- Instituto de Astronomía, UNAM, Ensenada, Mexico
The internal motions of the ionized gas in the shells of planetary nebulae (PNe) have served as relevant diagnostic tools to understand the development of this important stage of stellar evolution. This work highlights some of the kinematic studies that have impacted the most on the understanding of the structure and evolution of the ionized envelope. These studies include the basic wind-driven isotropic expansion, the occurrence of complex shell morphology and unexpected fluid dynamics, such as high-speed, bipolar, collimated outflows. The current status on the subject and possible future trends are discussed in the closing remarks.
1 Introduction
Kinematic studies have contributed to unveiling the nature and structure of planetary nebulae since the early astronomical applications with primitive spectroscopic techniques and photographic emulsions on glass plates. Since the beginning of the 20th century ground-breaking studies on this subject are found in the work of Campbell and Moore (1918), that clearly detected splitting of the most prominent emission lines in their sample of PNe. Furthermore, Zanstra (1932) demonstrated beyond any doubt that the ionized shells of planetary nebulae are expanding envelopes. Thus, it has been about a century of research on the characteristics of the emission lines from the ionized gas of the expanding envelopes of PNe. The detail and relevance on the physical information the emission lines convey has evolved hand in hand with the advances of more refined spectroscopic techniques. These modern techniques now provide simultaneous, high spatial and spectral resolution and improved sensitivity with solid state detector technology over a wide wavelength range. This work does not attempt to review all the advances in the field that have occurred and have contributed to build our current understanding of the PN phenomenon. For a broad review on the subject the reader is referred to the recent review by Kwitter and Henry (2022) and references therein. This contribution concentrates on reviewing some of the studies on PNe that, through the kinematic analysis of the internal motions in the ionized envelopes have greatly impacted our current understanding of their structure and evolution. In §2 a description of the basic concept on how a planetary nebula forms is presented. In §2.1 we discuss the Wilson diagram from a modern perspective in relation to the ADF or abundance discrepancy factor controversy. In §2.2 the success of the interacting winds model and the discovery of non-isotropic expansion and supersonic, bipolar outflows are discussed. In addition, some of the representative pioneer works that discovered the high-speed collimated outflows and complex shell morphology in PNe are presented. The main competing theories on the PN development, namely short-period binaries and magnetic fields, are presented in §2.3. Complex morphologies, kinematic patterns and consequences of projection effects are discussed in §2.4 together with morpho-kinematic tools that allow to disentangle the intricate 2D shapes projected on the sky and deduce 3D representations of the nebular structures. In §2.5 the Eskimo nebula, NGC 2392 is used as a case example of a nebulae with a rather complex morphology and kinematics and whose true structure is finally revealed by morpho-kinematic reconstruction and integral field spectroscopy. The use of PNe as probes of the galactic rotation curve is described in §2.6 and the PNe bulk expansion trends as a function of central star mass and evolutionary stage are presented in §2.7. Finally in §3 we provide some final remarks.
2 Internal Motions in Planetary Nebulae
Planetary Nebulae represent an important late stage of stellar evolution for stars of ∼ 1–8 M⊙. The star sheds most of its outer layers during the Asymptotic Giant Branch (AGB) through a dense and slow outflow. This material is chemically enriched and pollutes the immediate surroundings. Since these low and intermediate mass stars are common they can impact on the chemical evolution of the galaxy. After the star leaves the AGB stage a nearly bare stellar core heats up quickly and produces a fast and tenuous wind that will catch up with the slowly expanding envelope from the AGB. The fast wind - slow wind collision produces a reverse shock that creates an inner hot bubble, a relatively cool shell that forms the PN proper and becomes ionized by the UV stellar photons and an outer shock. This expanding structure presumably forms through isotropic mass-loss driving a close to a spherically-symmetric envelope. Eventually, after only a few thousand years, depending on the remaining stellar mass, the star’s luminosity will start fading rapidly, the ionized nebular structure would start to recombine and dilute and the PN stage would come to an end, leaving a slowly cooling white dwarf behind. This was the base concept of a planetary nebula in the decade of the 1970s.
2.1 Envelope Kinematics and the ADF Discrepancy
Wilson (1950) discovered a significant correlation among the ions of different levels of excitation with their radial velocities, essentially identifying the nowadays well known stratification of atomic species within the nebular plasma. This correlation is known as the Wilson Diagram. This is an early key element of information that in parallel with advances in atomic and photo-ionization physics eventually lead to the current models (e.g. CLOUDY, Ferland et al., 2013) that describe the fractional ionization in the expanding plasma as a function of distance from the stellar ionizing source. From these models emission line intensities can be converted into physical parameters such as electron temperature and densities that are required for the derivation of ionic abundances. However, one of the main, still unresolved conundrums in deriving ionic and total abundances from the expanding envelopes of PNe is the large abundance discrepancy factor (ADF) or forbidden vs. permitted discrepancy found in these objects. The derived ionic abundances from the emission line intensities are sensitive to the electron temperature and it has been known for more than half a century (Peimbert, 1967) that the temperatures derived from forbidden, collisionally excited lines are systematically larger than those derived from the permitted, recombination lines. Peimbert (1971) suggested the presence of temperature fluctuations within the plasma, however in some instances the fluctuations are of such a large amplitude that cannot be explained by standard photo-ionization models. For this reason Liu (2000) suggested the presence of a second plasma component consisting of hydrogen deficient, cold, clumps embedded in the plasma. Other alternatives have been explored such as enhanced electronic recombination (Garnett and Dinerstein 2001) but again they are unable to account for large ADFs (Wesson et al., 2018). Back to the Wilson diagram Richer et al. (2013), Richer et al. (2017), and Peña et al. (2017) have investigated the problem by analyzing the detailed kinematics of the emission lines excited by recombination and collision processes in several PNe. For their work they obtained spatially and velocity resolved echelle spectroscopy with the Very Large Telescope UT2 and the Magellan telescope, respectively. In their kinematic analysis they study the radial velocities of the emission lines that are supposed to originate from similar volumes in the envelope and therefore are expected to have similar velocities. A suitable ionization structure is considered for each particular case. However, they find clearly discrepant kinematics between the recombination and forbidden lines from the same parent ion. This indicates that there is an additional plasma component arising from a different volume, assuming ionization equilibrium. Thus temperature differences would seem to originate in a chemically-inohomogeneous medium with two plasma components where one of them emits collisonally excited lines and the other does not. Nevertheless, PNe with large ≳ 10 ADFs imply local temperature fluctuations whose origin is difficult to envisage. Bautista and Ahmed (2018) have modeled large temperature fluctuations that seem to propagate efficiently in the envelopes of PNe with short periods binary cores, as those expected from post common envelope systems where the period is shorter than the thermalization time in the plasma. Given the apparent close relationship between PNe with close binary cores (see §2.3) and large ADFs (Wesson et al., 2018) this is a very promising path to keep investigating. The problem is still an open one but now velocity shifts, line widths and line ratios of the discrepant emission lines open a new avenue to be used as firm diagnostics for new models.
2.2 Non-spherical Expansion, Axial and Point Symmetries and Supersonic Motions
The early observations of outflow motions and basic spherical shape of the shells originating in AGB stars led Kwok et al. (1978) and Kwok (1992) to propose the interacting winds model. This model predicts extended X-Ray emission from a hot bubble produced by the inner shock of the fast stellar wind that catches up with the slow moving mass component lost during the AGB phase (e.g. Kastner et al., 2012; Toalá and Arthur 2016). The interacting winds model provided the first analytical explanation of the PN structure and evolution of the ionized shell expanding at velocities in the order of 20–30 kms−1. However, it had already become clear that the morphology of PNe included other shapes and structures such as elliptical and bipolar with diverse eccentricities and in some cases fast axial outflows (e.g. López and Meaburn 1983). A generalized interacting winds model was then proposed by Kahn and West (1985) where the presence of an equatorial density enhancement would dictate the final shape outcome. On this basis morphological classifications (Balick 1987) were proposed and 1D and 2D numerical codes including radiation hydrodynamics were explored (e.g. Marten and Schöenberner 1991; Frank and Mellema 1994; Perinotto et al., 1994). An unexpected and surprising new phenomenon in PNe was the discovery by Gieseking et al. (1985) of the first high-speed, bipolar jet in the planetary nebula NGC 2392. A highly collimated, supersonic (±200 kms−1) narrow structure. This finding was surprising since jets were not supposed to be part of planetary nebulae. The Hubble Space Telescope had not been launched yet and the field was still relaying on seeing limited, ground-based images. In spite of this limitation it had already become apparent that planetary nebulae had much more complex structures than originally realized or envisaged (e.g. López et al., 1988). Bipolar structures were being found at infrared wavelengths since the earliest stages of the PN development, referred as the proto-PN stage (e.g. Kwok, 1993; Kwok et al., 2000). Balick et al. (1993) reported supersonic low-ionization emission regions in the form of bullets in mainly elliptical PNe. These fast outflowing regions, dubbed FLIERS, were symmetric with respect to the central star along the main axis. In the same year López et al. (1993) presented evidence of the first bipolar, rotating, episodic jet in the planetary nebula Fleming 1. This finding would be followed by the serendipitous discovery of the BRET (bipolar, rotating, episodic, jet) in the stunning object KjPn 8 (López et al., 1995; López et al., 1997). Hypersonic, bipolar outflows were discovered in He 3–1475 by Bobrowsky et al. (1995) and in MyCn 18 by Bryce et al. (1997) with outflowing projected speeds in excess of ±500 kms−1 and with velocities increasing with distance from the source, an effect commonly observed in the ballistic outflows of planetary nebulae. The Hubble Space Telescope had finally become fully operational by the mid 1990s and one of the areas immediately impacted by the fresh diffraction-limited images provided by this telescope were planetary nebulae. Morphologies with multiple collimated outflows and peripheral knots (e.g. Corradi et al., 1997; Corradi et al., 1999), point-symmetric structures (e.g. Guerrero et al., 1999; Miranda et al., 2006; López et al., 2012a) and quadrupolar lobes (Manchado et al., 1996) were soon adding to the extraordinary shapes and structures that were being unveiled by their complex morphology but particularly by their extraordinary kinematic footprint. The pervasive presence of highly collimated outflows in PNe had become apparent in most HST imagery of PNe and their supersonic speeds were routinely confirmed by spectroscopic means, as described in the examples quoted above. Guerrero et al. (2013) unveiled the presence of thin interfaces related to bow shocks from the collimated jet-like outflows and shocks at the border of the expanding nebular shells from [O III]/Hα HST image ratios of a sample of PNe. The kinematic confirmation is always essential to prevent misidentification of structural components in the nebular shell and to quantify the energies involved in these jets. Thus, the significance of jets in the formation and evolution process of PNe became a focus of attention, suggesting that jets were actually responsible for shaping the complex PN structures (Sahai and Trauger 1998) since the early stages of formation. However the reasons for the occurrence of these fast, collimated outflows were still far from clear. A good summary of the knowledge and understanding of the PNe phenomenon up to this point is found in Balick and Frank (2002).
2.3 Binarity and Magnetic Fields
In the last decades, the likely binary nature of the central stars of PNe has been under intense investigation since it could provide explanations to many of the yet unresolved problems present in the topics mentioned above. In analogy with other astronomical objects that are known to host bipolar jets, such as active galactic nuclei, Herbig-Haro outflows from young stellar objects and X-ray binaries, the unexpected presence of jets in PNe was soon theorized to involve an accretion disk. This disk would be part of the mechanism to launch the bipolar jets and it would be produced in this case as a result of a common envelope episode in a close binary system (Soker and Livio 1994; Soker 2006; Akashi and Soker 2008). It must be underlined that at the time planetary nebulae were understood as an evolutionary stage produced by single stars. Considering binary cores in PNe signifies a game-changer in the field with deep consequences in many areas; it is a new paradigm for the PN phenomenon. However producing the necessary accretion disks as a result of a common envelope episode necessary to launch jets faced some severe obstacles (e.g. Reyes-Ruiz and López 1999; De Marco 2009). On the observational side close binary stellar or sub-stellar companions are difficult to detect by spectroscopic means considering the short orbital periods involved, corresponding to separations of only a few stellar radii and systems immersed within the bright nebular glow of the ionized envelope. However photometric methods have proved successful in confirming companions in a number of PNe. Since binaries had been brought in, at least initially to explain the intricate morphologies and bipolar jets in PNe, it was naturally expected that the first close binary cores discovered in PNe would correspond to the kind of complex PNe with fast bipolar jets. Contrary to these expectations, the initial small sample of these PNe, amounting to a couple of dozen of objects (Miszalski et al., 2009) revealed diverse morphologies, mainly dominated by equatorial rings or density enhancements and in many cases showing ordinary expansion velocities (López et al., 2011). However, recently Zou et al. (2020) have produced 3D numerical simulations showing how, under the right conditions, the results of common envelope ejecta produce high pole-to equator density contrasts. The resulting equatorial density enhancements in their models are able to focus and drive bipolar lobes and even jet-like bipolar outflows from an originally isotropic stellar wind. The number of confirmed close binary systems found in PNe has been steadily increasing, it amounts now to about 60 systems. This enlarged sample continues to show a wide range of observed morphologies and particularly diverse kinematic structures. Binary-induced outflows models in PNe have awakened an active area of research in the field (e.g. Nordhaus and Blackman 2006; Muhammad and Soker 2008; García-Segura et al., 2021). It most be noted, however, that several problems in the common envelope theory remain still to be solved such as a reliable calculation of the efficiency to eject the envelope during the last stages of the process and the exact conditions under which a pair can actually reach a stable mass-transfer stage to produce a relevant accretion disk, though the latter may not be needed after all, according to Zou et al. (2020). These are vital elements of the theory regarding the possible shaping effects of the nebular envelope. Nevertheless, the relevance of close binary systems in the formation and evolution of PN has been now well established and much work will continue to be done in this context. A well informed and comprehensive review on this subject is found in Boffin and Jones (2019).
Magneto-hydrodynamic models involving rotating single stars with MHD stellar wind - disk wind interplay (Blackman et al., 2001) and toroidal stellar winds (García-Segura et al., 2005) became also of interest as a natural alternative to the binary hypothesis. These models have encountered difficulties in recent times to probe that a post-AGB single stellar core will rotate sufficiently fast to produce a noticeable dynamo effect or MHD toroidal winds. Nevertheless, their presence may still be required to aid in launching and collimating the jet-like outflows. Magnetic fields are difficult to detect in PNe. However, Sabin et al. (2007) report firm detection of magnetic fields with a toroidal distribution along the equatorial density enhancement in four PNe and two more with a less defined distribution. These observations were done in the sub-millimeter spectral region. Additional surveys in search of magnetic winds are required. In brief, the two main current avenues of research, binaries and MHD models, still have both important elements to resolve. It is expected, perhaps somehow optimistically, that the stellar evolution stage of PNe may get a unified view in a not too distant future.
2.4 Complex Morphologies and Projection Effects
As discussed in the previous section, the original concept of a PN as a spherically symmetric, evenly expanding shell of ionized matter took a colossal leap in the last decades. Describing the dynamical evolution of PNe becomes more and more cumbersome. We need now to explain not only the PN stage within the framework of stellar evolution (e.g. Miller Bertolami 2016) from stellar structure and nucleosynthesis calculations coupled to radiation hydrodynamics for the envelope, but in addition include common-envelope, close binary theory and MHD winds. These are all required elements in trying to explain the origin of PNe (as single or binary cores) and the increasing numbers of new structural features, such as the unexpected discovery of high-speed, collimated outflows, jets and lumps, bipolar, poly-polar and point-symmetric (twisted) morphologies with low-ionization knots or condensations and filaments distributed over the periphery of the ionized shell. The advent of the HST revealed what seemed an incredible heterogeneity and intricacy of forms and shapes of the ionized nebular shells. This seeming richness in diversity of shapes and forms soon led to suspicions and to investigate the possible misleading multiplicative geometric effects of projections of the same PN on the sky after rotation and inclination transformations of a few basic structures. Chong et al. (2012) showed that a model of multipolar PNe could explain a number of morphological structures combining the inclination with respect to the plane on the sky, the position angle of the lobes and the sensitivity or dynamic range of the observations. In this way geometric transmutation of a bipolar model nebula could recover diverse structural components thought as representing different classes. Furthermore, the myriad of morphologies observed were, surprisingly, not usually reflected in their kinematic patterns, as can be analysed from the San Pedro Mártir kinematic catalogue of galactic planetary nebulae (López et al., 2012b). This catalogue contains nearly four thousand long-slit, echelle spectra for about eight hundred PNe distributed over the disk, the halo and the galactic bulge. It is the largest of its kind. The position-velocity representations of the bi-dimensional, long-slit line profiles in this sample can be sorted out into groups. These groups reflect fairly closely the main morphological classes and secondary outflow structures observed in narrow-band imagery of PNe, though with less apparent diversity within the spectral groups. A way to reveal the 3D structure of a PN using kinematics as a tool is by combining its 2D projection on the sky with the radial velocity of a sufficiently large number of points over the image since the radial velocity vector essentially acts as the third spatial dimension perpendicular to the line of sight. Sabbadin et al. (2006) applied a tomographic method to recover the 3D density structure of a group of PNe from long-slit spectra. An expansion velocity law needs to be considered, being an homologous expansion the most common. The basic principle is this, think of an expanding soap bubble, in projection it looks simply as a circle but if one knows the expansion pattern, largest at the center and diminishing towards the poles, then a clear 3D pictures of the bubble emerges. The bubble expansion behaviour produces a velocity ellipsoid in the long slit line profile. Steffen and López (2006) (and see also Steffen et al., 2011) applied this principle to derive 3D morpho-kinematic models of PNe by combining the long-slit kinematic information with the 2D image. A neat example of this procedure is found in García-Díaz et al. (2012) where the highly complex form of the Eskimo nebula, NGC 2392, is disentangled via a thorough long-slit mapping, producing a reliable 3D representation of the object. The inner bubble, caps, filaments and bipolar jet are all clearly exposed in the analysis of the data and model. The results of this work also show how seemingly very different objects, such as NGC 2392, NGC 6543 and NGC 7009 are actually part of the same structural family only viewed at different angles, confirming the suspicions that morphological diversity is contaminated and artificially enhanced by some misleading 2D projection on the sky of what are truly 3D object (see Figure 3). Nevertheless, complexity is real in PNe, a recent extraordinary example is the analysis of NGC 6210, the “Turtle” nebula, where five axes of symmetry have been needed to explain its morpho-kinematic structure (Henney et al., 2021).
2.5 The Eskimo Nebula, a Case Example
Modern science advancement is highly technology dependent and astronomy is no exception. However, technology does not interpret data, though it makes it easier to acquire it and display it. Science needs the human wit for discovery. The original coudé spectrum taken by Gieseking et al. (1985) on unbaked IIa-O photographic emulsion revealed for the first time the presence of a highly collimated, bipolar outflow in a planetary nebula (see Figure 1). This was correctly described as a “jetlike multipolar mass ouflow with a velocity of nearly 200 kms−1 and a collimation less than 10°” with episodic activity. The orientation of the jet was also correctly deduced from their limited observations. This work opened the door to search for more bipolar jet-like outflows in PNe. As mentioned before, 27 years later, García-Díaz at al (2012) made a thorough long-slit mapping of the nebula with an efficient, single order echelle, nebular spectrometer (MES) and a modern CCD detector. Their kinematic results identified all the relevant structural features of the Eskimo, including the spatial distribution and extent of the bipolar jet (see Figures 2, 3). The full set of bi-dimensional arrays covering the entire face of the Eskimo can be consulted in the SPM kinematic catalogue of galactic PNe (López et al., 2012a). Nine years later, Guerrero et al. (2021) with the use of an integral field spectrometer (MEGARA) and a large telescope (GTC) produced the first ever image of the bipolar jet in the light of several emission lines superposed over the face of the Eskimo (see Figure 4). This image is derived from kinematic information that indicates the approaching and receding sides of the jet. The power of the technique and level of detail extracted from the data is remarkable. Thus, 36 years after its discovery we now have a clear picture of the kinematic structure of the jet in the Eskimo nebula, though we still do not fully understand the engine that drives it. Figures 1–4 provide a neat historic perspective of the increasing understanding of this iconic PN through constantly improving instrumentation techniques.
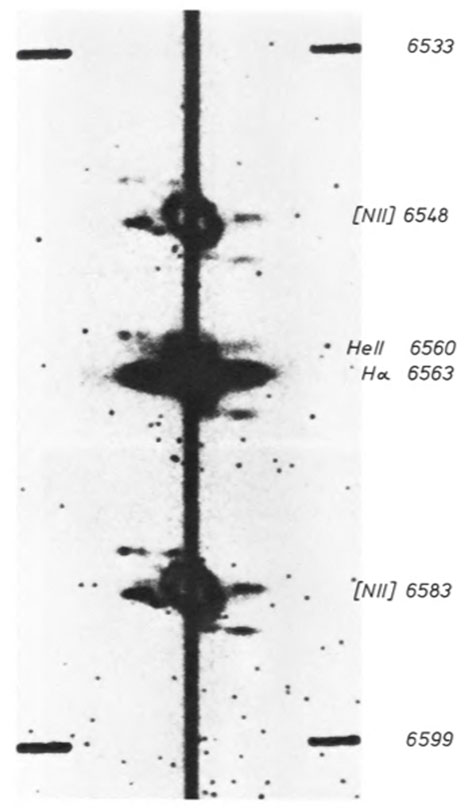
FIGURE 1. The long-slit coudé spectrum showing the discovery of the first jet in a planetary nebula. From Gieseking, Becker and Solf, 1985, ApJ 295, L17. ⓒ AAS. Reproduced with permission.
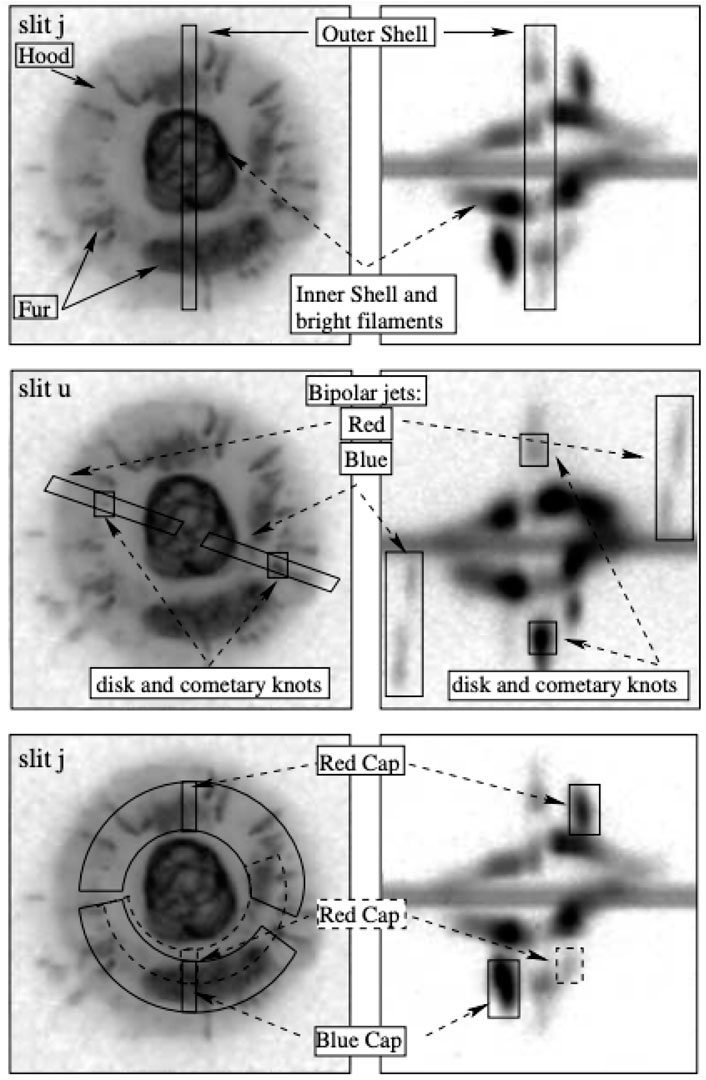
FIGURE 2. Selected position-velocity arrays from long-slit spectra, aligned across the core and the bipolar jet are shown against an HST image of NGC 2392 where the most prominent features are identified in both the images and the spectra. From García-Díaz et al., 2012, ApJ 761, 172. The full set of long-slit spectra for the Eskimo can be consulted in the SPM kinematic catalogue of galactic PNe (López et al., 2012a) ⓒ AAS. Reproduced with permission.
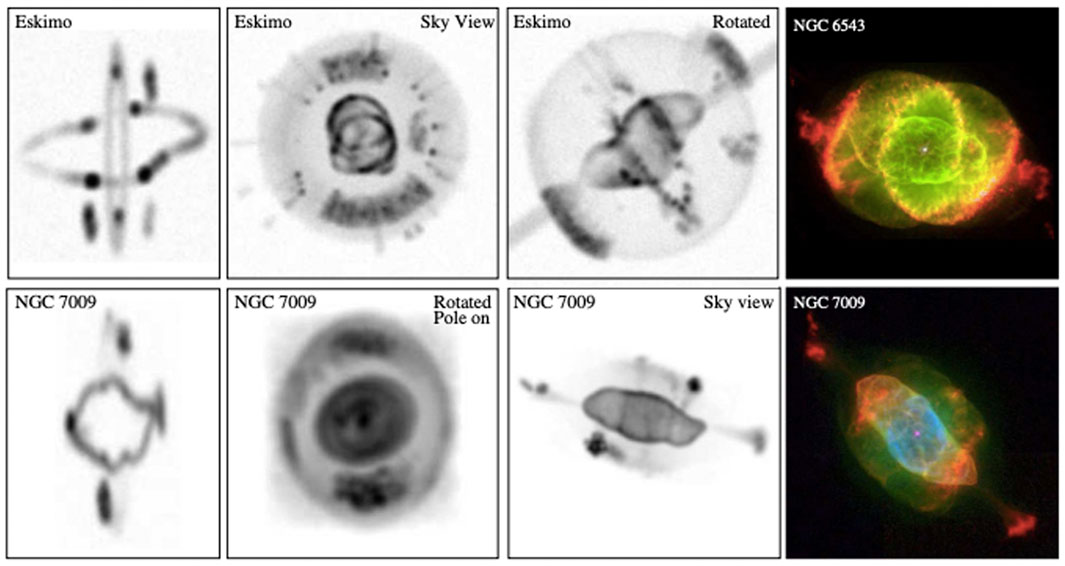
FIGURE 3. Upper row, left, a synthetic P-V diagram from the center of the Eskimo (compare with the top panel in Figure 2); next, the Eskimo image derived from the SHAPE morpho-kinematic model as seen on the sky; third panel, the same image but now the SHAPE model is rotated 100°; far right an HST image of NGC 6543, notice the close similarity of this nebula with the rotated model version of the Eskimo. Lower row, left, a SHAPE synthetic P-V diagram of NGC 7009 as it would look if obtained from a slit located at the center on a pole-on orientation, as shown in the SHAPE model image in the next panel; third bottom panel the SHAPE model image of NGC 7009 as seen on the sky and finally an HST image of this nebula. Notice the coincidences between the images in the upper and lower panels when rotations and orientations are taken into account. From García-Díaz et al., 2012, ApJ 761, 172. ⓒ AAS. Reproduced with permission.
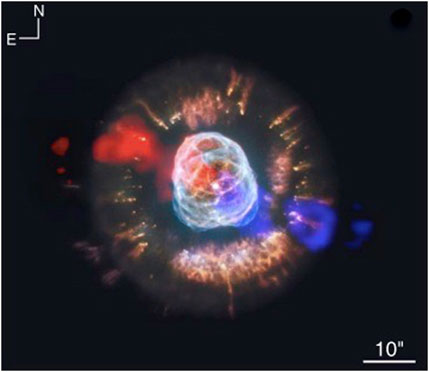
FIGURE 4. The approaching (blue) and receding (red) components of the bipolar jet in the Eskimo are superposed on an HST image. The spectral information covers the velocity range +190 to −190 km s−1 in the light of [N II] λ 6584 and obtained with an integral field spectrometer. From Guerrero et al., 2021, ApJ 909, 44. ⓒ AAS. Reproduced with permission.
2.6 PNe and the Galactic Rotation Curve
Although galactic structure is not directly related to the main subject of internal motions in PNe discussed here, the contribution of measuring the global radial velocity of the PNe population from their bright emission lines in the galactic context, i.e. reading the magnitude and direction of the radial velocity vector related to their position in the galaxy, merits a brief mention in this discussion on kinematics of galactic PNe. Planetary nebulae are very useful probes of galactic structure, they are distributed all over our galaxy, the disk, the bulge and the halo and are relatively easy to observe thanks to their bright emission lines. Schneider et al. (1983a) compiled a catalogue of radial velocities for the 524 planetary nebulae known at the time. Schneider and Terzian (1983b) then used this catalogue to probe the galactic rotation curve out to 15 kpc from the galactic center, and along the process calibrate an existing distance scale. Durand et al. (1998) repeated this exercise for 867 PNe with higher resolution data and with this new sample they calculated more accurate kinematic parameters of the galactic differential rotation curve for the disk, whereas Zijlstra et al. (1997), using a sample of 71 PNe towards the galactic center examined the rotation of the galactic bulge. Extragalactic PNe have also been observed in nearby galaxies of the local group and their individual bulk expansion and global radial velocities measured (e.g. Richer et al. (2010b).
2.7 The PNe Mean Expansion Trends
In spite of the various intricate elements that need to be considered when analysing in detail the internal kinematic structure of PNe, as discussed previously, the line profiles from the main bright shell, the region with the largest emission measure, can be used to derive a global, bulk expansion velocity of the PN. This region, also known as the rim is formed by the compression of the shocked stellar wind onto the slowly moving outer envelope. An inner shock develops between the free-flowing wind region and the shocked wind. The compressed matter is ionized and sets an outward ionization front into the still neutral material from the AGB wind and an outer shock front develops. Jacob et al. (2013) and Schönberner et al., 2018, have shown that the outer shock provides a better representation of the PN expansion velocity, although this region cannot be measured directly. However their 1D hydro models indicate that the measured bulk expansion velocities need to be multiplied by factors of approximately 1.3–1.5 to obtain the true outer shock velocity. Hydrodynamic models predict that the expansion pattern of the PN shell is influenced by the evolution of the central star. It is then of interest to compare the bulk expansion patterns for different evolutionary stages and galactic populations of PNe. In a string of papers (Richer et al., 2008; Richer et al., 2010a; Pereyra, Richer and López 2013; Pereyra, López and Richer 2016) the Ensenada group took advantage of their kinematic catalogue of galactic PNe to measure bulk expansion velocities and carry out statistically significant studies with homogeneous sets of data on the kinematics of several groups of PNe. They analysed the populations in the bulge, disk and halo, including in these groups stages of early, evolved, mature, and PNe with low-mass central stars. The result of these works are summarized in López et al. (2016) and essentially show for the first time from observations how the kinematic evolution of the ionized shell correlates closely with the central star mass and its evolutionary stage during this last phase of stellar evolution (see Figure 5).
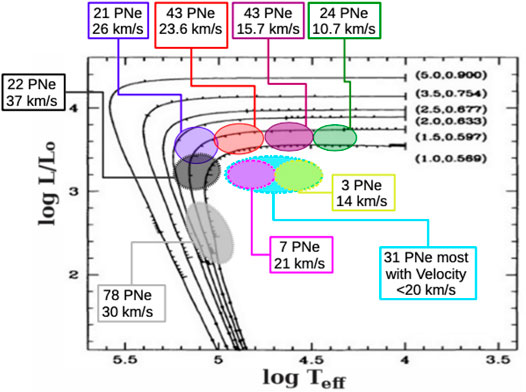
FIGURE 5. Mean bulk expansion velocities are indicated for several groups of PNe from the disk, bulge and halo covering diverse evolutionary stages and core masses. Their approximate locations on the H-R diagram reveal their kinematic evolution. From López et al., 2016 Journal of Physics Conf Ser 728032002. Originally published under a CC BY license.
3 Discussion
Planetary Nebulae are ideal astrophysical laboratories. Physicists and astronomers have used them for the past century to test models on stellar structure, nucleo-synthesis, stellar, galactic and chemical evolution, photo-ionization and hydrodynamics, supersonic flows and shocks, MHD winds, common-envelope evolution in close binary systems and galactic structure. In particular, kinematic studies of the ionized shell play a vital role in deciphering many of the questions related to the origin, structure and evolution of the PN envelope. In relation to the future, for the internal kinematics of the ionized gas in PNe it can be envisaged that the research in the field will pursue the following two main paths. On the observational side integral field spectrometers are powerful instruments that deliver simultaneous spatial and spectral information at selectable wavelengths. They deliver data cubes (2D spatial 1D spectral) that provide a very large amount of information in a single exposure. They are now available in the optical and infrared domains in all major telescopes in the world and they will become the preferred tools for PN research in the coming years. On the theoretical side, the exact physical mechanism to launch and collimate high-speed, bipolar outflows or jets from the core of a PN is the next great challenge. Close binary systems seem to be currently the most promising avenue of research and this is being pursued vigorously to resolve still important gaps in the theory and models. We will need to answer in the coming years if PNe as representatives of a late stage of evolution can be driven by single and/or binary cores and what are the physical mechanisms that rule the evolution of their ionized shells in each case.
Author Contributions
The author confirms being the sole contributor of this work and has approved it for publication.
Funding
JAL gratefully acknowledges financial support from Universidad Nacional Autónoma de México, UNAM, and CONACYT through projects DGAPA-PAPIIT IN103519 and CONACYT A1‐S‐15140.
Conflict of Interest
The author declares that the research was conducted in the absence of any commercial or financial relationships that could be construed as a potential conflict of interest.
Publisher’s Note
All claims expressed in this article are solely those of the authors and do not necessarily represent those of their affiliated organizations, or those of the publisher, the editors and the reviewers. Any product that may be evaluated in this article, or claim that may be made by its manufacturer, is not guaranteed or endorsed by the publisher.
Acknowledgments
JAL is indebted to his colleagues Michael G. Richer, Ma. Teresa García-Díaz and John Meaburn for generously sharing many discussions on the subjects of this review.
References
Akashi, M., and Soker, N. (2008). Shaping Planetary Nebulae by Light Jets. MNRAS 391, 1063–1074. doi:10.1111/j.1365-2966.2008.13935.x
Balick, B., and Frank, A. (2002). Shapes and Shaping of Planetary Nebulae. Annu. Rev. Astron. Astrophys. 40, 439–486. doi:10.1146/annurev.astro.40.060401.093849
Balick, B., Rugers, M., Terzian, Y., and Chengalur, J. N. (1993). Fast, Low-Ionization Emission Regions and Other Microstructures in Planetary Nebulae. Astrophysical J. 411, 778. doi:10.1086/172881
Balick, B. (1987). The Evolution of Planetary Nebulae. I - Structures, Ionizations, and Morphological Sequences. Astrophysical J. 94, 671. doi:10.1086/114504
Bautista, M. A., and Ahmed, E. E. (2018). Resonant Temperature Fluctuations in Nebulae Ionized by Short-Period Binary Stars. Astrophysical J. 866, 43. doi:10.3847/1538-4357/aad95a
Blackman, E. G., Frank, A., and Welch, C. (2001). Magnetohydrodynamic Stellar and Disk Winds: Application to Planetary Nebulae. Astrophysical J. 546, 288–298. doi:10.1086/318253
Bobrowsky, M., Zijlstra, A. A., Grebel, E. K., Tinney, C. G., te Lintel Hekkert, P., van de Steene, G. C., et al. (1995). He 3-1475 and its Jets. Astrophysical J. 446, L89. doi:10.1086/187937
Boffin, H. M. J., and Jones, D. (2019). The Importance of Binaries in the Formation and Evolution of Planetary Nebulae. Spring Briefs Astronomy. doi:10.1007/978-3-030-25059-1
Bryce, M., López, J. A., Holloway, A. J., and Meaburn, J. (1997). A Bipolar Knotty Outflow with Velocities of (±500 Kms−1) or above from the Engraved Hourglass Planetary Nebula MyCn 18. Astrophysical J. 487, 161. doi:10.1086/310904
Campbell, W. W., and Moore, J. H. (1918). The Spectrographic Velocities of the Brightline Nebulae. Publ. Lick Observatory 13, 77.
Chong, S.-N., Kwok, S., Imai, H., Tafoya, D., and Chibueze, J. (2012). Multipolar Planetary Nebulae: Not as Geometrically Diversified as Thought. Astrophysical J. 760, 115. doi:10.1088/0004-637x/760/2/115
Corradi, R. L. M., GUerrero, M., Manchado, A., and Mampaso, A. (1997). Multiple Collimated Outflows in a Planetary Nebula? New Astron. 2, 461–470. doi:10.1016/s1384-1076(97)00032-8
Corradi, R. L. M., Perinotto, M., Villaver, E., Mampaso, A., and Goncalves, D. R. (1999). Jets, Knots, and Tails in Planetary Nebulae: NGC 3918, K1‐2, and Wray 17‐1. Astrophysical J. 523, 721–733. doi:10.1086/307768
De Marco, O. (2009). The Origin and Shaping of Planetary Nebulae: Putting the Binary Hypothesis to the Test. Publ. Astron Soc. Pac 121, 316–342. doi:10.1086/597765
Durand, S., Acker, A., and Zijlstra, A. (1998). The Kinematics of 867 Galactic Planetary Nebulae. Astron. Astrophys. Suppl. Ser. 132, 13–20. doi:10.1051/aas:1998356
Ferland, G. J., Porter, R. L., van Hoof, P. A. M., Williams, R. J. R., Abel, N. P., Lykins, M. L., et al. (2013). The 2013 Release of Cloudy. RMxAA 49, 137.
Frank, A., and Mellema, G. (1994). A Radiation-Gasdynamical Method for Numerical Simulations of Ionized Nebulae: Radiation-Gasdynamic of PNe I. Astronomy Astrophysics 289, 937.
García-Segura, G., López, J. A., and Franco, J. (2005). Magnetically Driven Winds from Post-Asymptotic Giant Branch Stars: Solutions for High-Speed Winds and Extreme Collimation. Astrophysical J. 618, 919.
García-Segura, G., Taam, R. E., and Ricker, P. M. (2021). Common Envelope Shaping of Planetary Nebulae III. The Launching of Jets in Proto-Planetary Nebulae. Astrophysical J. 914, 111.
Garnett, D. R., and Dinerstein, H. L. (2001). The OII Recombination Line Abundance Problem in Planetary Nebulae. RMxAC 10, 13G.
Gieseking, F., Becker, I., and Solf, J. (1985). High Velocity Bipolar Mass Flow in the Planetary Nebula NGC 2392. Astrophysical J. 295, 17. doi:10.1086/184529
Guerreo, M. A., Toalá, J. A., Medina, J. J., Luridiana, V., Miranda, L. F., Riera, A., et al. (2013). Unveiling Schocks in Planetary Nebulae. Astronomy Astrophysics 557, A121.
Guerrero, M. A., Cazzoli, S., Rechy-García, J. S., Ramos-Larios, G., Montoro-Molina, B., Gómez-González, V. M. A., et al. (2021). Tomography of the Unique Ongoing Jet in the Planetary Nebula NGC 2392. Astrophysical J. 909, 44. doi:10.3847/1538-4357/abe2aa
Guerrero, M. A., Vázquez, R., and López, J. A. (1999). The Kinematics of Point-Symmetric Planetary Nebulae. Astrophysical J. 117, 967–973. doi:10.1086/300729
Henney, W. J., López, J. A., García-Díaz, M. T., Richer, M. G., and Richer, M. G. (2021). The Five Axes of the Turtle: Symmetry and Asymmetry in NGC 6210. MNRAS 502, 1070–1094. doi:10.1093/mnras/staa4014
Jacob, R., Schönberner, D., and Steffen, M. (2013). The Evolution of Planetary Nebulae VII. True Expansion Rates and Visibility Times. Astronomy Astrophysics 558, 78. doi:10.1051/0004-6361/201321532
Kahn, F. D., and West, K. A. (1985). Shapes of Planetary Nebulae. Mon. Notices R. Astronomical Soc. 212, 837–850. doi:10.1093/mnras/212.4.837
Kastner, J. H., Montez, R., Balick, B., Frew, D. J., Miszalski, B., Sahai, R., et al. (2012). The Chandra X-Ray Survey of Planetary Nebulae (ChanPlaNS): Probing Binarity, Magnetic Fields, and Wind Collisions. Astronomical J. 144, 58. doi:10.1088/0004-6256/144/2/58
Kwitter, K. B., and Henry, R. B. C. (2022). Planetary Nebulae: Sources of Enlightenment. PASP 134, 022001. doi:10.1088/1538-3873/ac32b1
Kwok, S., Hrivnak, B., and SuKate, Y. L. (2000). Discovery of a Disk Collimated Bipolar Ouflow in the Proto-Planetary Nebula IRAS 17106-3046. Astrophysical J. 544, 149. doi:10.1086/317304
Kwok, S. (1993). Proto-Planetary Nebulae. Annu. Rev. Astron. Astrophys. 31, 63–92. doi:10.1146/annurev.aa.31.090193.000431
Kwok, S., Purton, C. R., and Fitzgerald, P. M. (1978). On the Origin of Planetary Nebulae. Astrophysical J. 219, L125. doi:10.1086/182621
López, G. D., Richer, M. G., and lloyd, M. (2011). “The Kinematics and Morphology of Planetary Nebulae with Close Binary Systems,” in Asymmetric Planteary Nebuale V, Conference Held in Bowness-On-Wildermere, UK. Editors A. A. Ziljstra, F. Lykou, and E. Lagadec. 2011apn.confE.335L.
López, J. A., García-Díaz, Ma. T., Steffen, W., Riesgo, H., and Richer, M. G. (2012b). Morpho-Kinematic Analysis of the Point-Symmetric, Bipolar Nebulae Hb 5 and K 3-17: A Pathway to Poly-Polarity. Astrophysical J. 750, 131.
López, J. A., Meaburn, J., Bryce, M., and Holloway, A. J. (1988). The Morphology and Kinematics of the Complex Poly-Polar Nebula NGC 2440. Astrophysical J. 493, 803.
López, J. A., Meaburn, J., and Bryce, M. (1997). The Kinematics of the Extensive Bipolar Lobes and Symmetric Lobes of the Planetary Nebula KjPn 8 (PK 1123-00 1). Astrophysical J. 475, 705.
López, J. A., Meaburn, J., and Palmer, J. W. (1993). Kinematical Evidence of a Rotating, Episodic Jet in the Planetary Nebula Fg 1. Astrophysical J. 415, 135L.
López, J. A., and Meaburn, J. (1983). The Structure and Dynamics of the Bi-polar Nebula Mz-3. MNRAS 204, 203.
López, J. A., Richer, M. G., García-Díaz, M. T., Clark, D. M., Meaburn, J., Riesgo, H., et al. (2012a). The San Pedro Mártir Kinematic Catalogue of Galactic Planetary Nebulae. RMxAA 48, 3.
López, J. A., Richer, M. G., Pereyra, M., and García-Díaz, Ma. T. (2016). The Kinematic Evolution of the Ionized Shells in Planetary Nebulae. J. Phys. Conf Serries 728, 032002. doi:10.1088/1742-6596/728/3/032002
López, J. A., Vázquez, R., and Rodríguez, L. F. (1995). The Discovery of a Bipolar, Rotating, Episodic Jet (BRET) in the Planetary Nebula KjPn8. Astrophysical J. 455, 63.
Manchado, A., Stanghellini, L., and Guerrero, M. A. (1996). Quadrupolar Planetary Nebulae: A New Morphological Class. Astrophysical J. 466, L95–L98. doi:10.1086/310170
Marten, H., and Schönberner, D. (1991). On the Dynamical Evolution of Planetary Nebulae. Astronomy Astrophysics 248, 590.
Miller Bertolami, M. M. (2016). New Models for the Evolution of Post-Asymptotic Giant Branch Stars and Central Stars of Planetary Nebulae. Astronomy Astrophysics 588, A25. doi:10.1051/0004-6361/201526577
Miranda, L. F., Ayala, S., Vázquez, R., and Guillén, P. F. (2006). IC 5217 as a Double-Shell, Point-Symmetric Planetary Nebula with a Very Narrow Waist. Astronomy Astrophysics 456, 591–597. doi:10.1051/0004-6361:20065542
Miszalski, B., Acker, A., Parker, Q. A., and Moffat, A. F. J. (2009). Binary Planetary Nebulae Nuclei towards the Galactic Bulge. Astronomy Astrophysics 505, 249–263. doi:10.1051/0004-6361/200912176
Nordhaus, J., and Blackman, E. G. (2006). Low-Mass Binary-Induced Outflows from Asymptotic Giant Branch Stars. MNRAS 370, 2004–2012. doi:10.1111/j.1365-2966.2006.10625.x
Peimbert, M. (1971). Planetary Nebulae II. Electron Temperatures and Electron Densities. BOTT 6, 29.
Peimbert, M. (1967). Temperature Determinations of H II Regions. Astrophysical J. 150, 825. doi:10.1086/149385
Penã, M., Ruíz–Escobedo, F., Rechy–García, J., and García–Rojas, S. (2017). The Kinematic Behaviour of Optical Recombination Lines and Collisionally Excited Lines in Galactic Planetary Nebulae. MNRAS 472, 1182.
Pereyra, M., López, J. A., and Richer, M. G. (2016). The Kinematics of the Nebular Shells Around Low Mass Progenitors of PNe with Low Metallicity. Astronomical J. 151, 53. doi:10.3847/0004-6256/151/3/53
Pereyra, M., Richer, M. G., and López, J. A. (2013). The Deceleration of Nebular Shells in Evolved Planetary Nebulae. Astrophysical J. 771, 114. doi:10.1088/0004-637x/771/2/114
Perintto, M., Schönberner, D., Steffen, M., and Calonaci, C. (1994). The Evolution of Planetary Nebulae I. A Radiation-Hydrodynamic Parameter Study. Astronomy Astrophysics 473, 462.
Reyes-Ruiz, M., and López, J. A. (1999). Accretion Disks in Pre-planetary Nebulae. Astrophysical J. 524, 952.
Richer, M. G., Georgiev, L., Arrieta, A., and Torres-Peimbert, S. (2013). The Discrepant Kinematics of ORLs and CELs in NGC 7009 as a Function of Ionization Structure. Astrophysical J. 773, 133. doi:10.1088/0004-637x/773/2/133
Richer, M. G., Guillén Tavera, J. E., Arrieta, A., and Torres-Peimbret, S. (2017). The Temperature and Density from Permitted O II Lines in the Planetary Nebula NGC 7009. Astrophysical J. 870, 42.
Richer, M. G., López, J. A., Díaz-Méndez, E., Riesgo, H., Baéz, S. H., García-Díaz, Ma. T., et al. (2010b). The San Pedro Mártir Planetary Nebula Kinematic Catalogue: Extragalactic Planetary Nebulae. RMxAA 46, 19.
Richer, M. G., López, J. A., García-Díaz, M. T., Clark, D. M., Pereyra, M., and Díaz-Méndez, E. (2010a). The Evolution of the Kinematics of Nebular Shells in Planetary Nebulae in the Milky Way Bulge. Astrophysical J. 716, 857–865. doi:10.1088/0004-637x/716/1/857
Richer, M. G., López, J. A., Pereyra, M., Riesgo, H., García‐Díaz, M. T., Báez, S. H., et al. (2008). The Acceleration of the Nebular Shells in Planetary Nebulae in the Milky Way Bulge. Astrophysical J. 689, 203–212. doi:10.1086/592737
Sabbadin, F., Turatto, M., Ragazzoni, R., Cappellaro, E., and Benetti, S. (2006). The Structure of Planetary Nebulae: Theory vs. Practice. Astronomy Astrophysics 451, 937–949. doi:10.1051/0004-6361:20054554
Sabin, L., Zijlstra, A., and Greavess, J. S. (2007). Magnetic Fields in Planetary Nebulae and Post-AGB Nebulae. MNRAS 376, 1. doi:10.1111/j.1365-2966.2007.11445.x
Sahai, R., and Trauger, J. T. (1998). Multipolar Bubbles and Jets in Low-Excitation Planetary Nebulae: Toward a New Understanding of the Formation and Shaping of Planetary Nebulae. Astronomical J. 116, 1357–1366. doi:10.1086/300504
Schneider, S. E., and Terzian, Y. (1983b). Planetary Nebulae and the Galactic Rotation Curve. Astrophysical J. 274, L61. doi:10.1086/184151
Schneider, S. E., Terzian, Y., Purgathofer, A., and Perinotto, M. (1983a). Radial Velocities of Planetary Nebulae. Astrophysical J. Suppl. 52, 399. doi:10.1086/190874
Schönberner, D., Ballick, B., and Jacob, R. (2018). Expansion Patterns and Parallaxes for Planetary Nebulae. Astronomy Astrophysics 609, 126.
Soker, N., and Livio, M. (1994). Disks and Jets in Planetary Nebulae. Astrophysical J. 421, 219. doi:10.1086/173639
Soker, N. (2006). Observed Planetary Nebulae as Descendants of Interacting Binary Systems. Astrophysical J. 645, L57–L60. doi:10.1086/505794
Steffen, W., Koning, N., Wenger, S., Morisset, C., and Magnor, M. (2011). Shape: a 3D Modeling Tool for Astrophysics. IEEE Trans. Vis. Comput. Graph. 17, 454–465. doi:10.1109/tvcg.2010.62
Steffen, W., and López, J. A. (2006). Morpho-Kinematic Modeling of Planetary Nebulae with SHAPE. RMxAA 42, 99. doi:10.1017/s1743921306004029
Toalá, J., and Arthur, S. J. (2016). Formationa and X-Ray Emission from Hot Bubbles in Planetary Nebulae - II. Hot Bubble X-Ray Emission. MNRAS 463, 4438.
Wesson, R., Jones, D., García-Rojas, J., Boffin, H. M. J., and Corradi, R. L. M. (2018). Confirmation of the Link between Central Star Binarity and Extreme Abundance Discrepancy Factors in Planetary Nebulae. MNRAS 480, 4589–4613. doi:10.1093/mnras/sty1871
Wilson, O. C. (1950). A Survey of Internal Motions in the Planetary Nebulae. Astrophysical J. 111, 279. doi:10.1086/145264
Zanstra, H. (1932). The Expansion Hypothesis for Planetary Nebulae. Mon. Notices R. Astronomical Soc. 93, 131–149. doi:10.1093/mnras/93.2.131
Zijlstra, A. A., Acker, A., and Walsh, J. R. (1997). Radial Velocities of Planetary Nebulae towards the Galactic Bulge. Astron. Astrophys. Suppl. Ser. 125, 289–292. doi:10.1051/aas:1997220
Keywords: kinematics, planetary nebulae, outflows, binaries, shaping of stellar winds, stellar evolution
Citation: López JA (2022) Deconstructing the Characteristics of the Ionized Gas Component in Planetary Nebulae From Their Internal Motions. Front. Astron. Space Sci. 9:925731. doi: 10.3389/fspas.2022.925731
Received: 21 April 2022; Accepted: 27 May 2022;
Published: 01 July 2022.
Edited by:
Karen B Kwitter, Williams College, United StatesReviewed by:
Gerardo Ramos-Larios, University of Guadalajara, MexicoBruce Balick, University of Washington, United States
Copyright © 2022 López. This is an open-access article distributed under the terms of the Creative Commons Attribution License (CC BY). The use, distribution or reproduction in other forums is permitted, provided the original author(s) and the copyright owner(s) are credited and that the original publication in this journal is cited, in accordance with accepted academic practice. No use, distribution or reproduction is permitted which does not comply with these terms.
*Correspondence: J. A. López, amFsQGFzdHJvLnVuYW0ubXg=