phoenix lander (Hecht et al., 2009), Navarro-González et al. (2010) ran an analogous experiment in which they heated the Mars-like soil from the Atacama desert containing 32 ppm of organic carbon with 1% magnesium perchlorate and demonstrated that nearly all of the organic present in the samples were decomposed to CO2 and water, with the production of chloromethane and dichloromethane in trace amounts. Navarro-Gonzalez reconsidered the analysis results and suggested the burning of Martian soil sample would essentially decompose all the organics into water and CO2. Based on this experiment they developed a chemical kinetics model for the degree of oxidation and chlorination of organics in the Viking oven and re-analysed the Viking results. According to Navarro-González et al. (2010) re-analysis results, ≤0.1% perchlorate and 1.5–6.5 ppm organic carbon were present in the sample of Viking landing site 1, and ≤1% perchlorate and 0.7–2.6 ppm organic carbon were present in the sample of Viking landing site 2 (Table 1).
Phoenix mission
Phoenix mission touched down on Mars’ polar surface in March 2008 on a valley floor covered by the Scandia Formation, which is thought to be an ancient deposit from the Amazonian period (Arvidson et al., 2008; Smith et al., 2009). This formation encircles the northern edge of a shield volcano called Alba Patera (Arvidson et al., 2008). Phoenix heated soil and ice samples in the Thermal and Evolved-Gas Analyzer (TEGA) (Figure 2) in order to look for organic compounds (Boynton et al., 2009). For a mass range of 2–140 daltons, TEGA was made up of eight differential scanning calorimeter (DSC) ovens that were connected with a mass spectrometer (Boynton et al., 2009). The TEGA DSC monitored endothermic and exothermic processes when samples were heated from ambient temperature to roughly 1,000°C. Throughout the heating process, the mass spectrometer simultaneously detected all evolved gases, including any organic compounds and their byproducts. The evolved gas analysis (EGA) using Phoenix TEGA data did not reveal any organic fragments (Lauer et al., 2009; Ming et al., 2009). A perchlorate salt was found in the soil of Mars by the Microscopy, Electrochemical and Conductivity Analyzer (MECA) and Wet Chemistry Lab (WCL) (Hecht et al., 2009). It is probable that the O2 generated during the thermal decomposition of the perchlorate salt ignited organic fragments evolved in the temperature range of 300°C–600°C. During the heating, CO2 was produced as a byproduct of the burning of organic molecules. According to Ming et al. (2014), the possible causes of low temperature release of CO2 in Phoenix TEGA could be desorption of adsorbed CO2, thermal breakdown of Fe- and Mg-carbonates, and combustion of organic molecules in the Martian soils.
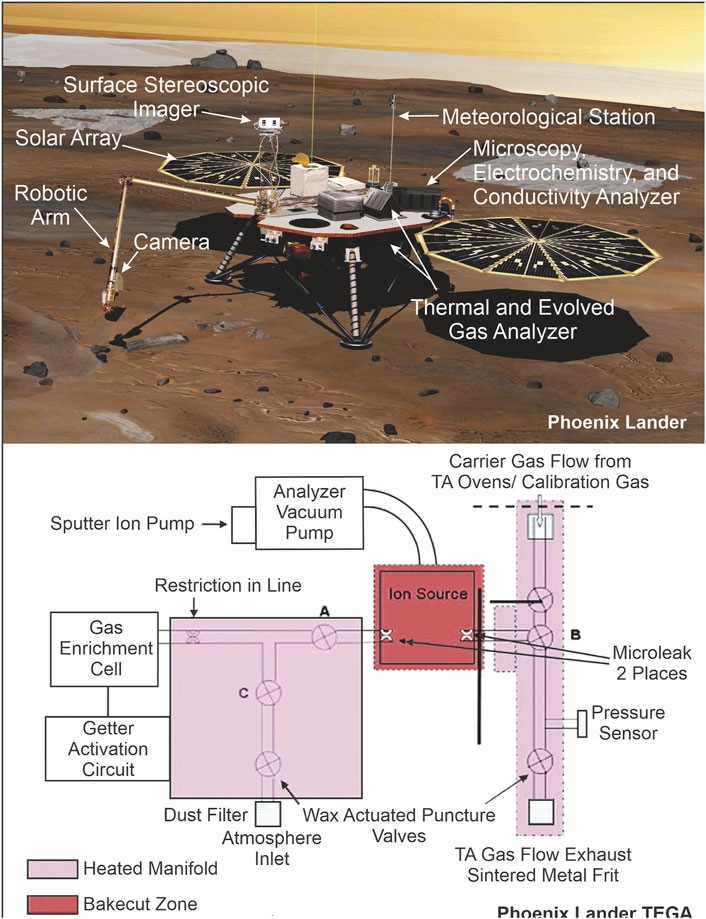
FIGURE 2. Phoenix Lander and TEGA instruments [sources: Huidobro et al. (2022) and NASA (2008)].
Curiosity mission
This is one of the strongest and long-standing missions which landed on Mars (in August 2012) in Aeolis Palus area inside Gale Crater. The main goal of Curiosity is to search the possible traces of life on Mars using the Sample Analysis Mars (SAM) lab suite it carried (Figure 3). SAM onboard the Curiosity rover has the capability to detect complex hydrocarbon molecules having astrobiological implication, by coupling the EGA and GCMS with two type of wet chemistry methods: 1) organic derivatization by adding N-methyl-N-(ter-butyldimethylsilyl) trifluoroacetamide (MTBSTFA) and dimethylformamide (DMF) in 4:1 by volume, 2) organic derivatization by adding 25% tetramethylammonium hydroxide (TMAH) in methanol (Millan et al., 2022a; Millan et al., 2022b). Glavin et al. (2013) reported first SAM-EGA-GCMS data of a Martian solid sample, from Rocknest aeolian deposit of Gale Crater and provided an extensive discussion on the possible origins of chlorinated hydrocarbons detected by SAM. Their studies detected several chlorinated chemical species above EGA background concentration including hydrochloric acid, chloromethane, and dichloromethane (Table 1). As these chlorinated organic species were not detected in the blank EGA run of SAM, the source of this organic carbon was combustion of reduced carbon in the presence of oxygen from oxychlorine. In the blank run 1,3-bis (1,1-dimethylethyl)-1,1,3,3-tetramethyldisiloxane and tert-butyldimethylsilanol were detected by GCMS. These two compounds are the fragmentary products of MTBSTFA (Glavin et al., 2013; Leshin et al., 2013; Millan et al., 2022a; Millan et al., 2022b). Therefore, it is assumed that the MTBSTFA and DMF vapor was perhaps leaked and reacted either with terrestrial or Martian water during the pyrolysis. A major difference between the peaks of 1,3-bis(1,1-dimethylethyl)-1,1,3,3-tetramethyldisiloxane for blank run and Rocknest sample run was that during the pyrolysis of the Rocknest sample, the peak intensity of 1,3-bis(1,1-dimethylethyl)-1,1,3,3-tetramethyldisiloxane sharply decreased with increase in O2 peak. This according to Glavin et al. (2013) was a result of MTBSTFA decomposition along with the chlorate minerals present in the samples which may have contributed to some chlorinated hydrocarbons. However, GCMS analysis of Rocknest samples after pyrolysis detected chlorinated hydrocarbons such as chloromethane, dichloromethane, trichloromethane, choromethylpropene, and chlorobenzene (Table 1) significantly above the background level recorded in black run (Glavin et al., 2013). The concentration of chlorohydrocarbons detected by EGA-GCMS was more than 50 times higher than detected by the Viking-GCMS (Biemann et al., 1977). But, this comparison of detected concentration by the Viking and SAM is less relevant as unlike SAM, Viking did not carry derivatizing agent MTBSTFA. Nevertheless EGA-GCMS analysis at various pyrolysis temperature cut (>500°C) demonstrated that light chlorinated hydrocarbons can be effectively purged from SAM gas processing unit between the analyses. Chlorobenzene was also detected in the blank GCMS run of SAM and to some level above in the Rocknest samples. Its most likely source has been traced to thermal degradation of SAM organic trap (Tenax TA) containing 2,6-diphenylphenylene oxide which can produce Benzene, Toluene, and lesser amount of biphenyl. Hence its sourcing from Rocknest sample was suggested unlikely (Glavin et al., 2013).
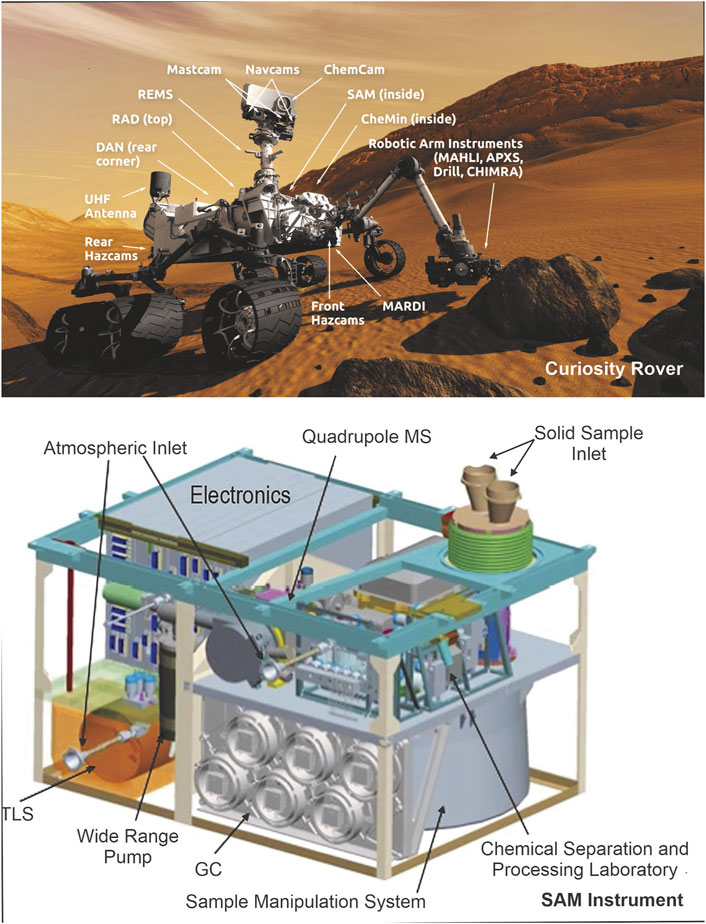
FIGURE 3. Curiosity Rover and SAM instruments [sources: Huidobro et al. (2022) and NASA (2012)].
Based on the results from EGA-GCMS, Freissinet et al. (2015) reported detection of 150–300 ppb chlorobenzene and up to 70 ppb C2-C4 dichloroalkanes in several locations of the Cumberland drill hole in the Sheepbed Mudstone at Yellowknife Bay (Table 1). Their findings indicate that when combined with EGA-GCMS data from multiple scooped and drilled samples, blank runs, and laboratory analogs, the relatively high concentrations of dichloroalkanes and chlorobenzene cannot be a result of the instrument’s internal sourcing. The Sheepbed Mudstone may contain chlorohydrocarbons as such (Szopa et al., 2020), but they are thought to be the products of chemical reactions occurring during pyrolysis between Martian oxychloride and the sample’s organic compounds. The finding of chlorinated organic molecules close to the surface indicates that reduced organic material with covalent bonds has endured despite the presence of several oxidants and high-energy radiation exposure due to the thin Martian atmosphere. Thus, the outcomes of the Freissinet et al. (2015) studies made a significant contribution to identifying possible preservation windows for reduced organic molecules on the Martian surface. In addition, this perspective on ancient Mars offers some context for a potentially habitable environment and represents a first step toward comprehending the existence and variety of potential prebiotic or biotic chemical fingerprints.
To circumvent the perchlorate degradation product of organic matter in the Martian sample analysis, Eigenbrode et al. (2018) analyzed the gases produced exclusively above 400°C, as salt present in the Martian regolith breakdown during heating to a temperature of 200°C in the SAM. Their results provided unambiguous evidence of organic molecules such as thiophenic, aromatic, and aliphatic hydrocarbon from the drill samples of Gale Crater. These sulfur-bearing hydrocarbons are considered to have originated from Mars surface (i.e. igneous, hydrothermal, atmospheric, or biological) and indicates presence of refractory organic materials in the samples (Freissinet et al., 2015; Eigenbrode et al., 2018).
The MTBSTFA derivatization experiment on Mars performed on Ogunquit Beach dune samples of Gale Crater detected several molecules containing benzoic acid, phenol, ammonia and phosphoric acid structures (Millan et al., 2022a; Millan et al., 2022b). Such molecules were either sourced from organic molecules on surface and subsurface environment due to strong oxidizing condition or from MTBSTFA present in SAM background or both (Millan et al., 2022a; Millan et al., 2022b). First TMAH experiment on Mars was performed at Mary Anning (MA) drill site in the Glen Torridon region of Gale Crater that detected a range of hydrocarbons e.g., benzene, toluene, trimethyl- and tetramethyl-benzene, naphthalene, methylnaphthalene, pentamethyl-benzene, benzoic acid methyl ester, dimethyl-, trimethyl-, and tetramethyl-benzenamine, dihydronaphthalene, 2-butyl-thiophene, and benzothiophene (Millan et al., 2022a; Millan et al., 2022b) (Table 1). As TMAH is a methylating agent that break polar bonds and methylate pyrolysis products in situ, suggests that hydrocarbons might have derived from macromolecules which was methylated by TMAH thermochemolysis. In this way pentamethyl benzene could be a product of multimethylation during TMAH reaction. Benzoic acid is detected both in SAM background and Mars samples, therefore its TMAH methylated product benzoic acid methyl ester is of currently unknown sources. The benzenamine may have originated on Mars or it could be a result of benzene reacting again with the N in TMAH. In addition, thiophene has been found in both refractory and mature organic phases in Martian meteorites (Steele et al., 2016; Eigenbrode et al., 2018). Hence, thiophenes found on Mars are thought to be pyrolysis breakdown products from various macromolecules.
Perseverance
The Perseverance rover (Figure 4) was launched by NASA in 2020 (Farley et al., 2020) in an effort to determine the potential habitability of Mars and possible evidence of past life. This mission landed (on February 2021) at Jazero Crater, a 45 km diameter impact structure in the Nili Fossae region of Mars, where a lake and river delta existed approximately 3.5 billion years ago (Mangold et al., 2007; Goudge et al., 2012; Goudge et al., 2015; Goudge et al., 2017; Farley et al., 2020). The main instrument onboard Perseverance to detect signs of past life is SHERLOC (Scanning Habitable Environments with Raman and Luminescence for Organics and Chemicals) (Hollis et al., 2021; Hollis et al., 2022). SHERLOC (Figure 4) uses a deep ultraviolet (DUV) laser to detect fluorescence emissions from aromatic compounds, as well as Raman scattered photons to identify organic functional groups, chemicals, and minerals (Bhartia et al., 2021). This enables SHERLOC to improve Martian studies by analyzing the abundance and variety of organic matter at the fine scale in different minerals to comprehend the potential for organic matter preservation in the Martian surface soils/outcrops, and help resolving organic biosignatures such as hopanes, steranes, and other organic macromolecules (Abbey et al., 2017; Bhartia et al., 2021). SHERLOC’s micro-mapping mode allows non-destructive, sub-picogram sensitive organic detection (Bhartia et al., 2010) to identify smaller-scale features. In order to confirm that all the observed spectral characteristics are intrinsic to the material, the spectrometer’s dust cover will occasionally be opened, and the instrument laser will be fired into the starry night sky (Bhartia et al., 2021). This method makes it possible to assess and eliminate any slight spectrum contamination from the optics or signature from Mars dust pollution deposited on optics from the data processing pipeline. Raman and Fluorescence data from first 208 Martian days of Perseverance on Mars has recently been published by Scheller et al. (2022). These analysis were conducted on three rock targets in two lithological units of the Jazero Crater floor. Reports of detailed scanning of the three samples by Scheller et al. (2022) demonstrated presence of carbonate, sulfate, phosphate, amorphous silicate bearing minerals in association with aromatic organic compounds most likely single- and double-ring aromatics. Carbonation of olivine in presence of briny water has been interpreted as an origin of carbonate minerals, whereas sulfate and perchlorate in the subsurface samples are indicated to have formed by precipitation from briny water. Presence of aromatic organic compounds in all the three targeted rocks is suggested by DUV analysis however, Raman analysis was unable to detect presence of organic compounds most likely due to insufficient organic matter concentration.
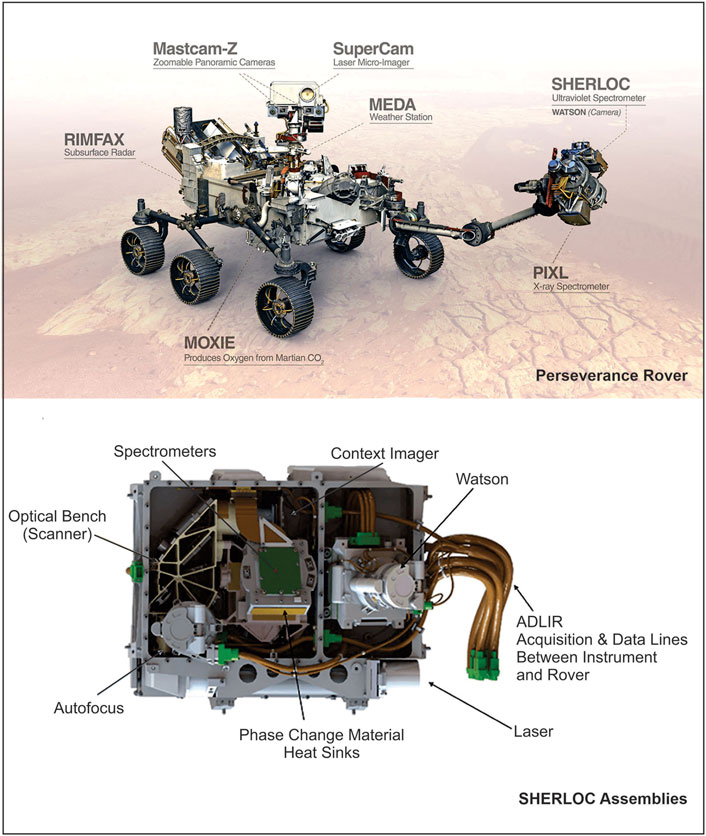
FIGURE 4. Perseverance Rover and SHERLOC instruments [Huidobro et al. (2022) and NASA (2020)].
Future Mars sample return
For more than 4 decades, the planetary science community has placed a high focus on Mars Sample Return (MSR) (Beaty et al., 2019). By analyzing Mars samples in terrestrial laboratories, we could gain a greater understanding of Mars than we could achieve with just in situ missions. Perseverance is capable of collecting samples to return to Earth. In support of its goal to collect sample for possible future Earth return, Perseverance carries an entirely new sampling and catching subsystem (SCS) (Townsend et al., 2022). SCS is used to collect rock core and regolith samples into individual ultraclean and sterile tubes, to photo-document and assess the collected volume of each sample, and to hermetically seal each tube. The sample tube can be manipulated through a sophisticated internal robotic mechanism called the Adaptive Catching Assembly (ACA) (Townsend et al., 2022), which can place the tube on the surface individually, at any time, and in one or more locations from which they can be retrieved. To maximize the likelihood of success of the Mars Sample Return (MSR) campaign duplicates have been collected for each particular target rock. One copy will be deposited at the end of the prime mission in the Jazero deposit identified at Three Forks, to ensure their availability for return to Earth in case Perseverance fail in the future. The second copy will be carried in the rover during its extended mission, with aim of collecting additional samples that the rover will directly deliver to the lander of the retrieval mission. The Mars 2020 science team has identified two hypothetical sample caches in order to examine: 1) the geology of the Jezero Crater, which will be collected during the prime mission, and, 2) the ancient crust outside of the Jezero Crater, which will be collected during a potential extended mission (Herd et al., 2022). The recent successful sample collection by the Perseverance rover and ongoing work by the National Aeronautics and Space Administration (NASA) and European Space Agency (ESA) on developing missions that could retrieve and transport the samples to Earth have put MSR on track to become a reality (Kminek et al., 2022).
Preservation of organic matter on Mars surface
Comets, asteroids, meteorites, and interplanetary dust particles deliver organic compounds to planetary surfaces abiotically (Chyba and Sagan, 1992; Ehrenfreund et al., 2011). Based on scaling the terrestrial influx to a Martian scenario and measurements of the Martian atmosphere, the estimated current influx of abiotic organic molecules to the surface of Mars is around 100–300 tons per year (Eigenbrode et al., 2018). Given that majority of the Martian surface is covered by billions of years old rocks (Carr and Head, 2010), it must have received abundance of organic molecules over this time span. But various Mars lander missions so far found it challenging to detect organic molecules unambiguously on the Martian surface. This suggests a more rapid degradation (by ionic radiation or oxidizing agents) of organic matter than accumulation. However, the crystal structures of minerals found on Mars, such as sulfates and clay minerals, may retain organic molecules, shielding them from the hostile environment (Keil and Mayer, 2014).
The results of the Mars missions to date mostly point to the existence of powerful oxidants and ultraviolet light, which can significantly shorten the residence period of organic matter on the Martian surface and represent the biggest barrier to its identification using in situ techniques. However, recent evidences of organic compounds from SAM observations (Glavin et al., 2013; Freissinet et al., 2015; Eigenbrode et al., 2018; Millan et al., 2022a; Millan et al., 2022b) demonstrate the possibilities and ways to detect in situ organic matter on Mars. Moreover, Applin et al. (2015) found that oxalate minerals are particularly susceptible to accumulation at the surface, as their experiments indicate they are as stable to UV radiation as sulfates and carbonates. Other significant degrading agents for organic materials on Mars are solar energetic particles and galactic cosmic rays which are more powerful than UV as its influence can extends much deeper (up to 9 m) in the subsurface than UV light (Pavlov et al., 2002; Dartnell et al., 2007b; a; Pavlov et al., 2012; Pavlov et al., 2022).
According to Fornaro et al. (2018), it is extremely difficult to develop a general mechanism to predict the behavior of Martian minerals. However, an accurate simulation of Martian environment considering all the key factors would contribute significantly to understanding the trends. Studies of organic matter association with different mineralogy have shown that some minerals aid in organic preservation while others speed up their degradation (Fornaro et al., 2018; Brucato and Fornaro, 2019). For instance, Poch et al. (2015) found that when adenine and glycine molecules were exposed to mid-UV irradiation in iron (III) smectite clay and non-tronite at very high molecule-mineral mass ratios, the effectiveness of the two organic molecules’ photodecomposition was reduced by a factor of 5. This suggests not only physical shielding by non-tronite but also organic molecules stabilization by their interaction with the minerals allow recombination of dissociated organic molecule fragments (Poch et al., 2015). Furthermore, dos Santos et al. (2016) studied preservation of 25 amino acids in various minerals like olivine, enstatite, goethite, hematite, gypsum, jarosite, labradorite, augite, montmorillonite, non-tronite, and saponite, as well as basaltic lava. In their studies, clay minerals were found to exhibit amino acid-protective properties. The typical characteristics of clay minerals, such as their high surface area, which promotes molecular adsorption, their small pore sizes, which prevent radiation from penetrating, and their ideal interlayer sites for the accommodation of organic compounds, which create a shielded environment against external agents, were used to explain this behavior (dos Santos et al., 2016). Such minerals have been detected at several sites on Mars (Bishop et al., 2008; Ehlmann et al., 2009; Ehlmann et al., 2011a; Ehlmann et al., 2011b; Noe Dobrea et al., 2012; Carter et al., 2013; Ehlmann and Edwards, 2014; Vaniman et al., 2014; Adeli et al., 2015; Michalski et al., 2015) which has also been seen as a trace of past active hydrosphere. Millan et al. (2022b) discovered that highest diversity of organic molecules in Martian soil was associated with samples having highest proportion of clay and calcium sulfate minerals. These results comply with the recent findings that phyllosilicate clay minerals show better preservation potential for organic molecules, especially in active Fe-Mn redox environment (Gasda et al., 2022).
Detection of high abundance of thiophenic and other sulfur containing organic carbon in the Murray Formation mudstone (Confidence Hills) infer the possibility that sulfurization of organic matter during deposition or during early diagenesis would also have added their preservation (Eigenbrode et al., 2018). Observations from Earth’s natural environment have indicated that sulfurization of organic matter increases their refractory properties by reducing reactive functional groups and adding small cross links between small unstable molecules (Aubrey et al., 2006; Kotler et al., 2008). Incidentally, studies of terrestrial organic-mineral interaction have demonstrated better preservation of organic molecules by entrapment within the crystal structure (Aubrey et al., 2006; Kotler et al., 2008). Significant capacity to store organic molecules has also been seen in terrestrial hypersaline halite- and perchlorate subsurface deposits, which are common on Mars (Fernández-Remolar et al., 2013).
Applin et al. (2015), who reinterpreted the results collected by various pyrolysis-based instruments aboard Viking, Phoenix, and Curiosity, have recently reported the potential existence of oxalates on Mars in various regions (Biemann et al., 1977; Boynton et al., 2009; Mahaffy et al., 2012; Ming et al., 2014). Furthermore, a re-analysis of a Martian mudstone by Applin et al. (2015) using X-ray diffraction (XRD) of the Mars Science Laboratory (MSL) onboard Curiosity rover revealed that the data are compatible with the existence of refractory Ca, Fe, or Mg oxalates at low levels. It has been shown that oxalates can be produced from other organic compounds through both biological (Johnston and Vestal, 1993; Baran, 2014) and abiotic pathways (Durand and Nicaise, 1980; Peltzer et al., 1984). By interacting with aqueous cations, oxalate in solution forms weddellite, whewellite, and glushinskite, which are extremely resistant to chemical and physical degradation (Wilson et al., 1980; Chen et al., 2000; Frost and Weier, 2003; Frost, 2004). According to Cheng et al. (2016) oxalate formation may be a widespread process in the Martian surface condition and potentially play a key role in global carbon cycling and organic matter geochemistry.
Challenges in distinguishing between biogenic and abiogenic hydrocarbons
Various hydrocarbons can be formed abiotically in terrestrial and extraterrestrial environments by high temperature and pressure Fischer-Tropsch synthesis (McCollom, 2003). Aliphatic hydrocarbons and amino acids are among the byproducts of Fischer-Tropsch type synthesis, according to laboratory investigations; these substances may be oxidized at the surface to more stable molecules. Other abiotic processes may also result in the formation of organics, possibly even in the current Martian environment. Through photolytic or catalytic reactions triggered by light at ultraviolet and visible wavelengths, many organic compounds can be produced, for examples photolysis of water and CO2 mixtures (Franz et al., 2020). Formic acid, formaldehyde, and oxalate, as well as methane and alcohol, have been produced in laboratory studies by these processes. It has also been demonstrated that ultraviolet irradiation of siderite in the presence of water produces formate, formaldehyde, and its derivatives (Franz et al., 2020). These organic compounds may be oxidized on the Martian surface to form oxalate minerals (Benner et al., 2000; Eigenbrode et al., 2018).
SAM stepped combustion experiment study on Yellowknife Bay Mudstone at Gale Crater, Mars by Stern et al. (2022) demonstrated that CO2 evolved at < 550°C was enriched in 13C (δ13C = +1.5‰ ± 3.8‰), based on the mass balance calculation they suggested that 431 μg C/g of the released CO2 represented indigenous organic and inorganic Martian carbon. At > 550°C released CO2 was enriched in 12C (δ13C = −32.9 to −10.1‰) which represented 273 ± 30 μg C/g of mineral-bound refractory organic carbon. This amount of organic carbon is 40 times more than reported by Eigenbrode et al. (2018) and even higher than reported in Martian meteorites (Steele et al., 2016). Franz et al. (2020) found the isotopic composition to be compatible with macromolecular organic carbon derived from igneous sources, meteoritic infall, diagenetically-altered biomass, or a mix of these. Therefore, the source of high-temperature organic carbon cannot be conclusively identified.
Before 2003, all efforts to find statistically significant methane on Mars were unsuccessful (Maguire, 1977; Krasnopolsky et al., 1997; Lellouch et al., 2000). Subsequently four research groups claimed CH4 detection (Formisano et al., 2004; Krasnopolsky et al., 2004; Mumma et al., 2009; Webster et al., 2018; Giuranna et al., 2019). Using Thermal Emission Spectrometer onboard Mars Global Surveyor, Fonti and Marzo, (2010) suggested presence of CH4 on the Martian surface, however, later re-analysis of the data could not reproduce unambiguous evidence for CH4 (Fonti et al., 2015). Many other researchers failed to detect CH4 on Mars (Krasnopolsky, 2007; Villanueva et al., 2013; Aoki et al., 2018; Korablev et al., 2019). More recently House et al. (2022) reported anomalously 13C depleted methane (−137‰ ± 8‰) in the drilled samples from multiple horizons of the Gale Crater of Mars using SAM instruments and skeptically suggested three possible scenarios for its production: 1) photolysis of biological methane, 2) photoreduction of CO2, and 3) deposition of cosmic dust. Nevertheless, they concluded that identifying which of the three scenarios most likely represented the condition that existed on the 3 billion years old Martian surface is not possible based on the current knowledge.
Future Mars mission strategy to detect hydrocarbons
The prerequisites for a future Mars rover mission that would look for organic chemicals on the Martian surface and address their biogenic or abiotic origin must be understood by researchers based on the outcomes of previous Mars missions. So far organic matter search on Mars has achieved moderate success by demonstrating the release of simple organic molecules (i.e., aliphatic hydrocarbons) and some larger molecules (thiophenic and aromatic hydrocarbons) during the pyrolysis of drilled samples from the sedimentary outcrops (Freissinet et al., 2015; Eigenbrode et al., 2018). However, there is still no information about the source organic material present in sedimentary rocks. Also, it is unknown whether that organic matter is linked with a specific mineral phase. Given these uncertainties, combining previously used experimental techniques with new ones to analyze samples collected at a future landing site using both old and new approaches is the most meaningful strategy for continuing the in situ research of organic Mars. This will assure continuity throughout all landed Mars missions until samples are returned from Mars and new data can be compared to that from earlier missions.
The ExoMars rover mission, which will launch in the near future, is a component of the ExoMars project, which also includes the 2016 ExoMars orbiter mission. The ExoMars rover’s high-level scientific objectives are: 1) to look for evidence of past and extant life on Mars, and 2) analyze geochemical and water conditions with increasing depth in the shallow subsurface of Mars. The most important capability of the ExoMars rover payload instrument is to drill and retrieve a 2 m deep core that will be crushed and analyzed for organic matter using Mars Organic Matter Analyzer (Goetz et al., 2016). According to experimental and modeling research (Kminek and Bada, 2006; Pavlov et al., 2012), Martian rocks at a depth of 2 m are expected to remain less affected by UV radiation and galactic cosmic radiation (Pavlov et al., 2002; Pavlov et al., 2012; Pavlov et al., 2022).
In the case of a complicated depositional scenario, where the deposition and denudation cycle takes place, determining whether the subsurface Martian samples were unaffected throughout its history is extremely challenging. For example, given that generally Mars underwent low volcanic activity during the past two billion years, the deposition scenario in which sediment was rapidly deposited, and subsequently exposed for long-term denudation (Gale Crater) might also be a common process on the surface of Mars. However, it is conceivable that the rover could find organic-bearing strata that primarily underwent insignificant exposure to high particle radiation over the geological timescale and may have preserved near original signature of ancient organic deposit. Thus, drilling down to a depth of 2 m would be a huge step toward gaining access to such least altered organic molecule containing Martian rock samples. In order to search the ancient life on ancient Mars, the ExoMars rover requires this insight back in time.
Derivatization/thermochemolysis-GCMS, Laser Desorption and Ionization (LDI)-MS, and Pyrolysis-GCMS are the three operational modes of MOMA. MOMA-MS is not made to identify light molecules (e.g. CO2, N2, O2, CH4, and HCl) that are either a part of Martian atmosphere or change during pyrolysis, even though that mass threshold of MS can be modified during operations (Goetz et al., 2016). In MOMA, derivatization agent (DA) will be chosen during surface operations on Mars based on the kinds of organic residues that are anticipated to be found in the sample. Based on the success of SAM derivatization experiments MTBSTFA seems to be the most versatile DA and it is expected to be used in MOMA. Amino acid or carboxylic acid with chiral centers will be separated using dimethylformamide dimethylacetal (DMF-DMA) that preserves the asymmetrical center and is used with the enantio-selective GC column onboard MOMA to achieve chiral separation. For example, since biology on Earth specifically uses left-handed amino acids, one of the main goals of ExoMars mission will be to determine the homochirality or enantiomeric excess of potential Martian amino acids. Another selected DA is TMAH that will be helpful in volatilizing non-soluble refractory kerogen fraction. In addition, laser techniques such as MOMA-LDI and Raman spectroscopy can be used to avoid thermal decomposition of organic matter (Li et al., 2015).
Discussion and conclusion
From Viking to Mars 2020, rover missions searching for signatures of extinct life in Martian surface rocks and sediments have moderately advanced our knowledge of challenges lying ahead in finding organic molecules of biological origin. These challenges also include understanding the carbon cycling of Mars, identifying sites with better preservation potential for hydrocarbons, developing more refined methods for improved extraction of indigenous organic molecules with minimum contamination. The lack of a non-ambiguous detection of organic molecule by pyrolysis-GCMS method onboard Viking and Phoenix missions (Biemann et al., 1977; Mazur et al., 1978; Lauer et al., 2009) made researchers to reevaluate the design and methods of the corresponding units for the subsequent missions. These findings prompted the use of derivatization wet chemistry in the subsequent rover mission i.e., Curiosity and the future ExoMars mission to enhance the chances of organic molecule detection. This method uses derivatization agents such as MTBSTFA, DMF, and TMAH to improve the thermal stability of compounds and ameliorate volatility by substituting their active polar functional groups (Schummer et al., 2009). Using the derivatization/thermochemolysis method Curiosity detected several Cl, S, and, N containing aliphatic and aromatic compounds (Table 1) above the background level of the instrument (Glavin et al., 2013; Leshin et al., 2013; Ming et al., 2014; Freissinet et al., 2015; Eigenbrode et al., 2018; Millan et al., 2022a; Millan et al., 2022b). There are, however, some unanswered questions about the origin of the detected organic compounds: 1) How much of the detected organic compounds represent Martian indigenous organic compounds? 2) Which and how much of the detected organic compounds are derived from indigenous biogenic/abiogenic hydrocarbons?
MTBSTFA, DMF, and TMAH have been generally employed as derivatizing agents for organic compound studies on Earth with GCMS temperature ramping up between 50°C and 200°C at which the derivatization agents are found relatively stable (Molnar-Perl and Katona, 2000; Schummer et al., 2009). But the MTBSTFA, DMF, and TMAH derivatization experiments by Curiosity on Mars has been conducted at a higher temperature (generally >200°) at which the degradation product of these derivatization agents is not properly known. Recently, a lab based simple flash pyrolysis study of MTBSTFA and DMF demonstrated that both the derivatization agent degrade at higher temperature to produce several organic products including dimethylamino-acetonitrile, styrene, benzene, phenol, entylbenzene, 3-methylebenzonitrile, toluene, napthalene, methyl-naphthalene, naphtonitrile, pyrene, above ≥500°C (Table 2) (He, 2021). Thus, results from Martian missions employing derivatization agents can have contaminant complex organic compounds and need to be interpreted with caution. A systematic study of high temperature degradation products of MTBSTFA, DMF, and TMAH in Martian analog conditions can be helpful in resolving such issue and in refining our current understanding of complex hydrocarbons and their origin in Martian surface and subsurface samples.
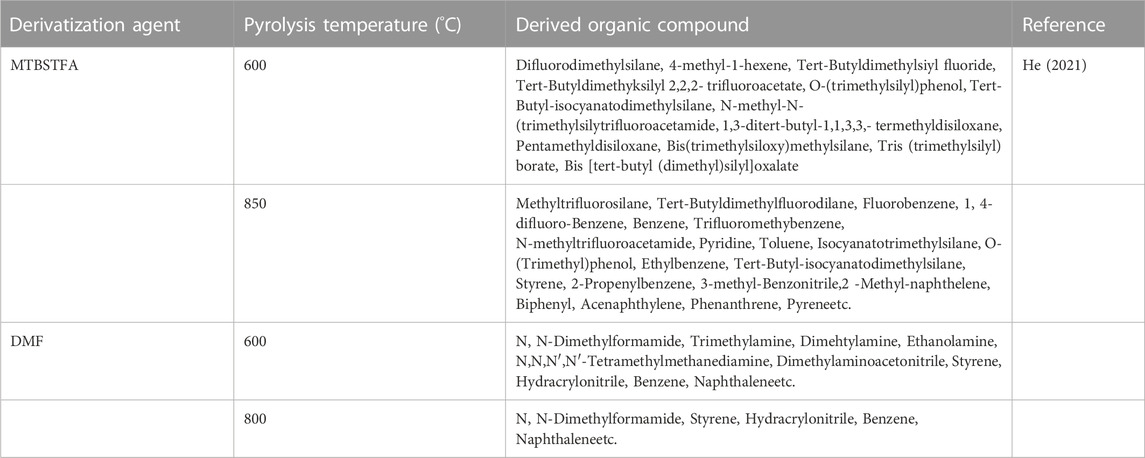
TABLE 2. Lab based flash pyrolysis (at > 500°C) products of derivatization agents MTBSTFA and DMF (He, 2021).
The use of non-destructive techniques, such as the Raman and fluorescence spectroscopy currently employed by Perseverance, and the Raman and infrared spectroscopy plus the laser desorption techniques that will be employed by the future ExoMars mission, will minimize possible alteration and contamination of the original organic material due to the measurements themselves. This is essential to provide more insights into the nature of the organics detected in the Martian samples, while we wait for their return to Earth and analysis with more sensitive techniques.
Author contributions
AA: Conceptualization, writing an original draft, data Curation, reviewing and writing the final draft.
Acknowledgments
I would like thank my colleagues from NASA spaceward bound India initiative for their time-to-time updates on Mars related research that encouraged me to write this review paper. Also, I want to express my gratitude to the reviewers for their insightful comments, which helped this manuscript’s quality tremendously. The BSIP contribution number for this MS is BSIP/RDCC/Publication no 47/2022-2023.
Conflict of interest
The author declares that the research was conducted in the absence of any commercial or financial relationships that could be construed as a potential conflict of interest.
Publisher’s note
All claims expressed in this article are solely those of the authors and do not necessarily represent those of their affiliated organizations, or those of the publisher, the editors and the reviewers. Any product that may be evaluated in this article, or claim that may be made by its manufacturer, is not guaranteed or endorsed by the publisher.
References
Abbey, W. J., Bhartia, R., Beegle, L. W., Deflores, L., Paez, V., Sijapati, K., et al. (2017). Deep UV Raman spectroscopy for planetary exploration: The search for in situ organics. Icarus 290, 201–214. doi:10.1016/j.icarus.2017.01.039
Adeli, S., Hauber, E., Le Deit, L., and Jaumann, R. (2015). Geologic evolution of the eastern Eridania basin: Implications for aqueous processes in the southern highlands of Mars. J. Geophys. Res. Planets 120, 1774–1799. doi:10.1002/2015je004898
Aoki, S., Richter, M., Dewitt, C., Boogert, A., Encrenaz, T., Sagawa, H., et al. (2018). Stringent upper limit of CH4 on Mars based on SOFIA/EXES observations. Astronomy Astrophysics 610, A78. doi:10.1051/0004-6361/201730903
Aponte, J. C., Dworkin, J. P., and Elsila, J. E. (2014). Assessing the origins of aliphatic amines in the Murchison meteorite from their compound-specific carbon isotopic ratios and enantiomeric composition. Geochimica Cosmochimica Acta 141, 331–345. doi:10.1016/j.gca.2014.06.035
Applin, D., Izawa, M., Cloutis, E., Goltz, D., and Johnson, J. (2015). Oxalate minerals on mars? Earth Planet. Sci. Lett. 420, 127–139. doi:10.1016/j.epsl.2015.03.034
Arvidson, R., Adams, D., Bonfiglio, G., Christensen, P., Cull, S., Golombek, M., et al. (2008). Mars Exploration Program 2007 Phoenix landing site selection and characteristics. J. Geophys. Res. Planets 113, E00A03. doi:10.1029/2007je003021
Atreya, S. K., Mahaffy, P. R., and Wong, A.-S. (2007). Methane and related trace species on Mars: Origin, loss, implications for life, and habitability. Planet. Space Sci. 55, 358–369. doi:10.1016/j.pss.2006.02.005
Aubrey, A., Cleaves, H. J., Chalmers, J. H., Skelley, A. M., Mathies, R. A., Grunthaner, F. J., et al. (2006). Sulfate minerals and organic compounds on Mars. Geology 34, 357–360. doi:10.1130/g22316.1
Bada, J. L., Glavin, D. P., Mcdonald, G. D., and Becker, L. (1998). A search for endogenous amino acids in Martian meteorite ALH84001. Science 279, 362–365. doi:10.1126/science.279.5349.362
Baker, V. R. (2006). Geomorphological evidence for water on Mars. Elements 2, 139–143. doi:10.2113/gselements.2.3.139
Baran, E. J. (2014). Review: Natural oxalates and their analogous synthetic complexes. J. Coord. Chem. 67, 3734–3768. doi:10.1080/00958972.2014.937340
Beaty, D. W., Grady, M. M., Mcsween, H. Y., Sefton-Nash, E., Carrier, B. L., Altieri, F., et al. (2019). The potential science and engineering value of samples delivered to Earth by Mars sample return: International MSR Objectives and Samples Team (iMOST). Meteorit. Planet. Sci. 54, S3–S152. doi:10.1111/maps.13242
Becker, L., Glavin, D. P., and Bada, J. L. (1997). Polycyclic aromatic hydrocarbons (PAHs) in Antarctic Martian meteorites, carbonaceous chondrites, and polar ice. Geochimica Cosmochimica Acta 61, 475–481. doi:10.1016/s0016-7037(96)00400-0
Becker, L., Popp, B., Rust, T., and Bada, J. L. (1999). The origin of organic matter in the Martian meteorite ALH84001. Adv. Space Res. 24, 477–488. doi:10.1016/s0273-1177(99)00090-3
Benner, S. A., Devine, K. G., Matveeva, L. N., and Powell, D. H. (2000). The missing organic molecules on Mars. Proc. Natl. Acad. Sci. 97, 2425–2430. doi:10.1073/pnas.040539497
Bhartia, R., Beegle, L. W., Deflores, L., Abbey, W., Razzell Hollis, J., Uckert, K., et al. (2021). Perseverance’s scanning habitable environments with Raman and luminescence for organics and chemicals (SHERLOC) investigation. Space Sci. Rev. 217, 1–115.
Bhartia, R., Salas, E. C., Hug, W. F., Reid, R. D., Lane, A. L., Edwards, K. J., et al. (2010). Label-free bacterial imaging with deep-UV-laser-induced native fluorescence. Appl. Environ. Microbiol. 76, 7231–7237. doi:10.1128/aem.00943-10
Biemann, K., Oro, J., Toulmin, P., Orgel, L., Nier, A., Anderson, D., et al. (1977). The search for organic substances and inorganic volatile compounds in the surface of Mars. J. Geophys. Res. 82, 4641–4658. doi:10.1029/js082i028p04641
Bishop, J. L., Dobrea, E. Z. N., Mckeown, N. K., Parente, M., Ehlmann, B. L., Michalski, J. R., et al. (2008). Phyllosilicate diversity and past aqueous activity revealed at Mawrth Vallis, Mars. Science 321, 830–833. doi:10.1126/science.1159699
Borg, L. E., and Draper, D. S. (2003). A petrogenetic model for the origin and compositional variation of the Martian basaltic meteorites. Meteorit. Planet. Sci. 38, 1713–1731. doi:10.1111/j.1945-5100.2003.tb00011.x
Boynton, W., Ming, D., Kounaves, S., Young, S., Arvidson, R., Hecht, M., et al. (2009). Evidence for calcium carbonate at the Mars Phoenix landing site. Science 325, 61–64. doi:10.1126/science.1172768
Brucato, J. R., and Fornaro, T. (2019). “Role of mineral surfaces in prebiotic processes and space-like conditions,” in Biosignatures for Astrobiology (Springer), 183–204.
Carr, M. H., and Head, J. W. (2010). Geologic history of mars. Earth Planet. Sci. Lett. 294, 185–203. doi:10.1016/j.epsl.2009.06.042
Carrier, B. L., Beaty, D. W., Meyer, M. A., Blank, J., Chou, L., Dassarma, S., Des Marais, D., Eigenbrode, J., Grefenstette, N., and Lanza, N. (2020). Mars extant life: What's next? Conference report. Astrobiology 20, 785–814. doi:10.1089/ast.2020.2237
Carter, J., Poulet, F., Bibring, J. P., Mangold, N., and Murchie, S. (2013). Hydrous minerals on Mars as seen by the CRISM and OMEGA imaging spectrometers: Updated global view. J. Geophys. Res. Planets 118, 831–858. doi:10.1029/2012je004145
Chen, J., Blume, H.-P., and Beyer, L. (2000). Weathering of rocks induced by lichen colonization—A review. Catena 39, 121–146. doi:10.1016/s0341-8162(99)00085-5
Cheng, Z., Fernández-Remolar, D., Izawa, M., Applin, D., Chong Díaz, M., Fernandez-Sampedro, M., et al. (2016). Oxalate formation under the hyperarid conditions of the Atacama desert as a mineral marker to provide clues to the source of organic carbon on Mars. J. Geophys. Res. Biogeosciences 121, 1593–1604. doi:10.1002/2016jg003439
Chyba, C., and Sagan, C. (1992). Endogenous production, exogenous delivery and impact-shock synthesis of organic molecules: An inventory for the origins of life. Nature 355, 125–132. doi:10.1038/355125a0
Dartnell, L. R., Desorgher, L., Ward, J. M., and Coates, A. J. (2007a). Martian sub-surface ionising radiation: Biosignatures and geology. Biogeosciences 4, 545–558. doi:10.5194/bg-4-545-2007
Dartnell, L. R., Desorgher, L., Ward, J. M., and Coates, A. J. (2007b). Modelling the surface and subsurface Martian radiation environment: Implications for astrobiology. Geophys. Res. Lett. 34, L02207. doi:10.1029/2006gl027494
Davis, W. L., and Mckay, C. P. (1996). Origins of life: A comparison of theories and application to mars. Orig. Life Evol. Biosphere 26, 61–73. doi:10.1007/bf01808160
Dos Santos, R., Patel, M., Cuadros, J., and Martins, Z. (2016). Influence of mineralogy on the preservation of amino acids under simulated Mars conditions. Icarus 277, 342–353. doi:10.1016/j.icarus.2016.05.029
Durand, B., and Nicaise, G. (1980). Kerogen: Insoluble organic matter from sedimentary rocks. Paris: Editions technip, 35–53.
Ehlmann, B. L., and Edwards, C. S. (2014). Mineralogy of the martian surface. Annu. Rev. Earth Planet. Sci. 42, 291–315. doi:10.1146/annurev-earth-060313-055024
Ehlmann, B. L., Mustard, J. F., Clark, R. N., Swayze, G. A., and Murchie, S. L. (2011a). Evidence for low-grade metamorphism, hydrothermal alteration, and diagenesis on Mars from phyllosilicate mineral assemblages. Clays Clay Minerals 59, 359–377. doi:10.1346/ccmn.2011.0590402
Ehlmann, B. L., Mustard, J. F., Murchie, S. L., Bibring, J.-P., Meunier, A., Fraeman, A. A., et al. (2011b). Subsurface water and clay mineral formation during the early history of Mars. Nature 479, 53–60. doi:10.1038/nature10582
Ehlmann, B. L., Mustard, J. F., Swayze, G. A., Clark, R. N., Bishop, J. L., Poulet, F., et al. (2009). Identification of hydrated silicate minerals on Mars using MRO-CRISM: Geologic context near Nili Fossae and implications for aqueous alteration. J. Geophys. Res. Planets 114, E00D08. doi:10.1029/2009je003339
Ehrenfreund, P., Röling, W., Thiel, C., Quinn, R., Sephton, M., Stoker, C., et al. (2011). Astrobiology and habitability studies in preparation for future mars missions: Trends from investigating minerals, organics and biota. Int. J. Astrobiol. 10, 239–253. doi:10.1017/s1473550411000140
Eigenbrode, J. L., Summons, R. E., Steele, A., Freissinet, C., Millan, M., Navarro-González, R., et al. (2018). Organic matter preserved in 3-billion-year-old mudstones at Gale crater, Mars. Science 360, 1096–1101. doi:10.1126/science.aas9185
Elsila, J. E., Aponte, J. C., Blackmond, D. G., Burton, A. S., Dworkin, J. P., and Glavin, D. P. (2016). Meteoritic amino acids: Diversity in compositions reflects parent body histories. ACS Central Sci. 2, 370–379. doi:10.1021/acscentsci.6b00074
Elsila, J. E., Callahan, M. P., Glavin, D. P., Dworkin, J. P., and Brückner, H. (2011). Distribution and stable isotopic composition of amino acids from fungal peptaibiotics: Assessing the potential for meteoritic contamination. Astrobiology 11, 123–133. doi:10.1089/ast.2010.0505
Fairén, A. G., Davila, A. F., Lim, D., Bramall, N., Bonaccorsi, R., Zavaleta, J., et al. (2010). Astrobiology through the ages of mars: The study of terrestrial analogues to understand the habitability of mars. Astrobiology 10, 821–843. doi:10.1089/ast.2009.0440
Farley, K. A., Williford, K. H., Stack, K. M., Bhartia, R., Chen, A., De La Torre, M., et al. (2020). Mars 2020 mission overview. Space Sci. Rev. 216, 1–41. doi:10.1007/s11214-020-00762-y
Fernández-Remolar, D. C., Chong-Díaz, G., Ruíz-Bermejo, M., Harir, M., Schmitt-Kopplin, P., Tziotis, D., et al. (2013). Molecular preservation in halite- and perchlorate-rich hypersaline subsurface deposits in the Salar Grande basin (Atacama Desert, Chile): Implications for the search for molecular biomarkers on Mars. J. Geophys. Res. Biogeosciences 118, 922–939. doi:10.1002/jgrg.20059
Fonti, S., Mancarella, F., Liuzzi, G., Roush, T., Frouard, M. C., Murphy, J., et al. (2015). Revisiting the identification of methane on Mars using TES data. Astronomy Astrophysics 581, A136. doi:10.1051/0004-6361/201526235
Fonti, S., and Marzo, G. (2010). Mapping the methane on mars. Astronomy Astrophysics 512, A51. doi:10.1051/0004-6361/200913178
Formisano, V., Atreya, S., Encrenaz, T., Ignatiev, N., and Giuranna, M. (2004). Detection of methane in the atmosphere of Mars. science 306, 1758–1761. doi:10.1126/science.1101732
Fornaro, T., Steele, A., and Brucato, J. R. (2018). Catalytic/protective properties of martian minerals and implications for possible origin of life on Mars. Life 8, 56. doi:10.3390/life8040056
Franz, H., Mahaffy, P., Webster, C., Flesch, G., Raaen, E., Freissinet, C., et al. (2020). Indigenous and exogenous organics and surface–atmosphere cycling inferred from carbon and oxygen isotopes at Gale crater. Nat. Astron. 4, 526–532. doi:10.1038/s41550-019-0990-x
Freissinet, C., Glavin, D., Mahaffy, P. R., Miller, K., Eigenbrode, J., Summons, R., et al. (2015). Organic molecules in the sheepbed mudstone, gale crater, Mars. J. Geophys. Res. Planets 120, 495–514. doi:10.1002/2014je004737
Frost, R. L. (2004). Raman spectroscopy of natural oxalates. Anal. Chim. Acta 517, 207–214. doi:10.1016/j.aca.2004.04.036
Frost, R. L., and Weier, M. L. (2003). Raman spectroscopy of natural oxalates at 298 and 77 K. J. Raman Spectrosc. 34, 776–785. doi:10.1002/jrs.1052
Gasda, P. J., Comellas, J., Essunfeld, A., Das, D., Bryk, A. B., Dehouck, E., et al. (2022). Overview of the morphology and chemistry of diagenetic features in the clay-rich glen Torridon unit of Gale Crater, mars. J. Geophys. Res. Planets 127, e2021JE007097. doi:10.1029/2021je007097
Giuranna, M., Viscardy, S., Daerden, F., Neary, L., Etiope, G., Oehler, D., et al. (2019). Independent confirmation of a methane spike on Mars and a source region east of Gale Crater. Nat. Geosci. 12, 326–332. doi:10.1038/s41561-019-0331-9
Glavin, D. P., Elsila, J. E., Mclain, H. L., Aponte, J. C., Parker, E. T., Dworkin, J. P., et al. (2021). Extraterrestrial amino acids and L-enantiomeric excesses in the CM 2 carbonaceous chondrites Aguas Zarcas and Murchison. Meteorit. Planet. Sci. 56, 148–173. doi:10.1111/maps.13451
Glavin, D. P., Freissinet, C., Miller, K. E., Eigenbrode, J. L., Brunner, A. E., Buch, A., et al. (2013). Evidence for perchlorates and the origin of chlorinated hydrocarbons detected by SAM at the Rocknest aeolian deposit in Gale Crater. J. Geophys. Res. Planets 118, 1955–1973. doi:10.1002/jgre.20127
Goetz, W., Brinckerhoff, W., Arevalo, R., Freissinet, C., Getty, S., Glavin, D., et al. (2016). Moma: The challenge to search for organics and biosignatures on mars. Int. J. Astrobiol. 15, 239–250. doi:10.1017/s1473550416000227
Goudge, T. A., Milliken, R. E., Head, J. W., Mustard, J. F., and Fassett, C. I. (2017). Sedimentological evidence for a deltaic origin of the Western fan deposit in Jezero crater, Mars and implications for future exploration. Earth Planet. Sci. Lett. 458, 357–365. doi:10.1016/j.epsl.2016.10.056
Goudge, T. A., Mustard, J. F., Head, J. W., and Fassett, C. I. (2012). Constraints on the history of open-basin lakes on Mars from the composition and timing of volcanic resurfacing. J. Geophys. Res. Planets 117. doi:10.1029/2012je004115
Goudge, T. A., Mustard, J. F., Head, J. W., Fassett, C. I., and Wiseman, S. M. (2015). Assessing the mineralogy of the watershed and fan deposits of the Jezero crater paleolake system, Mars. J. Geophys. Res. Planets 120, 775–808. doi:10.1002/2014je004782
Greenwood, J. P., Itoh, S., Sakamoto, N., Vicenzi, E. P., and Yurimoto, H. (2008). Hydrogen isotope evidence for loss of water from Mars through time. Geophys. Res. Lett. 35, L05203. doi:10.1029/2007gl032721
Grotzinger, J. P., Gupta, S., Malin, M. C., Rubin, D. M., Schieber, J., Siebach, K., et al. (2015). Deposition, exhumation, and paleoclimate of an ancient lake deposit, Gale crater, Mars. Science 350, aac7575. doi:10.1126/science.aac7575
Grotzinger, J. P., Sumner, D. Y., Kah, L., Stack, K., Gupta, S., Edgar, L., et al. (2014). A habitable fluvio-lacustrine environment at Yellowknife Bay, Gale Crater, mars. Science 343, 1242777. doi:10.1126/science.1242777
He, Y. (2021). Search for organic compounds with MTBSTFA/DMF derivatization and TMAH thermochemolysis on Mars. Univ. Paris-Saclay.
Hecht, M. H., Kounaves, S. P., Quinn, R., West, S. J., Young, S. M., Ming, D. W., et al. (2009). Detection of perchlorate and the soluble chemistry of martian soil at the Phoenix lander site. Science 325, 64–67. doi:10.1126/science.1172466
Herd, C., Bosak, T., Stack, K., Sun, V., Gupta, S., Shuster, D., et al. (2022). The notional plan for sample collections by the perseverance rover for Mars sample return.
Hirschmann, M. M., and Withers, A. C. (2008). Ventilation of CO2 from a reduced mantle and consequences for the early Martian greenhouse. Earth Planet. Sci. Lett. 270, 147–155. doi:10.1016/j.epsl.2008.03.034
Hollis, J. R., Abbey, W., Beegle, L. W., Bhartia, R., Ehlmann, B. L., Miura, J., et al. (2021). A deep-ultraviolet Raman and Fluorescence spectral library of 62 minerals for the SHERLOC instrument onboard Mars 2020. Planet. Space Sci. 209, 105356. doi:10.1016/j.pss.2021.105356
Hollis, J. R., Moore, K. R., Sharma, S., Beegle, L., Grotzinger, J. P., Allwood, A., et al. (2022). The power of paired proximity science observations: Co-Located data from SHERLOC and PIXL on mars. Icarus 387, 115179. doi:10.1016/j.icarus.2022.115179
House, C. H., Wong, G. M., Webster, C. R., Flesch, G. J., Franz, H. B., Stern, J. C., et al. (2022). Depleted carbon isotope compositions observed at Gale crater, Mars. Proc. Natl. Acad. Sci. 119, e2115651119. doi:10.1073/pnas.2115651119
Huidobro, J., Aramendia, J., Arana, G., and Madariaga, J. M. (2022). Reviewing in situ analytical techniques used to research Martian geochemistry: From the Viking Project to the MMX future mission. Anal. Chim. Acta 1197, 339499. doi:10.1016/j.aca.2022.339499
Johnston, C. G., and Vestal, J. R. (1993). Biogeochemistry of oxalate in the Antarctic cryptoendolithic lichen-dominated community. Microb. Ecol. 25, 305–319. doi:10.1007/bf00171895
Jull, A., Beck, J., and Burr, G. (2000). Isotopic evidence for extraterrestrial organic material in the Martian meteorite, Nakhla. Geochimica Cosmochimica Acta 64, 3763–3772. doi:10.1016/s0016-7037(00)00458-0
Jull, A., Courtney, C., Jeffrey, D., and Beck, J. (1998). Isotopic evidence for a terrestrial source of organic compounds found in Martian meteorites Allan Hills 84001 and Elephant Moraine 79001. Science 279, 366–369. doi:10.1126/science.279.5349.366
Keppler, F., Vigano, I., Mcleod, A., Ott, U., Früchtl, M., and Röckmann, T. (2012). Ultraviolet-radiation-induced methane emissions from meteorites and the Martian atmosphere. Nature 486, 93–96. doi:10.1038/nature11203
Kminek, G., and Bada, J. L. (2006). The effect of ionizing radiation on the preservation of amino acids on Mars. Earth Planet. Sci. Lett. 245, 1–5. doi:10.1016/j.epsl.2006.03.008
Kminek, G., Meyer, M. A., Beaty, D. W., Carrier, B. L., Haltigin, T., and Hays, L. E. (2022). Mars sample return (MSR): Planning for returned sample science. Astrobiology 22, S-1–S-4. doi:10.1089/ast.2021.0198
Korablev, O., Vandaele, A. C., Montmessin, F., Fedorova, A. A., Trokhimovskiy, A., Forget, F., et al. (2019). No detection of methane on mars from early ExoMars trace gas orbiter observations. Nature 568, 517–520. doi:10.1038/s41586-019-1096-4
Kotler, J. M., Hinman, N. W., Yan, B., Stoner, D. L., and Scott, J. R. (2008). Glycine identification in natural jarosites using laser desorption fourier transform mass spectrometry: Implications for the search for life on mars. Astrobiology 8, 253–266. doi:10.1089/ast.2006.0102
Krasnopolsky, V. A. (2007). Comment on “methane on mars: A product of H2O photolysis in the presence of CO” by A. Bar-nun and V. Dimitrov. Icarus 188, 540–542. doi:10.1016/j.icarus.2006.12.019
Krasnopolsky, V. A., Maillard, J. P., and Owen, T. C. (2004). Detection of methane in the martian atmosphere: Evidence for life? Icarus 172, 537–547. doi:10.1016/j.icarus.2004.07.004
Krasnopolsky, V., Bjoraker, G., Mumma, M., and Jennings, D. (1997). High-resolution spectroscopy of mars at 3.7 and 8 μm: A sensitive search for H2O2, H2CO, HCl, and CH4, and detection of hdo. J. Geophys. Res. Planets 102, 6525–6534. doi:10.1029/96je03766
Lapen, T. J., Righter, M., Brandon, A., Debaille, V., Beard, B., Shafer, J., et al. (2010). A younger age for ALH84001 and its geochemical link to shergottite sources in Mars. Science 328, 347–351. doi:10.1126/science.1185395
Lauer, H., Ming, D., Sutter, B., Golden, D., Morris, R., and Boynton, W. (2009). “thermal and evolved gas analysis of magnesium perchlorate: Implications for perchlorates in soils at the mars Phoenix landing site perchlorates in soils at the mars Phoenix landing site,” in 40th annual lunar and planetary science conference), 2196.
Lellouch, E., Encrenaz, T., De Graauw, T., Erard, S., Morris, P., Crovisier, J., et al. (2000). The 2.4– spectrum of Mars observed with the infrared space observatory. Planet. Space Sci. 48, 1393–1405. doi:10.1016/s0032-0633(00)00118-5
Leshin, L., Mahaffy, P., Webster, C., Cabane, M., Coll, P., Conrad, P., et al. (2013). Volatile, isotope, and organic analysis of martian fines with the Mars Curiosity rover. science 341, 1238937. doi:10.1126/science.1238937
Lewis, J., Eigenbrode, J., Wong, G., Mcadam, A., Archer, P., Sutter, B., et al. (2021). Pyrolysis of oxalate, acetate, and perchlorate mixtures and the implications for organic salts on Mars. J. Geophys. Res. Planets 126, e2020JE006803. doi:10.1029/2020je006803
Li, X., Danell, R. M., Brinckerhoff, W. B., Pinnick, V. T., Van Amerom, F., Arevalo, R. D., et al. (2015). Detection of trace organics in Mars analog samples containing perchlorate by laser desorption/ionization mass spectrometry. Astrobiology 15, 104–110. doi:10.1089/ast.2014.1203
Maguire, W. C. (1977). Martian isotopic ratios and upper limits for possible minor constituents as derived from Mariner 9 infrared spectrometer data. Icarus 32, 85–97. doi:10.1016/0019-1035(77)90051-3
Mahaffy, P. (2008). Exploration of the habitability of Mars: Development of analytical protocols for measurement of organic carbon on the 2009 Mars Science Laboratory. Space Sci. Rev. 135, 255–268. doi:10.1007/s11214-007-9223-1
Mahaffy, P. R., Webster, C. R., Cabane, M., Conrad, P. G., Coll, P., Atreya, S. K., et al. (2012). The sample analysis at Mars investigation and instrument suite. Space Sci. Rev. 170, 401–478. doi:10.1007/s11214-012-9879-z
Malin, M. C. (1976). Age of martian channels. J. Geophys. Res. 81, 4825–4845. doi:10.1029/jb081i026p04825
Mangold, N., Poulet, F., Mustard, J., Bibring, J. P., Gondet, B., Langevin, Y., et al. (2007). Mineralogy of the Nili Fossae region with OMEGA/mars express data: 2. Aqueous alteration of the crust. J. Geophys. Res. Planets 112. doi:10.1029/2006je002835
Marais, D. D., and Walter, M. (1999). Astrobiology: Exploring the origins, evolution, and distribution of life in the universe. Annu. Rev. Ecol. Syst. 30, 397–420. doi:10.1146/annurev.ecolsys.30.1.397
Masursky, H., Boyce, J., Dial, A., Schaber, G., and Strobell, M. (1977). Classification and time of formation of Martian channels based on Viking data. J. Geophys. Res. 82, 4016–4038. doi:10.1029/js082i028p04016
Mazur, P., Barghoorn, E. S., Halvorson, H. O., Jukes, T. H., Kaplan, I. R., and Margulis, L. (1978). Biological implications of the viking mission to mars. Space Sci. Rev. 22, 3–34. doi:10.1007/bf00215812
Mccollom, T. M. (2003). Formation of meteorite hydrocarbons from thermal decomposition of siderite (FeCO3). Geochimica Cosmochimica Acta 67, 311–317. doi:10.1016/s0016-7037(02)00945-6
Mckay, D. S., Gibson, E. K., Thomas-Keprta, K. L., Vali, H., Romanek, C. S., Clemett, S. J., et al. (1996). Search for past life on mars: Possible relic biogenic activity in martian meteorite ALH84001. Science 273, 924–930. doi:10.1126/science.273.5277.924
Mcsween, H. Y. (1994). What we have learned about Mars from SNC meteorites. Meteoritics 29, 757–779. doi:10.1111/j.1945-5100.1994.tb01092.x
Michalski, J. R., Cuadros, J., Bishop, J. L., Dyar, M. D., Dekov, V., and Fiore, S. (2015). Constraints on the crystal-chemistry of Fe/Mg-rich smectitic clays on Mars and links to global alteration trends. Earth Planet. Sci. Lett. 427, 215–225. doi:10.1016/j.epsl.2015.06.020
Michalski, J. R., and Niles, P. B. (2010). Deep crustal carbonate rocks exposed by meteor impact on Mars. Nat. Geosci. 3, 751–755. doi:10.1038/ngeo971
Millan, M., Teinturier, S., Malespin, C., Bonnet, J., Buch, A., Dworkin, J., et al. (2022a). Organic molecules revealed in Mars’s Bagnold Dunes by Curiosity’s derivatization experiment. Nat. Astron. 6, 129–140. doi:10.1038/s41550-021-01507-9
Millan, M., Williams, A. J., Mcadam, A. C., Eigenbrode, J. L., Steele, A., Freissinet, C., et al. (2022b). Sedimentary organics in glen Torridon, Gale Crater, mars: Results from the SAM instrument suite and supporting laboratory analyses. J. Geophys. Res. Planets 127, e2021JE007107. doi:10.1029/2021je007107
Ming, D., Morris, R., Niles, B., Lauer, H., Archer, P., Sutter, B., et al. (2009). “Combustion of organic molecules by the thermal decomposition of perchlorate salts: Implications for organics at the Mars Phoenix Scout Landing Site,” in 40th lunar and planetary science conference).
Ming, D. W., Archer, P., Glavin, D., Eigenbrode, J., Franz, H., Sutter, B., et al. (2014). Volatile and organic compositions of sedimentary rocks in Yellowknife Bay, Gale Crater, mars. science 343, 1245267. doi:10.1126/science.1245267
Molnar-Perl, I., and Katona, Z. F. (2000). GC-MS of amino acids as their trimethylsilyl/t-butyldimethylsilyl derivatives: In model solutions III. Chromatographia 51, S228–S236. doi:10.1007/bf02492811
Morris, R. V., Ruff, S. W., Gellert, R., Ming, D. W., Arvidson, R. E., Clark, B. C., et al. (2010). Identification of carbonate-rich outcrops on Mars by the Spirit rover. Science 329, 421–424. doi:10.1126/science.1189667
Mumma, M. J., Villanueva, G. L., Novak, R. E., Hewagama, T., Bonev, B. P., Disanti, M. A., et al. (2009). Strong release of methane on Mars in northern summer 2003. Science 323, 1041–1045. doi:10.1126/science.1165243
Nasa (2012). Curiosity rover mission overview. [Online]. Available at: https://mars.nasa.gov/msl/mission/overview/(Accessed November, 2022).
Nasa (2020). Mars 2020 mission perseverance rover. [Online]. Available at: https://mars.nasa.gov/mars2020/spacecraft/instruments/(Accessed November, 2022).
Nasa (2008). Phoenix lander on mars. [Online]. Available at: https://mars.nasa.gov/resources/1929/phoenix-lander-on-mars/.[Accessed 2022.
Nasa (2010). Viking lander model. [Online]. Available at: https://mars.nasa.gov/resources/3355/viking-lander-model/(Accessed November, 2022).
Navarro-González, R., Vargas, E., De La Rosa, J., Raga, A. C., and Mckay, C. P. (2010). Reanalysis of the Viking results suggests perchlorate and organics at midlatitudes on Mars. J. Geophys. Res. Planets 115, E12010. doi:10.1029/2010je003599
Nisbet, E., and Sleep, N. (2001). The habitat and nature of early life. Nature 409, 1083–1091. doi:10.1038/35059210
Noe Dobrea, E., Wray, J., Calef, F., Parker, T., and Murchie, S. (2012). Hydrated minerals on Endeavour Crater's rim and interior, and surrounding plains: New insights from CRISM data. Geophys. Res. Lett. 39. doi:10.1029/2012gl053180
Oparin, A. (1965). The origin of life and the origin of enzymes. Adv. Enzymol. Relat. Areas Mol. Biol. 27, 347–380. doi:10.1002/9780470122723.ch7
Pavlov, A. A., Mclain, H. L., Glavin, D. P., Roussel, A., Dwork2in, J. P., Elsila, J. E., et al. (2022). Rapid radiolytic degradation of amino acids in the martian shallow subsurface: Implications for the search for extinct life. Astrobiology 22, 1099–1115. doi:10.1089/ast.2021.0166
Pavlov, A. K., Blinov, A. V., and Konstantinov, A. N. (2002). Sterilization of Martian surface by cosmic radiation. Planet. Space Sci. 50, 669–673. doi:10.1016/s0032-0633(01)00113-1
Pavlov, A., Vasilyev, G., Ostryakov, V., Pavlov, A., and Mahaffy, P. (2012). Degradation of the organic molecules in the shallow subsurface of Mars due to irradiation by cosmic rays. Geophys. Res. Lett. 39. doi:10.1029/2012gl052166
Peltzer, E., Bada, J., Schlesinger, G., and Miller, S. (1984). The chemical conditions on the parent body of the Murchison meteorite: Some conclusions based on amino, hydroxy and dicarboxylic acids. Adv. Space Res. 4, 69–74. doi:10.1016/0273-1177(84)90546-5
Poch, O., Jaber, M., Stalport, F., Nowak, S., Georgelin, T., Lambert, J.-F., et al. (2015). Effect of nontronite smectite clay on the chemical evolution of several organic molecules under simulated martian surface ultraviolet radiation conditions. Astrobiology 15, 221–237. doi:10.1089/ast.2014.1230
Scheller, E. L., Hollis, J. R., Cardarelli, E. L., Steele, A., Beegle, L. W., Bhartia, R., et al. (2022). Aqueous alteration processes in Jezero crater, Mars− implications for organic geochemistry. Science 378, 1105–1110. doi:10.1126/science.abo5204
Schuerger, A. C., Moores, J. E., Clausen, C. A., Barlow, N. G., and Britt, D. T. (2012). Methane from UV-irradiated carbonaceous chondrites under simulated Martian conditions. J. Geophys. Res. Planets 117. doi:10.1029/2011je004023
Schulze-Makuch, D., Irwin, L. N., and Guan, H. (2002). Search parameters for the remote detection of extraterrestrial life. Planet. Space Sci. 50, 675–683. doi:10.1016/s0032-0633(01)00121-0
Schummer, C., Delhomme, O., Appenzeller, B. M., Wennig, R., and Millet, M. (2009). Comparison of MTBSTFA and BSTFA in derivatization reactions of polar compounds prior to GC/MS analysis. Talanta 77, 1473–1482. doi:10.1016/j.talanta.2008.09.043
Sephton, M. A., and Hazen, R. M. (2013). On the origins of deep hydrocarbons. Rev. Mineralogy Geochem. 75, 449–465. doi:10.2138/rmg.2013.75.14
Sephton, M., Wright, I., Gilmour, I., De Leeuw, J., Grady, M., and Pillinger, C. (2002). High molecular weight organic matter in martian meteorites. Planet. Space Sci. 50, 711–716. doi:10.1016/s0032-0633(02)00053-3
Smith, P. H., Tamppari, L., Arvidson, R., Bass, D., Blaney, D., Boynton, W. V., et al. (2009). H2O at the Phoenix landing site. Science 325, 58–61. doi:10.1126/science.1172339
Steele, A., Fries, M., Amundsen, H., Mysen, B., Fogel, M., Schweizer, M., et al. (2007). Comprehensive imaging and Raman spectroscopy of carbonate globules from Martian meteorite ALH 84001 and a terrestrial analogue from Svalbard. Meteorit. Planet. Sci. 42, 1549–1566. doi:10.1111/j.1945-5100.2007.tb00590.x
Steele, A., Mccubbin, F., Fries, M., Kater, L., Boctor, N., Fogel, M., et al. (2012). A reduced organic carbon component in martian basalts. Science 337, 212–215. doi:10.1126/science.1220715
Steele, A., Mccubbin, F. M., and Fries, M. D. (2016). The provenance, formation, and implications of reduced carbon phases in Martian meteorites. Meteorit. Planet. Sci. 51, 2203–2225. doi:10.1111/maps.12670
Stern, J. C., Malespin, C. A., Eigenbrode, J. L., Webster, C. R., Flesch, G., Franz, H. B., et al. (2022). Organic carbon concentrations in 3.5-billion-year-old lacustrine mudstones of Mars. Proc. Natl. Acad. Sci. 119, e2201139119. doi:10.1073/pnas.2201139119
Stern, R., and Jedrzejas, M. J. (2008). Carbohydrate polymers at the center of life’s origins: The importance of molecular processivity. Chem. Rev. 108, 5061–5085. doi:10.1021/cr078240l
Szopa, C., Freissinet, C., Glavin, D. P., Millan, M., Buch, A., Franz, H. B., et al. (2020). First detections of dichlorobenzene isomers and trichloromethylpropane from organic matter indigenous to mars mudstone in Gale Crater, mars: Results from the sample analysis at mars instrument onboard the curiosity rover. Astrobiology 20, 292–306. doi:10.1089/ast.2018.1908
Townsend, J., Klein, D., and Brooks, S. (2022). “Robotics verification and validation strategies for perseverance rover sampling and caching,” in IEEE aerospace conference (AERO) (IEEE), 1–13.
Vaniman, D., Bish, D., Ming, D., Bristow, T., Morris, R., Blake, D., et al. (2014). Mineralogy of a mudstone at Yellowknife Bay, Gale Crater, mars. science 343, 1243480. doi:10.1126/science.1243480
Villanueva, G., Mumma, M., Novak, R., Radeva, Y., Käufl, H., Smette, A., et al. (2013). A sensitive search for organics (CH4, CH3OH, H2CO, C2H6, C2H2, C2H4), hydroperoxyl (HO2), nitrogen compounds (N2O, NH3, HCN) and chlorine species (HCl, CH3Cl) on Mars using ground-based high-resolution infrared spectroscopy. Icarus 223, 11–27. doi:10.1016/j.icarus.2012.11.013
Webster, C. R., Mahaffy, P. R., Atreya, S. K., Moores, J. E., Flesch, G. J., Malespin, C., et al. (2018). Background levels of methane in Mars’ atmosphere show strong seasonal variations. Science 360, 1093–1096. doi:10.1126/science.aaq0131
Wilson, M., Jones, D., and Russell, J. (1980). Glushinskite, a naturally occurring magnesium oxalate. Mineral. Mag. 43, 837–840. doi:10.1180/minmag.1980.043.331.02
Yen, A., Kim, S., Hecht, M., Frant, M., and Murray, B. (2000). Evidence that the reactivity of the martian soil is due to superoxide ions. Science 289, 1909–1912. doi:10.1126/science.289.5486.1909
Yen, A. S., Murray, B., Rossman, G. R., and Grunthaner, F. J. (1999). Stability of hydroxylated minerals on mars: A study on the effects of exposure to ultraviolet radiation. J. Geophys. Res. Planets 104, 27031–27041. doi:10.1029/1999je001065
Zahnle, K., Freedman, R. S., and Catling, D. C. (2011). Is there methane on Mars? Icarus 212, 493–503. doi:10.1016/j.icarus.2010.11.027
Keywords: Mars, organic matter detection, Vikings, Phoenix, Curiosity, ExoMars, extraterrestrial life, Perseverance
Citation: Ansari AH (2023) Detection of organic matter on Mars, results from various Mars missions, challenges, and future strategy: A review. Front. Astron. Space Sci. 10:1075052. doi: 10.3389/fspas.2023.1075052
Received: 20 October 2022; Accepted: 31 January 2023;
Published: 10 February 2023.
Edited by:
Alberto Fairén, Center for Astrobiology (CSIC), SpainReviewed by:
Teresa Fornaro, Osservatorio Astrofisico di Arcetri (INAF), ItalyJennifer Claire Stern, Goddard Space Flight Center, National Aeronautics and Space Administration, United States
Copyright © 2023 Ansari. This is an open-access article distributed under the terms of the Creative Commons Attribution License (CC BY). The use, distribution or reproduction in other forums is permitted, provided the original author(s) and the copyright owner(s) are credited and that the original publication in this journal is cited, in accordance with accepted academic practice. No use, distribution or reproduction is permitted which does not comply with these terms.
*Correspondence: Arif H. Ansari, YS5oLmFuc2FyaUBic2lwLnJlcy5pbg==,