- Space Vehicles Directorate, AFRL/RVBX, Kirtland AFB, Albuquerque, NM, United States
The study of solar energetic particles (SEPs) is an important area of solar research and space weather. An SEP event extends over large regions of the heliosphere, involves energy ranges varying by decades, and evolves over various time and spatial scales and with ion composition, but with SEP observations limited to in situ detections on a few spacecraft for any given event, we are unable to observe these properties synoptically. Solar studies in general are the beneficiaries of imaging and remote sensing observations over practically all wavelengths and timescales from ground and space based detectors that drive increasingly highly sophisticated models. I see this divide as creating a two-class system for researchers, with us SEP researchers as second class members. Following a brief review of my experience with solar imagery and failed ideas on remote imaging of SEP events, I review two remarkable developments that give hope for some new SEP imaging technique. Finally, I discuss two poorly understood questions of impulsive and gradual SEP events that I think can be feasibly approached with current modeling techniques.
Introduction
Solar-heliophysics encompasses a broad range of topics and research techniques. Over the past several decades I believe there has been a growing broad division in the community between those who work with remote observations and imaging techniques, and others, like me, who are confined to getting their primary solar data only from in situ observations. I have in mind observations of solar energetic particles (SEPs) that can be observed only at several heliospheric locations, currently confined to the ecliptic plane. It is great that we have Parker Solar Probe and Solar Orbiter, but the point is that no matter how many SEP detectors we have in space, we are still just drawing samples of a broadly distributed and evolving phenomenon, the SEP event (I’m thinking E > 10 MeV ions and maybe E > 0.5 MeV electrons). There may be multiple sources of unknown size scales from various seed populations and spatial distributions of unknown numbers of SEPs over wide (or maybe narrow) ranges of longitude and latitude for a given SEP event, and the story gets more complex as we ask about different SEP energy ranges and ions of different rigidities. We continue to depend on statistics of many SEP events just to get a rough handle on their basic energy and spatial distributions. The blind man is much better informed about his proverbial elephant than we are about SEP events in the heliosphere. In the meantime from the remote observers, I am dazzled by solar images and model representations of increasing spatial, temporal, and thermal or energy resolution with ever more detailed physics.
Maybe years of frustration are catching up with me. It didn’t always seem this way. I began with analysis of SEP proton events in grad school, then at NRL I worked with OGO-V X-ray flares from the NRL full-sun proportional counter. A definite change of focus from SEPs to flare X-rays, but the basic tool was still analysis of time series of detector counting rates at different energy bands. The object was to get temporal variations of X-ray spectra (flare temperatures and emission measures) or SEP energies and their characteristic values. While one case was in situ observations (SEPs) and the other remote observations of full disk emission from the Sun, that seemed like a minor distinction. It was all solar flare physics.
Solar Images Confront Author
I remember my first encounter with a full disk solar X-ray rocket image, proudly presented for my consideration in an early meeting with my new boss, Pippo Vaiana, the American Science and Engineering (AS&E) physicist in charge of solar observations within the Ricardo Giacconi X-ray astrophysics group. I had a sense of panic that I would somehow have to make a big transition from working with simple time series data to getting physics out of those dark photographic blobs representing solar active regions. Then came the AS&E X-ray telescope on Skylab with lots of solar X-ray images on film. Locations and evolution of coronal holes or numbers and distributions of bright points seemed like straightforward approaches to take from solar X-ray images, and after a poor start (Kahler, Krieger, and Vaiana, 1975), I got used to analyzing X-ray flare images and later analyzed X-ray coronal hole boundaries (e.g., Kahler and Hudson, 2002). Continued interest in SEP events led to correlations of radio bursts and CMEs with SEP events, which did not require image analysis. I began collaborating with Don Reames and Ed Cliver about 1982, again looking only at CME or radio burst listings, not needing image analysis. Work using SEP events as probes of magnetic clouds followed (Kahler et al., 2011), again no remote images needed.
The Divide of Imaging versus in Situ
During the past 2 decades it has been impressive to see the great successes of solar imaging missions. SOHO images greatly eclipsed the pioneering Skylab images, thanks to the revolution from film detectors to CCDs, with ever greater fields of view and spatial resolutions as images of Hinode, SDO, IRIS, PSP, and other missions are presented and analyzed in detail. Advances in physical models combining detailed calculations with quantitative images of magnetic field lines and ionic radiation have led to deeper appreciation of the physical processes in space and time in the solar atmosphere and interplanetary space. Figure 1 shows several recent examples in which authors have combined models and data to extend imagery to heliospheric SEP ion distributions.
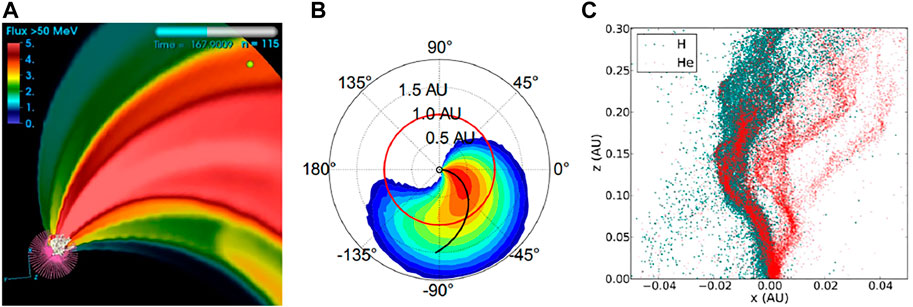
FIGURE 1. (A): E > 50 MeV proton distribution of the 14 July 2000 event 3 h after injection with the STAT model of Linker et al. (2019). (B): Equatorial log color-coded distribution of 10 MeV protons 3 h after impulsive injection based on the FP-FLRP model of Laitinen et al. (2016). (C): model images of H (turquoise) and 4He (red) SEP spatial distributions following spatially separated but simultaneous impulsive injections (Guo et al., 2022).
In stark contrast, the observation of SEPs can be made only with in situ detectors, from which we can produce intensity-time profiles (Figure 2A), evolving or fluence energy spectra, and spatial distributions of SEP events by compiling event averages (Lario et al., 2006; Cohen et al., 2017; see Figure 2B), but a host of questions about spatial/temporal/compositional/energetic evolution of SEP events remains untouched and unapproachable. As a researcher of SEP events, I am frustrated and envious of my first-class colleagues who traffic in spiffy, eye-catching solar/heliospheric images even more spectacular than those of Figure 1. If you are a magazine or journal science editor hoping to engage your reader with a single image from heliospheric SEP physics, do you go with an example from Figure 1 or from Figure 2? Are the SEP distributions of Figure 1 right on or badly off the mark? We’ll never know because we can’t image the SEP events we now study.
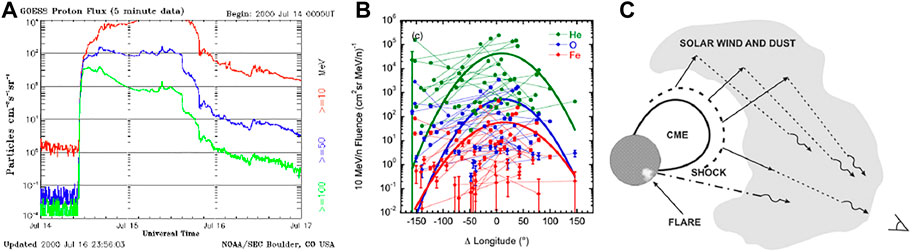
FIGURE 2. (A): GOES profiles of the 14 July 2000 SEP event modeled in Figure 1. (B): Longitude of event fluences for 10 MeV nuc−1 He, O, and Fe with best fit curves (Cohen et al., 2017). (C): schematic of remote observation of SEPs against a grey background of SW and dust (Kahler and Ragot, 2008).
This is not my first whine on the physical barrier to becoming a first-class heliospheric research citizen with images of real SEP particle distributions, evolving in time, and color-coded for energy, maybe even (I’m dreaming here) composition. With co-author B. Ragot we set the goal (Figure 2C) of exploring possible ways that SEPs might interact with the SW to produce neutral radiation that can be imaged by a detector and maybe deconvolved to produce 3-D spatial reconstructions. We (Kahler and Ragot, 2008) found that 4–7 MeV ion de-excitation from SEP collisions with heliospheric 16O and 12C would be far too weak for observation, but that π0-decay γ-rays as detectable signatures of E ≥ 300 MeV nuc−1 SEP ions was possible in large events.
Further candidate remote SEP signatures of positron-decay 0.511 MeV line emission from E > 300 MeV protons; neutrons and the 2.23 MeV neutron-capture line from E > 30 MeV nuc−1 ions; synchrotron emission from E > 0.3 MeV electrons; and transition radiation (TR) from E < 100 keV electrons and from ions were discussed in Kahler and Ragot (2009). TR arises any time a particle crosses an inhomogeneous medium with variation in refractive index and has likely been observed in decimetric bursts of turbulent flares (Fleishman et al., 2005) and in type II bursts from narrow density structures in wakes of CMEs (Chernov et al., 2007). It is best generated by electrons in dense regions where ωp >>ωB, but by protons only in tenuous regions of ωp << ωB (Fleishman and Kahler, 1992). A common theme is that it is not enough to detect such radiation, but it must be imaged to distinguish populations trapped in the corona from those of interplanetary space.
Two Hopeful Surprises
SEP Event ENAs
At the time of our second paper (Kahler and Ragot, 2009) energetic neutral atoms (ENAs) were known as messengers of distant energetic particle populations and the basic tool of the then recently launched (2008) Interstellar Boundary Explorer (IBEX) mission (McComas et al., 2009) to explore the heliospheric termination shock and heliosheath. We acknowledged, but did not explore, ENAs as possible probes of SEPs, so it was an exciting surprise to learn that a SEP event on 5 December 2006 had been detected on STEREO with ENAs by Mewaldt et al. (2009). This was an appropriately big deal at the time and a quick glimpse of ENAs as a promising basis of SEP imaging, as they propagated directly to Earth through a thick sludge of heliospheric magnetic turbulence that retarded the arrival of the charged SEPs composing the main event. The STEREO A/B Low Energy Telescopes (LET-A and LET-B) were not designed to select neutrals, so it was the timing profile and directional information that confirmed the presence of SEPs (Figure 3A). While the LETs are not ENA detectors, separating charged and neutral particles, there were favorable conditions to observe that event. It was a very big (2000 pfu for E > 10 MeV proton) event, able to produce an observable ENA flux at one AU, and it originated on the east limb, allowing a sufficient temporal delay of the onset of the far larger SEP event. The ENAs were further confined to E < 5 MeV, as the cross section for the charge exchange cross section with ambient O+6 coronal/SW ions drops rapidly with energy (Mewaldt et al., 2009). Finally, the SW density drops at least as r−2, presumably negating their use as heliospheric probes of SEPs, although charge exchange calculations of protons with atomic H, O6+, and C4+ by Wang et al. (2014) suggest that ENA detectors of sufficiently low background could detect particle acceleration in the low corona. The Earth’s dipole magnetic field may be such a detector, channeling high energy charged particles to the poles and converting low energy (>0.8 MeV) ENAs into quasi-trapped magnetic equatorial protons (Mason et al., 2021).
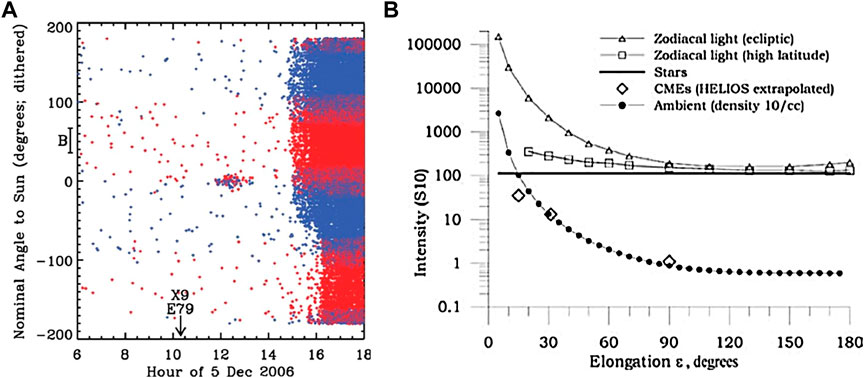
FIGURE 3. (A): Plot of the measured angle to the Sun for individual 1.6–12 MeV proton events on 5 December 2006 (red = LET-A; blue = LET-B). Note the group of counts within ±10⁰ of the Sun from ∼1130 UT to ∼1350 UT, well before the SEP onset at ∼1445 UT (Mewaldt et al., 2009). (B): Surface brightness in S10 units versus solar elongation angle for zodiacal and star light, and of expected CME brightness extrapolated from Helios measurements. A calculation of an ambient medium having a density of 10 e− cm−3 at one AU and an inverse-square density drop off with solar distance is also shown (Jackson et al., 2004).
White Light Interplanetary CMEs
Imaging the SW was thought impossible until Helios B white-light photometer observations revealed the passages of CMEs through its heliospheric field of view (Jackson, 1985). Observing Thomson-scattering of solar white-light photons was also at one time considered a difficult challenge, but because of a very steady zodiacal and stellar white-light background the Solar Mass Ejection Imager imaged CMEs two orders of magnitude fainter than that background (Jackson et al., 2004; Figure 3B). The combination of interplanetary scintillation (IPS) and white-light observations now enable the SW velocities and densities to be reconstructed throughout the inner heliosphere (Jackson et al., 1988, 2020); see https://ips.ucsd.edu), a feat considered impossible at one time and suggesting that there may yet be hope for some new way to image SEPs in space.
Coronal/Interplanetary SEP Imaging
The hope and plea here is that somebody somewhere will get a brilliant idea to detect some kind of neutral radiation from energetic ions and electrons distributed throughout the heliosphere as a SEP event. The odds are really long, but the rewards are enormous. We (currently second-class research citizens stuck with our in situ observations) will be able to join our fellow first-class citizens in proudly displaying images of SEP events and making direct comparisons with increasingly sophisticated model outputs. The advances in understanding where SEPs originate relative to shocks and coronal and solar wind features, followed by their transport histories will greatly accelerate our understanding of SEP physics.
A Plea for Two Needed SEP Models
I will end this story with a modest request to the SEP modeling community for two efforts addressing currently neglected targets that I think well within the capabilities of several SEP models. Rather than the usual procedure of starting with SEP events observed at one AU to estimate injection spectra and numbers, the models would start with injected SEP profiles and calculate resulting SEP numbers and energies observed in one AU detectors.
Total Numbers in 3He Events
For nearly 50 years (Reames, 2021) SEP events have been observed with substantial enhancements (>100 ×) of 3He/4He over the coronal/SW abundance of 5 × 10−4 in the few MeV/nuc energy range. Those events are generally small and impulsive, with source regions in coronal flares and jets. The 3He acceleration process was first explained by absorption of electromagnetic ion cyclotron waves, but currently favored (Reames, 2021) is magnetic reconnection in confined coronal volumes, which may account for upper limits to the observed 3He event fluence distribution observed at one AU (Ho et al., 2005; Figure 4). The 3He acceleration process appears to occur differently from that of 4He (Ho et al., 2019) and may even completely strip a coronal source region of all 3He ions (Reames, 2021).
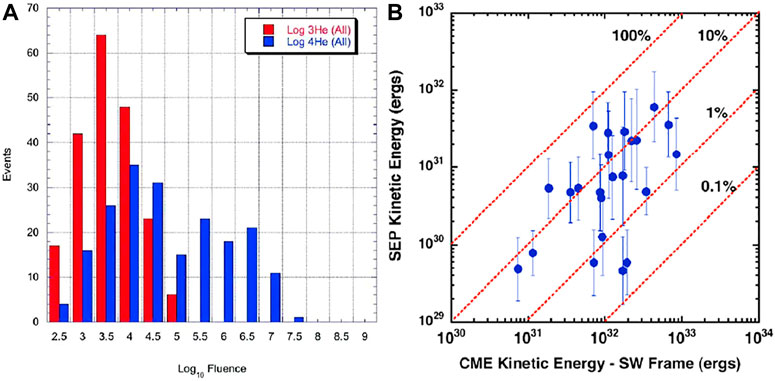
FIGURE 4. (A): Plot of 3He events versus fluence (cm2 sr MeV/nucleon)−1 observed 1997 to 2003 on ACE (Ho et al., 2005). (B): Plot of SEP event energies versus the associated CME kinetic energies for 23 events (Mewaldt et al., 2008).
Well-developed models of jets (Panesar et al., 2016, 2017; Wyper et al., 2018) and extensive observations of 3He coronal sources (Bučík, 2020; Bučík et al., 2021) make it imperative that we compare a calculated 3He ion injection population with corresponding one AU observations to determine the accelerated fraction of the source 3He as a measure of the strength of the acceleration process. This has not been attempted since the Reames (1999) estimate assuming a source region area of 3,000 km2, density of ρ = 1010 cm−3, scale height of 104 km, 3He/4He = 5 × 10−5 for a total 5 × 1031 3He in the volume. He assumed a large one AU event of 105 cm−2 3He (see Figure 4A) resulting from uniform injection in a 20⁰ cone followed by scatter-free propagation and concluded that >10% of the source 3He was accelerated. Surely we (the modelling community intended) can do much better than that. The basic goal is to connect the number of 3He in the source region to the number accelerated and injected from the corona. A size estimate of a reconnection region of an observed jet source could serve as the basis of an input 3He number with a nearly delta function injection in space and time assumed for the accelerated population.
Nearly all 0.02–2 MeV/nuc 3He-rich events are associated with type III radio bursts (Wang et al., 2012; Bučík et al., 2016; Bučík et al., 2018; Bučík et al., 2021), which are used for timing 3He injections but could also aid substantially in the 3He source volume estimate. If we are lucky, the coronal injection regions of the type III-burst electrons are shared by the 3He ions, so the coronal size and location of a 3He event and its extent into the heliosphere will be defined by that of the type III radio burst. It is not yet feasible to image type III radio bursts, but that is exactly one of the goals of the NASA SunRISE mission, due for launch in 2023 (Kasper et al., 2019). SunRISE consists of six small spacecraft at supra-geosynchronous orbit with radio telescopes operating the in the 0.1–22 MHz region, which extends from 10 Rs to one AU.
Shock SEPs for CME Energetics
Fast CMEs are the drivers of shocks that accelerate coronal and SW seed particles to energies sometimes reaching GeV energies (Reames, 2020). That SEP energy is ultimately derived from the kinetic energy of the CME, so an important question is the conversion efficiency of the CME energy into that of SEPs. Mewaldt et al. (2008) examined this question for the 50 biggest E > 30 MeV SEP events of 1996–2003 using associated CME speeds and masses given for 23 of those events in Gopalswamy et al.(2004, 2005). The CME energies are estimated to be accurate within a factor of 2, but the hard part of the comparison was to estimate the total SEP energies, which Mewaldt et al. (2008) calculated with fluence spectra observed from 10 keV/nuc to 1,000 MeV/nuc. Included in their energy calculation were numbers of H and He particle crossings at one AU and adiabatic energy losses for each crossing. The source longitude and latitude distributions were assumed to fall off exponentially from central meridian with e-folding drop-offs of 25° east of CM and 45° to the west and 35° with latitude. For six events the abundances of He and heavier ions were measured, and for the remaining 17 events protons were assumed to be 75 ± 7% of the total energy. Assuming that the shock properties depend on the CME speed relative to the SW, Mewaldt et al. (2008) subtracted an assumed SW speed of 400 km/s from the CME speeds to calculate the CME kinetic energies. The resulting comparison is reproduced in Figure 4B, where the median efficiency is 6.5%.
A similar improved comparison based on simulations of one AU SEP scatterings and energy losses by Chollet et al. (2010) and Gaussian spatial distributions of SEP events adopted by Lario et al. (2006) was carried out by Emslie et al. (2012) for 20 SEP events with results (their Figure 2B) comparable to those of Figure 4B. Another comparison of 94 SEP and CME energies by Kahler and Vourlidas (2013) used CME speeds at measured centers of mass rather than the leading edge speeds and a rotationally symmetric exponential distribution with an e-folding angle of 45° for SEP events with spectra determined by only the 2 and 20 MeV H fluences. Their Figure 7 also showed high SEP efficiencies, including some exceeding unity.
The preceding works all used spatial, spectral, and transport assumptions to convert one AU observed SEP fluences to total numbers and energies of the produced SEPs. The results can be very model-dependent, however. With a simple CME latitude correction Gopalswamy et al. (2021) increased the number of interplanetary E > 500 MeV protons by about an order of magnitude in five of 14 SEP events calculated by de Nolfo et al. (2019) in their study of solar sustained gamma-ray events. In general, however, the assumption parameters are not tested in these SEP calculations. I propose that modelers go the other way, starting with a CME shock model producing SEP events of known spatial, temporal, and energy distributions. The model, with full transport properties, would then track and predict both the total accelerated SEP energies and the intensities and fluences observed at a designated one AU detector. In this scheme the shock longitudinal and latitudinal widths and acceleration timescale variations with energy could all be tracked. The advantage of this approach is that the SEP distributions and energies are known and can be compared with resulting one AU SEP observations and CME energies. Multiple model runs can then establish the uncertainties of the reverse process of estimating SEP energies solely from the one AU observations. The SEP efficiencies of CMEs are too important to be left in their current state of understanding.
Conclusion
I am resigned to continue my SEP investigations as a second-class citizen of the heliospheric research community, operating in the slow lane of in situ observations, while hoping for a better future through some great discovery. In the meantime I would be delighted to see acceptance of my challenges of the preceding Section.
Data Availability Statement
The original contributions presented in the study are included in the article/Supplementary Material. Further inquiries can be directed to the corresponding author.
Author Contributions
The author confirms being the sole contributor of this work and has approved it for publication.
Funding
This work was funded by AFOSR task 22RVCOR006.
Conflict of Interest
The author declares that the research was conducted in the absence of any commercial or financial relationships that could be construed as a potential conflict of interest.
Publisher’s Note
All claims expressed in this article are solely those of the authors and do not necessarily represent those of their affiliated organizations, or those of the publisher, the editors and the reviewers. Any product that may be evaluated in this article, or claim that may be made by its manufacturer, is not guaranteed or endorsed by the publisher.
Acknowledgments
The views expressed are those of the author and do not reflect the official guidance or position of the United States Government, the Department of Defense or of the United States Air Force. My work has been supported by AFRL and AFOSR and has benefited from interactions with many colleagues, especially DR. I thank JB for inviting this Perspective, which has benefited from reviewer comments and discussions within the International Space Science Institute (ISSI) Team ID 425 “Origins of 3He-rich SEPs”.
References
Bučík, R. (2020). 3He-Rich Solar Energetic Particles: Solar Sources. Space Sci. Rev. 216 (2), 24. doi:10.1007/s11214-020-00650-5
Bučík, R., Innes, D. E., Mason, G. M., and Wiedenbeck, M. E. (2016). Association of 3He-Rich Solar Energetic Particles with Large-Scale Coronal Waves. ApJ 833 (1), 63. doi:10.3847/1538-4357/833/1/63
Bučík, R., Innes, D. E., Mason, G. M., Wiedenbeck, M. E., Gómez-Herrero, R., and Nitta, N. V. (2018). 3He-rich Solar Energetic Particles in Helical Jets on the Sun. ApJ 852 (2), 76. doi:10.3847/1538-4357/aa9d8f
Bučík, R., Mulay, S. M., Mason, G. M., Nitta, N. V., Desai, M. I., and Dayeh, M. A. (2021). Temperature in Solar Sources of 3He-Rich Solar Energetic Particles and Relation to Ion Abundances. ApJ 908 (2), 243. doi:10.3847/1538-4357/abd62d
Chernov, G. P., Kaiser, M. L., Bougeret, J.-L., Fomichev, V. V., and Gorgutsa, R. V. (2007). Fine Structure of Solar Radio Bursts Observed at Decametric and Hectometric Waves. Sol. Phys. 241 (1), 145–169. doi:10.1007/s11207-007-0258-y
Chollet, E. E., Giacalone, J., and Mewaldt, R. A. (2010). Effects of Interplanetary Transport on Derived Energetic Particle Source Strengths. J. Geophys. Res. 115 (A6), A06101. doi:10.1029/2009ja014877
Cohen, C. M. S., Mason, G. M., and Mewaldt, R. A. (2017). Characteristics of Solar Energetic Ions as a Function of Longitude. ApJ 843 (2), 132. doi:10.3847/1538-4357/aa7513
de Nolfo, G. A., Bruno, A., Ryan, J. M., Dalla, S., Giacalone, J., Richardson, I. G., et al. (2019). Comparing Long-Duration Gamma-Ray Flares and High-Energy Solar Energetic Particles. ApJ 879 (2), 90. doi:10.3847/1538-4357/ab258f
Emslie, A. G., Dennis, B. R., Shih, A. Y., Chamberlin, P. C., Mewaldt, R. A., Moore, C. S., et al. (2012). Global Energetics of Thirty-Eight Large Solar Eruptive Events. ApJ 759 (1), 71. doi:10.1088/0004-637x/759/1/71
Fleishman, G. D., and Kahler, S. W. (1992). Microwave Transition Radiation in Solar Flares and in Astrophysics. ApJ 394, 688. doi:10.1086/171622
Fleishman, G. D., Nita, G. M., and Gary, D. E. (2005). Evidence for Resonant Transition Radiation in Decimetric Continuum Solar Bursts. ApJ 620 (1), 506–516. doi:10.1086/427022
Gopalswamy, N., Yashiro, S., Krucker, S., Stenborg, G., and Howard, R. A. (2004). Intensity Variation of Large Solar Energetic Particle Events Associated with Coronal Mass Ejections. J. Geophys. Res. Space Phys. 109 (12), A12105. doi:10.1029/2004ja010602
Gopalswamy, N., Yashiro, S., Liu, Y., Michalek, G., Vourlidas, A., Kaiser, M. L., et al. (2005). Coronal Mass Ejections and Other Extreme Characteristics of the 2003 October-November Solar Eruptions. J. Geophys. Res. Space Phys. 110, A09S15. doi:10.1029/2004ja010958
Gopalswamy, N., Yashiro, S., Mäkelä, P., Xie, H., and Akiyama, S. (2021). The Common Origin of High-Energy Protons in Solar Energetic Particle Events and Sustained Gamma-Ray Emission from the Sun. ApJ 915 (2), 82. doi:10.3847/1538-4357/ac004f
Guo, F., Zhao, L., Cohen, C. M. S., Giacalone, J., Leske, R. A., Wiedenbeck, M. E., et al. (2022). Variable Ion Compositions of Solar Energetic Particle Events in the Inner Heliosphere: A Field Line Braiding Model with Compound Injections. ApJ 924 (1), 22. doi:10.3847/1538-4357/ac3233
Ho, G. C., Mason, G. M., and Allen, R. C. (2019). 3He-Rich Solar Energetic Particle Events with No Measurable 4He Intensity Increases. Sol. Phys. 294 (3), 33. doi:10.1007/s11207-019-1420-z
Ho, G. C., Roelof, E. C., and Mason, G. M. (2005). The Upper Limit on 3He Fluence in Solar Energetic Particle Events. ApJ 621 (2), L141–L144. doi:10.1086/429251
Jackson, B. V., Buffington, A., Cota, L., Odstrcil, D., Bisi, M. M., Fallows, R., et al. (2020). Iterative Tomography: A Key to Providing Time- Dependent 3-D Reconstructions of the Inner Heliosphere and the Unification of Space Weather Forecasting Techniques. Front. Astron. Space Sci. 7, 76. doi:10.3389/fspas.2020.568429
Jackson, B. V., Buffington, A., Hick, P. P., Altrock, R. C., Figueroa, S., Holladay, P. E., et al. (2004). The Solar Mass-Ejection Imager (SMEI) Mission. Sol. Phys. 225 (1), 177–207. doi:10.1007/s11207-004-2766-3
Jackson, B. V. (1985). Imaging of Coronal Mass Ejections by the HELIOS Spacecraft. Solar Phys. 100 (1/2), 563–574. doi:10.1007/978-94-009-4588-3_27
Jackson, B. V., Rompolt, B., and Švestka, Z. (1988). Solar and Interplanetary Observations of the Mass Ejection on 7 May, 1979. Sol. Phys. 115 (2), 327–343. doi:10.1007/bf00148732
Kahler, S. W., Haggerty, D. K., and Richardson, I. G. (2011). Magnetic Field-Line Lengths in Interplanetary Coronal Mass Ejections Inferred from Energetic Electron Events. ApJ 736 (2), 106. doi:10.1088/0004-637x/736/2/106
Kahler, S. W., and Hudson, H. S. (2002). Boundary Structures and Changes in Long‐lived Coronal Holes. ApJ 574 (1), 467–476. doi:10.1086/340937
Kahler, S. W., Krieger, A. S., and Vaiana, G. S. (1975). Morphological Evolution of X-ray Flare Structures from the Rise through the Decay Phase. ApJ 199 (2), L57. doi:10.1086/181848
Kahler, S. W., and Ragot, B. R. (2008). Remote Sensing of Gamma‐Ray Emission from Solar Energetic Proton Interactions with the Solar Wind. ApJ 675 (1), 846–852. doi:10.1086/526416
Kahler, S. W., and Ragot, B. R. (2009). Viewing Radiation Signatures of Solar Energetic Particles in Interplanetary Space. Adv. Space Res. 43 (10), 1484–1490. doi:10.1016/j.asr.2009.01.013
Kahler, S. W., and Vourlidas, A. (2013). A Comparison of the Intensities and Energies of Gradual Solar Energetic Particle Events with the Dynamical Properties of Associated Coronal Mass Ejections. ApJ 769 (2), 143. doi:10.1088/0004-637x/769/2/143
Kasper, J. C., Lazio, J., Romero-Wolf, A., Bain, H. M., Bastian, T., Cohen, C., et al. (2019). The Sun Radio Interferometer Space Experiment (SunRISE) Mission Concept. San Francisco: American Geophysical Union. Fall Meeting 2019, abstract #SH33A-02.
Laitinen, T., Kopp, A., Effenberger, F., Dalla, S., and Marsh, M. S. (2016). Solar Energetic Particle Access to Distant Longitudes through Turbulent Field-Line Meandering. A&A 591, A18. doi:10.1051/0004-6361/201527801
Lario, D., Kallenrode, M. B., Decker, R. B., Roelof, E. C., Krimigis, S. M., Aran, A., et al. (2006). Radial and Longitudinal Dependence of Solar 4-13 MeV and 27-37 MeV Proton Peak Intensities and Fluences: Helios and IMP 8 Observations. ApJ 653, 1531–1544. doi:10.1086/508982
Linker, J. A., Caplan, R. M., Schwadron, N., Gorby, M., Downs, C., Torok, T., et al. (2019). Coupled MHD-Focused Transport Simulations for Modeling Solar Particle Events. J. Phys. Conf. Ser. 1225 (1), 012007. doi:10.1088/1742-6596/1225/1/012007
Mason, G. M., Greenspan, M. E., Kanekal, S. G., Leske, R. A., Looper, M. D., Mazur, J. E., et al. (2021). Evidence for Energetic Neutral Hydrogen Emission from Solar Particle Events. ApJ 923 (2), 195. doi:10.3847/1538-4357/ac2fa2
McComas, D. J., Allegrini, F., Bochsler, P., Bzowski, M., Collier, M., Fahr, H., et al. (2009). IBEX-interstellar Boundary Explorer. Space Sci. Rev. 146 (1-4), 11–33. doi:10.1007/s11214-009-9499-4
Mewaldt, R. A., Cohen, C. M. S., Giacalone, J., Mason, G. M., Chollet, E. E., Desai, M. I., et al. (2008). How Efficient Are Coronal Mass Ejections at Accelerating Solar Energetic Particles? PARTICLE ACCELERATION and TRANSPORT IN the HELIOSPHERE and BEYOND: 7th Ann. Internat. Astrophys. Conf. AIP Conf. Proc. 1039, 111. doi:10.1063/1.2982431
Mewaldt, R. A., Leske, R. A., Stone, E. C., Barghouty, A. F., Labrador, A. W., Cohen, C. M. S., et al. (2009). STEREO Observations of Energetic Neutral Hydrogen Atoms during the 2006 December 5 Solar Flare. ApJ 693 (1), L11–L15. doi:10.1088/0004-637x/693/1/l11
Panesar, N. K., Sterling, A. C., Moore, R. L., and Chakrapani, P. (2016). Magnetic Flux Cancelation as the Trigger of Solar Quiet-Region Coronal Jets. ApJ 832 (1), L7. doi:10.3847/2041-8205/832/1/l7
Panesar, N. K., Sterling, A. C., and Moore, R. L. (2017). Magnetic Flux Cancellation as the Origin of Solar Quiet-Region Pre-jet Minifilaments. ApJ 844 (2), 131. doi:10.3847/1538-4357/aa7b77
Reames, D. V. (2021). Fifty Years of 3He-Rich Events. Front. Astron. Space Sci. 8, 164. doi:10.3389/fspas.2021.760261
Reames, D. V. (2020). Four Distinct Pathways to the Element Abundances in Solar Energetic Particles. Space Sci. Rev. 216 (2), 20. doi:10.1007/s11214-020-0643-5
Reames, D. V. (1999). Particle Acceleration at the Sun and in the Heliosphere. Space Sci. Rev. 90 (3/4), 413–491. doi:10.1023/a:1005105831781
Wang, L., Li, G., Shih, A. Y., Lin, R. P., and Wimmer-Schweingruber, R. F. (2014). Simulation of Energetic Neutral Atoms from Solar Energetic Particles. ApJ 793 (2), L37. doi:10.1088/2041-8205/793/2/l37
Wang, L., Lin, R. P., Krucker, S., and Mason, G. M. (2012). A Statistical Study of Solar Electron Events over One Solar Cycle. ApJ 759 (1), 69. doi:10.1088/0004-637x/759/1/69
Keywords: solar energetic particles, solar flares (1496), coronal mass ejection, solar corona and wind, heliospheric magnetic field
Citation: Kahler S (2022) Second-Class Citizen in the Heliophysics Community. Front. Astron. Space Sci. 9:892965. doi: 10.3389/fspas.2022.892965
Received: 09 March 2022; Accepted: 24 March 2022;
Published: 13 April 2022.
Edited by:
Joseph E. Borovsky, Space Science Institute, United StatesReviewed by:
Lulu Zhao, University of Michigan, United StatesDonald Reames, University of Maryland, United States
Copyright © 2022 Kahler. This is an open-access article distributed under the terms of the Creative Commons Attribution License (CC BY). The use, distribution or reproduction in other forums is permitted, provided the original author(s) and the copyright owner(s) are credited and that the original publication in this journal is cited, in accordance with accepted academic practice. No use, distribution or reproduction is permitted which does not comply with these terms.
*Correspondence: Stephen Kahler, c3RlcGhlbi5rYWhsZXJAdXMuYWYubWls