- 1Department of Radiology, New Jersey Medical School, Rutgers University, Newark, NJ, United States
- 2Natural Sciences Department, Middlesex College, Edison, NJ, United States
- 3Department of Health Sciences, Canadian Nuclear Laboratories, Chalk River, ON, Canada
Introduction: With current goals of increased space exploration and travel to Mars, there has been great interest in understanding the long-term effects of high atomic number, high energy (HZE) ion exposure on various organ systems and the immune system. Little is known about late effects on the immune system after HZE exposure. Therefore, our objective was to determine how natural killer (NK) cell populations were affected in geriatric mice that were exposed to HZE particles during middle-age, thereby representing elderly retired astronauts that undertook deep space missions.
Methods: 10 month old male CBA/CaJ mice were whole-body irradiated: sham (control); 150-cGy gamma-rays (delivered in 1 fraction); 40-cGy 1-GeV/nu 28Si14+ ions (delivered in 3 fractions); 40-cGy 1-GeV/nu 16O8+ ions (1 fraction); and 40-cGy 1-GeV/nu 16O8+ ions (3 fractions). The mice were sacrificed 1–1.5 yr post-exposure, and the spleens harvested. Splenocyte effector (E) cells were harvested and added to 51Cr-labeled Yac-1 target (T) cells in E:T ratios of 12:1, 25:1, 50:1, and 100:1. NK cytotoxicity was measured with 51Cr release. In addition, 2 million splenocytes were aliquoted and stained with a seven-antibody cocktail, and flow cytometry was used to determine the percentage of NK, B lymphocytes, and T lymphocytes in the splenocyte population.
Results: Mice exposed to either a single fraction of 150-cGy gamma rays or 40-cGy 16O8+ ions in 3 fractions were found to have significant decreases in NK cytotoxicity of approximately 30% and 25%, respectively. No significant differences were observed in NK cytotoxicity for 40-cGy 16O8+ ions delivered in 1 fraction, or 40-cGy 28Si14+ ions delivered in 3 fractions. No significant differences were observed in the percentage of spleen cells that were NK (%NK) amongst the groups.
Conclusion: Fractionated HZE ion exposure has the potential to affect the innate arm of the immune system long after exposure, leading to decreases in NK cell function. Therefore, protective countermeasures may need to be considered to decrease the risk of reduced long-term immune function in elderly retired astronauts that undertook deep space missions.
Introduction
The mission to Mars has been a key objective for NASA in recent years. One obstacle in achieving this objective is the high-Z, high-energy (HZE) ion radiation exposure astronauts will face during the voyage duration. Current NASA guidelines allow astronauts a permissible career exposure limit corresponding to 3% risk of radiation-exposure-induced death (REID) for cancer at a 95% confidence level to protect against uncertainties in risk projections (NASA, 2022). The average effective dose an astronaut receives while spending 6 months in the International Space Station is approximately 72 mSv (Cucinotta et al., 2008). An astronaut undergoing a journey to and from Mars is expected to be exposed to approximately 660 mSv (Cucinotta et al., 2012). It has been estimated that during the journey, every cell in the body will be traversed by a low LET proton once every 3–4 days, and an HZE ion every few months (Kerr, 2013). Despite the much lower traversal incidence of HZE particles, their high linear energy transfer (LET) nature produces more complex DNA damage along their trajectory, which is difficult for cellular DNA-repair mechanisms to repair. Potential consequences can include chronic oxidative stress within the cell and surrounding bystander cells (Buonanno et al., 2011), as well as permanent DNA damage which can lead to cell dysregulation and cancer (Bielefeldt-Ohmann et al., 2012).
Exposure to HZE-ions has been demonstrated to have effects in multiple organ systems, including the nervous system and cardiovascular systems (Boerma et al., 2015a; Boerma et al., 2015b; Klein et al., 2021). Another critical organ system that may be potentially affected from heavy ion exposure is the immune system. The immune system is involved in combating invasion from outside sources, such as bacteria, viruses, and parasites, as well as normal surveillance of host cells. Dysregulation of the immune system can lead to a myriad of both acute and chronic issues, including infection, autoimmune disease, and cancer (Ikeda and Togashi, 2022). One component of the immune system is the natural killer (NK) cell. While historically considered part of the innate arm of the immune response, NK cells interact with both the innate and adaptive arms of the immune response (Vivier et al., 2011). In conjunction with CD8+ T lymphocytes, NK cells are involved in the detection and elimination of cancer cells. Where CD8+ T lymphocytes require the expression of antigens on major histocompatibility complex (MHC) Class I receptors to carry out their function, NK cells are able to react to cells avoiding immunodetection through decreased MHC Class I surface expression (Karre et al., 1986). Additionally, NK cells can also recognize NKG2D ligands, a family of ligands that are expressed at low levels in healthy tissues but become elevated under cellular stress conditions, including ULBP1-6 and MICA/B (Zingoni et al., 2018). The NK cell’s cytotoxic function is activated upon recognition, causing cell death (Abel et al., 2018). Recent research has also demonstrated that NK cells may possess the ability to “remember,” similar to memory T cells (Beaulieu, 2021). When NK cell populations are depressed, both in size and function long-term, there is an elevated risk for viral infections (Orange, 2013; Mace and Orange, 2016) and cancer initiation and progression (Nakajima et al., 1987; Chiossone et al., 2018; Moon and Powis, 2019). In an 11-year study of a Japanese resident cohort, subjects in the lowest tertile for NK cytotoxic function were more likely to develop cancer (Imai et al., 2000). Other studies found that patients with poor NK cell infiltration had worse prognoses for multiple cancer types, highlighting the importance of the NK cell’s role in the immune system (Coca et al., 1997; Ishigami et al., 2000a; Ishigami et al., 2000b; Villegas et al., 2002).
It has been well established that cells of the immune system are sensitive to ionizing radiation (Kajioka et al., 2000; Heylmann et al., 2014). Astronauts returning from spaceflight were shown to have considerable decreases in circulating immune cells as early as the 1960s (Crucian et al., 2020). Recovery to pre-flight levels have also been observed to be delayed, as long as 2 months post-return despite the relatively short flight duration. Previous studies in astronauts have shown alterations in the immune system after both short-term (<1 month) (Konstantinova et al., 1995; Crucian et al., 2013) and long-term (≥1 month) spaceflights (Buchheim et al., 2019). More recently, the monozygous twin study conducted by NASA also observed changes in both immune cell number and gene expression after spaceflight, again demonstrating alterations in both immune cell population size and function (Garrett-Bakelman et al., 2019). Studies on the cytotoxic function of NK cells of rat splenocytes after short-duration flights on COSMOS 2044 demonstrated decreased ability to kill target cells (Rykova et al., 1992; Lesnyak et al., 1996). NK cell function in astronauts has also been observed to be decreased upon return from spaceflight (Mehta et al., 2001), with substantial variability in the time required to return to normal function. However, these spaceflights were in low Earth orbit (LEO) where the magnetosphere provides some protection against HZE particles, a protection that will not be available to astronauts traveling to and from Mars. Furthermore, they did not follow NK function over time scales commensurate with an astronaut reaching a geriatric stage of life. Therefore, the present study was conducted to determine the long-term effects of HZE-ion exposure on splenic NK cell population size and function. Ten-month old mice were irradiated in our study to represent astronauts about 40 years of age. A 1–1.5-year postirradiation period between irradiation and NK assay was undertaken to simulate an elderly retired Mars astronaut.
Materials and methods
The overall experimental approach is depicted in Figure 1. Details regarding the individual steps are described below.
Cell culture
YAC-1 mouse lymphoma cells (kindly provided by Drs. Vincent Tsiagbe and Nicholas Ponzio) were grown in suspension in a 75 cm2 flask containing 15 ml RPMI media (Sigma-Aldrich) supplemented with 2 mM l-glutamine (Gibco), 200 UI penicillin/200 μg/ml streptomycin (Corning), and 10% fetal bovine serum (Gibco) at 37°C with 5% CO2. Cells were passaged at a 1:10 ratio every 3 days. Cells used in the NK cytotoxic function assay were split 1:3 the day prior.
Animals
Male CBA/CaJ mice aged 10 months (Jackson Laboratories) were used for this study. They were raised at Jackson Laboratories prior to being shipped directly to the NSRL Animal Facility, where they were acclimated for 7–10 days. Mice were then irradiated as described below, and 4–10 days after irradiation they were shipped to the Rutgers RBHS Animal Facility in Newark, NJ, where they were housed on a 12 h light/dark cycle and given Purina chow and water ad libitum.
Irradiation
Irradiations were done at the NASA Space Radiation Laboratory (NSRL) located at Brookhaven National Laboratory (BNL). Groups of mice were placed in a multi-compartment ventilated chamber made of 5-mm thick Lucite (Figure 2). Each chamber held a single mouse and they were arranged one-deep along a rectangular grid containing many chambers. Unsedated mice were loaded quickly into their chambers and whole-body irradiated with 0 cGy (control), 40-cGy 1-GeV/u 28Si14+ ions (delivered in 3 consecutive fractions (3F), 1 per day), 40-cGy 1-GeV/u 16O8+ ions (delivered in 1 fraction (1F)), or 40-cGy 1-GeV/u 16O8+ ions (3F, 1 per day). The isovelocity (1 GeV/u) 16O8+ and 28Si14+ ions have LET values of 14 and 44 keV/μm in water, respectively. Calculations using the software application SRIM showed that the LETs of these ions changed by less than 1% and 3%, respectively, upon traversing 5 cm of water. Another group was whole-body irradiated at BNL with 1 fraction of 662-keV gamma rays using a JL Shepherd Mark I irradiator (1 Gy/min) and given an absorbed dose of 150 cGy. Details of the mouse groups are provided in Table 1. All animal studies were approved by the IACUC at both Rutgers and BNL.
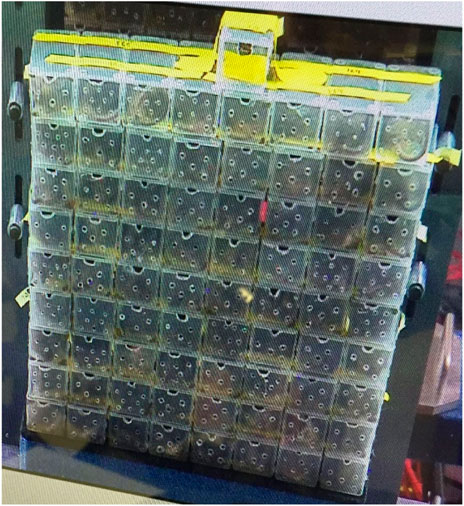
FIGURE 2. Setup for irradiating mice with 1 GeV/u 28Si14+ or 16O8+ ions. The 9 × 8 structure holds 72 mice in individual compartments. Ventilation holes and a high-speed fan kept animals at comfortable temperature.
Spleen harvest and splenocyte isolation
The spleen plays significant roles in hematopoiesis, red blood cell clearance, and immunological functions. Its immunological functions are conducted primarily be the splenocytes (white blood cells in the spleen). There are many subpopulations of splenocytes such as T cells, B cells, and NK cells, each playing different roles in the immune system. Spleens were harvested from animals 1–1.5 yr after radiation exposure. Animals were given a ketamine/xylazine anesthetic cocktail, followed by cervical dislocation. Splenocytes were manually separated from the spleen by scraping the spleens over a wire mesh in RPMI medium with a rubber policeman, layered onto Histopaque® (Sigma-Aldrich) and centrifuged at 400 g for 30 min. The buffy coat layer containing splenocytes was carefully removed with a pipet, and the cells were washed and centrifuged in culture media twice at 400 g for 10 min and cell concentrations were then determined with a Coulter ZM cell counter.
Natural killer cytotoxic function assay
To assess the NK cell activity, YAC-1 cells were suspended in 1 ml fresh medium and labeled with 9.25 MBq (250 μCi) of 51Cr sodium chromate (Perkin Elmer, NEZ30001MC (185 MBq/mL)) for 1 h at 37°C. Two washes with media were done to remove extracellular activity. After labeling, 5000 YAC-1 cells (targets, T) were seeded into wells of a 96 well plate in 0.1 ml medium. Splenocytes (effectors, E) were then seeded into the wells at effector:target (E:T) ratios of 12.5:1, 25:1, 50:1, and 100:1. The plate was then incubated for 5 h at 37°C and 95% air, 5% CO2. After incubation, the plate was spun at 500 g for 5 min, and 100 µl aliquots of the supernatant were aliquoted in triplicate to 12 X 75 tubes for 51Cr release measurement with a Cobra gamma counter (Packard). The intracellular 51Cr is released when the NK cells lyse the target YAC-1 cells and therefore 51Cr in the supernatant is used as a measure of cell kill. Accordingly, the 51Cr gamma ray counts per minute (cpm) were recorded for each sample. The % Kill was calculated for each group, i, according to the standard chromium release assay as per Eq. 1.
where cpmi is for the supernatant of the test sample (targets + effectors), cpmspontaneous release is for the supernatant of the target cell only sample (no effectors), and cpmtotal release is for the supernatant of lysed sample (targets + effectors).
Splenic cell subpopulation characterization
To characterize radiation-induced changes in the representation of key subpopulations of the splenocytes, two million splenocytes were aliquoted into a 5-ml tube and stained with an antibody cocktail containing the following: BUV395 anti-CD3e (clone 145-2C11 BD Horizon™), Alexa Fluor 700 anti-CD4 (clone RM4-5 BioLegend®), PE/Cy7 anti-CD8a (clone 53–6.7 BioLegend®), FITC anti-CD45R/B220 (clone RA3-6 B2 BioLegend®), PE anti-IgM (clone RMM-1 BioLegend®), and Pacific blue anti-CD49b (clone DX5 BioLegend®). The stained cells were analyzed by flow cytometry using 6 color analysis on a BD LSRFortessa™ X-20 equipped with 5 lasers (355, 405, 488, 561, and 642 nm). Compensation parameters were set using single-stained OneComp® control beads (eBioScience) prior to samples being run. NK cells were identified as being CD4 negative (CD4–), CD8 negative (CD8–), IgM negative (IgM–), B220R negative (B220R–), CD49b positive (CD49b+), and CD3 negative (CD3–). The resulting FCS files were imported into FlowJo™ (BD) for gating analysis. Identification of splenocyte subpopulations is given in Table 2.
Analysis
Figures and statistical analysis for subpopulation characterization were done in SigmaPlot 14.5. One-way ANOVA using Bonferroni’s method was used to determine if subpopulations were significantly affected. When the one-way ANOVA arrived at no significance (p > 0.05), then two-tailed t-tests for each treatment relative to control were conducted. Figures for NK cytotoxic function were created in SigmaPlot 14.5. The NK cytotoxicity data was analyzed for statistical significance in OriginPro® (OriginLab®) using F tests that compared unweighted linear least-squares fits for each dataset with that of control.
Results
Changes in CD4+ and natural killer cell populations
The relative number of splenocytes that were harvested from each group is plotted in Figure 3A. The number of splenocytes in the spleens of irradiated mice were not significantly different than controls when tested with the one-way ANOVA. However, t-tests between individual groups and control indicated that the 150-cGy gamma-ray treated mice had significantly more splenocytes compared to unirradiated control (p = 0.023, Figure 3A). In contrast, mice exposed to a single fraction or fractionated schedule of the selected ions showed no significant changes in splenocyte number compared to control (Figure 3A).
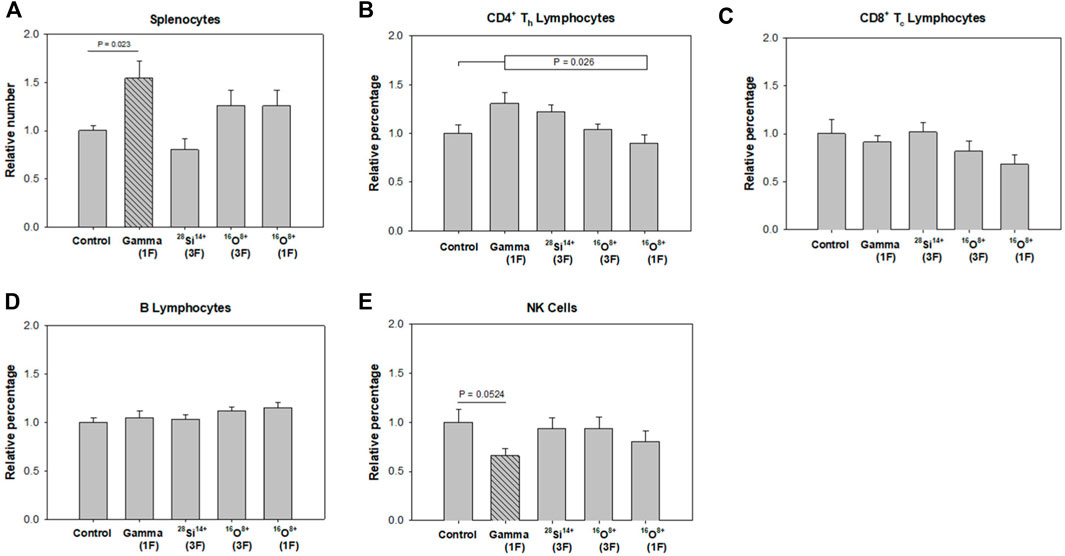
FIGURE 3. Changes in splenocyte numbers and splenocyte subpopulation percentages relative to unirradiated controls at 1–1.5 yr after irradiation of 10-month old mice with 0 cGy (Control, n = 13), 150-cGy gamma-rays in a single fraction [Gamma (1F), n = 6], 40-cGy 28Si14+ given in three fractions [28Si14+ (3F), n = 6], 40-cGy 16O8+ given in three fractions [16O8+ (3F), n = 12], and 40-cGy 16O8+ given as a single fraction [16O8+ (1F), n = 12]. (A) splenocytes, (B) CD4+ Th lymphocytes, (C) CD8+ Tc lymphocytes, (D) B lymphocytes, (E) NK cells. Error bars are standard error of the mean. The cross-hatched bars indicate a statistically significant difference (p < 0.05) from control.
A one-way ANOVA indicated that the percentages of CD4+ Th lymphocytes in each group of irradiated animals were not significantly different than in the control group. However, it did give significance when irradiated groups taken together were compared to the control group (p = 0.026, Figure 3B). Finally, a one-way ANOVA did give significance when pairwise comparing the 16O8+ single-fraction treated animals and the gamma-ray treated animals (p = 0.038). No significant changes were found among the CD8+ Tc lymphocyte and B lymphocyte populations among any of the groups (Figures 3C,D).
Finally, a one-way ANOVA indicated that the percentages of NK cells in each group of irradiated animals were not significantly different than in the control group. However, a t-test indicated that the 150-cGy gamma-ray treated mice demonstrated a decrease in percentage of NK cells when compared to unirradiated control (p = 0.052, Figure 3E).
Cytotoxic function of natural killer cells
The %Kill are plotted as a function of E:T ratios for each irradiation condition (Figure 4). The 150-cGy gamma-ray treated mice were shown to have significantly decreased NK cytotoxic function compared to sham-irradiated control (p = 7.55 × 10−4). The fractionated 40-cGy 28Si14+ treated mice showed no significant difference (p = 0.74). The single-fraction 40-cGy 16O8+ treated mice also did not show any significant difference compared to control (p = 0.63), however the fractionated 40-cGy 16O8+ treated mice showed significant decreases in NK cytotoxic function compared to control (p = 0.0036).
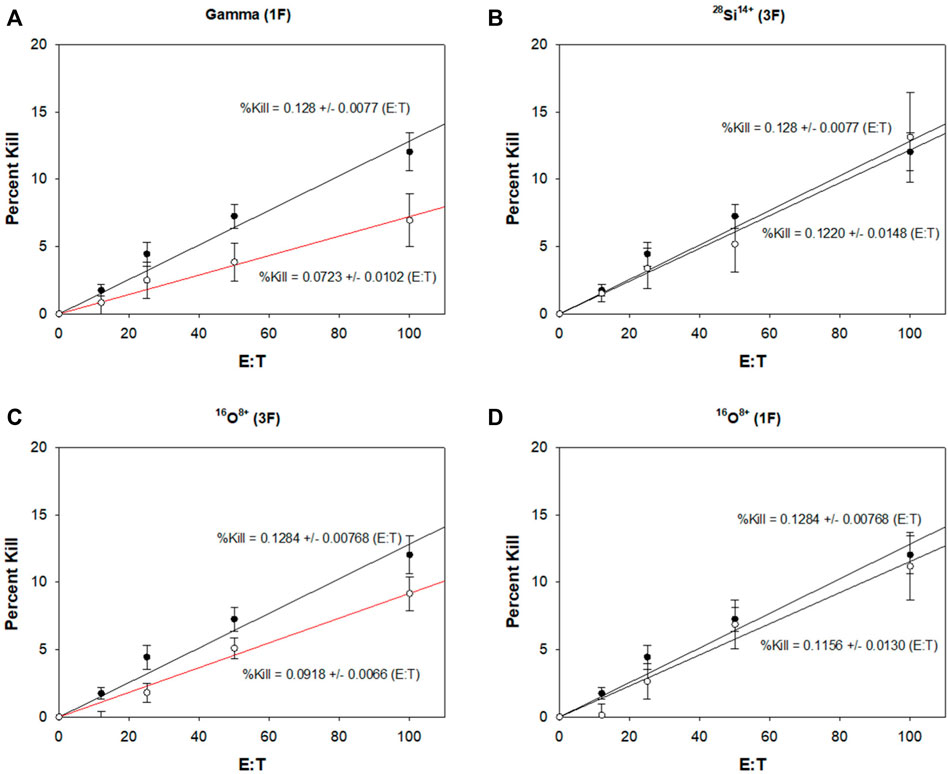
FIGURE 4. Effects of heavy ion exposure on NK cell cytotoxic function 1–1.5 yr after irradiation of 10-month old mice. Unirradiated controls (•, n = 13) are shown in each plot. (A) 150-cGy gamma rays given in a single fraction [Gamma (1F), n = 6], (B) 40-cGy 28Si14+ given in three fractions [28Si14+ (3F), n = 6], (C) 40-cGy 16O8+ given in three fractions [16O8+ (3F), n = 12], and (D) 40-cGy 16O8+ given as a single fraction (16O8+ (1F), n = 12). Linear least squares fits to the data are indicated by the solid lines. The slopes and their standard error are provided for each fit. Significance relative to controls is denoted by the red lines for p < 0.05. Both gamma (1F) and 16O8+ (3F) treatment groups demonstrated decreased NK cytotoxic function compared to control. Error bars are standard error of the mean.
Discussion
Given that alterations in size and function of NK populations can have a significant impact on long-term health, our studies on HZE-radiation-induced changes in NK cells are important for risk assessment for astronauts that conduct extended missions in space. The mice used in this study were 10 months old at irradiation, corresponding to a typical middle-aged adult astronaut, while the 1–1.5 yr assay timepoint was intended to correspond to the time required for a retired astronaut to reach a geriatric stage of life. This timepoint was selected to determine whether an astronaut’s radiation exposure during their career would have lasting effects on NK cells, at a stage of life where overall immunity has decreased with age and cancer susceptibility has increased. Our 150-cGy gamma-ray positive control group showed a depressed splenic NK cell population percentage even 1–1.5 yr post-exposure when compared to the sham-irradiated negative control (Figure 3E). Additionally, these mice demonstrated significantly decreased NK cytotoxic function (Figure 4A), which would translate to impaired ability to combat both viral infections as well as precancerous cells. Interestingly, despite an unaltered splenic NK cell population percentage (Figure 3E), the fractionated 40-cGy 16O8+ treated mice also demonstrated a significant decrease in NK cytotoxic function (Figure 4C). This contrasts the response to single-fraction 40-cGy 16O8+ (Figure 4D) and fractionated 40-cGy 28Si14+ treated mice (Figure 4B), which both demonstrated no significant differences in either splenic NK population size or NK cytotoxic function. Curiously, despite their lower LET, fractionated 16O8+ ions (14 keV/μm) had a greater impact on NK cytotoxic function than 28Si14+ ions (44 keV/μm). These results suggest that NK cytotoxic function may be altered by exposure to HZE particles on a specific heavy ion basis rather than simply on an LET basis, although it should be noted that the sample size for 28Si14+ ions is small (n = 6). Furthermore, the exposure schedule may also play a role in determining whether NK cell function is impacted. Given that in deep space every cell in the body will be traversed by a low-LET proton once every 3–4 days, and an HZE ion every few months (Kerr, 2013), the fractionated regimens used in this work are more akin to space exposures than the single fraction. Therefore, the fact that NK cytotoxic function was affected long after exposure to fractionated 40-cGy 16O8+ and not by the single-fraction 40-cGy 16O8+ is significant and warrants further exploration with this and other HZE particles. Furthermore, it is notable that the NK cell population percentage was similar to control in this case (Figure 3E), yet the NK cells demonstrated impaired cytotoxic function (Figure 4C). When taking into consideration that the radiation exposure will be a fractionated mix of HZE particles during spaceflight, these results suggest long-term overall suppression and decreased function of the NK cells, which in turn may lead to elevated risk to both cancer and viral infections. This conclusion is tempered by the fact that animals were not irradiated with a mixture of protons and HZE ions. As stated above, a given cell in the astronaut’s body will be traversed by low-LET protons before being traversed by an HZE ion. It has been demonstrated that pre-exposure with protons can mitigate adverse effects of a subsequent exposure to HZE particles (Buonanno et al., 2015; Fornalski et al., 2022). As summarized in a recent report from the National Aeronautics and Space Agency (NASA), there is considerable experimental evidence for adaptive responses (Huff et al., 2016). Others have discussed the data from astronauts on the International Space Station (ISS) who were tested for chromosome aberrations before, after 1 mission, and then again after a 2nd mission (Chancellor et al., 2014). Aberrations were increased after the first mission but decreased over time to an above pre-mission level, and then did not increase further after the second mission. The evidence for adaptive responses has prompted proposals to select astronauts for deep space missions based on their intrinsic capacity for robust adaptive responses (Mortazavi et al., 2003; Bevelacqua and Mortazavi, 2017). Their adaptive responses for a variety of biological endpoints could be tested in vitro in ground-based studies using tissues from prospective astronauts (Mortazavi et al., 2003). Such an approach could use exposure sequences comprised of protons and HZE ions using the NSRL galactic cosmic ray simulator for rapid sequences of the different radiations, or spaced further in time using protons first, followed by HZE ions.
One area of note is that the mice used in this study were male. Differences in immune response due to sexual dimorphism have been well documented; for instance, females were found to have lower incidences of bacterial, viral, and parasitic infections, but higher incidences of autoimmune diseases when compared to males (Shepherd et al., 2020). A study on NK degranulation activity in response to Hepatitis B viral infection also found higher levels of spontaneous degranulation in female patients compared to their male counterparts, despite identical degranulation capacity (Macek Jilkova et al., 2017). Hirokawa et al. showed greater rate of decline in NK cell number in aging males (Hirokawa et al., 2013). Additionally, better NK cell function was observed in healthy elderly females by Al-Attar et al. (2016). Therefore, additional studies of how NK cell function is affected after space radiation exposure in females must be considered.
Data availability statement
The original contributions presented in the study are included in the article, further inquiries can be directed to the corresponding author.
Ethics statement
The animal study was reviewed and approved by the Rutgers IACUC, Brookhaven National Laboratory IACUC.
Author contributions
CL performed flow cytometry and drafted manuscript. DH conducted NK cytoxicity assays. SD and EA planned and conducted irradiations. RH planned and analyzed NK cytotoxicity experiments, and edited manuscript.
Funding
This study was supported by NASA NNJ13ZSA002N and NIH R01 CA198073.
Acknowledgments
This work benefitted from the technical support of the NJMS Flow Cytometry and Immunology Core Laboratory and the NJMS Confocal Imaging Facility. The authors thank Drs. Jason Domogauer and Nicholas Colangelo for assisting with irradiations, and Dr. Lisamarie Moore, Dr. Jianbo Guo, and Dr. Shravanthi Chidambaram for cataloging and dissections. Also thanks to Adam Rusek’s team at the NASA NSRL.
Conflict of interest
The authors declare that the research was conducted in the absence of any commercial or financial relationships that could be construed as a potential conflict of interest.
Publisher’s note
All claims expressed in this article are solely those of the authors and do not necessarily represent those of their affiliated organizations, or those of the publisher, the editors and the reviewers. Any product that may be evaluated in this article, or claim that may be made by its manufacturer, is not guaranteed or endorsed by the publisher.
References
Abel, A. M., Yang, C., Thakar, M. S., and Malarkannan, S. (2018). Natural killer cells: Development, maturation, and clinical utilization. Front. Immunol. 9, 1869. doi:10.3389/fimmu.2018.01869
Al-Attar, A., Presnell, S. R., Peterson, C. A., Thomas, D. T., and Lutz, C. T. (2016). The effect of sex on immune cells in healthy aging: Elderly women have more robust natural killer lymphocytes than do elderly men. Mech. Ageing Dev. 156, 25–33. doi:10.1016/j.mad.2016.04.001
Beaulieu, A. M. (2021). Transcriptional and epigenetic regulation of memory NK cell responses. Immunol. Rev. 300 (1), 125–133. doi:10.1111/imr.12947
Bevelacqua, J. J., and Mortazavi, S. M. J. (2017). Commentary: Human pathophysiological adaptations to the space environment. Front. Physiol. 8, 1116. doi:10.3389/fphys.2017.01116
Bielefeldt-Ohmann, H., Genik, P. C., Fallgren, C. M., Ullrich, R. L., and Weil, M. M. (2012). Animal studies of charged particle-induced carcinogenesis. Health Phys. 103 (5), 568–576. doi:10.1097/HP.0b013e318265a257
Boerma, M., Nelson, G. A., Sridharan, V., Mao, X. W., Koturbash, I., and Hauer-Jensen, M. (2015a). Space radiation and cardiovascular disease risk. World J. Cardiol. 7 (12), 882–888. doi:10.4330/wjc.v7.i12.882
Boerma, M., Singh, P., Sridharan, V., Tripathi, P., Sharma, S., and Singh, S. P. (2015b). Effects of local heart irradiation in a glutathione S-transferase alpha 4-null mouse model. Radiat. Res. 183 (6), 610–619. doi:10.1667/RR13979.1
Buchheim, J. I., Matzel, S., Rykova, M., Vassilieva, G., Ponomarev, S., Nichiporuk, I., et al. (2019). Stress related shift toward inflammaging in cosmonauts after long-duration space flight. Front. Physiol. 10, 85. doi:10.3389/fphys.2019.00085
Buonanno, M., de Toledo, S. M., and Azzam, E. I. (2011). Increased frequency of spontaneous neoplastic transformation in progeny of bystander cells from cultures exposed to densely ionizing radiation. PLoS One 6 (6), e21540. doi:10.1371/journal.pone.0021540
Buonanno, M., De Toledo, S. M., Howell, R. W., and Azzam, E. I. (2015). Low-dose energetic protons induce adaptive and bystander effects that protect human cells against DNA damage caused by a subsequent exposure to energetic iron ions. J. Radiat. Res. 56 (3), 502–508. doi:10.1093/jrr/rrv005
Chancellor, J. C., Scott, G. B., and Sutton, J. P. (2014). Space radiation: The number one risk to astronaut health beyond low Earth orbit. Life (Basel) 4 (3), 491–510. doi:10.3390/life4030491
Chiossone, L., Dumas, P. Y., Vienne, M., and Vivier, E. (2018). Natural killer cells and other innate lymphoid cells in cancer. Nat. Rev. Immunol. 18 (11), 671–688. doi:10.1038/s41577-018-0061-z
Coca, S., Perez-Piqueras, J., Martinez, D., Colmenarejo, A., Saez, M. A., Vallejo, C., et al. (1997). The prognostic significance of intratumoral natural killer cells in patients with colorectal carcinoma. Cancer 79 (12), 2320–2328. -p. doi:10.1002/(sici)1097-0142(19970615)79:12<2320::aid-cncr5>3.0.co;2-p
Crucian, B. E., Makedonas, G., Sams, C. F., Pierson, D. L., Simpson, R., Stowe, R. P., et al. (2020). Countermeasures-based improvements in stress, immune system dysregulation and latent herpesvirus reactivation onboard the international space station - relevance for deep space missions and terrestrial medicine. Neurosci. Biobehav. Rev. 115, 68–76. doi:10.1016/j.neubiorev.2020.05.007
Crucian, B., Stowe, R., Mehta, S., Uchakin, P., Quiriarte, H., Pierson, D., et al. (2013). Immune system dysregulation occurs during short duration spaceflight on board the space shuttle. J. Clin. Immunol. 33 (2), 456–465. doi:10.1007/s10875-012-9824-7
Cucinotta, F. A., Kim, M.-H. Y., Willingham, V., and George, K. A. (2008). Physical and biological organ dosimetry analysis for international space station astronauts. Radiat. Res. 170 (1), 127–138. doi:10.1667/rr1330.1
Cucinotta, F. A., Kim, M. H. Y., and Chappell, L. J. (2012). Space radiation cancer risk projections and uncertainties. Springfield, VA: National Technical Information Service. TP-2013-0217375. doi:10.13140/RG.2.1.1413.3528
Fornalski, K. W., Adamowski, L., Dobrzynski, L., Jarmakiewicz, R., Powojska, A., and Reszczynska, J. (2022). The radiation adaptive response and priming dose influence: The quantification of the raper-yonezawa effect and its three-parameter model for postradiation DNA lesions and mutations. Radiat. Environ. Biophys. 61 (2), 221–239. doi:10.1007/s00411-022-00963-9
Garrett-Bakelman, F. E., Darshi, M., Green, S. J., Gur, R. C., Lin, L., Macias, B. R., et al. (2019). The NASA twins study: A multidimensional analysis of a year-long human spaceflight. Science 364 (6436), eaau8650. doi:10.1126/science.aau8650
Heylmann, D., Rodel, F., Kindler, T., and Kaina, B. (2014). Radiation sensitivity of human and murine peripheral blood lymphocytes, stem and progenitor cells. Biochimica Biophysica Acta - Rev. Cancer 1846 (1), 121–129. doi:10.1016/j.bbcan.2014.04.009
Hirokawa, K., Utsuyama, M., Hayashi, Y., Kitagawa, M., Makinodan, T., and Fulop, T. (2013). Slower immune system aging in women versus men in the Japanese population. Immun. Ageing 10 (1), 19. doi:10.1186/1742-4933-10-19
Huff, J., Carnell, L., Blattnig, S., Chappell, L., George, K., Lumpkins, S., et al. (2016). Evidence report: Risk of radiation carcinogenesis. Springfield, VA: National Technical Information Service. JSC-CN-35748.
Ikeda, H., and Togashi, Y. (2022). Aging, cancer, and antitumor immunity. Int. J. Clin. Oncol. 27 (2), 316–322. doi:10.1007/s10147-021-01913-z
Imai, K., Matsuyama, S., Miyake, S., Suga, K., and Nakachi, K. (2000). Natural cytotoxic activity of peripheral-blood lymphocytes and cancer incidence: An 11-year follow-up study of a general population. Lancet 356 (9244), 1795–1799. doi:10.1016/S0140-6736(00)03231-1
Ishigami, S., Natsugoe, S., Tokuda, K., Nakajo, A., Che, X., Iwashige, H., et al. (2000a). Prognostic value of intratumoral natural killer cells in gastric carcinoma. Cancer 88 (3), 577–583. doi:10.1002/(sici)1097-0142(20000201)88:3<577::aid-cncr13>3.0.co;2-v
Ishigami, S., Natsugoe, S., Tokuda, K., Nakajo, A., Xiangming, C., Iwashige, H., et al. (2000b). Clinical impact of intratumoral natural killer cell and dendritic cell infiltration in gastric cancer. Cancer Lett. 159 (1), 103–108. doi:10.1016/s0304-3835(00)00542-5
Kajioka, E. H., Andres, M. L., Li, J., Mao, X. W., Moyers, M. F., Nelson, G. A., et al. (2000). Acute effects of whole-body proton irradiation on the immune system of the mouse. Radiat. Res. 153 (5), 587–594. doi:10.1667/0033-7587(2000)153[0587:aeowbp]2.0.co;2
Karre, K., Ljunggren, H. G., Piontek, G., and Kiessling, R. (1986). Selective rejection of H-2-deficient lymphoma variants suggests alternative immune defence strategy. Nature 319 (6055), 675–678. doi:10.1038/319675a0
Kerr, R. A. (2013). Radiation will make astronauts' trip to Mars even riskier. Science 340 (6136), 1031. doi:10.1126/science.340.6136.1031
Klein, P. M., Parihar, V. K., Szabo, G. G., Zoldi, M., Angulo, M. C., Allen, B. D., et al. (2021). Detrimental impacts of mixed-ion radiation on nervous system function. Neurobiol. Dis. 151, 105252. doi:10.1016/j.nbd.2021.105252
Konstantinova, I. V., Rykova, M., Meshkov, D., Peres, C., Husson, D., and Schmitt, D. A. (1995). Natural killer cells after ALTAIR mission. Acta Astronaut. 36 (8-12), 713–718. doi:10.1016/0094-5765(95)00161-1
Lesnyak, A., Sonnenfeld, G., Avery, L., Konstantinova, I., Rykova, M., Meshkov, D., et al. (1996). Effect of SLS-2 spaceflight on immunologic parameters of rats. J. Appl. Physiol. (1985). 81 (1), 178–182. doi:10.1152/jappl.1996.81.1.178
Mace, E. M., and Orange, J. S. (2016). Genetic causes of human NK cell deficiency and their effect on NK cell subsets. Front. Immunol. 7, 545. doi:10.3389/fimmu.2016.00545
Macek Jilkova, Z., Decaens, T., Marlu, A., Marche, H., Jouvin-Marche, E., and Marche, P. N. (2017). Sex differences in spontaneous degranulation activity of intrahepatic natural killer cells during chronic hepatitis B: Association with estradiol levels. Mediat. Inflamm. 2017, 1–5. doi:10.1155/2017/3214917
Mehta, S. K., Kaur, I., Grimm, E. A., Smid, C., Feeback, D. L., and Pierson, D. L. (2001 1985). Decreased non-MHC-restricted (CD56+) killer cell cytotoxicity after spaceflight. J. Appl. Physiol. (1985). 91 (4), 1814–1818. doi:10.1152/jappl.2001.91.4.1814
Moon, W. Y., and Powis, S. J. (2019). Does natural killer cell deficiency (NKD) increase the risk of cancer? NKD may increase the risk of some virus induced cancer. Front. Immunol. 10, 1703. doi:10.3389/fimmu.2019.01703
Mortazavi, S. M., Cameron, J. R., and Niroomand-rad, A. (2003). Adaptive response studies may help choose astronauts for long-term space travel. Adv. Space Res. 31 (6), 1543–1551. doi:10.1016/s0273-1177(03)00089-9
Nakajima, T., Mizushima, N., and Kanai, K. (1987). Relationship between natural killer activity and development of hepatocellular carcinoma in patients with cirrhosis of the liver. Jpn. J. Clin. Oncol. 17 (4), 327–332.
NASA (2022). NASA space flight human-system standard: Volume 1: Crew health. National Aeronautics and Space Administration.Washington, DC, USA.
Orange, J. S. (2013). Natural killer cell deficiency. J. Allergy Clin. Immunol. 132 (3), 515–525. doi:10.1016/j.jaci.2013.07.020
Rykova, M. P., Sonnenfeld, G., Lesnyak, A. T., Taylor, G. R., Meshkov, D. O., Mandel, A. D., et al. (1992). Effect of spaceflight on natural killer cell activity. J. Appl. Physiol. (1985). 73 (2), 196S–200S. doi:10.1152/jappl.1992.73.2.S196
Shepherd, R., Cheung, A. S., Pang, K., Saffery, R., and Novakovic, B. (2020). Sexual dimorphism in innate immunity: The role of sex hormones and epigenetics. Front. Immunol. 11, 604000. doi:10.3389/fimmu.2020.604000
Villegas, F. R., Coca, S., Villarrubia, V. G., Jimenez, R., Chillon, M. J., Jareno, J., et al. (2002). Prognostic significance of tumor infiltrating natural killer cells subset CD57 in patients with squamous cell lung cancer. Lung Cancer 35 (1), 23–28. doi:10.1016/s0169-5002(01)00292-6
Vivier, E., Raulet, D. H., Moretta, A., Caligiuri, M. A., Zitvogel, L., Lanier, L. L., et al. (2011). Innate or adaptive immunity? The example of natural killer cells. Science 331 (6013), 44–49. doi:10.1126/science.1198687
Keywords: heavy ion, ionizing radiation, natural killer cells (NK cells), splenocytes, late effect of radiation
Citation: Leung CN, Howell DM, De Toledo SM, Azzam EI and Howell RW (2022) Late effects of heavy-ion space radiation on splenocyte subpopulations and NK cytotoxic function. Front. Astron. Space Sci. 9:949432. doi: 10.3389/fspas.2022.949432
Received: 20 May 2022; Accepted: 24 October 2022;
Published: 07 November 2022.
Edited by:
Cristina Consolandi, University of Hawaii at Manoa, United StatesReviewed by:
Pavel Bláha, National Institute of Nuclear Physics of Naples, ItalyS. M. J. Mortazavi, Fox Chase Cancer Center, United States
Copyright © 2022 Leung, Howell, De Toledo, Azzam and Howell. This is an open-access article distributed under the terms of the Creative Commons Attribution License (CC BY). The use, distribution or reproduction in other forums is permitted, provided the original author(s) and the copyright owner(s) are credited and that the original publication in this journal is cited, in accordance with accepted academic practice. No use, distribution or reproduction is permitted which does not comply with these terms.
*Correspondence: Roger W. Howell, cmhvd2VsbEBydXRnZXJzLmVkdQ==