- 1Department of Earth Sciences, Dartmouth College, Hanover, NH, United States
- 2Department of Chemistry, Dartmouth College, Hanover, NH, United States
Past environments on Mars contained abundant water, suggesting certain regions may have been conducive to life as we know it and implying the potential for microbial inhabitants. Gale and Jezero craters, home of the Perseverance and Curiosity rovers, hosted ancient lakes that experienced periods of active hydrologic cycling and prolonged drying intervals. Exploration of these basins (and future operations on Mars) will benefit from detailed characterizations of analogous environments on Earth, where life detection strategies at various spatial scales (i.e., rover to orbiter) can be tested and validated. Investigations of terrestrial analogs are critical for understanding (1) how microorganisms generate chemical biosignatures in environments characterized by multiple extreme conditions; (2) the impact of environmental conditions and mineralogy on biosignature preservation; and (3) what technologies and techniques are needed to detect biosignatures remotely or in situ. Here, we survey five terrestrial sites analogous to climate conditions proposed for Late Noachian to Early Hesperian Mars, when craters are thought to have hosted active lakes. We review the geologic setting, environmental conditions, microbial habitability, extant microbial communities, and preserved biomarkers at each analog and discuss their relevance to the search for signs of life in Martian craters with in situ and remote instrumentation. The analogs range from active to desiccated lake systems, temperate to hyper-arid climates, and have acidic to neutral-pH and hypo- to hyper-saline waters. Each analog hosts microorganisms adapted to multiple extremes (polyextremophiles), including aspects of water availability (i.e., surface waters versus shallow subsurface water versus groundwater) and physiochemistry (e.g., water activity, salinity, temperature, alkalinity, pH, and redox potential) that can form macrobiological features such as microbial mats. Comparing the expected achievable spatial resolution of several key Mars instruments to the spatial extent of macrobiological features at each analog reveals that most features are unlikely to be resolved from orbit and require rover-scale instruments for detection. We recommend that future studies at these analogs use multi-scale remote sensing surveys to determine thresholds for detecting macrobiological features and map how patterns in mineralogy or physical characteristics of environments correlate to modern-day microbial communities or preserved biomarkers. It will also be critical to determine how the characteristics of macrobiological features, such as areal extent, percent cover, thickness, pigments, etc., impact detectability thresholds. These findings can provide vital information on potential topographic or spectroscopic signatures of life, and at what scales they are detectable. This research is critical to guide sample collection locations within craters like Jezero, and for selecting landing sites for future missions in evaporative Martian basins and other rocky bodies.
1 Introduction
Through a series of robotic orbiters, landers, and rovers, Mars exploration has resulted in an impressive amount of data showcasing the broad range of processes that have occurred on the red planet over the last ∼4 Ga (Carr and Head, 2010). Most notable has been the discovery that the surface of Mars has been transformed by water (Carr, 2012; see references in review by Palucis and Morgan, (2020), which implies the possibility for the development of life (Domagal-Goldman et al., 2016). The Martian surface records three main geologic periods (Figure 1) — the Noachian (∼4–3.6 Ga), Hesperian (∼3.7–3 Ga), and the Amazonian (∼3 Ga to present-day) — which differ in the volume and persistence of liquid water present on the surface (Kereszturi, 2012b; Ramirez and Craddock, 2018). Craters throughout the Noachian appear to be continually modified at rates higher than present day (e.g., Craddock and Howard, 2002; Forsberg-Taylor et al., 2004), suggesting that water, in addition to wind, played a significant role in their modification (Changela et al., 2021). Many of the valley networks on Mars formed during a climate optimum at the end of the Noachian, near the Noachian-Hesperian transition (Fassett and Head, 2008a; Kereszturi and Petrik, 2020). Surface waters then declined through the Hesperian, though alluvial fans, deltas, and smaller valley networks continued to form through the Early Amazonian (e.g., Fassett and Head, 2008b; Grant and Wilson, 2012; Hauber et al., 2013). Modern-day Mars is a cold (mean temperature 213 K) and hypobaric global desert, where surface conditions rarely reach the triple point of water, though deliquescence can occur at night due to elevated humidity (Pál et al., 2019) and allow the emergence of microscopic liquid water today (Martín-Torres et al., 2015). Periods of high obliquity throughout the Amazonian (Laskar et al., 2004; Holo et al., 2018), however, may have allowed for the precipitation of water ice distant to the poles at lower latitudes, where ice could be warmed to provide surface runoff (Soare et al., 2008).

FIGURE 1. Timeline comparing the major epochs on Mars to the corresponding eons on Earth. Paleolakes likely existed in Gale and Jezero craters on Mars during the Late Noachian to Early Hesperian.
Much of Mars’ water history has been determined by interpreting remote sensing data, where decades of missions have led to continual improvements in data quality and resolution (Supplementary Table S1), so that orbital data coverage rivals that which is publicly available for Earth. Satellite-based data sets from visual and thermal cameras have revealed fluvial features that span from thousands of kilometers long (e.g., valley networks like Uzboi-Ladon-Marova) down to the meter-scale (e.g., individual scroll bars on delta deposits) (Christensen et al., 2004; Malin et al., 2007; McEwen et al., 2007). Hyperspectral data from OMEGA (Observatoire pour la Minéralogie, l’Eau, les Glaces et l’Activité) and CRISM (the Compact Reconnaissance Imaging Spectrometer for Mars) have revealed a globally diverse mineralogy that supports weathering occurred due to liquid water (e.g., Bibring et al., 2006; Ehlmann and Edwards, 2014). In the last two decades, rover and lander data has provided high-resolution geologic, geomorphic, and geochemical data at the local scale, contributing both an increased understanding of the history of Mars (Squyres et al., 2004; Ming et al., 2006; Grotzinger et al., 2014, 2015; Mangold et al., 2021), and providing ground truthing data for orbitally-derived datasets (e.g., Hamilton et al., 2014; Arvidson et al., 2015).
The array of orbiters and landers sent to Mars have not only provided an abundance of evidence that Mars may have once maintained environments partly similar to those on Earth, they have provided a detailed global perspective on where we might focus efforts and resources for life detection missions (des Marais, 2010). If extinct or extant life on Mars is similar to life on Earth in a most general aspect, it requires liquid water, energy sources (to support a metabolism), and chemical elements such as carbon, hydrogen, oxygen, nitrogen, phosphorus, sulfur, and trace metals for biosynthesis (Jakosky and Shock, 1998). Accordingly, a habitable environment for early Martian life must be characterized by: the presence of water, temperatures and pressures that permit liquid water and necessary biomolecules to exist, accessible chemical elements, chemical and/or light energy, and protection of biomolecules from cosmic rays and ultraviolet radiation (des Marais, 2010).
When the Mars Science Laboratory (MSL) Curiosity rover was sent to Gale crater in 2012 (Figure 2), a primary mission goal was to determine whether the crater had hosted one or more habitable environments during its history. It was clear from the stratigraphy, mineralogy, and landforms within Gale, which had also been well studied from orbit (Malin and Edgett, 2000; Anderson and Bell, 2010; Milliken et al., 2010), that liquid water had once been present, but it was not clear from orbit whether the landing site constituted a habitable environment. Sedimentary evidence at the rover-scale supports orbital interpretations that Gale once hosted a large lake for ∼105–107 years with fluctuating lake levels, during the Late Noachian to Early Hesperian (Grotzinger et al., 2015). The ancient lake in Gale likely had a neutral pH, low salinity, variable redox states of iron and sulfur, and contained all the major chemical elements necessary for life. Therefore, this lake was deemed habitable for hypothetical chemolithoautotrophic microbes (Grotzinger et al., 2014). The successes of the MSL mission, and the Mars Exploration Rovers (MER) mission before it (e.g., Squyres and Knoll, 2005), have resulted in NASA’s Mars Exploration Program goals progressing from “follow the water” to “search for habitable environments” to now “seek signs of life.” As such, the newest Mars rover, Perseverance, is exploring an ancient lake system within Jezero crater (Figure 2) with a specific focus on searching for signs of ancient microbial life (Farley et al., 2020). Part of this mission involves the collection of Martian soils and rocks that will be brought back to Earth for detailed analysis (Beaty et al., 2019).
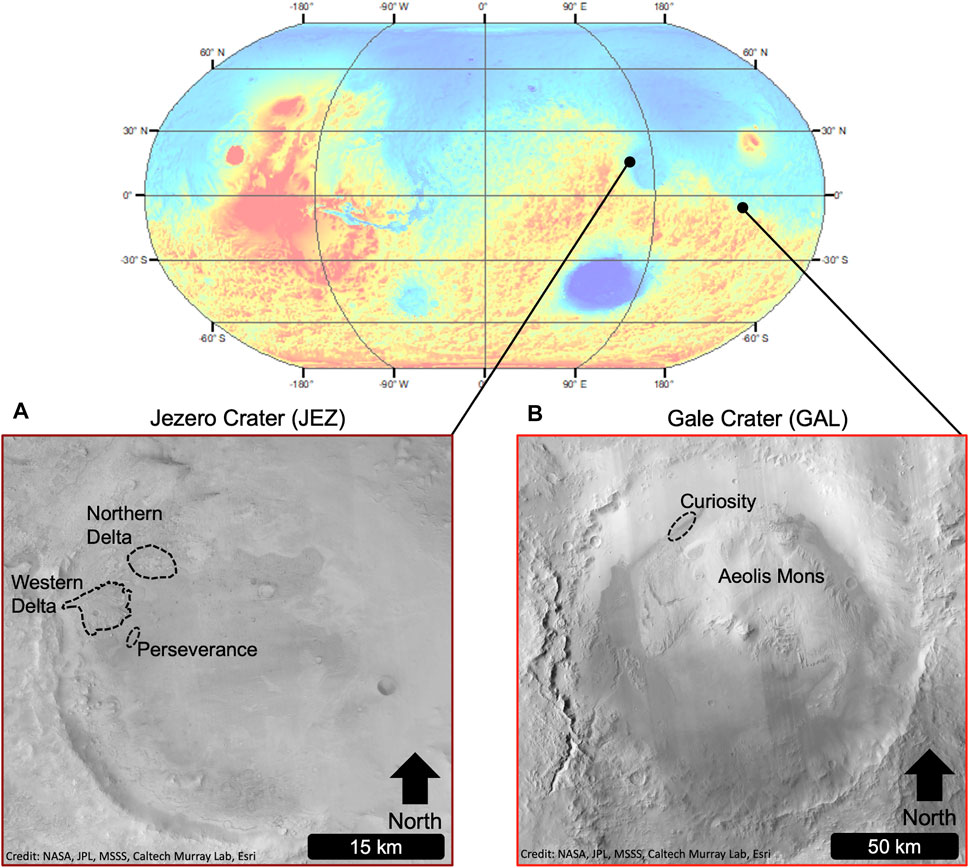
FIGURE 2. Global context for Jezero and Gale craters on Mars. The Perseverance and Curiosity rovers are exploring these areas, which are probable targets for future life detection missions. (A) Jezero crater is characterized by deltaic deposits on the western and northern edges of the crater. The Perseverance rover’s approximate area of operation (as of December 2021) is shown. (B) Gale crater contains the prominent Mt. Sharp (Aeolis Mons) deposit. The Curiosity rover’s approximate area of operation (as of December 2021) is shown north of Aeolis Mons.
Successful mission design and data collection for sample return missions, including the current Mars 2020 mission in Jezero crater and the subsequent Mars Sample Return campaign, requires an understanding of (1) how microbial life responds to changes in water availability and activity and geochemical gradients under drying conditions, including changes to microbial spatial distributions or their association with different mineral assemblages (e.g., clays, silicas, carbonates), (2) how and what type of biosignatures (chemical, textural, or morphological) are preserved through time and in which minerals and deposits, and (3) how surface processes and geochemistry might affect where and how biosignatures are concentrated within specific environments. As Earth is the only planet known to host life, understanding the three requirements detailed above necessitates a better characterization of terrestrial analogs to inform Martian landing site selection over a range of past possible Mars climate scenarios. It is important to consider the spatial scale of data products from terrestrial analogs; to be most relevant to Mars exploration, data collection should span from orbital down to rover scales.
In this review we focus on five previously identified terrestrial analogs for Mars that have the potential to inform us about biosignature preservation in ancient Martian paleo-lakes, specifically those discovered in Gale and Jezero craters. These analog sites host mineral assemblages similar to some Martian craters and encompass a range of chemistries and climates [e.g., “warm and wet” (e.g. Ramirez and Craddock, 2018); “cold and wet” (Fairén, 2010), “icy/snowball with transient warming” (e.g., Wordsworth et al., 2013; Wordsworth, 2016), or semiarid to hyperarid (e.g., Horvath and Andrews-Hanna, 2017, 2021)] proposed for Late Noachian to Early Hesperian Mars. Each analog also hosts polyextremophiles that are predominantly microbial. While we do not consider an exhaustive list of potential analogs in this study, we believe the body of research at these sites are representative of the types of data and studies being conducted on Earth that can increase our understanding of the connections among environmental and geochemical conditions, microbial habitability, and biosignature preservation potential on Mars. Our synthesis of research at these sites also identifies major gaps and recommends future work to bridge these gaps.
1.1 Ancient Martian Lake Systems
Two of the best studied habitable environments on Mars are within Gale and Jezero craters (Figure 2). Gale crater is a Late Noachian- to Early Hesperian-aged crater that is ∼150 km in diameter (Thomson et al., 2011). It is located along the crustal dichotomy, a topographic boundary that separates the heavily cratered southern highlands of Mars and the sparsely cratered northern lowlands. The crater hosts a 5-km-high sedimentary mound, Aeolis Mons (i.e., Mt. Sharp), which is the Curiosity mission’s primary science target (Grotzinger et al., 2012). This is due to the fact that lower strata of Mt. Sharp contains hematitic and hydrated clay- and sulfate-bearing rock, which were detected from orbit (Milliken and Bish, 2010; Fraeman et al., 2013), but are accessible on the ground by the Curiosity rover. Investigations by the rover, as well as from satellite imagery, have determined that Gale crater hosted a series of large lakes, both before (Grotzinger et al., 2015) and after (e.g., Palucis et al., 2016) the emplacement of Mt. Sharp. These lakes may have been as large as ∼5,800 km2 in area and up to ∼0.7 km deep (Palucis et al., 2016). The sources of water to the lakes were likely from a combination of groundwater and surface runoff, the latter potentially resulting from snowmelt (Grotzinger et al., 2015; Palucis et al., 2016; Roseborough et al., 2021). The climate during the time of lakes is debated, with modeling and geomorphologic arguments suggesting a relatively arid- to semi-arid climate with temperatures above freezing (Horvath and Andrews-Hanna, 2021), whereas the mineralogy and geochemistry support more dynamic climates with periods of prolonged warm and semi-arid conditions on a mostly cold/snowball Mars (Fukushi et al., 2019). Fukushi et al. (2019) estimated that early lakes in Gale developed in ∼104 to ∼106 year-long semiarid climates based on pore water chemistry from the last wetting event near the Curiosity landing site (i.e., Yellowknife Bay), which is based on finding water with neutral pH, mild salinity, low levels of dissolved CO2, and highly oxidizing conditions (Eh > 300 mV, where Eh is redox potential).
Jezero crater is a ∼45 km diameter crater located in the Nili Fossae region of Mars. It was selected as the landing site of the Perseverance rover due to the presence of two sedimentary fan-like structures inferred to be deltas from orbit (e.g., Fassett and Head, 2008b; Goudge et al., 2015) which was later confirmed with sedimentological ground observations (Mangold et al., 2021). These deposits and their mineralogy indicate an ancient habitable lake (with similar water conditions to Gale) that formed during the Late Noachian to the Early Amazonian (Ehlmann et al., 2008; Goudge et al., 2015; Mangold et al., 2020). Jezero is an ideal location to search for biosignatures on Mars due to its geologic context (an ancient lake), its mineral diversity from orbit (including hydrated magnesium carbonates and olivine, clay minerals, and silicas), and its potential to preserve biosignatures based on these specific mineral deposits (Grant et al., 2018). There are also potential hydrothermal deposits within Jezero, as well as aqueously altered mafic and ultramafic rock, allowing for the search for traces of prebiotic chemical processes (Bosak et al., 2021).
1.2 Criteria for Terrestrial Mars Analogs
Based on these two landing sites, terrestrial analogs would ideally meet several specific Mars-like conditions. Both the Gale and Jezero lakes are thought to have been active during the Late Noachian through the end of the Hesperian. There is debate about the range of temperatures on the Martian surface at this time, but there is no clear geomorphic or sedimentologic evidence that the lakes were ice-covered (e.g., Palucis et al., 2016), such that average temperatures may have been above freezing when lakes were present. Work by Fukushi et al. (2019) suggests redox conditions at Gale supported distinct periods of warming on an otherwise cold (∼0°C) Mars. Given these constraints, terrestrial lake systems that span mean annual temperatures at and above 0°C are desirable targets. Both the Gale and Jezero lakes appear to have been fed from a combination of groundwater and surface water, which is the case for many terrestrial lake systems, though some studies suggest that the runoff was sourced from snowmelt (versus direct precipitation) (Grotzinger et al., 2015; Palucis et al., 2016; Roseborough et al., 2021). Mars is a mostly basaltic planet (Tanaka et al., 2014), so ideal Earth analogs would be hosted in basaltic terrains, with mafic to ultramafic rocks that lack extensive metamorphism or tectonics. Ideal analogs would also have similar mineralogies to those found at Gale and Jezero, namely the presence of magnesium carbonates, Fe/Mg clay minerals, amorphous silica, hematite, and sulfates (Vaniman et al., 2014; Goudge et al., 2015; Rampe et al., 2017). In order to document how polyextremophile microorganisms respond or vary as a function of water activity and chemistry, analog systems should also have well-documented water salinities, pH, and redox potential, in addition to well-characterized microbial populations, and process and/or metabolism specific biosignatures. Lastly, as it is thought that Martian lake systems went through periods of wetting and drying (Stein et al., 2018), knowing the hydrologic history of each analog site is important, specifically periods of lake desiccation due to climate change or glaciation and changes in water sources or chemistries over time.
2 Terrestrial Analog Sites
We review five terrestrial analog sites that span climatic, geological, and geochemical conditions (Figure 3) that meet the above criteria for Late Noachian to Hesperian lakes on Mars. As surface and orbital observations support multiple periods of lake stability in Gale crater, likely separated by long durations of aridity, we chose several Atacama salars as an analog for hyperarid Mars (Fairén et al., 2010). The low temperatures, high solar irradiance, and hypersalinity of soils make this a robust analog (Azua-Bustos et al., 2017; Häder and Cabrol, 2018), and the formation of modern microbialites in surface waters provides a natural laboratory to investigate microbialite formation in Mars-like environments (Farías et al., 2014). During lake highstands on Mars, the climate has been suggested to be either “warm and wet,” “cold and wet,” or “icy/snowball Mars with transient warming” (or fluctuate between warmer/wetter and colder/drier conditions) based on modeling, geomorphology, and/or geochemical constraints (e.g., Fairén, 2010; Wordsworth, 2016; Horvath and Andrews-Hanna, 2017, 2021; Hurowitz et al., 2017). To cover periglacial and icy climates with varying degrees of aridity, we chose two polar sites, the Haughton Impact crater in the Canadian Arctic, and the McMurdo Dry Valleys of Antarctica, which host some of the oldest lakes in Antarctica (Hendy et al., 1977). Haughton impact crater was also chosen as it is morphologically similar to Martian lake basins (i.e., an impact crater) and hosts endolithic communities in impact-shocked rocks, providing opportunities to study how we might conduct subsurface searches for life. Rio Tinto was chosen as an analog for a “warm and wet,” but acidic, Mars, and Lonar crater was chosen due to its geologic and morphologic similarity to Martian lake basins (i.e., an impact crater within basaltic terrain). We note, however, that the high population density and agricultural activity of the Lonar region (Menzel et al., 2013, 2014) may limit its utility in studies of microbial habitability due to confounding anthropogenic influences. In addition, many of these analogs have been used to test instruments, rovers, and field work logistics due to their similarities to the conditions expected on Mars (e.g., Rio Tinto (Orgel et al., 2014), Haughton Impact Crater (Lee, 2002; Kereszturi, 2011), the Antarctic Dry Valleys (Anderson et al., 1992), and the Atacama Desert (Cabrol et al., 2001).
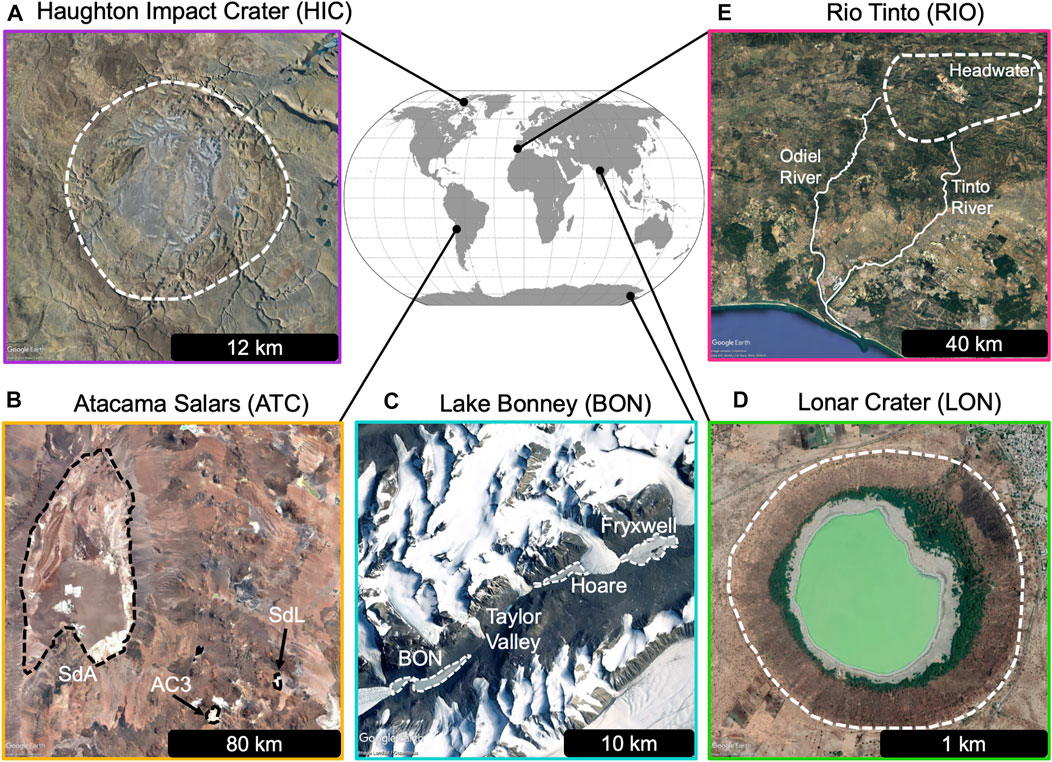
FIGURE 3. Map of the Earth showing the locations of five terrestrial Mars analogs. Panels show the regional context of each site. (A) Haughton Impact Crater on Devon Island, Nunavut Canada. Seasonal meltwater flows out of the crater through the river valley on the northeast side of the crater wall. (B) The Atacama Desert in Chile, this region contains many hypersaline saltflats including Salar de Atacama (SdA), Salar de Laco (SdL), Salar de Aguas Calientes 3 (AC3). (C) Lake Bonney is one of several perennially ice-covered, chemically stratified lakes surrounded by bare rock in the Mcmurdo Dry Valleys of Antarctica. Neighbording lakes include Fryxell and Hoare. Glacial streams and the lake margins (moat) melt during the brief Austral summer. (D) Lonar Crater and lake in Lonar, India. Lonar is the only basaltic crater lake on Earth. The lake’s watershed experiences heavy agricultural cultivation. (E) The Rio Tinto River flows from heavily mined headwaters to the Atlantic Ocean. The headwaters are highly acidic and are characterized by high concentrations of iron and other heavy metals.
The intent of this review is to focus on a subset of sites relevant to Late Noachian to Early Hesperian lakes (and their possible associated climates) across which we can compare remote and in situ observations, not to review every terrestrial environment that has been proposed as a Mars analog. We present an approach that can now be applied to the many other terrestrial analogs. While no terrestrial analog is a perfect representation for Martian lakes, together, these five analogs (1) provide windows into the microbial habitability and preservation potential in Martian craters that once hosted lakes, (2) have been relatively well-studied in terms of their geologic history and environmental history, and (3) constitute natural laboratories in which to test Mars mission concepts and technologies from the orbital to lander/rover/drone scales (Figure 4, Supplementary Table S2). Below we synthesize a cross-site comparison of geological setting, climate, mineralogy, water chemistry, and active microbiology, generated from an extensive literature review. This work is consolidated in Table 1.
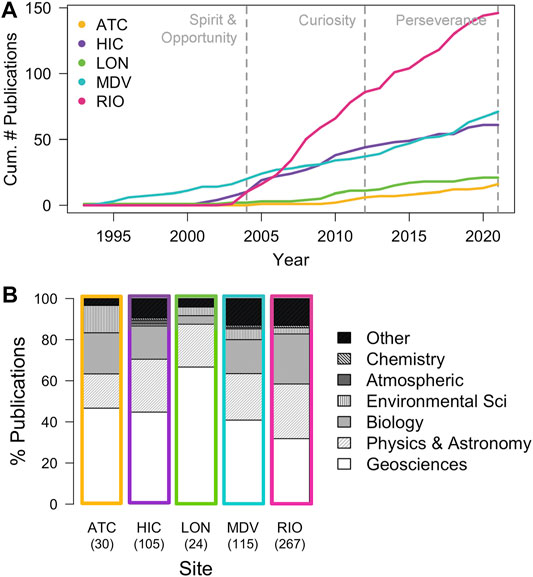
FIGURE 4. (A) Cumulative number of Web of Science publications between 1993 and 2021 that match the search term “Mars” and terms for each terrestrial analog site. ATC = “Atacama salars” or “saltflats”; HIC = “Haughton Impact Crater” or “structure”; LON = “Lonar lake” or “crater”; MDV = “McMurdo Dry Valleys”; RIO = “Rio Tinto” or “Riotinto” or “Tinto River.” Search conducted October 2021. Dashed vertical lines denote when notable rovers landed on Mars. (B) The % of publications for each site that match disciplinary categories. These broad categories are based on category assignments generated by Web of Science. Total number of publications is indicated parenthetically beneath the analog site code. See Supplementary Table S2 for details.
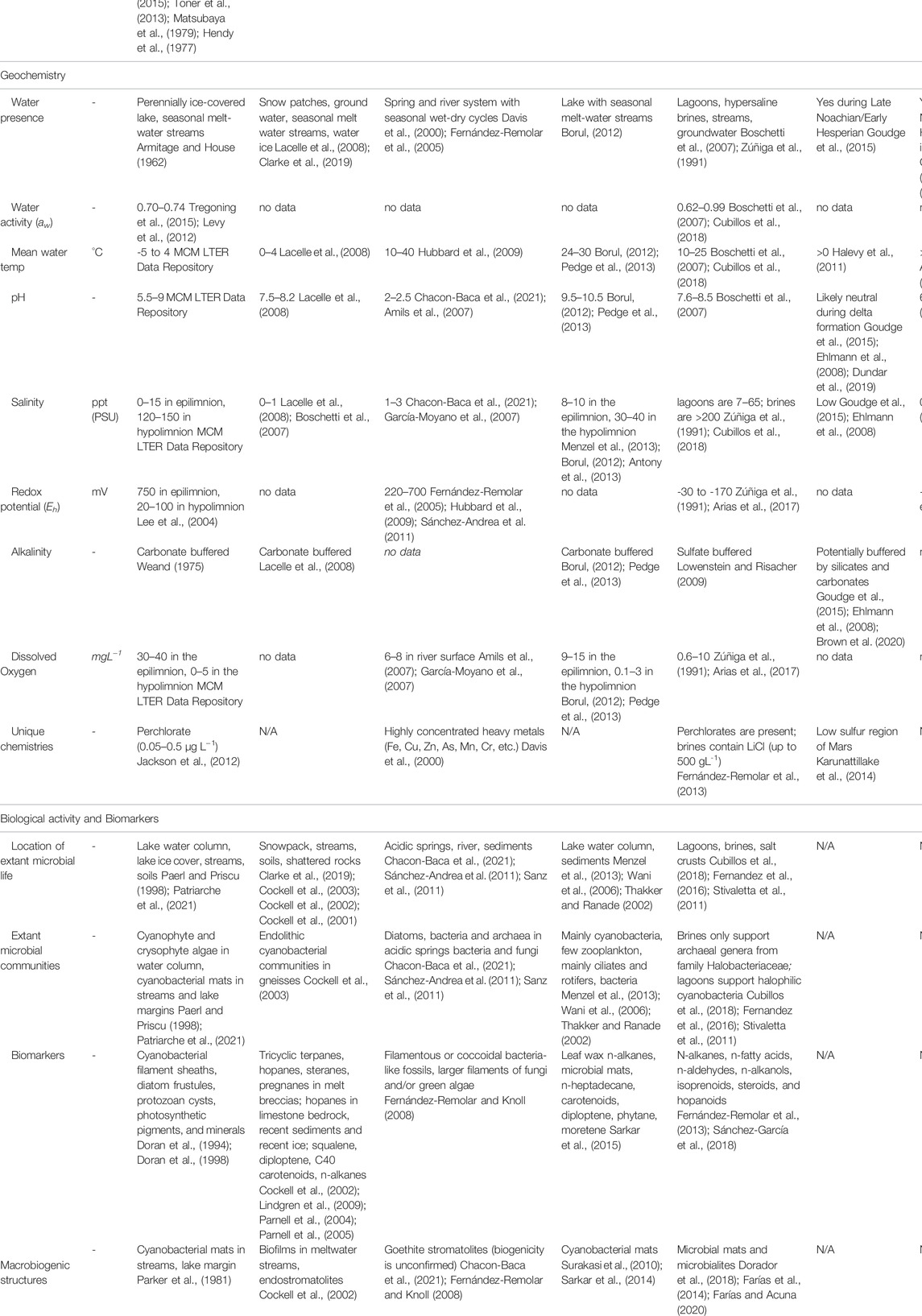
TABLE 1. A cross-site comparison of selected environmental, mineralogical, chemical, and biological parameters.
2.1 Haughton Impact Crater
Located on Devon Island in the Canadian Arctic, the Haughton Impact Crater (HIC) is a large crater (23 km in diameter) formed by an impact approximately 39 Ma (Osinski and Lee, 2005). While a crater lake may have been present immediately after the impact, subsequent erosion removed any evidence along with impactites (Osinski and Lee, 2005). A lake formed within ∼8–10 million years of the impact, resulting in the Haughton Formation of dolomite-rich Miocene-age lacustrine sediments (Hickey et al., 1988). The paleohydrology of the lake responsible for the Haughton Formation is poorly constrained, and Quaternary glacial and fluvioglacial sediments overlay the formation itself (Lacelle et al., 2008). The modern climate at HIC is broadly consistent with a polar desert (Lacelle et al., 2008), and water is present mainly as ice, including surface ice, ground ice, and semi-permanent snowbanks (Clarke et al., 2019), with seasonal streams that transport cobble to gravel-sized sediment constituting the only liquid water (Osinski and Lee, 2005; Lacelle et al., 2008). Geochemical characterization of these water sources is consistent with dolomite dissolution (Lacelle et al., 2008), with several lacustrine sediment samples from the Haughton Formation yielding high sulfate concentrations (Léveillé, 2007). Solute concentrations and pH values (7.5–8.3) were lowest in residual snowbanks and increased in snow meltwater and streams, indicating progressive carbonate dissolution (Lacelle et al., 2008).
As a desiccated impact structure that once held a paleolake and currently has a cold desert climate, HIC is a compelling analog to Gale and Jezero craters. Despite the harsh environment, there is abundant evidence of biological activity and biomarker preservation in HIC. Biofilms form on meltwater streaks (several m wide and 100 s of m long) on the crater wall that are comparable to recurring slope lineae on Mars (Clarke et al., 2019) and may be possible to characterize with sub-meter resolution imagery (i.e. aerial, drone, or field-based data). Large sulfur isotope fractionations in the sulfate-rich bedrock (Parnell et al., 2010) provides evidence for persistent microbial sulfate reduction. Endolithic microbial communities, including cyanobacterial species, can thrive in the impact-shocked rocks at HIC (Cockell et al., 2002, 2003). Finally, various lipid biomarkers of biogenic origin, including tricyclic terpenes, hopanes, steranes, and pregnanes (Parnell et al., 2006; Lacelle et al., 2009; Lindgren et al., 2009), occur in HIC microenvironments such as melt breccias, dolomitic limestone bedrock, and young surface ice (Parnell et al., 2004, 2005).
2.2 Atacama Salars
The Atacama Desert in Northern Chile is the oldest continually arid region on Earth, with desert conditions having developed in the Late Triassic (Clarke, 2006; Jordan et al., 2014). Precipitation of up to 25 mm yr−1 falls mainly in the austral summer and potential evaporation is ∼2000 mm yr−1, resulting in a negative hydric water balance (Risacher et al., 1999). Persistent desert conditions have formed distinctly Mars-like soils, which are up to 2 million years old, and are characterized by extremely low moisture, low concentrations of organic matter, and Mars-like salt compositions, including perchlorates (Ericksen, 1983; Navarro-González et al., 2003; Ewing et al., 2008). Atacama surface soils have the highest concentrations of perchlorate found naturally on Earth (up to 0.6 wt%), which approach the concentrations reported for Martian soils (0.5–1 wt%, Davila et al., 2013). This mountainous region contains many closed (up to hundreds of meters) evaporitic basins, known as salt flats or salars, some of which contain saline lakes or liquid brines.
Salar de Atacama is the largest evaporitic basin (Arriagada et al., 2006; Flahaut et al., 2017). Smaller basins, such as Salar de Laco and Salar de Aguas Calientes, are located at higher elevation (Flahaut et al., 2017; Aerts et al., 2020). Considered together, these salars represent a natural gradient in elevation, temperature, evaporation, and soil moisture (Risacher et al., 2003; Aerts et al., 2020). Solar irradiance is uniformly high. Salar lakes (lagoons) and pools are fed by ephemeral streams, snowmelt, springs, and/or groundwater; evaporation exceeds inflows in all cases and salinities range from hypo to hypersaline (Boschetti et al., 2007). Salar brines are hypersaline (350–550 gL-1 of salt), near neutral pH, with low redox potential (<-30 mV) (Risacher et al., 2003; Boschetti et al., 2007; Arias et al., 2017; Cubillos et al., 2018). No brines in the Atacama are alkaline (Lowenstein and Risacher, 2009). Mean annual air temperatures range from 1 to 14°C, depending on elevation, and mean daily water temperatures range from 10 to 25°C, depending on season and elevation (Boschetti et al., 2007; Cubillos et al., 2018).
Despite the multiple extreme conditions, patchy microbial communities survive in both surface and subsurface soils and in the hypersaline brines, though the population of bacterial cells in soils is low (or was not detected) in some areas (Navarro-González et al., 2003; Connon et al., 2007; Crits-Christoph et al., 2013). Fueled by the hygroscopic abilities of halite, endoevaporitic microbial communities (dominated by phototrophs), persist 3–7 mm beneath salt crusts (Wierzchos et al., 2006; Davila et al., 2008). Halophilic archaea from the family Halobacteriaceae, some of which are capable of surviving in high lithium environments, thrive in salt crusts, brines, and pools (Cubillos et al., 2018).
Salar soils contain biomarkers in Neogene evaporites that record past aqueous conditions (Pueyo et al., 2002). Lipid biomarkers, which are diagnostic of prokaryotic life (Wilhelm et al., 2017, 2019; Sánchez-García et al., (2018)), are well-preserved in salars due to the hyperaridity (Wilhelm et al., 2017) and presence of halite and gypsum salts (Fernández-Remolar et al., 2013; dos Santos et al., 2016).
2.3 Lake Bonney, Dry Valleys, Antarctica
The McMurdo Dry Valleys (MDV) contain numerous lakes and are the largest ice-free portion of the Antarctic continent (McKnight et al., 1999). Conditions in this polar desert ecosystem are cold (mean annual temperature is −17 to −25°C) and arid (mean annual precipitation is <10 mm), and experience strong katabatic winds from the polar plateau (Fountain et al., 1999; Doran et al., 2002; Doran and Fountain, 2021).
The MDV region contains several ice-covered lakes in hydraulically closed basins that receive inputs mainly from local glaciers (Fountain et al., 1999; Gooseff et al., 2011). Lake Bonney is the most-well studied, beginning in the 1960s (e.g., Armitage and House, 1962), and receives water inputs from four ephemeral streams fed by Taylor Glacier, which it abuts. Water remains liquid (−5°C to +6°C) due to heat from solar radiation, stream inflow, and the latent heat of ice formation (McKay et al., 1985). Bonney consists of two lobes (East and West) that are connected by a shallow sill; both lobes have highly stratified water columns that contain a fresh, well-oxygenated epilimnion that overlies a hypersaline (>70 ppt), suboxic or anoxic, nutrient rich hypolimnion (Spigel and Priscu, 1998; Gooseff et al., 2011). Of relevance to Mars, perchlorate has been measured in the water column (0.05–0.5 μgL−1) (Jackson et al., 2012).
Antarctic perennially ice-covered aquatic environments are considered oases for life in a harsh, polyextreme environment. Lake Bonney’s water column hosts a complex microbial ecosystem with evidence for phylogenetically diverse bacteria, microalgae and fungi and many microbial metabolisms, including photoautotrophy, mixotrophy, and chemolithoautotrophy (Paerl and Priscu, 1998; Vick-Majors et al., 2014; Vick-Majors and Priscu, 2019; Patriarche et al., 2021). Despite the ice cover limiting light to <3% of incident, photosynthesis by cryptophytes and chrysophytes occurs in the epilimnion and phytoplankton and bacterioplankton production are maximized directly beneath the ice cover and just above the chemocline, where nutrients are supplied via diffusion (Priscu et al., 1988). Microbial sulfate reduction occurs below the chemocline in both lobes where sulfate is present (Lyons and Welch, 2016). Cyanobacterial mats are common in the lake margins (moats) that form during the short summer and in glacial meltwater streams (Wood et al., 2008; Kohler et al., 2016); dominant taxa include Phormidium, Nostoc, and Oscillatoria species, though diatom algae are also present (Gooseff et al., 2011). Recent research demonstrates the potential for these microbiological mat communities to be characterized via orbital data (Power et al., 2020; Salvatore et al., 2020).
Lake sediments contain many biomarkers, including cyanobacterial filament sheaths, diatom frustules, protozoan cysts, photosynthetic pigments, and minerals (e.g., carbonates) associated with microbial activity (Doran et al., 1994). This site is the only analog that is completely devoid of plants, metazoans, and human influence and therefore provides a unique opportunity to study microbial interactions in a Mars-like system with few confounding factors.
2.4 Lonar Lake
Lonar Lake (LON) is shallow (max depth 6 m) and occupies a young impact crater formed between 15 and 570 ka (Sengupta and Bhandari, 1988; Sengupta et al., 1997; Storzer and Koeberl, 2004; Jourdan et al., 2011). The crater is located on a ∼65 Ma Deccan basalt that overlies the southern Indian shield in the Buldhana district, Maharashtra, India (Kumar, 2005; Wright, 2014). Primary minerals include plagioclase (labradorite) and pyroxenes (augite and pigeonite) (Maloof et al., 2010). Hydrothermal alteration materials including saponite, celadonite, and calcite (Hagerty and Newsom, 2003), are proposed to be the result of groundwater interactions with remnant impact energy (Nayak, 1996). The impact excavated into the multi-layer basaltic aquifer, forming a closed basin lake approximately 25–65 m below local springs (Menzel et al., 2013, 2014; Komatsu et al., 2014). Lonar lies in the core monsoon zone, and surface runoff is the dominant water source during the monsoon season from July to September, with groundwater springs on the crater walls predominant in the dry season (Komatsu et al., 2014; Menzel et al., 2014). Streams have incised Dhara Canyon and formed an alluvial fan inside the crater (Menzel et al., 2013, 2014). The lake is saline (8–40 ppt) and hyperalkaline (3,000–5,000 mg/L) due to evaporative concentration, with pH from 9.5 to 10.5 (Borul, 2012; Menzel et al., 2013; Pedge and Ahirrao, 2013). The water column is highly eutrophic, and the water appears yellow-green to dark green due to the high concentration of algae and dissolved solids (Surve et al., 2021). Laminated sediments form in the anoxic zone (below 4 m), constituting one of the few climate records in Central India (Menzel et al., 2013).
Microbial life is diverse in the waters of Lonar lake, including cyanobacteria, planctomycetes, spirochaetes, and an alkalophilic methanogen (Thakker and Ranade, 2002). Cytophagales and microaerophilic Bacteroidaceae are abundant in soils and contribute to organic matter remineralization (Wani et al., 2006). Lake sediments preserve a variety of biomarkers (i.e., n-alkanes from leaf waxes, n-heptadecane, carotenoids, diplotene, phytane, and moretene) ranging from 1.4 to 10.1 Ka (Sarkar et al., 2015).
2.5 Río Tinto, Spain (RIO)
The Río Tinto system, located in the Iberian Pyritic Belt in Southwest Spain, is the world’s largest acidic river (∼95 km long) (Boulter, 1993). The estuary system, formed via hydrothermal activity approximately 2 Ma (Fernández-Remolar et al., 2005), flows through ore deposits (iron and copper sulfides) to the Atlantic Ocean. These subsurface deposits include copiapite, jarosite, schwertmannite, halotrichite, and gypsum and are generally depleted in organic compounds (Edwards et al., 2007). Despite freshwater inputs and seasonal wetting-drying cycles, the Tinto River headwaters are characterized by high acidity (pH 2–2.5) and high concentrations of iron and other heavy metals (i.e., Cu, Zn, As, Mn, Cr, etc.) (Amils et al., 2007; Hubbard et al., 2009; Chacon-Baca et al., 2021). The headwaters are an extremely oxidizing environment with redox potential ranging from 220 to 700 mV (Fernández-Remolar et al., 2005; Hubbard et al., 2009). Many minerals with relevance to Mars form in the seasonally arid streambeds, including iron oxides and ferric sulfates (such as hydronium jarosite) (Fernández-Remolar et al., 2005).
Most biomass at Río Tinto is constrained to the riverbed and rock surfaces, where filamentous algae and fungi form biofilms that trap prokaryotic organisms (Amaral Zettler et al., 2002; Amils et al., 2007; Aguilera et al., 2010), but iron and sulfur oxidizing microbes do grow in subsurface sulfide deposits via chemolithotrophic metabolisms (Fernández-Remolar et al., 2005; Amils et al., 2007). High concentrations of subsurface methane suggest anaerobic methane oxidizing microbes may also be present (the MARTE Team et al., 2008). While subsurface deposits are generally organic-poor, smectite phyllosilicates contain organic carbon and preserve a diversity of biomarkers (Fernández-Remolar and Knoll, 2008). Ironstones in headwater terraces contain well-preserved macroscopic and microscopic biosignatures within iron oxide precipitates (Fernández-Remolar et al., 2005).
3 Synthesis
While each analog site is unique with respect to geologic setting, climate, and geochemistry, all have relevance to Late Noachian through Early Hesperian lakes on Mars, and together inform our understanding of how habitability and biosignature preservation varies across extant terrestrial analogs. Each analog also has considerable remote and in situ geological and biological observations. Below we compare water geochemistry at analog sites to that predicted for crater lakes at Gale and Jezero, discuss patterns in biosignature preservation at each analog, and explore how incorporating remotely sensed observations with rover-scale field-studies can increase the value of such studies to the field of astrobiology.
3.1 Microbial Habitability
Field sites relevant to astrobiology experience environmental conditions that expand our understanding of the limits for life (defined as microbial growth or metabolism). On Earth, microbial habitability is limited primarily by water availability and activity (aw), where water activity is related to the amount of water in a liquid (Price and Sowers, 2004; Merino et al., 2019). Microbes prefer higher aw liquids and the lower limit observed for life is aw = 0.6, but most microbes cannot grow at aw < 0.9 and only extremophilic microbes can survive below aw = 0.75 (Oren, 2008). Other geochemical characteristics of water, such as temperature and salinity, influence life via the modulation of water availability. In addition to water, life requires an energy source to drive chemical reactions (Jelen et al., 2016). Physiochemical gradients generate variable redox conditions and these gradients along with proton gradients, likely fueled metabolism and growth as microbial life arose on Earth (Lane et al., 2010).
Each terrestrial analog experiences multiple extreme conditions, and yet microbial communities persist at all sites, demonstrating the ability of extremophilic life to adapt simultaneously to multiple stressors. This occurrence suggests that life may have evolved to withstand the conditions of nearly every niche on Earth, albeit at varying abundances and levels of diversity (Merino et al., 2019). To examine the limits of habitability on Earth and make inferences about the potential habitability of Mars, it is useful to compare the unique combination of boundary conditions that control habitability and influence the structure and function of microbial communities at terrestrial analogs to those predicted for ancient Mars lakes. Here, we characterize four key environmental parameters at each analog—pH, temperature, salinity, and redox potential (Figure 5). The similarity of analog boundary conditions to the conditions predicted for Late Noachian to Early Hesperian lakes in Gale and Jezero craters is indicated by overlapping polygons in this multidimensional space. The polar desert analogs (HIC and BON) are characterized by cold, neutral water with moderate salinity (<50 ppt) and a wide range of redox potentials, and in these ways are most similar to our current understandings of the Martian paleolakes in Gale and Jezero craters. While the remaining sites show less similarity in these four parameters than HIC or BON, they are important for gaining insight into the capability of life to adapt to environments with very low water activity (ATC brines), where mineral assemblages are similar to Mars (RIO), and in basaltic crater lakes (LON).
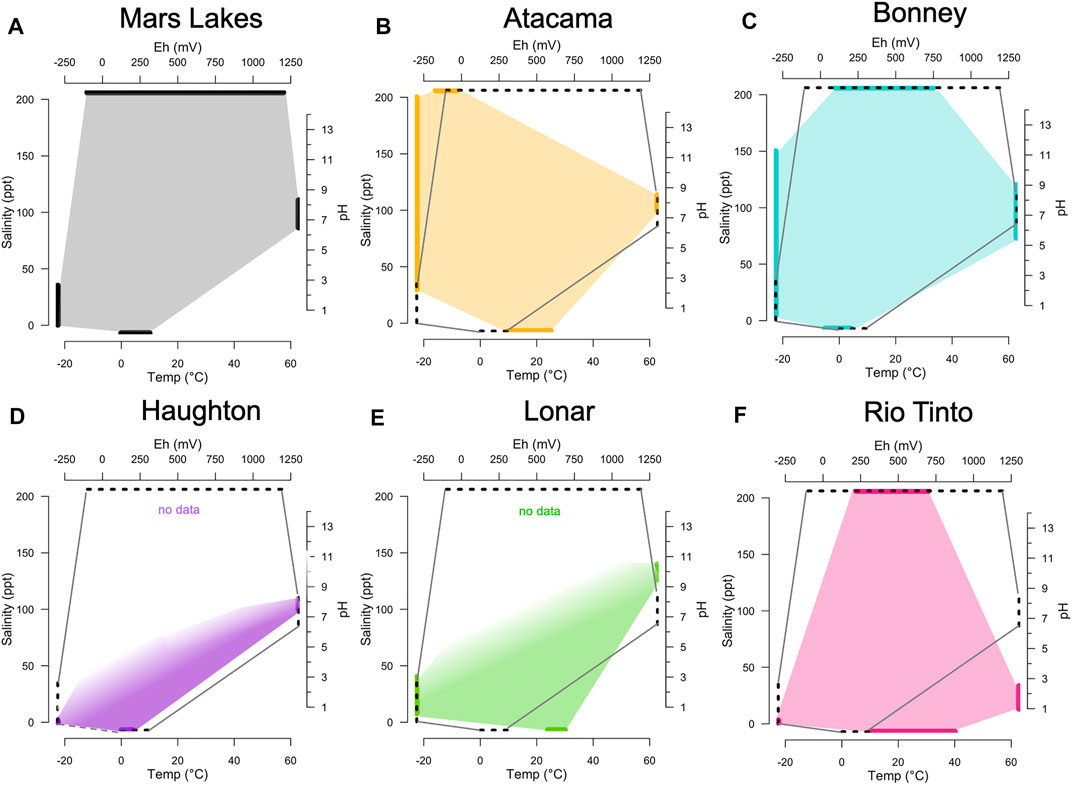
FIGURE 5. (A) The temperature, salinity, pH and redox potential (Eh) boundaries predicted for Mars lakes during the Late Noachian to Early Hesperian. Martian lakes compared to (B–F) boundaries observed for five terrestrial Mars analog sites discussed in the main text. Each polygon edge (thick lines) represents the range observed for a single parameter. Filled polygon areas represent parameter distributions in multidimensional space. The dashed polygons in (B–F) compare the martian lakes parameter distribution to each terrestrial analog. Missing values are indicated by the absence of a polygon edge on a particular axis. Note that the Mars lakes polygon (A) depicts best estimates of parameter ranges when liquid water was present. All of the terrestrial analog polygons (B–F) depict boundaries for liquid water present in the modern environment. Modeled after Merino et al., 2019.
These comparisons between environmental conditions at terrestrial analogs and in Mars paleo-lakes (Figure 5) may assist researchers to select the analog that is most appropriate to address specific research questions and goals. One limitation of these comparisons is that they depict only the endpoints of published environmental parameters in the present and do not consider how boundary conditions co-vary spatially or temporally (e.g., over seasonal, interannual, or geological time scales). For example, shortly after impacts, hydrothermal systems are known to develop (Osinski et al., 2013). Hydrothermal systems have been proposed as locations where life may have originated on Earth or on Mars. Most impact events that form large craters (>2 km diameter on Earth, >5 km on Mars) have the potential to general a hydrothermal system (Osinski et al., 2013). The geochemical parameters of the hydrothermal system will evolve over time as the impact crater cools (eg., Kring et al., 2020). On Earth, salinities in hydrothermal fluids are often initially high (∼20% NaCl) at high temps (75–100°C) and decrease as temperatures cool (Lüders and Rickers, 2004). Anoxic or potentially euxinic conditions can develop depending on how isolated the crater basin is from the surrounding aqueous environment (O’Sullivan et al., 2016). Redox conditions depend on the minerals present, and hydrothermal craters on Earth can support several microbial metabolisms, including iron reduction, sulfate reduction, sulfur reduction, and methane oxidation (Kring et al., 2020). Habitable temperatures (<120°C) can persist in impact-induced hydrothermal systems for ∼103–105 years, depending on the impact size and host rock permeability (Abramov, 2004; Kring et al., 2020).
Microorganisms are present within the entirety of the phase space represented at each analog. The diversity, evenness, and abundance of microbial life, however, will vary greatly within the phase space as fewer organisms can tolerate the more “extreme” range of conditions, especially when multiple extreme conditions co-occur. Understanding how these parameters combine to limit life and shape its distribution in time and space is critical to further our understanding of whether life could have evolved on Mars and where to look for it.
3.2 Biosignature Preservation Potential at Analog Sites
Biosignature preservation potential refers to the likelihood that an organic biomarker is lithified and survives diagenesis and/or other degradation processes to be detectable in the present day. Biosignature preservation is extremely heterogeneous due to many factors that vary over small spatial scales (i.e., mm to cm), such as water activity and availability, UV radiation, oxygenation (redox potential), and the formation of organic-mineral complexes which can protect lipids from degradation (Hays et al., 2017). By compiling previous research conducted at the selected Mars analog sites we can examine how mineralogy and environmental conditions impact biosignature preservation potential. A variety of organic biomarkers have been recovered from Mars analogs (Table 1, Supplementary Figure S1, Supplementary Table S3), however, we focused our analysis on lipids, which are important targets for identifying biosignatures on Mars because these complex organic molecules have a low probability of abiotic origin (Des Marais, 2013) and are likely to survive long-term storage (i.e., ∼106 years) on Mars (Georgiou and Deamer, 2014; Srivastava et al., 2020). Lipid biomarkers have been recovered at all five sites, demonstrating that every site preserves biosignatures over Quaternary to Jurassic timescales (up to 108 years) regardless of differences in local mineralogy (Table 1, Supplementary Figure S1, Supplementary Table S3). Lipid biomarkers are categorized into five classes based on hydrocarbon identity and structure after Brocks and Summons (2003). While the taxonomic specificity of lipid biomarkers varies, in general, they provide some insight into what classes of organisms were present in ancient ecosystems (Summons et al., 2021).
Because the surficial mineralogy of Mars can be characterized remotely, understanding how mineralogy affects preservation of biomolecules on Earth is a priority. For example, studies have shown that soils and sediments with high abundances of clay minerals (phyllosilicates) prevent diagenesis and tend to preserve organics well (e.g., Hays et al., 2017). Clay mineral assemblages are found at all analog sites except HIC and may promote biomarker preservation. All six classes of lipid biomarkers have been detected in clay minerals at BON, though the age of these biomarkers has not been determined (Doran et al., 1994). Lipid biomarkers are also well-preserved in halide minerals, such as the halite and gypsum salts found in ATC (Fernández-Remolar et al., 2013; dos Santos et al., 2016). At RIO, 2–8 Ma fossils are well-preserved, likely due to the presence of iron oxide (microfossils) and goethite (macrofossils) (Fernández-Remolar and Knoll, 2008). Iron oxides increase preservation by providing radiation protection (Gómez Gómez et al., 2004). This process is especially relevant to sedimentary rocks formed at Meridiani Planum, the landing site of the Opportunity rover, and suggests these rocks are capable of preserving evidence of microbial life (Fernández-Remolar and Knoll, 2008).
Environmental factors also impact preservation. Radiation exposure decreases preservation potential because long exposures can destroy organic molecules and alter their associated isotopic ratios (Summons et al., 2011). Ultraviolet radiation exposure is especially high at high latitudes, like BON and HIC, and at high elevations, like regions of ATC. UV radiation protection might be encountered in deep lakes, lakes with high suspended solid concentrations, or areas with rapid soil/sediment accumulation (Santschi et al., 1990).
A second key environmental factor impacting biomarker preservation on Earth is the oxygen exposure time (Reiche et al., 2018). Highly productive aquatic systems tend to see high organic matter preservation owing to high respiration rates and correspondingly low oxygen levels (Wetzel, 2001). Similarly, high sediment accumulation rates in deltaic settings reduce the oxygen exposure time, thereby promoting preservation (Naeem et al., 2000; Hays et al., 2017). Deeper lakes promote preservation because anoxic conditions reduce oxidative diagenesis (Berner, 1989) and support sulfurization, which can preserve biosignatures (Raven et al., 2016). Sulfurization enhances preservation over geologic time scales by converting organic matter into more recalcitrant macromolecules. Sulfate minerals are common in Gale, for example in the Murray Formation, and sulfurization is a potential mechanism for preserving organic matter in mudstones. Exploring sulfurization preservation in analogs with deep, closed basin lakes with anoxic bottom waters, like Lake Bonney, would have strong relevance to Gale Crater. In particular, future studies should assess how organic matter structure, sedimentation rate and redox conditions impact the process of sulfurization. Most organic matter preserved in lacustrine and deltaic environments, however, is of terrestrial origin, and may obscure the signal of less productive in situ aquatic communities. Lake Bonney and Haughton Impact Crater, whose watersheds are either completely or partially devoid of terrestrial vegetation, may be especially useful for future biomarker research.
Subaerial environments on Earth’s surface that do not have standing lakes or pools, like Haughton Impact Crater and Atacama soils, preserve a variety of organic, chemical, and isotopic biosignatures (Horgan, 2016). Extremely dry soils, like those of the Atacama, appear to preserve biomarkers well, especially when shielded from UV radiation (Fagliarone et al., 2020). The concentration of organics in these environments is variable, where high concentrations of organic matter are rare and often associated with microbial mats (Hays et al., 2017). Given the uncertainty in surface coverage of microbial communities in desert soils and potentially Mars, determining biomarker preservation potential in these environments is difficult.
The biomarker record at analog sites is complex and there are numerous caveats for interpretation even at locations whose geologic history and modern environment are well-characterized. More research is necessary to further elucidate the process of biomarker preservation within mineral matrices at terrestrial analogs, especially over longer timescales. Our analysis identifies which minerals are currently underexplored with respect to lipid biomarkers at each analog site (Supplementary Figure S1). Such work is vital to predict what diagenetic processes could occur in similar environments on Mars and can inform site selection for future astrobiological missions.
3.3 Biosignature Preservation Potential on Mars
Many of the sedimentary rocks on the Martian surface have experienced minimal alteration since their deposition due to the lack of robust plate tectonics on Mars (Breuer and Spohn, 2003). For example, the ancient smectite-bearing mudstones found in Gale crater show little mineralogical evidence for burial diagenesis, despite burial depths of up to 5 km (Borlina et al., 2015). This phenomenon likely increases the potential for biomarkers to be preserved. However, there are also many challenges for biomarker preservation on Mars, which were reviewed extensively by Hays and others (2017). Briefly, these include (1) water activity and availability, which impact both habitability and biosignature preservation potential; (2) chemical oxidants, such as perchlorates, are common in Martian regolith and can degrade organic biosignatures; and (3) high ultraviolet radiation on Mars’ surface. Exposure ages on Mars vary and some areas of Gale Crater are as young as 80 ± 30 million years (Farley et al., 2014), but continued exposure to ionizing radiation will eventually destroy all biomarker signals in the top few cm’s of exposed regolith and can impact biomarkers in the top 1 m of the surface (Hassler et al., 2014; Hays et al., 2017).
The rovers currently exploring Gale and Jezero craters, Curiosity and Perseverance, respectively, both possess analytical instrumentation capable of detecting lipid biosignatures (i.e., alkanes, isoprenoids, fatty acids, or alcohols) and other organic biomarkers. Curiosity, in Gale Crater, is outfitted with a suite of instruments known as the Sample Analysis at Mars (SAM), which can detect a variety of biosignatures ranging from polar and non-polar organics, large organic molecules (>20 C atoms per molecule), and refractory organics (Mahaffy et al., 2012). Perseverance, in Jezero Crater, carries the Scanning Habitable Environments with Raman and Luminescence for Organics and Chemicals (SHERLOC), which uses a UV Raman spectrometer and fine-scale imaging capabilities to detect biosignatures (Beegle et al., 2015).
Detecting certain lipid classes via mass spectroscopy methods often requires extraction in organic solvents and separation of the total lipid extract into component parts based on polarity (Azua-Bustos et al., 2020). SAM has the capabilities to perform some extractions, including volatilizing lipids for GC-MS analysis with tetramethylammonium hydroxide (Williams et al., 2019). The spectroscopic method used by SHERLOC does not require pre-derivatization but is more susceptible to matrix effects, which can occur when organics are mixed with regolith or sediment and have the potential to confound biomarker detection (Abbey et al., 2017). The detection limits for SAM (on the order of ppb, Williams et al., 2019) are approximately 1000 times more sensitive than for SHERLOC (on the order of ppm, Beegle et al., 2015). The Rosalind Franklin is scheduled to launch in September 2022 and expected to land in Oxia Planum, a clay-rich plain (Mandon et al., 2021), in mid-2023. This rover will detect biomarkers via the Mars Organic Molecular Analyzer (MOMA), which contains a gas chromatographic-mass spectrometer and a Raman spectrometer (Siljeström et al., 2014; Goesmann et al., 2017). MOMA cannot perform complex pre-treatments and has a detection limit similar to SHERLOC (Siljeström et al., 2014; Goesmann et al., 2017). Unlike the other rovers, the Rosalind Franklin can drill to 2 m depths (Kereszturi et al., 2016), which may allow the collection of regolith with higher preservation potential. The concentration and distribution of biomarkers will have a large impact on the ability of rovers to detect biomarkers in situ due to these inherent instrument sensitivities. Thus, identification of targets that are most likely to harbor preserved biomarkers is critical. Field studies with rover-scale technologies at terrestrial analogs provides an opportunity to identify favorable working conditions for specific instruments or techniques and can increase the chance of detecting features of interest (e.g., Bontognali et al., 2021).
3.4 Remote Sensing at Analogs to Guide Exploration on Mars
Remote sensing techniques are a cornerstone of Mars research because they enable frequent coverage of large areas and are a cost-effective precursor to in situ analysis (Malin et al., 2007; van der Meer et al., 2012). Interpretation of satellite data informs landing site selection (Golombek et al., 2017; Grant et al., 2011, 2018) and rover-based spectral and image data are used to determine targets for follow up in situ analysis, and in some cases, sample collection (i.e., Farley et al., 2020). In addition to providing information on topography and mineralogy, remote sensing may also be useful for detecting evidence of life and identifying regions on Mars amenable to subsurface sampling (Kereszturi, 2012a).
Applying remote sensing to terrestrial analogs with well-characterized biological features provides an opportunity to develop techniques to remotely detect signs of extant (and possibly extinct) microbial life. These techniques could be used to inform rover landing site selection and sample collection on Mars, as well as other terrestrial bodies with potential astrobiological importance. In Section 3.4.1, we consider the spatial scale and morphology of macrobiogenic features (> cm scale) at each analog in comparison with the resolution of Mars remote imagers to determine what types of observations would be necessary to detect these microbially-generated features. In Section 3.4.2, we discuss visible and near infrared (VNIR) spectral features of minerals present at each analog in relation to instrumentation coverage of Mars imagers. Further, we consider which of the minerals that can be spectrally detected have high biomarker preservation potential, which can inform the search for putative biomarkers on Mars (Bosak et al., 2021).
3.4.1 Remote Observation of Biogenic Features in Terrestrial Analogs
Microbial life can form macroscopic biological features (> cm scale) including microbial mats/biofilms, microbialites, ooids, etc. Microbial mats and biofilms are present at all five analog sites, though these vary in species composition, morphology, and extent (spatial and temporal) (Table 1, Supplementary Table S4). The McMurdo Dry Valleys host patchy cyanobacterial mats in the margins (moats) of ice-covered lakes and seasonal melt-water streams that are active only during the brief summer weeks (Sohm et al., 2020). These mats contain bacteria, archaea, and eukarya (diatoms) (Mikucki et al., 2010) and can span tens of meters around the lake margins and in streams (Power et al., 2020). Biofilms at Haughton Impact crater are similarly seasonally ephemeral; dark biofilm aggregates (species composition unknown) grow in melt streaks from semi-permanent snow patches during summer drainage (Clarke et al., 2019). While these melt streaks can reach up to 1 km in length, they are more commonly several hundred meters in length, and have an average width of 7 m. Extensive microbial mat communities in the surface waters and margins of Lonar Lake, dominated by phototrophs, show the opposite seasonality, where mat thickness and color depends on freshwater inflow, and these mats sometimes disappear entirely during the arid summer months (Surakasi et al., 2010; Sarkar et al., 2014). At their maximum extent, these mats can cover the entire lake surface (approximately 1 km2). In contrast, thick surficial biofilms at Rio Tinto are ubiquitous on rock surfaces and are present year round (Fernández-Remolar and Knoll, 2008; Chacon-Baca et al., 2021). RIO biofilms are dominated by fungi that form dense streamers and host acid-tolerant bacteria and algae (mainly diatoms and chlorophytes) (Amaral Zettler et al., 2002; Fernández-Remolar and Knoll, 2008). In Atacama salars, sediment biofilms and microbial mats in hypersaline lake surface waters are dominated by halophilic bacteria and archaea, and are present year-round (Dorador et al., 2018; Farías, 2020). The distribution of these mats is uneven and depends on small-scale variations in environmental controls.
Microbialites, formed when mineral detritus is trapped by extrapolymeric substances produced by microbial mats (Noffke et al., 2001), are present in Haughton and potentially Rio Tinto. At HIC, finely laminated calcite deposits occur in dolomitic bedrock outcrops within the impact structure and around its southern rim; these endostromatolites were likely formed via abiotic and microbially-mediated mineralization (Lacelle et al., 2009). The preserved microbial communities in limestone fissures mostly consist of aerobic and chemoheterotrophic bacteria (Pellerin et al., 2009). In the Rio Tinto headwaters, multilayered deposits of finely laminated goethite resemble stromatolites, though the biogenicity of these deposits has not been confirmed and they may have been formed via seasonally episodic sedimentation (Fernández-Remolar and Knoll, 2008).
Because macrobiological features can be large (cm to km) and distinct from the surrounding abiotic environment, the ability to remotely detect (via orbiters or drones) their presence based on certain characteristics has been demonstrated at many locations on Earth, including Mars analog sites. For example, microbial mats can also be remotely sensed based on spectral characteristics. In the Fryxell Basin of the McMurdo Dry Valleys, high-resolution orbital imagers have used unique infrared spectral signatures to detect local microbial mat communities (mainly filamentous cyanobacteria) and to analyze their spatial and temporal variability (Salvatore et al., 2020). Analyses of microbial mat coverage from orbital remote sensing can be paired with field data to estimate biomass and carbon stocks of these microbial communities with high accuracy (Power et al., 2020; Salvatore et al., 2021). Similarly, Warren-Rhodes and others (2007) demonstrated that chlorophyll pigment biosignatures in the Atacama Desert could be detected via VNIR spectra from satellite imagery, as could environments previously identified to hold high potential for hosting microbial life (such as alluvial fans and deltas).
Stromatolites also can be remotely sensed based on their morphology. The biogenicity of stromatolites, however, is difficult to confirm and relying on the morphology as the sole means of detection has a high likelihood of leading to a false positive (McMahon and Cosmidis, 2021). A combination of microtextural and geochemical data and optical microscopy will likely be necessary to confirm stromatolite biogenicity (Goodwin and Papineau, 2022) and will require the use of rover cameras and sampling assays on Mars (McMahon and Cosmidis, 2021).
While terrestrial satellites can detect these macrobiological features remotely, the ability to detect similar features on Mars will depend on instrument capabilities and require an interpretive framework informed by terrestrial biology. Comparing the expected achievable spatial resolution of several key Mars instruments to the spatial extent of macrobiological features at each analog reveals that most features are unlikely to be resolved from orbit and would require rover instruments for detection (Figure 6). Figure 6 depicts the maximum spatial extent of macrobiological features, many of which are patchy in distribution and vary seasonally and interannually. Additionally, the spatial extent represents the maximum extent in one dimension and does not take total area into account. Remotely resolving features like the biofilms in meltwater streaks at HIC, which are long (up to 1 km) but narrow (several m) will likely require sub-meter pixel sampling, as elongated features require higher spatial resolution to be accurately extracted (Lechner et al., 2009). These terrestrial analogs provide an opportunity to identify spatial thresholds (i.e., minimum extent) for remote detectability of macrobiological features. Future work should examine how biological and physical characteristics of these features, such as color, pigment concentrations, thickness, percent cover, activity, etc., impact detectability.
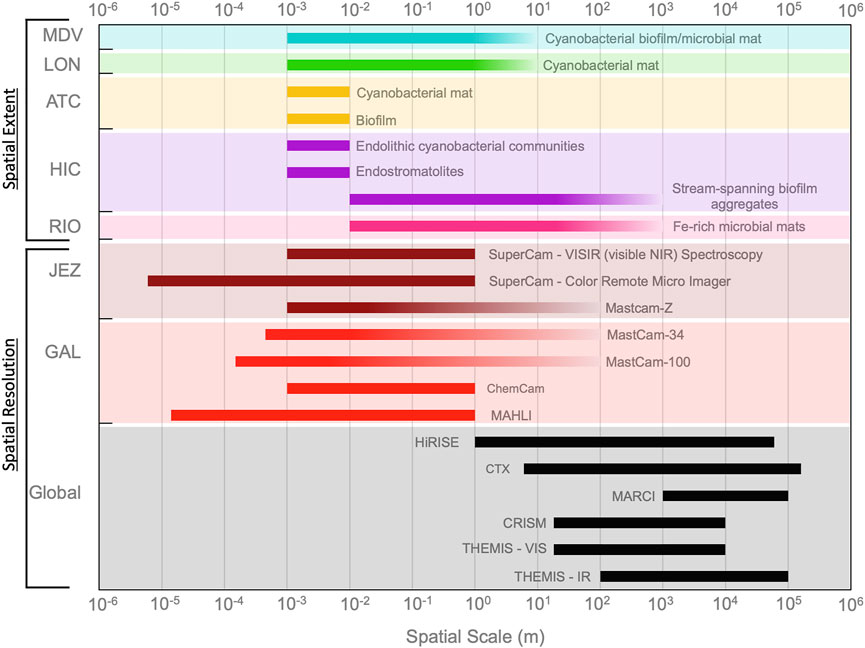
FIGURE 6. Spatial extent of macrobiological features at each analog site compared to the spatial resolution of imaging instrumentation on the Curiosity rover in Gale crater, the Perseverance rover in Jezero crater, and global data sets from the Mars Reconnaissance Orbiter and Mars Odyssey Orbiter. Rover-scale instrumentation would be required to spatially resolve the majority of macrobiological features at analog sites. See Supplementary Tables S1, S4 for details on Mars instruments and analog macrobiological features, respectively.
Because direct visual detection of active macrobiological features on Mars is unlikely, more research into understanding if characteristics of preserved microbial communities at these analog sites can be remotely sensed and how duration of preservation impacts observability, is critical. Remote sensing data spanning sub-cm to km spatial scales are necessary to determine what characteristics of modern and/or preserved microbial communities at terrestrial analogs can be detected. Environments where the presence and concentration of biological material has high spatial variability, such as Atacama Salars (Navarro-González et al., 2003; Connon et al., 2007; Crits-Christoph et al., 2013), McMurdo Dry Valleys (Power et al., 2020), and Haughton Impact Crater (Clarke et al., 2019), present a unique opportunity to develop and test methods for remotely mapping biosignatures (both their presence and spatial extent) at various spatial scales. Advancing the ability to remotely detect regions likely to harbor preserved biomarkers or characterize the spatial extent of ancient macrobiological features at terrestrial analog sites would be invaluable for informing landing site and sample selection on Mars (Bosak et al., 2021).
3.4.2 Spectroscopic Characterization of Mineralogy
Mineralogical information on Mars helps to constrain past formation environments and is a significant consideration in landing site selection (Golombek et al., 2012; Grant et al., 2011, 2018) and identification of rover targets (e.g., Fraeman et al., 2020). Mapping the surface mineralogy of Mars is accomplished via remote sensing at satellite- and rover-scales (e.g., Bibring et al., 2006; Ehlmann and Edwards, 2014; Johnson et al., 2015). In order to identify minerals and formation environments with higher biopreservation potential, characteristic spectral features are identified, and interpretation is informed by knowledge of mineralogy and biopreservation on Earth (see Bosak et al., 2021 and refs within). To optimize the environmental interpretation of remote spectral data, analog sites with well-characterized extant microbial communities and/or preserved biomarkers should be spectrally mapped at various scales with instruments similar to those on Mars (e.g. Flahaut et al., 2017).
Mineral formation environments on Earth are well-characterized and compiled into large libraries of mineral spectra (e.g., Kokaly et al., 2017). We compare visible and near infrared (VNIR) spectral characterizations of major mineral groups at each analog site with documented spectral coverage of select instrumentation on Mars (Figure 7). The wavelength range of Mars instruments encompasses all absorption features of the minerals at the analog sites. Several mineral groups have not yet been spectrally characterized in situ at analog sites, in some cases due to the challenges inherent to field-based spectral analysis [e.g., oxides at HIC appear transparent in the IR (Greenberger et al., 2020)]. MDV sediment samples from Lake Hoare were collected in the field and later analyzed with lab based VNIR spectroscopy (Bishop et al., 2014). While ancient biomarkers have not been detected in all mineral groups, several groups, including oxides and volcanics at ATC, hydrated silicas and carbonates at BON, and hydrated silicas at RIO, are known to host preserved biomarkers. It is critical to determine how mineral composition impacts biosignature preservation (Bosak et al., 2021), and in situ, field-based efforts are needed to characterize minerals that host preserved biomarkers based on their physical and optical properties, chemical composition, and crystal structure. If any characteristics indicative of high preservation potential can be remotely sensed, this finding could aid identification of targets on Mars.
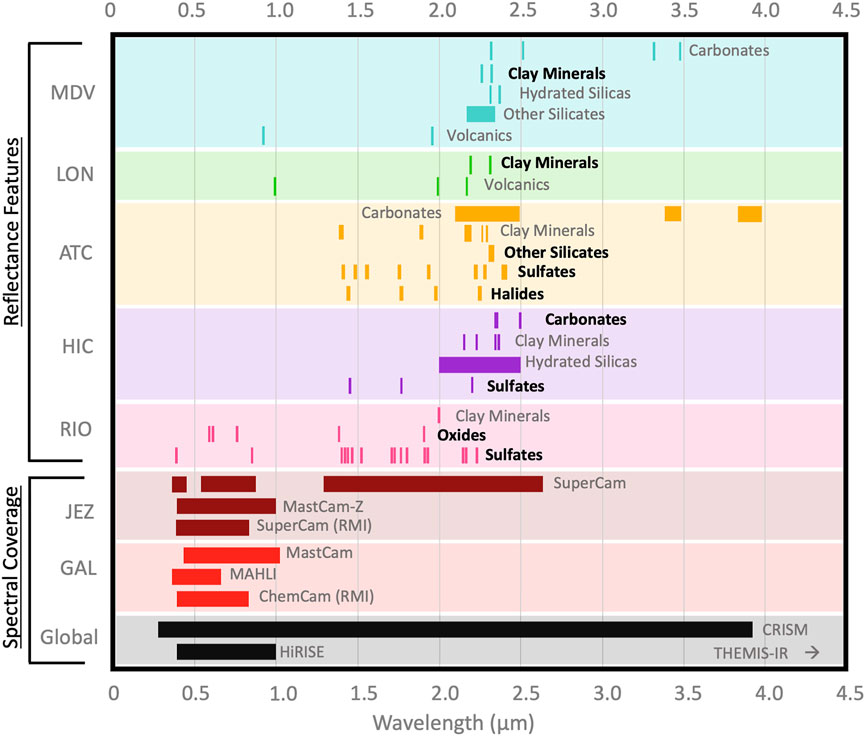
FIGURE 7. Characteristic spectral features (wavelength in um) of dominant mineral groups at terrestrial analogs measured with VNIR reflectance spectroscopy compared to the wavelength coverage of Mars-based passive imagers. Preserved lipid biomarkers have been detected in mineral groups with bolded names. Mineral spectra for the McMurdo Dry Valleys were analyzed in a laboratory (Bishop et al., 2014), spectra for all other analogs were determined remotely. Supplementary Tables S1,S3,S5 catalog relevant details on Mars instruments, lipid biomarkers, and mineral spectra, respectively.
Initial efforts to characterize mineralogy using field-based spectroscopy at rover-scales have been made at several analog sites (e.g., RIO: Roach et al., 2006; Sobron et al., 2014; ATC: Flahaut et al., 2017; Wang et al., 2018; HIC: Greenberger et al., 2020). In one study in Antarctica, mineralogy identified via remotely collected spectral data was calibrated with laboratory analyses, to allow more accurate determinations of mineral variations over an entire valley (∼20 km2) (Salvatore et al., 2014). The number of studies that couple mineralogical data across spatial scales remain limited, however. Given the dependence of Mars exploration on remote mapping of minerals to determine targets of biological interest, analog studies that couple biological and geological measurements across spatial scales (kilometer to sub-centimeter) (e.g., Warren-Rhodes et al., 2007; Power et al., 2020; Salvatore et al., 2020, 2021) are a priority.
In addition to passive sensing and VNIR spectra, Mars rovers have several active mineral characterization methods. Raman spectroscopy has been shown to be highly complementary with reflectance spectroscopy for target characterization (Cloutis et al., 2021), and Raman and XRD (e.g., Sobron et al., 2014; Flahaut et al., 2017), and X-ray fluorescence (e.g., Greenberger et al., 2020) have been applied to analog sites to calibrate and validate remote measurements of mineral compositions. These efforts are limited to smaller, rover-scale analyses and would be aided by remote observations at larger scales.
4 Conclusion and Future Priorities
In this review, we assessed the environmental conditions, geochemistry, microbial communities, and mineralogy of five terrestrial analogs that can inform biosignature preservation potential in different early Mars environments. We find that the polar desert analogs (HIC and BON), characterized by cold, neutral water with moderate salinity (<50 ppt) and a wide range of redox potentials, are most similar geochemically (and in terms of basin morphology and hydrology) to the Late Noachian to Early Hesperian Martian environment characterized at Gale crater (Fukushi et al., 2019). All analogs host polyextremophilic microbes that form microbial mats or biofilms, and these macrobiological features vary in spatial and temporal extent. At their maximum extent, these features would likely be unresolvable with satellite-scale observations (i.e., Mars Reconnaissance Orbiter and Mars Odyssey Orbiter) and would require rover-scale instrumentation for detection. The spatial threshold for remote detectability will vary based on the features morphology (i.e., percent cover, color, thickness) and season (i.e., perennial biofilms at RIO vs. biofilms in ephemeral melt-water streams at HIC and BON) and remains unconstrained due to the lack of studies that bridge rover- and orbital- spatial scales.
A thorough understanding of biosignature formation, preservation, and interpretation on Earth (Bosak et al., 2021) will increase the likelihood of detecting biosignatures (Neveu et al., 2018) and prevent false positives (McMahon and Cosmidis, 2021). While a variety of organic biomarkers have been detected at all analog sites, the influence of mineralogy and climate on preservation potential is complicated and remains unclear. These analog sites provide an excellent opportunity to better understand the chemistry of biomarker preservation within mineral matrices, especially over longer timescales (>106 years), and such research will provide insight into how diagenetic processes could potentially alter biosignatures in paleolake environments on Mars. This work would be especially useful if permits methods to be developed for remotely detecting minerals with high biomarker preservation potential.
Rover-scale instrumentation currently provides the best chance for in situ detection of biosignatures on Mars. Current (Curiosity and Perseverance) and proposed rover missions (Rosalind Franklin) will continue to improve and refine the search for biosignature detection on Mars (i.e., Martín-Torres et al., 2015; Kereszturi et al., 2016; Cuadros et al., 2022). Orbiter-scale data is used to determine rover site selection (e.g., Grant et al., 2018), however, and data products at all available spatial scales are coupled to characterize Martian environments. Despite the existence of rover- and orbiter-scale data at the terrestrial analogs reviewed here, few studies explicitly couple data products from these scales, and even fewer correlate these data to assess how remote sensing can be used to detect patterns of habitability (past or present) (e.g., soil wetness, pigment concentrations, organic carbon stocks, etc.). Concerted efforts to couple rover-scale environmental measurements with remotely sensed data in the Atacama and the McMurdo Dry Valleys (e.g. Warren-Rhodes et al., 2007 and Power et al., 2020, Salvatore et al., 2020, 2021, respectively), demonstrate the value of integrating data products from disparate spatial scales.
In order to best inform interpretation of Mars satellite and rover imagery, analog studies should correlate multi-scale remote sensing assessments of broad-scale mineralogy and pigment concentrations with other rover-scale, in situ observations of local geochemistry, extant microbial communities, and preserved biomarkers. The five analogs reviewed here provide an ideal range of environmental conditions in which to generate these multi-scale datasets. This research will help determine how biosignature preservation varies with mineralogy and environmental conditions, and at what scale these indicators can be remotely detected. The interpretive framework that will result from this work will be critical to the search for life in evaporative Martian basins (and other terrestrial bodies).
Author Contributions
MP and WL conceived of the initial review topic and lead discussions, all authors performed data collection, analysis and initial figure generation, CH, MM and MP wrote the paper, CH and MM made all final figures and tables and MP and WL commented on and edited the manuscript.
Funding
Funding for students was provided by: NASA NH Space Grant; NASA-SSW-80NSSC19K0539 (MP); NASA-MDAP-80NSSC21K1097 (MP); NASA 20-EXO20-0022 (WL); NSF-EAR 1928309 (WL); and the Simons Foundation Project #623881 (WL).
Conflict of Interest
The authors declare that the research was conducted in the absence of any commercial or financial relationships that could be construed as a potential conflict of interest.
Publisher’s Note
All claims expressed in this article are solely those of the authors and do not necessarily represent those of their affiliated organizations, or those of the publisher, the editors and the reviewers. Any product that may be evaluated in this article, or claim that may be made by its manufacturer, is not guaranteed or endorsed by the publisher.
Supplementary Material
The Supplementary Material for this article can be found online at: https://www.frontiersin.org/articles/10.3389/fspas.2022.849078/full#supplementary-material
References
Abbey, W. J., Bhartia, R., Beegle, L. W., DeFlores, L., Paez, V., Sijapati, K., et al. (2017). Deep UV Raman Spectroscopy for Planetary Exploration: The Search for In Situ Organics. Icarus 290, 201–214. doi:10.1016/j.icarus.2017.01.039
Abramov, O. (2004). Numerical Modeling of an Impact-Induced Hydrothermal System at the Sudbury Crater. J. Geophys. Res. 109, E10007. doi:10.1029/2003JE002213
Abrevaya, X. C., Anderson, R., Arney, G., Atri, D., Azúa-Bustos, A., Bowman, J. S., et al. (2016). The Astrobiology Primer v2.0. Astrobiology 16, 561–653. doi:10.1089/ast.2015.1460
Aerts, J. W., Riedo, A., Melton, D. J., Martini, S., Flahaut, J., Meierhenrich, U. J., et al. (2020). Biosignature Analysis of Mars Soil Analogs from the Atacama Desert: Challenges and Implications for Future Missions to Mars. Astrobiology 20, 766–784. doi:10.1089/ast.2019.2063
Aguilera, A., González-Toril, E., Souza-Egipsy, V., Amaral-Zettler, L., Zettler, E., and Amils, R. (2010). “Phototrophic Biofilms from Río Tinto, an Extreme Acidic Environment, the Prokaryotic Component,” in Microbial Mats Cellular Origin, Life in Extreme Habitats and Astrobiology. Editors J. Seckbach, and A. Oren (Dordrecht: Springer Netherlands), 469–481. doi:10.1007/978-90-481-3799-2_24
Amaral Zettler, L. A., Gómez, F., Zettler, E., Keenan, B. G., Amils, R., and Sogin, M. L. (2002). Eukaryotic Diversity in Spain's River of Fire. Nature 417, 137. doi:10.1038/417137a
Amils, R., Amils, R., Fernández-Remolar, D., Gómez, F., González-Toril, E., Rodríguez, N., et al. (2008). “Subsurface Geomicrobiology of the Iberian Pyritic Belt,” in Microbiology of Extreme Soils Soil Biology. Editors P. Dion, and C. S. Nautiyal (Berlin, Heidelberg: Springer Berlin Heidelberg), 205–223. doi:10.1007/978-3-540-74231-9_10
Amils, R., González-Toril, E., Fernández-Remolar, D., Gómez, F., Aguilera, Á., Rodríguez, N., et al. (2007). Extreme Environments as Mars Terrestrial Analogs: The Rio Tinto Case. Planet. Space Sci. 55, 370–381. doi:10.1016/j.pss.2006.02.006
Anderson, R., and Bell, J. F. (2010). Geologic Mapping and Characterization of Gale Crater and Implications for its Potential as a Mars Science Laboratory landing Site. Mars 5, 76–128. doi:10.1555/mars.2010.0004
Angino, E. E., Turner, M. D., and Zeller, E. J. (1962). RECONNAISSANCE GEOLOGY OF LOWER TAYLOR VALLEY, VICTORIA LAND, ANTARCTICA. Geol. Soc. Am. Bull. 73 (12), 1553–1561. doi:10.1130/0016-7606(1962)73[1553:RGOLTV]2.0.CO;2
Antony, C. P., Kumaresan, D., Hunger, S., Drake, H. L., Murrell, J. C., and Shouche, Y. S. (2013). Microbiology of Lonar Lake and Other Soda Lakes. ISME J. 7, 468–476.
Arias, D., Cisternas, L. A., and Rivas, M. (2017). Biomineralization of Calcium and Magnesium Crystals from Seawater by Halotolerant Bacteria Isolated from Atacama Salar (Chile). Desalination 405, 1–9. doi:10.1016/j.desal.2016.11.027
Armitage, K. B., and House, H. B. (1962). A Limnological Reconnaissance in the Area of Mcmurdo Sound, Antarctica1. Limnol. Oceanogr. 7, 36–41. doi:10.4319/lo.1962.7.1.0036
Arriagada, C., Cobbold, P. R., and Roperch, P. (2006). Salar de Atacama basin: A record of compressional tectonics in the central Andes since the mid-Cretaceous. Tectonics 25 (1), a–n. doi:10.1029/2004TC001770
Arvidson, R. E., Bell, J. F., Catalano, J. G., Clark, B. C., Fox, V. K., Gellert, R., et al. (2015). Mars Reconnaissance Orbiter and Opportunity Observations of the Burns Formation: Crater Hopping at Meridiani Planum. J. Geophys. Res. Planets 120, 429–451. doi:10.1002/2014JE004686
Azua-Bustos, A., Fairén, A. G., Silva, C. G., Carrizo, D., Fernández-Martínez, M. Á., Arenas-Fajardo, C., et al. (2020). Inhabited Subsurface Wet Smectites in the Hyperarid Core of the Atacama Desert as an Analog for the Search for Life on Mars. Sci. Rep. 10, 19183. doi:10.1038/s41598-020-76302-z
Beaty, D. W., Grady, M. M., McSween, H. Y., Sefton-Nash, E., Carrier, B. L., Altieri, F., et al. (2019). The Potential Science and Engineering Value of Samples Delivered to Earth by Mars Sample Return. Meteorit. Planet. Sci. 54, S3–S152. doi:10.1111/maps.13242
Beegle, L., Bhartia, R., White, M., DeFlores, L., Abbey, W., Yen-Hung Wu, Y.-H., et al. (2015). “SHERLOC: Scanning Habitable Environments with Raman & Luminescence for Organics & Chemicals,” in 2015 IEEE Aerospace Conference, 1–11. doi:10.1109/AERO.2015.7119105
Berner, R. A. (1989). Biogeochemical Cycles of Carbon and Sulfur and Their Effect on Atmospheric Oxygen over Phanerozoic Time. Glob. Planet. Change 1, 97–122. doi:10.1016/0921-8181(89)90018-0
Bibring, J.-P., Langevin, Y., Mustard, J. F., Poulet, F., Arvidson, R., Gendrin, A., et al. (2006). Global Mineralogical and Aqueous Mars History Derived from OMEGA/Mars Express Data. Science 312, 400–404. doi:10.1126/science.1122659
Bishop, J. L., Englert, P. A. J., Patel, S., Tirsch, D., Roy, A. J., Koeberl, C., et al. (2014). Mineralogical Analyses of Surface Sediments in the Antarctic Dry Valleys: Coordinated Analyses of Raman Spectra, Reflectance Spectra and Elemental Abundances. Phil. Trans. R. Soc. A. 372, 20140198. doi:10.1098/rsta.2014.0198
Bobst, A. L., Lowenstein, T. K., Jordan, T. E., Godfrey, L. V., Ku, T. L., and Luo, S. (2001). A 106ka Paleoclimate Record From Drill Core of the Salar de Atacama, Northern Chile. Palaeogeogr. Palaeoclimatol. Palaeoecol. 173, 21–42. doi:10.1016/S0031-0182(01)00308-X
Bontognali, T. R. R., Meister, Y., Kuhn, B., Josset, J.-L., Hofmann, B. A., and Kuhn, N. (2021). Identifying Optimal Working Conditions for Close-Up Imagining during the ExoMars Rover mission. Planet. Space Sci. 208, 105355. doi:10.1016/j.pss.2021.105355
Borlina, C. S., Ehlmann, B. L., and Kite, E. S. (2015). Modeling the thermal and Physical Evolution of Mount Sharp's Sedimentary Rocks, Gale Crater, Mars: Implications for Diagenesis on the MSL Curiosity Rover Traverse. J. Geophys. Res. Planets 120, 1396–1414. doi:10.1002/2015JE004799
Bosak, T., Moore, K. R., Gong, J., and Grotzinger, J. P. (2021). Searching for Biosignatures in Sedimentary Rocks from Early Earth and Mars. Nat. Rev. Earth Environ. 2, 490–506. doi:10.1038/s43017-021-00169-5
Boschetti, T., Cortecci, G., Barbieri, M., and Mussi, M. (2007). New and past geochemical data on fresh to brine waters of the Salar de Atacama and Andean Altiplano, northern Chile. Geofluids 7, 33–50. doi:10.1111/j.1468-8123.2006.00159.x
Boulter, C. A. (1993). Comparison of Rio Tinto, Spain, and Guaymas Basin, Gulf of California: An Explanation of a Supergiant Massive Sulfide deposit in an Ancient Sill-Sediment Complex. Geol 21, 801–804. doi:10.1130/0091-7613(1993)021<0801:cortsa>2.3.co;2
Breuer, D., and Spohn, T. (2003). Early Plate Tectonics versus Single-Plate Tectonics on Mars: Evidence from Magnetic Field History and Crust Evolution. J. Geophys. Res. 108. doi:10.1029/2002JE001999
Brocks, J. J., and Summons, R. E. (2003). “Sedimentary Hydrocarbons, Biomarkers for Early Life,” in Treatise on Geochemistry (Amsterdam, Netherlands: Elsevier), 63–115. doi:10.1016/B0-08-043751-6/08127-5
Brown, A. J., Viviano, C. E., and Goudge, T. A. (2020). Olivine‐Carbonate Mineralogy of the Jezero Crater Region. J. Geophys. Res. Planets 125 (3), e2019JE006011. doi:10.1029/2019JE006011
Cabrol, N. A., Bettis, E. A., Glenister, B., Chong, G., Herrera, C., Jensen, A., et al. (2012). Nomad Rover Field Experiment, Atacama Desert, Chile: 2. Identification of Paleolife Evidence Using a Robotic Vehicle: Lessons and Recommendations for a Mars Sample Return Mission. J. Geophys. Res. 106, 7807–7815. doi:10.1029/1999JE001181
Carr, M. H., and Head, J. W. (2010). Geologic History of Mars. Earth Planet. Sci. Lett. 294, 185–203. doi:10.1016/j.epsl.2009.06.042
Carr, M. H. (2012). The Fluvial History of Mars. Phil. Trans. R. Soc. A. 370, 2193–2215. doi:10.1098/rsta.2011.0500
Chacon-Baca, E., Santos, A., Sarmiento, A. M., Luís, A. T., Santisteban, M., Fortes, J. C., et al. (2021). Acid Mine Drainage as Energizing Microbial Niches for the Formation of Iron Stromatolites: The Tintillo River in Southwest Spain. Astrobiology 21, 443–463. doi:10.1089/ast.2019.2164
Changela, H. G., Chatzitheodoridis, E., Antunes, A., Beaty, D., Bouw, K., Bridges, J. C., et al. (2021). Mars: New Insights and Unresolved Questions. Int. J. Astrobiology 20, 394–426. doi:10.1017/S1473550421000276
Christensen, P. R., Jakosky, B. M., Kieffer, H. H., Malin, M. C., McSween, Jr., H. Y., Nealson, K., et al. (2004). The thermal Emission Imaging System (THEMIS) for the Mars 2001 Odyssey Mission. Space Sci. Rev. 110, 85–130. doi:10.1023/b:spac.0000021008.16305.94
Clarke, J. D. A. (2006). Antiquity of Aridity in the Chilean Atacama Desert. Geomorphology 73, 101–114. doi:10.1016/j.geomorph.2005.06.008
Clarke, J., Knightly, P., and Rupert, S. (2019). Melt-water Formed Dark Streaks on Slopes of Haughton Crater as Possible Mars Analogues. Int. J. Astrobiology 18, 518–526. doi:10.1017/S1473550418000526
Cloutis, E., Applin, D., Connell, S., Kubanek, K., Kuik, J., Parkinson, A., et al. (2021). A Simulated Rover Exploration of a Long-Lived Hypersaline spring Environment: The East German Creek (MB, Canada) Mars Analogue Site. Planet. Space Sci. 195, 105130. doi:10.1016/j.pss.2020.105130
Cockell, C. S., Lee, P., Osinski, G., Horneck, G., and Broady, P. (2002). Impact-induced Microbial Endolithic Habitats. Meteorit. Planet. Sci. 37, 1287–1298. doi:10.1111/j.1945-5100.2002.tb01029.x
Cockell, C. S., Lee, P., Schuerger, A. C., Hidalgo, L., Jones, J. A., and Stokes, M. D. (2001). Microbiology and Vegetation of Micro-Oases and Polar Desert, Haughton Impact Crater, Devon Island, Nunavut, Canada. Arct. Antarct. Alp. Res. 33 (3), 306–318.
Cockell, C. S., Osinski, G. R., and Lee, P. (2003). The Impact Crater as a Habitat: Effects of Impact Processing of Target Materials. Astrobiology 3, 181–191. doi:10.1089/153110703321632507
Connon, S. A., Lester, E. D., Shafaat, H. S., Obenhuber, D. C., and Ponce, A. (2007). Bacterial Diversity in Hyperarid Atacama Desert Soils. J. Geophys. Res. 112, a–n. doi:10.1029/2006JG000311
Craddock, R. A., and Howard, A. D. (2002). The Case for Rainfall on a Warm, Wet Early Mars. J.-Geophys.-Res. 107, 21–1. doi:10.1029/2001JE001505
Crits-Christoph, A., Robinson, C. K., Barnum, T., Fricke, W. F., Davila, A. F., Jedynak, B., et al. (2013). Colonization Patterns of Soil Microbial Communities in the Atacama Desert. Microbiome 1, 28. doi:10.1186/2049-2618-1-28
Cuadros, J., Michalski, J. R., Bishop, J. L., Mavris, C., Fiore, S., and Dekov, V. (2022). Mars-rover Cameras Evaluation of Laboratory Spectra of Fe-Bearing Mars Analog Samples. Icarus 371, 114704. doi:10.1016/j.icarus.2021.114704
Cubillos, C. F., Aguilar, P., Grágeda, M., and Dorador, C. (2018). Microbial Communities From the World's Largest Lithium Reserve, Salar de Atacama, Chile: Life at High LiCl Concentrations. J. Geophys. Res. Biogeosci. 123, 3668–3681. doi:10.1029/2018JG004621
Davila, A. F., Gómez-Silva, B., de los Rios, A., Ascaso, C., Olivares, H., McKay, C. P., et al. (2008). Facilitation of Endolithic Microbial Survival in the Hyperarid Core of the Atacama Desert by mineral Deliquescence. J. Geophys. Res. 113, a–n. doi:10.1029/2007JG000561
Davila, A. F., Willson, D., Coates, J. D., and McKay, C. P. (2013). Perchlorate on Mars: a Chemical hazard and a Resource for Humans. Int. J. Astrobiology 12, 321–325. doi:10.1017/S1473550413000189
Davis, R., Welty, A., Borrego, J., Morales, J., Pendon, J., and Ryan, J. G. (2001). Rio Tinto Estuary (Spain): 5000 Years of Pollution. Environ. Geol. 39, 1109–1116.
des Marais, D. J. (2010). Exploring Mars for Evidence of Habitable Environments and Life. Proc. Am. Philos. Soc. 154, 402–421.
Des Marais, D. J. (2013). “Planetary Climate and the Search for Life,” in Comparative Climatology of Terrestrial Planets (Tucson, Arizona, USA: University of Arizona Press). doi:10.2458/azu_uapress_9780816530595-ch24
Dorador, C., Fink, P., Hengst, M., Icaza, G., Villalobos, A. S., Vejar, D., et al. (2018). Microbial community composition and trophic role along a marked salinity gradient in Laguna Puilar, Salar de Atacama, Chile. Antonie Van Leeuwenhoek 111, 1361–1374. doi:10.1007/s10482-018-1091-z
Doran, P., and Fountain, A. (2021). Daily Measurement Summaries from Lake Bonney Meteorological Station (BOYM), McMurdo Dry Valleys, Antarctica (1993-2019, Ongoing). Environ. Data Initiat.doi:10.6073/PASTA/E95A3CD4D463A3EE37D590CF878C73AD
Doran, P., and Gooseff, M. (2012). McMurdo Dry Valleys LTER: Lake Level Surveys in the McMurdo Dry Valleys, Antarctica From 1991 to Present. doi:10.6073/PASTA/3D0E0C4D844792D9964DD4179A3D83AA
Doran, P. T., McKay, C. P., Clow, G. D., Dana, G. L., Fountain, A. G., Nylen, T., et al. (2002). Valley Floor Climate Observations from the McMurdo Dry Valleys, Antarctica, 1986-2000. J.‐Geophys.‐Res. 107. doi:10.1029/2001JD002045
Doran, P. T., Wharton, R. A., Des Marais, D. J., and McKay, C. P. (1998). Antarctic Paleolake Sediments and the Search for Extinct Life on Mars. J. Geophys. Res. Planets 103, 28481–28493. doi:10.1029/98JE01713
Doran, P. T., Wharton, R. A., and Lyons, W. B. (1994). Paleolimnology of the McMurdo Dry Valleys, Antarctica. J. Paleolimnol. 10, 85–114. doi:10.1007/BF00682507
dos Santos, R., Patel, M., Cuadros, J., and Martins, Z. (2016). Influence of Mineralogy on the Preservation of Amino Acids under Simulated Mars Conditions. Icarus 277, 342–353. doi:10.1016/j.icarus.2016.05.029
Dundar, M., Ehlmann, B. L., and Leask, E. (2019). Machine-Learning-Driven New Geologic Discoveries at Mars Rover Landing Sites: Jezero Crater and NE Syrtis. Planetology. doi:10.1002/essoar.10501294.1
Edwards, H. G. M., Vandenabeele, P., Jorge-Villar, S. E., Carter, E. A., Perez, F. R., and Hargreaves, M. D. (2007). The Rio Tinto Mars Analogue Site: An Extremophilic Raman Spectroscopic Study. Spectrochimica Acta A: Mol. Biomol. Spectrosc. 68, 1133–1137. doi:10.1016/j.saa.2006.12.080
Ehlmann, B. L., and Edwards, C. S. (2014). Mineralogy of the Martian Surface. Annu. Rev. Earth Planet. Sci. 42, 291–315. doi:10.1146/annurev-earth-060313-055024
Ehlmann, B. L., Mustard, J. F., Fassett, C. I., Schon, S. C., Head III, J. W., Des Marais, D. J., et al. (2008). Clay Minerals in delta Deposits and Organic Preservation Potential on Mars. Nat. Geosci 1, 355–358. doi:10.1038/ngeo207
Ericksen, G. E. (1983). The Chilean Nitrate Deposits: The Origin of the Chilean Nitrate Deposits, Which Contain a Unique Group of saline Minerals, Has Provoked Lively Discussion for More Than 100 Years. Am. Sci. 71, 366–374.
Ewing, S. A., Macalady, J. L., Warren-Rhodes, K., McKay, C. P., and Amundson, R. (2008). Changes in the Soil C Cycle at the Arid-Hyperarid Transition in the Atacama Desert. J. Geophys. Res. 113, a–n. doi:10.1029/2007JG000495
Fagliarone, C., Napoli, A., Chiavarini, S., Baqué, M., de Vera, J.-P., and Billi, D. (2020). Biomarker Preservation and Survivability under Extreme Dryness and Mars-Like UV Flux of a Desert Cyanobacterium Capable of Trehalose and Sucrose Accumulation. Front. Astron. Space Sci. 7, 31. doi:10.3389/fspas.2020.00031
Fairén, A. G., Davila, A. F., Lim, D., Bramall, N., Bonaccorsi, R., Zavaleta, J., et al. (2010). Astrobiology through the Ages of Mars: The Study of Terrestrial Analogues to Understand the Habitability of Mars. Astrobiology 10, 821–843. doi:10.1089/ast.2009.0440
Farías, M. E., Contreras, M., Rasuk, M. C., Kurth, D., Flores, M. R., Poiré, D. G., et al. (2014). Characterization of bacterial diversity associated with microbial mats, gypsum evaporites and carbonate microbialites in thalassic wetlands: Tebenquiche and La Brava, Salar de Atacama, Chile. Extremophiles 18 (2), 311–329. doi:10.1007/s00792-013-0617-6
Farías, M. E. (2020). Microbial Ecosystems in Central Andes Extreme Environments: Biofilms, Microbial Mats, Microbialites and Endoevaporites. Berlin, Germany: Springer Nature.
Farías, M. E., and Saona Acuña, L. A. (2020). “Modern Microbial Mats and Endoevaporite Systems in Andean Lakes: A General Approach,” in Microbial Ecosystems in Central Andes Extreme Environments, 21–33. doi:10.1007/978-3-030-36192-1_2
Farley, K. A., Malespin, C., Mahaffy, P., Grotzinger, J. P., Vasconcelos, P. M., Milliken, R. E., et al. (2014). In Situ Radiometric and Exposure Age Dating of the Martian Surface. Science 343, 1247166. doi:10.1126/science.1247166
Farley, K. A., Williford, K. H., Stack, K. M., Bhartia, R., Chen, A., de la Torre, M., et al. (2020). Mars 2020 Mission Overview. Space Sci. Rev. 216, 142. doi:10.1007/s11214-020-00762-y
Fassett, C. I., and Head, J. W. (2008a). The Timing of Martian valley Network Activity: Constraints from Buffered Crater Counting. Icarus 195, 61–89. doi:10.1016/j.icarus.2007.12.009
Fassett, C. I., and Head, J. W. (2008b). Valley Network-Fed, Open-basin Lakes on Mars: Distribution and Implications for Noachian Surface and Subsurface Hydrology. Icarus 198, 37–56. doi:10.1016/j.icarus.2008.06.016
Fernandez, A. B., Rasuk, M. C., Visscher, P. T., Contreras, M., Novoa, F., Poire, D. G., et al. (2016). Microbial Diversity in Sediment Ecosystems (Evaporites Domes, Microbial Mats, and Crusts) of Hypersaline Laguna Tebenquiche, Salar de Atacama, Chile. Front. Microbiol. 7. doi:10.3389/fmicb.2016.01284
Fernández-Remolar, D. C., Chong-Díaz, G., Ruíz-Bermejo, M., Harir, M., Schmitt-Kopplin, P., Tziotis, D., et al. (2013). Molecular Preservation in Halite- and Perchlorate-Rich Hypersaline Subsurface Deposits in the Salar Grande basin (Atacama Desert, Chile): Implications for the Search for Molecular Biomarkers on Mars. J. Geophys. Res. Biogeosci. 118, 922–939. doi:10.1002/jgrg.20059
Fernández-Remolar, D. C., and Knoll, A. H. (2008). Fossilization Potential of Iron-Bearing Minerals in Acidic Environments of Rio Tinto, Spain: Implications for Mars Exploration. Icarus 194, 72–85. doi:10.1016/j.icarus.2007.10.009
Fernández-Remolar, D. C., Morris, R. V., Gruener, J. E., Amils, R., and Knoll, A. H. (2005). The Río Tinto Basin, Spain: Mineralogy, Sedimentary Geobiology, and Implications for Interpretation of Outcrop Rocks at Meridiani Planum, Mars. Earth Planet. Sci. Lett. 240, 149–167. doi:10.1016/j.epsl.2005.09.043
Ferris, F., Hallbeck, L., Kennedy, C., and Pedersen, K. (2004). Geochemistry of Acidic Rio Tinto Headwaters and Role of Bacteria in Solid Phase Metal Partitioning. Chem. Geol. 212, 291–300.
Flahaut, J., Martinot, M., Bishop, J. L., Davies, G. R., and Potts, N. J. (2017). Remote Sensing and In Situ Mineralogic Survey of the Chilean Salars: An Analog to Mars Evaporate Deposits? Icarus 282, 152–173. doi:10.1016/j.icarus.2016.09.041
Forsberg-Taylor, N. K., Howard, A. D., and Craddock, R. A. (2004). Crater Degradation in the Martian highlands: Morphometric Analysis of the Sinus Sabaeus Region and Simulation Modeling Suggest Fluvial Processes. J. Geophys. Res. 109, 1–12. doi:10.1029/2004JE002242
Fountain, A. G., Lyons, W. B., Burkins, M. B., Dana, G. L., Doran, P. T., Lewis, K. J., et al. (1999). Physical Controls on the Taylor Valley Ecosystem, Antarctica. BioScience 49, 961. doi:10.2307/1313730
Fraeman, A. A., Arvidson, R. E., Catalano, J. G., Grotzinger, J. P., Morris, R. V., Murchie, S. L., et al. (2013). A Hematite-Bearing Layer in Gale Crater, Mars: Mapping and Implications for Past Aqueous Conditions. Geology 41, 1103–1106. doi:10.1130/G34613.1
Fraeman, A. A., Edgar, L. A., Rampe, E. B., Thompson, L. M., Frydenvang, J., Fedo, C. M., et al. (2020). Evidence for a Diagenetic Origin of Vera Rubin Ridge, Gale Crater, Mars: Summary and Synthesis of Curiosity 's Exploration Campaign. J. Geophys. Res. Planets 125, e2020JE006527. doi:10.1029/2020JE006527
Fukushi, K., Sekine, Y., Sakuma, H., Morida, K., and Wordsworth, R. (2019). Semiarid Climate and Hyposaline lake on Early Mars Inferred from Reconstructed Water Chemistry at Gale. Nat. Commun. 10, 4896. doi:10.1038/s41467-019-12871-6
García-Moyano, A., González-Toril, E., Aguilera, A., and Amils, R. (2007). Prokaryotic Community Composition and Ecology of Floating Macroscopic Filaments From an Extreme Acidic Environment, Rio Tinto (SW, Spain). Syst. Appl. Microbiol. 30, 601–614.
Georgiou, C. D., and Deamer, D. W. (2014). Lipids as Universal Biomarkers of Extraterrestrial Life. Astrobiology 14, 541–549. doi:10.1089/ast.2013.1134
Goesmann, F., Brinckerhoff, W. B., Raulin, F., Goetz, W., Danell, R. M., Getty, S. A., et al. (2017). The Mars Organic Molecule Analyzer (MOMA) Instrument: Characterization of Organic Material in Martian Sediments. Astrobiology 17, 655–685. doi:10.1089/ast.2016.1551
Golombek, M., Grant, J., Kipp, D., Vasavada, A., Kirk, R., Fergason, R., et al. (2012). Selection of the Mars Science Laboratory Landing Site. Space Sci. Rev. 170, 641–737. doi:10.1007/s11214-012-9916-y
Golombek, M., Kipp, D., Warner, N., Daubar, I. J., Fergason, R., Kirk, R. L., et al. (2017). Selection of the InSight Landing Site. Space Sci. Rev. 211, 5–95. doi:10.1007/s11214-016-0321-9
Gómez Gómez, F., Grau Carles, A., Vazquez, L., and Amils, R. (2004). UV Radiation Effects over Microorganisms and Study of Protective Agents. Evol. Life 545, 21–25.
Goodwin, A., and Papineau, D. (2022). Biosignatures Associated with Organic Matter in Late Paleoproterozoic Stromatolitic Dolomite and Implications for Martian Carbonates. Astrobiology 22, 49–74. doi:10.1089/ast.2021.0010
Gooseff, M. N., McKnight, D. M., Doran, P., Fountain, A. G., and Lyons, W. B. (2011). Hydrological Connectivity of the Landscape of the McMurdo Dry Valleys, Antarctica. Geogr. Compass 5, 666–681. doi:10.1111/j.1749-8198.2011.00445.x
Goudge, T. A., Mustard, J. F., Head, J. W., Fassett, C. I., and Wiseman, S. M. (2015). Assessing the Mineralogy of the Watershed and Fan Deposits of the Jezero Crater Paleolake System, Mars. J. Geophys. Res. Planets. doi:10.1002/2014JE004782.Received
Grant, J. A., Golombek, M. P., Grotzinger, J. P., Wilson, S. A., Watkins, M. M., Vasavada, A. R., et al. (2011). The Science Process for Selecting the landing Site for the 2011 Mars Science Laboratory. Planet. Space Sci. 59, 1114–1127. doi:10.1016/j.pss.2010.06.016
Grant, J. A., Golombek, M. P., Wilson, S. A., Farley, K. A., Williford, K. H., and Chen, A. (2018). The Science Process for Selecting the landing Site for the 2020 Mars Rover. Planet. Space Sci. 164, 106–126. doi:10.1016/j.pss.2018.07.001
Grant, J. A., and Wilson, S. A. (2012). A Possible Synoptic Source of Water for Alluvial Fan Formation in Southern Margaritifer Terra, Mars. Planet. Space Sci. 72, 44–52. doi:10.1016/j.pss.2012.05.020
Greenberger, R. N., Ehlmann, B. L., Osinski, G. R., Tornabene, L. L., and Green, R. O. (2020). Compositional Heterogeneity of Impact Melt Rocks at the Haughton Impact Structure, Canada: Implications for Planetary Processes and Remote Sensing. J. Geophys. Res. Planets 125. doi:10.1029/2019JE006218
Grotzinger, J. P., Sumner, D. Y., Kah, L. C., Stack, K., Gupta, S., Edgar, L., et al. (2014). A Habitable Fluvio-Lacustrine Environment at Yellowknife Bay, Gale Crater, Mars. Science 343, 1242777. doi:10.1126/science.1242777
Grotzinger, J. P., Crisp, J., Vasavada, A. R., Anderson, R. C., Baker, C. J., Barry, R., et al. (2012). Mars Science Laboratory Mission and Science Investigation. Space Sci. Rev. 170, 5–56. doi:10.1007/s11214-012-9892-2
Grotzinger, J. P., Gupta, S., Malin, M. C., Rubin, D. M., Schieber, J., Siebach, K., et al. (2015). Deposition, Exhumation, and Paleoclimate of an Ancient lake deposit, Gale Crater, Mars. Science 350, aac7575. doi:10.1126/science.aac7575
Häder, D.-P., and Cabrol, N. A. (2018). “UV and Life Adaptation Potential on Early Mars: Lessons From Extreme Terrestrial Analogs,” in From Habitability to Life on Mars (Elsevier), 233–248.
Hagerty, J. J., and Newsom, H. E. (2003). Hydrothermal Alteration at the Lonar Lake Impact Structure, India: Implications for Impact Cratering on Mars. Meteorit. Planet. Sci. 38, 365–381. doi:10.1111/j.1945-5100.2003.tb00272.x
Halevy, I., Fischer, W. W., and Eiler, J. M. (2011). Carbonates in the Martian Meteorite Allan Hills 84001 Dormed at 18 4 C in a Near-Surface Aqueous Environment. Proc. Natl. Acad. Sci. U.S.A. 108, 16895–16899. doi:10.1073/pnas.1109444108
Hall, B. L., Denton, G. H., Heath, S. L., Jackson, M. S., and Koffman, T. N. B. (2015). Accumulation and Marine Forcing of Ice Dynamics in the Western Ross Sea During the Last Deglaciation. Nat. Geosci. 8, 625–628. doi:10.1038/ngeo2478
Hamilton, V. E., Vasavada, A. R., Sebastián, E., de la Torre Juárez, M., Ramos, M., Armiens, C., et al. (2014). Observations and Preliminary Science Results from the First 100 Sols of MSL Rover Environmental Monitoring Station Ground Temperature Sensor Measurements at Gale Crater. J. Geophys. Res. Planets 119, 745–770. doi:10.1002/2013JE004520
Hassler, D. M., Zeitlin, C., Wimmer-Schweingruber, R. F., Ehresmann, B., Rafkin, S., Eigenbrode, J. L., et al. (2012). Mars’ Surface Radiation Environment Measured with the Mars Science Laboratory’s Curiosity Rover. Sci. 343, 1244797. doi:10.1126/science.1244797
Hauber, E., Platz, T., Reiss, D., Le Deit, L., Kleinhans, M. G., Marra, W. A., et al. (2013). Asynchronous Formation of Hesperian and Amazonian-Aged Deltas on Mars and Implications for Climate. J. Geophys. Res. Planets 118, 1529–1544. doi:10.1002/jgre.20107
Hawes, I., Jungblut, A. D., Elster, J., Van de Vijver, B., and Mukucki, J. (2019). Inland Aquatic Biodiversity in Antarctica. Antarct. Environ. Portal Inland Aquat. Environments. doi:10.18124/439s-wk64
Hays, L. E., Graham, H. V., Des Marais, D. J., Hausrath, E. M., Horgan, B., McCollom, T. M., et al. (2017). Biosignature Preservation and Detection in Mars Analog Environments. Astrobiology 17, 363–400. doi:10.1089/ast.2016.1627
Hendy, C. H. (2000). Late Quaternary Lakes in the Mcmurdo sound Region of Antarctica. Geogr. Ann. A: Phys. Geogr. 82, 411–432.
Hendy, C. H., Healy, T. R., Rayner, E. M., Shaw, J., and Wilson, A. T. (1979). Late Pleistocene Glacial Chronology of the Taylor Valley, Antarctica, and the Global Climate. Quat. Res. 11, 172–184. doi:10.1016/0033-5894(79)90002-4
Hendy, C. H., Wilson, A. T., Popplewell, K. B., and House, D. A. (1977). Dating of Geochemical Events in Lake Bonney, Antarctica, and Their Relation to Glacial and Climate Changes. New Zealand J. Geol. Geophys. 20, 1103–1122. doi:10.1080/00288306.1977.10420698
Hickey, L. J., Johnson, K. R., and Dawson, M. R. (1988). The Stratigraphy, Sedimentology, and Fossils of the Haughton Formation: A Post-Impact Crater-Fill, Devon Island, N.W.T., Canada. Meteoritics 23, 221–231. doi:10.1111/j.1945-5100.1988.tb01284.x
Holo, S. J., Kite, E. S., and Robbins, S. J. (2018). Mars Obliquity History Constrained by Elliptic Crater Orientations. Earth Planet. Sci. Lett. 496, 206–214. doi:10.1016/j.epsl.2018.05.046
Horgan, B. (2016). Strategies for Searching for Biosignatures in Ancient Martian Sub-aerial Surface Environments. Biosignat Preserv Detect. Mars Analog Env 7463.
Horvath, D. G., and Andrews-Hanna, J. C. (2017). Reconstructing the Past Climate at Gale Crater, Mars, from Hydrological Modeling of Late-Stage Lakes. Geophys. Res. Lett. 44, 8196–8204. doi:10.1002/2017GL074654
Horvath, D. G., and Andrews-Hanna, J. C. (2021). The Hydrology and Climate of Mars during the Sedimentary Infilling of Gale Crater. Earth Planet. Sci. Lett. 568, 117032. doi:10.1016/j.epsl.2021.117032
Hubbard, C. G., Black, S., and Coleman, M. L. (2009). Aqueous Geochemistry and Oxygen Isotope Compositions of Acid Mine Drainage from the Río Tinto, SW Spain, Highlight Inconsistencies in Current Models. Chem. Geology. 265, 321–334. doi:10.1016/j.chemgeo.2009.04.009
Hurowitz, J. A., Grotzinger, J. P., Fischer, W. W., McLennan, S. M., Milliken, R. E., Stein, N., et al. (2017). Redox Stratification of an Ancient lake in Gale Crater, Mars. Science 356. doi:10.1126/science.aah6849
Irwin, R. P., Howard, A. D., Craddock, R. A., and Moore, J. M. (2005). An Intense Terminal Epoch of Widespread Fluvial Activity on Early Mars: 2. Increased Runoff and Paleolake Development. J. Geophys. Res. 110, E12S15. doi:10.1029/2005JE002460
Jackson, W. A., Davila, A. F., Estrada, N., Berry Lyons, W., Coates, J. D., and Priscu, J. C. (2012). Perchlorate and Chlorate Biogeochemistry in Ice-Covered Lakes of the McMurdo Dry Valleys, Antarctica. Geochimica et Cosmochimica Acta 98, 19–30. doi:10.1016/j.gca.2012.09.014
Jakosky, B. M., and Shock, E. L. (1998). The Biological Potential of Mars, the Early Earth, and Europa. J. Geophys. Res. 103, 19359–19364. doi:10.1029/98JE01892
Jelen, B. I., Giovannelli, D., and Falkowski, P. G. (2016). The Role of Microbial Electron Transfer in the Coevolution of the Biosphere and Geosphere. Annu. Rev. Microbiol. 70, 45–62. doi:10.1146/annurev-micro-102215-095521
Johnson, J. R., Bell, J. F., Bender, S., Blaney, D., Cloutis, E., DeFlores, L., et al. (2015). ChemCam Passive Reflectance Spectroscopy of Surface Materials at the Curiosity landing Site, Mars. Icarus 249, 74–92. doi:10.1016/j.icarus.2014.02.028
Jordan, T. E., Kirk-Lawlor, N. E., Blanco, N. P., Rech, J. A., and Cosentino, N. J. (2014). Landscape Modification in Response to Repeated Onset of Hyperarid Paleoclimate States since 14 Ma, Atacama Desert, Chile. Geol. Soc. America Bull. 126, 1016–1046. doi:10.1130/B30978.1
Jourdan, F., Moynier, F., Koeberl, C., and Eroglu, S. (2011). 40Ar/39Ar Age of the Lonar Crater and Consequence for the Geochronology of Planetary Impacts. Geology 39, 671–674. doi:10.1130/G31888.1
Kampf, S. K., Tyler, S. W., Ortiz, C. A., Muñoz, J. F., and Adkins, P. L. (2005). Evaporation and Land Surface Energy Budget at the Salar de Atacama, Northern Chile. J. Hydrol. 310, 236–252. doi:10.1016/j.jhydrol.2005.01.005
Karunatillake, S., Wray, J. J., Gasnault, O., McLennan, S. M., Rogers, A. D., Squyres, S. W., et al. (2014). Sulfates Hydrating Bulk Soil in the Martian Low and Middle Latitudes: Sulfates May Hydrate Bulk Soil on Mars. Geophys. Res. Lett. 41, 7987–7996. doi:10.1002/2014GL061136
Kereszturi, A., Bradak, B., Chatzitheodoridis, E., and Ujvari, G. (2016). Indicators and Methods to Understand Past Environments from ExoMars Rover Drills. Orig Life Evol. Biosph. 46, 435–454. doi:10.1007/s11084-016-9492-3
Kereszturi, A. (2011). Geologic Field Work on Mars: Distance and Time Issues during Surface Exploration. Acta Astronautica 68, 1686–1701. doi:10.1016/j.actaastro.2010.11.008
Kereszturi, A. (2012a). Landing Site Rationality Scaling for Subsurface Sampling on Mars-Case Study for ExoMars Rover-like Missions. Planet. Space Sci. 72, 78–90. doi:10.1016/j.pss.2012.07.007
Kereszturi, A., and Petrik, A. (2020). Age Determination for valley Networks on Mars Using Tectonic-Fluvial Interaction. Planet. Space Sci. 180, 104754. doi:10.1016/j.pss.2019.104754
Kereszturi, A. (2012b). Review of Wet Environment Types on Mars with Focus on Duration and Volumetric Issues. Astrobiology 12, 586–600. doi:10.1089/ast.2011.0686
Keys, H. J. R. (1980). Air Temperature, Wind, Precipitation and Atmospheric Humidity in the McMurdo Region, Antarctica. Department of Geology Publication No. 17 Antarctic Data Series Volume 7 Available at: https://www.wgtn.ac.nz/antarctic/publications/antarctic-data-series/ADS10.pdf (Accessed December 23, 2021).
Kohler, T. J., Van Horn, D. J., Darling, J. P., Takacs-Vesbach, C. D., and McKnight, D. M. (2016). Nutrient Treatments Alter Microbial Mat Colonization in Two Glacial Meltwater Streams from the McMurdo Dry Valleys, Antarctica. FEMS Microbiol. Ecol. 92, fiw049. doi:10.1093/femsec/fiw049
Kokaly, R. F., Clark, R. N., Swayze, G. A., Livo, K. E., Hoefen, T. M., Pearson, N. C., et al. (2017). USGS Spectral Library Version 7. Reston, VA: U.S. Geological Survey Data Series. doi:10.3133/ds1035
Komatsu, G., Senthil Kumar, P., Goto, K., Sekine, Y., Giri, C., and Matsui, T. (2014). Drainage Systems of Lonar Crater, India: Contributions to Lonar Lake Hydrology and Crater Degradation. Planet. Space Sci. 95, 45–55. doi:10.1016/j.pss.2013.05.011
Kring, D. A., Tikoo, S. M., Schmieder, M., Riller, U., Rebolledo-Vieyra, M., Simpson, S. L., et al. (2020). Probing the Hydrothermal System of the Chicxulub Impact Crater. Sci. Adv. 6, eaaz3053. doi:10.1126/sciadv.aaz3053
Kumar, P. S. (2005). Structural Effects of Meteorite Impact on basalt: Evidence from Lonar Crater, India. J. Geophys. Res. 110, B12402. doi:10.1029/2005JB003662
Lacelle, D., Juneau, V., Pellerin, A., Lauriol, B., and Clark, I. D. (2008). Weathering Regime and Geochemical Conditions in a Polar Desert Environment, Haughton Impact Structure Region, Devon Island, Canada. Can. J. Earth Sci. 45, 1139–1157. doi:10.1139/E08-063
Lacelle, D., Pellerin, A., Clark, I. D., Lauriol, B., and Fortin, D. (2009). (Micro)morphological, Inorganic-Organic Isotope Geochemisty and Microbial Populations in Endostromatolites (Cf. Fissure Calcretes), Haughton Impact Structure, Devon Island, Canada: The Influence of Geochemical Pathways on the Preservation of Isotope Biomarkers. Earth Planet. Sci. Lett. 281, 202–214. doi:10.1016/j.epsl.2009.02.022
Lane, N., Allen, J. F., and Martin, W. (2010). How Did LUCA Make a Living? Chemiosmosis in the Origin of Life. BioEssays 32, 271–280. doi:10.1002/bies.200900131
Laskar, J., Robutel, P., Joutel, F., Gastineau, M., Correia, A. C. M., and Levrard, B. (2004). A Long-Term Numerical Solution for the Insolation Quantities of the Earth. A&A 428, 261–285. doi:10.1051/0004-6361:20041335
Laybourn-Parry, J., and Wadham, J. L. (2014). Antarctic Lakes. Oxford: Oxford University Press. doi:10.1093/acprof:oso/9780199670499.001.0001
Lechner, A. M., Stein, A., Jones, S. D., and Ferwerda, J. G. (2009). Remote Sensing of Small and Linear Features: Quantifying the Effects of Patch Size and Length, Grid Position and Detectability on Land Cover Mapping. Remote Sensing Environ. 113, 2194–2204. doi:10.1016/j.rse.2009.06.002
Lee, P. (2002). Mars on Earth: The NASA Naughton-Mars Project. Ad Astera: The Magazine of the National Space Society.
Lee, P. A., Mikucki, J. A., Foreman, C. M., Priscu, J. C., DiTullio, G. R., Riseman, S. F., et al. (2004). Thermodynamic Constraints on Microbially Mediated Processes in Lakes of the McMurdo Dry Valleys, Antarctica. Geomicrobiol. J. 21, 221–237. doi:10.1080/01490450490275884
Lee, P., and Osinski, G. R. (2005). The Haughton-Mars Project: Overview of Science Investigations at the Haughton Impact Structure and Surrounding Terrains, and Relevance to Planetary Studies. Meteorit. Planet Sci. 40, 1755–1758. doi:10.1111/j.1945-5100.2005.tb00144.x
Léveillé, R. (2007). “Formation of Jarosite and Mars-like Minerals in a Polar Desert: Implications for Mars Aqueous Geochemistry,” in Geological Society of America, Annual Meeting, Abstract with Program, 284.
Levy, J. S., Fountain, A. G., Welch, K. A., and Lyons, W. B. (2012). Hypersaline “wet patches” in Taylor Valley, Antarctica. Geophys. Res. Lett. 39. doi:10.1029/2012GL050898
Lindgren, P., Parnell, J., Bowden, S., Taylor, C., Osinski, G. R., and Lee, P. (2009). Preservation of Biological Markers in Clasts within Impact Melt Breccias from the Haughton Impact Structure, Devon Island. Astrobiology 9, 391–400. doi:10.1089/ast.2008.0270
Lowenstein, T. K., and Risacher, F. (2009). Closed Basin Brine Evolution and the Influence of Ca-Cl Inflow Waters: Death Valley and Bristol Dry Lake California, Qaidam Basin, China, and Salar de Atacama, Chile. Aquat. Geochem. 15, 71–94. doi:10.1007/s10498-008-9046-z
Lüders, V., and Rickers, K. (2004). Fluid Inclusion Evidence for Impact-Related Hydrothermal Fluid and Hydrocarbon Migration in Creataceous Sediments of the ICDP-Chicxulub Drill Core Yax-1. Meteoritics Planet. Sci. 39, 1187–1197. doi:10.1111/j.1945-5100.2004.tb01136.x
Lyons, B., and Welch, K. (2016). McMurdo Dry Valleys Limnological Chemistry, Ion Concentrations and Silicon. doi:10.6073/PASTA/2CBB9E62342BDF6118B20553BE7B922F
Mahaffy, P. R., Webster, C. R., Cabane, M., Conrad, P. G., Coll, P., Atreya, S. K., et al. (2012). The Sample Analysis at Mars Investigation and Instrument Suite. Space Sci. Rev. 170, 401–478. doi:10.1007/s11214-012-9879-z
Malin, M. C., Bell, J. F., Cantor, B. A., Caplinger, M. A., Calvin, W. M., Clancy, R. T., et al. (2007). Context Camera Investigation on Board the Mars Reconnaissance Orbiter. J. Geophys. Res. Planets 112. doi:10.1029/2006je002808
Malin, M. C., and Edgett, K. S. (2000). Sedimentary Rocks of Early Mars. Science 290, 1927–1937. doi:10.1126/science.290.5498.1927
Maloof, A. C., Stewart, S. T., Weiss, B. P., Soule, S. A., Swanson-Hysell, N. L., Louzada, K. L., et al. (2010). Geology of Lonar Crater, India. Geol. Soc. America Bull. 122, 109–126. doi:10.1130/B26474.1
Mandon, L., Parkes Bowen, A., Quantin-Nataf, C., Bridges, J. C., Carter, J., Pan, L., et al. (2021). Morphological and Spectral Diversity of the Clay-Bearing Unit at the ExoMars Landing Site Oxia Planum. Astrobiology 21, 464–480. doi:10.1089/ast.2020.2292
Mangold, N., Dromart, G., Ansan, V., Salese, F., Kleinhans, M. G., Massé, M., et al. (2020). Fluvial Regimes, Morphometry, and Age of Jezero Crater Paleolake Inlet Valleys and Their Exobiological Significance for the 2020 Rover Mission Landing Site. Astrobiology 20, 994–1013. doi:10.1089/ast.2019.2132
Mangold, N., Gupta, S., Gasnault, O., Dromart, G., Tarnas, J. D., Sholes, S. F., et al. (2021). Perseverance Rover Reveals an Ancient delta-lake System and Flood Deposits at Jezero Crater, Mars. Science 374, 711–717. doi:10.1126/science.abl4051
Martín-Torres, F. J., Zorzano, M.-P., Valentín-Serrano, P., Harri, A.-M., Genzer, M., Kemppinen, O., et al. (2015). Transient Liquid Water and Water Activity at Gale Crater on Mars. Nat. Geosci 8, 357–361. doi:10.1038/ngeo2412
Matsubaya, O., Sakai, H., Torii, T., Burton, H., and Kerry, K. (1979). Antarctic saline lakes—Stable Isotopic Ratios, Chemical Compositions and Evolution. Geochimica et Cosmochimica Acta 43, 7–25. doi:10.1016/0016-7037(79)90042-5
McEwen, A. S., Eliason, E. M., Bergstrom, J. W., Bridges, N. T., Hansen, C. J., Delamere, W. A., et al. (2007). Mars Reconnaissance Orbiter’s High Resolution Imaging Science experiment (HiRISE). J. Geophys. Res. Planets 112. doi:10.1029/2005je002605
McKay, C. P., Clow, G. D., Wharton, R. A., and Squyres, S. W. (1985). Thickness of Ice on Perennially Frozen Lakes. Nature 313, 561–562. doi:10.1038/313561a0
McKnight, D. M., Niyogi, D. K., Alger, A. S., Bomblies, A., Conovitz, P. A., and Tate, C. M. (1999). Dry Valley Streams in Antarctica: Ecosystems Waiting for Water. BioScience 49, 985. doi:10.2307/1313732
McMahon, S., and Cosmidis, J. (2021). False Biosignatures on Mars: Anticipating Ambiguity. J. Geol. Soc. 179, jgs2021–050. doi:10.1144/jgs2021-050
Menzel, P., Gaye, B., Mishra, P. K., Anoop, A., Basavaiah, N., Marwan, N., et al. (2014). Linking Holocene Drying Trends from Lonar Lake in Monsoonal central India to North Atlantic Cooling Events. Palaeogeogr. Palaeoclimatol. Palaeoecol. 410, 164–178. doi:10.1016/j.palaeo.2014.05.044
Menzel, P., Gaye, B., Wiesner, M. G., Prasad, S., Stebich, M., Das, B. K., et al. (2013). Influence of Bottom Water Anoxia on Nitrogen Isotopic Ratios and Amino Acid Contributions of Recent Sediments from Small Eutrophic Lonar Lake, central India. Limnol. Oceanogr. 58, 1061–1074. doi:10.4319/lo.2013.58.3.1061
Merino, N., Aronson, H. S., Bojanova, D. P., Feyhl-Buska, J., Wong, M. L., Zhang, S., et al. (2019). Living at the Extremes: Extremophiles and the Limits of Life in a Planetary Context. Front. Microbiol. 10, 780. doi:10.3389/fmicb.2019.00780
Mikucki, J., Lyons, W. B., Hawes, I., Lanoil, B. D., and Doran, P. T. (2010). “Saline Lakes and Ponds in the McMurdo Dry Valleys: Ecological Analogs to Martian Paleolake Environments,”. Life in Antarctic Deserts and other Cold Dry Environments. Editors P. T. Doran, W. B. Lyons, and D. M. McKnight (Cambridge: Cambridge University Press), 160–194.
Milliken, R. E., and Bish, D. L. (2010). Sources and Sinks of clay Minerals on Mars. Philos. Mag. 90, 2293–2308. doi:10.1080/14786430903575132
Milliken, R. E., Grotzinger, J. P., and Thomson, B. J. (2010). Paleoclimate of mars as Captured by the Stratigraphic Record in Gale Crater. Geophys. Res. Lett. 37, 1–6. doi:10.1029/2009GL041870
Ming, D. W., Mittlefehldt, D. W., Morris, R. V., Golden, D., Gellert, R., Yen, A., et al. (2006). Geochemical and Mineralogical Indicators for Aqueous Processes in the Columbia Hills of Gusev Crater, Mars. J. Geophys. Res. Planets 111. doi:10.1029/2005je002560
Naeem, S., Hahn, D. R., and Schuurman, G. (2000). Producer-decomposer Co-dependency Influences Biodiversity Effects. Nature 403, 762–764. doi:10.1038/35001568
Navarro-González, R., Rainey, F. A., Molina, P., Bagaley, D. R., Hollen, B. J., de la Rosa, J., et al. (2003). Mars-like Soils in the Atacama Desert, Chile, and the Dry Limit of Microbial Life. Science 302, 1018–1021. doi:10.1126/science.1089143
Nayak, K. (1996). A Hypothesis for the Salinity of lake Water and Economic Potential of the Lonar Impact Crater, India. Antarct. Meteorol. XXI 21, 136–137.
Neveu, M., Hays, L. E., Voytek, M. A., New, M. H., and Schulte, M. D. (2018). The Ladder of Life Detection. Astrobiology 18, 1375–1402. doi:10.1089/ast.2017.1773
Noffke, N., Gerdes, G., Klenke, T., and Krumbein, W. E. (2001). Microbially Induced Sedimentary Structures: A New Category within the Classification of Primary Sedimentary Structures. J. Sediment. Res. 71, 649–656. doi:10.1306/2DC4095D-0E47-11D7-8643000102C1865D
Obryk, M. K., Doran, P. T., Waddington, E. D., and Mckay, C. P. (2017). The influence of Föhn Winds on Glacial Lake Washburn and Palaeotemperatures in the McMurdo Dry Valleys, Antarctica, During the Last Glacial Maximum. Antarct. Sci. 29, 457–467. doi:10.1017/S0954102017000062
Oren, A. (2008). Life at Low Water Activity: Halophilic Microorganisms and Their Adaptations. The Biochemist 30, 10–13. doi:10.1042/BIO03004010
Orgel, C., Kereszturi, Á., Váczi, T., Groemer, G., and Sattler, B. (2014). Scientific Results and Lessons Learned from an Integrated Crewed Mars Exploration Simulation at the Rio Tinto Mars Analogue Site. Acta Astronautica 94, 736–748. doi:10.1016/j.actaastro.2013.09.014
Osinski, G. R., and Lee, P. (2005). Intra-crater Sedimentary Deposits at the Haughton Impact Structure, Devon Island, Canadian High Arctic. Meteorit. Planet. Sci. 40, 1887–1899. doi:10.1111/j.1945-5100.2005.tb00152.x
Osinski, G. R., Tornabene, L. L., Banerjee, N. R., Cockell, C. S., Flemming, R., Izawa, M. R. M., et al. (2013). Impact-generated Hydrothermal Systems on Earth and Mars. Icarus 224, 347–363. doi:10.1016/j.icarus.2012.08.030
O’Sullivan, E. M., Goodhue, R., Ames, D. E., and Kamber, B. S. (2016). Chemostratigraphy of the Sudbury Impact basin Fill: Volatile Metal Loss and post-impact Evolution of a Submarine Impact basin. Geochimica et Cosmochimica Acta 183, 198–233. doi:10.1016/j.gca.2016.04.007
Paerl, H. W., and Priscu, J. C. (1998). Microbial Phototrophic, Heterotrophic, and Diazotrophic Activities Associated with Aggregates in the Permanent Ice Cover of Lake Bonney, Antarctica. Microb. Ecol. 36, 221–230. doi:10.1007/s002489900109
Pál, B., Kereszturi, Á., Forget, F., and Smith, M. D. (2019). Global Seasonal Variations of the Near-Surface Relative Humidity Levels on Present-Day Mars. Icarus 333, 481–495. doi:10.1016/j.icarus.2019.07.007
Palucis, M. C., Dietrich, W. E., Williams, R. M. E., Hayes, A. G., Parker, T., Sumner, D. Y., et al. (2016). Sequence and Relative Timing of Large Lakes in Gale Crater (Mars) after the Formation of Mount Sharp. J. Geophys. Res. Planets 121, 472–496. doi:10.1002/2015je004905
Parker, B. C., Simmons, G. M., Love, F. G., Wharton, R. A., and Seaburg, K. G. (1981). Modern Stromatolites in Antarctic Dry Valley Lakes. Biosci. 31, 656–661. doi:10.2307/1308639
Parnell, J., Bowden, S., Cockell, C., Osinski, G., and Lee, P. (2006). “Surface mineral Crusts: a Priority Target in Search for Life on Mars,” in 37th Lunar and Planetary Science Conference, Houston, Texas, USA, 13-17 Mar 2006.
Parnell, J., Boyce, A., Thackrey, S., Muirhead, D., Lindgren, P., Mason, C., et al. (2010). Sulfur Isotope Signatures for Rapid Colonization of an Impact Crater by Thermophilic Microbes. Geology 38, 271–274. doi:10.1130/G30615.1
Parnell, J., Lee, P., Osinski, G. R., and Cockell, C. S. (2005). Application of Organic Geochemistry to Detect Signatures of Organic Matter in the Haughton Impact Structure. Meteorit. Planet. Sci. 40, 1879–1885. doi:10.1111/j.1945-5100.2005.tb00151.x
Parnell, J., Osinski, G., Lee, P., Cockell, C., and Taylor, C. (2004). “Hopane Biomarkers Traced from Bedrock to Recent Sediments and Ice at the Haughton Impact Structure, Devon Island: Implications for the Search for Biomarkers on Mars,” in 35th Lunar and Planetary Science Conference, Houston, Texas, USA, 15-19 Mar 2004.
Patriarche, J. D., Priscu, J. C., Takacs‐Vesbach, C., Winslow, L., Myers, K. F., Buelow, H., et al. (2021). Year‐Round and Long‐Term Phytoplankton Dynamics in Lake Bonney, a Permanently Ice‐Covered Antarctic Lake. J. Geophys. Res. Biogeosci 126. doi:10.1029/2020JG005925
Pedge, S. S., and Ahirrao, S. D. (2013). Assessment of Environmental Impact on Lonar Lake Water, (MS) India. Middle East. J. Sci. Res. 15, 1285–1289.
Pellerin, A., Lacelle, D., Fortin, D., Clark, I. D., and Lauriol, B. (2009). Microbial Diversity in Endostromatolites (cf.Fissure Calcretes) and in the Surrounding Permafrost Landscape, Haughton Impact Structure Region, Devon Island, Canada. Astrobiology 9, 807–822. doi:10.1089/ast.2008.0302
Power, S. N., Salvatore, M. R., Sokol, E. R., Stanish, L. F., and Barrett, J. E. (2020). Estimating Microbial Mat Biomass in the McMurdo Dry Valleys, Antarctica Using Satellite Imagery and Ground Surveys. Polar Biol. 43, 1753–1767. doi:10.1007/s00300-020-02742-y
Prasad, S., Anoop, A., Riedel, N., Sarkar, S., Menzel, P., Basavaiah, N., et al. (2014). Prolonged Monsoon Droughts and Links to Indo-Pacific Warm Pool: A Holocene Record From Lonar Lake, Central India. Earth Planet. Sci. Lett. 391, 171–182. doi:10.1016/j.epsl.2014.01.043
Price, P. B., and Sowers, T. (2004). Temperature Dependence of Metabolic Rates for Microbial Growth, Maintenance, and Survival. Proc. Natl. Acad. Sci. U.S.A. 101, 4631–4636. doi:10.1073/pnas.0400522101
Priscu, J. C., Priscu, L. R., Howard-Williams, C., and Vincent, W. F. (1988). Diel Patterns of Photosynthate Biosynthesis by Phytoplankton in Permanently Ice-Covered Antarctic Lakes under Continuous Sunlight. J. Plankton Res. 10, 333–340. doi:10.1093/plankt/10.3.333
Pueyo, J. J., Chong, G., and Jensen, A. (2002). Neogene Evaporites in Desert Volcanic Environments: Atacama Desert, Northern Chile. Sedimentology 48, 1411–1431. doi:10.1046/j.1365-3091.2001.00428.x
Ramirez, R. M., and Craddock, R. A. (2018). The Geological and Climatological Case for a Warmer and Wetter Early Mars. Nat. Geosci 11, 230–237. doi:10.1038/s41561-018-0093-9
Rampe, E. B., Ming, D. W., Blake, D. F., Bristow, T. F., Chipera, S. J., Grotzinger, J. P., et al. (2017). Mineralogy of an Ancient Lacustrine Mudstone Succession from the Murray Formation, Gale Crater, Mars. Earth Planet. Sci. Lett. 471, 172–185. doi:10.1016/j.epsl.2017.04.021
Raven, M. R., Sessions, A. L., Adkins, J. F., and Thunell, R. C. (2016). Rapid Organic Matter Sulfurization in Sinking Particles from the Cariaco Basin Water Column. Geochimica et Cosmochimica Acta 190, 175–190. doi:10.1016/j.gca.2016.06.030
Rech, J. A., Quade, J., and Betancourt, J. L. (2002). Late Quaternary Paleohydrology of the Central Atacama Desert (lat 22°–24°S), Chile. Geol. Soc. Am. Bull. 114, 334–348. doi:10.1130/0016-7606(2002)114<0334:LQPOTC>2.0.CO;2
Reiche, S., Rampen, S. W., Dorhout, D. J. C., Sinninghe Damsté, J. S., and Schouten, S. (2018). The Impact of Oxygen Exposure on Long-Chain Alkyl Diols and the Long Chain Diol index (LDI) - a Long-Term Incubation Study. Org. Geochem. 124, 238–246. doi:10.1016/j.orggeochem.2018.08.003
Risacher, F., Alonso, H., and Salazar, C. (1999). Geoquímica de Aguas en Cuencas Cerradas: I, II y III Regiones - Chile. Volumen III: Estudio de Cuencas de la II Región. Convenio de Cooperación DGA – UCN – IRD (S.I.T. No 51).
Risacher, F., Alonso, H., and Salazar, C. (2003). The Origin of Brines and Salts in Chilean Salars: a Hydrochemical Review. Earth-Science Rev. 63, 249–293. doi:10.1016/S0012-8252(03)00037-0
Roach, L. H., Mustard, J., Gendrin, A., Fernández-Remolar, D., Amils, R., and Amaral-Zettler, L. (2006). Finding Mineralogically Interesting Targets for Exploration from Spatially Coarse Visible and Near IR Spectra. Earth Planet. Sci. Lett. 252, 201–214. doi:10.1016/j.epsl.2006.09.044
Roseborough, V., Horvath, D. G., and Palucis, M. C. (2021). Was Gale Crater (Mars) Connected to a Regionally Extensive Groundwater System? Geophys. Res. Lett. 48. doi:10.1029/2020GL092107
Salvatore, M. R., Barrett, J. E., Borges, S. R., Power, S. N., Stanish, L. F., Sokol, E. R., et al. (2021). Counting Carbon: Quantifying Biomass in the McMurdo Dry Valleys through Orbital & Field Observations. Int. J. Remote Sensing 42, 8597–8623. doi:10.1080/01431161.2021.1981559
Salvatore, M. R., Borges, S. R., Barrett, J. E., Sokol, E. R., Stanish, L. F., Power, S. N., et al. (2020). Remote Characterization of Photosynthetic Communities in the Fryxell basin of Taylor Valley, Antarctica. Antarctic Sci. 32, 255–270. doi:10.1017/S0954102020000176
Salvatore, M. R., Mustard, J. F., Head, J. W., Marchant, D. R., and Wyatt, M. B. (2014). Characterization of Spectral and Geochemical Variability within the Ferrar Dolerite of the McMurdo Dry Valleys, Antarctica: Weathering, Alteration, and Magmatic Processes. Antartic Sci. 26, 49–68. doi:10.1017/S0954102013000254
Sánchez-Andrea, I., Rodríguez, N., Amils, R., and Sanz, J. L. (2011). Microbial Diversity in Anaerobic Sediments at Río Tinto, a Naturally Acidic Environment with a High Heavy Metal Content. Appl. Environ. Microbiol. 77, 6085–6093. doi:10.1128/AEM.00654-11
Sánchez-García, L., Aeppli, C., Parro, V., Fernández-Remolar, D., García-Villadangos, M., Chong-Diaz, G., et al. (2018). Molecular Biomarkers in the Subsurface of the Salar Grande (Atacama, Chile) Evaporitic Deposits. Biogeochem. 140, 31–52. doi:10.1007/s10533-018-0477-3
Santschi, P., Höhener, P., Benoit, G., and Buchholtz-ten Brink, M. (1990). Chemical Processes at the Sediment-Water Interface. Mar. Chem. 30, 269–315. doi:10.1016/0304-4203(90)90076-O
Sanz, J. L., Rodríguez, N., Díaz, E. E., and Amils, R. (2011). Methanogenesis in the Sediments of Rio Tinto, an Extreme Acidic River: Methanogenesis in Rio Tinto Sediments. Environ. Microbiol. 13, 2336–2341. doi:10.1111/j.1462-2920.2011.02504.x
Sarkar, S., Prasad, S., Wilkes, H., Riedel, N., Stebich, M., Basavaiah, N., et al. (2015). Monsoon Source Shifts during the Drying Mid-holocene: Biomarker Isotope Based Evidence from the Core 'monsoon Zone' (CMZ) of India. Quat. Sci. Rev. 123, 144–157. doi:10.1016/j.quascirev.2015.06.020
Sarkar, S., Wilkes, H., Prasad, S., Brauer, A., Riedel, N., Stebich, M., et al. (2014). Spatial Heterogeneity in Lipid Biomarker Distributions in the Catchment and Sediments of a Crater lake in central India. Org. Geochem. 66, 125–136. doi:10.1016/j.orggeochem.2013.11.009
Sengupta, D., and Bhandari, N. (1988). Formation Age of the Lonar Crater. Lunar Planet. Sci. XiX 1537, 1059–1060.
Sengupta, D., Bhandari, N., and Watanabe, S. (1997). Formation Age of Lonar Meteor Crater, India. Rev. Fis. Apl. E Instrumentacao 12, 1–7.
Siljeström, S., Freissinet, C., Goesmann, F., Steininger, H., Goetz, W., Steele, A., et al. (2014). Comparison of Prototype and Laboratory Experiments on MOMA GCMS: Results from the AMASE11 Campaign. Astrobiology 14, 780–797. doi:10.1089/ast.2014.1197
Soare, R. J., Osinski, G. R., and Roehm, C. L. (2008). Thermokarst Lakes and Ponds on Mars in the Very Recent (Late Amazonian) Past. Earth Planet. Sci. Lett. 272, 382–393. doi:10.1016/j.epsl.2008.05.010
Sobron, P., Bishop, J. L., Blake, D. F., Chen, B., and Rull, F. (2014). Natural Fe-Bearing Oxides and Sulfates from the Rio Tinto Mars Analog Site: Critical Assessment of VNIR Reflectance Spectroscopy, Laser Raman Spectroscopy, and XRD as mineral Identification Tools. Am. Mineral. 99, 1199–1205. doi:10.2138/am.2014.4595
Sohm, J. A., Niederberger, T. D., Parker, A. E., Tirindelli, J., Gunderson, T., Cary, S. C., et al. (2020). Microbial Mats of the McMurdo Dry Valleys, Antarctica: Oases of Biological Activity in a Very Cold Desert. Front. Microbiol. 11, 2599. doi:10.3389/fmicb.2020.537960
Spigel, R. H., and Priscu, J. C. (1998). Physical Limnology of the McMurdo Dry Valleys Lakes. Ecosyst. Dyn. Polar Desert Mcmurdo Dry Val. Antarct. 72, 153–187.
Squyres, S. W., Grotzinger, J. P., Arvidson, R. E., Bell, J. F., Calvin, W., Christensen, P. R., et al. (2004). In Situ Evidence for an Ancient Aqueous Environment at Meridiani Planum, Mars. Science 306, 1709–1714. doi:10.1126/science.1104559
Squyres, S. W., and Knoll, A. H. (2005). Sedimentary Rocks at Meridiani Planum: Origin, Diagenesis, and Implications for Life on Mars. Earth Planet. Sci. Lett. 240, 1–10. doi:10.1016/j.epsl.2005.09.038
Srivastava, A., Olsson-Francis, K., Pearson, V., Schwenzer, S., Macey, M., Toubes-Rodrigo, M., et al. (2020). Preservation and Detection of Lipid Biomarkers within Martian Sulfates. Oral. doi:10.5194/epsc2020-97
Stein, N., Grotzinger, J. P., Schieber, J., Mangold, N., Hallet, B., Newsom, H., et al. (2018). Desiccation Cracks Provide Evidence of lake Drying on Mars, Sutton Island Member, Murray Formation, Gale Crater. Geology 46, 515–518. doi:10.1130/G40005.1
Stivaletta, N., Barbieri, R., Cevenini, F., and López-García, P. (2011). Physicochemical Conditions and Microbial Diversity Associated with the Evaporite Deposits in the Laguna de la Piedra (Salar de Atacama, Chile). Geomicrobiol. J. 28, 83–95. doi:10.1080/01490451003653102
Storzer, D., and Koeberl, C. (2004). “Age of the Lonar Impact Crater, India: First Results from Fission Track Dating,” in Lunar and Planetary Science Conference, 1309.
Summons, R. E., Amend, J. P., Bish, D., Buick, R., Cody, G. D., Des Marais, D. J., et al. (2011). Preservation of Martian Organic and Environmental Records: Final Report of the Mars Biosignature Working Group. Astrobiology 11, 157–181. doi:10.1089/ast.2010.0506
Summons, R. E., Welander, P. V., and Gold, D. A. (2021). Lipid Biomarkers: Molecular Tools for Illuminating the History of Microbial Life. Nat. Rev. Microbiol. 20, 174–185. doi:10.1038/s41579-021-00636-2
Surakasi, V. P., Antony, C. P., Sharma, S., Patole, M. S., and Shouche, Y. S. (2010). Temporal Bacterial Diversity and Detection of Putative Methanotrophs in Surface Mats of Lonar Crater lake. J. Basic Microbiol. 50, 465–474. doi:10.1002/jobm.201000001
Surve, R. R., Shirke, A. V., Athalye, R. R., and Sangare, M. M. (2021). A Review on Chemical and Ecological Status of Lonar Lake. Curr. World Environ. 16 (1), 61–69. doi:10.12944/CWE.16.1.07
Tanaka, K. L., Skinner, J. A., Dohm, J. M., Irwin, R. P., Kolb, E. J., Fortezzo, C. M., et al. (2014). Geologic Map of Mars. Available at: http://repository.si.edu/xmlui/handle/10088/22643 (Accessed December 24, 2021).doi:10.3133/sim3292
Taton, A., Grubisic, S., Balthasart, P., Hodgson, D. A., Laybourn-Parry, J., and Wilmotte, A. (2006). Biogeographical Distribution and Ecological Ranges of Benthic Cyanobacteria in East Antarctic Lakes. FEMS Microbiol. Ecol. 57, 272–289. doi:10.1111/j.1574-6941.2006.00110.x
Thakker, C. D., and Ranade, D. R. (2002). An Alkalophilic Methanosarcina Isolated from Lonar Crater. Curr. Sci. 82, 455–458.
Thomson, B. J., Bridges, N. T., Milliken, R., Baldridge, A., Hook, S. J., Crowley, J. K., et al. (2011). Constraints on the Origin and Evolution of the Layered mound in Gale Crater, Mars Using Mars Reconnaissance Orbiter Data. Icarus 214, 413–432. doi:10.1016/j.icarus.2011.05.002
Toner, J. D., Sletten, R. S., and Prentice, M. L. (2013). Soluble Salt Accumulations in Taylor Valley, Antarctica: Implications for paleolakes and Ross Sea Ice Sheet Dynamics. J. Geophys. Res. Earth Surf. 118, 198–215. doi:10.1029/2012JF002467
Tregoning, G. S., Kempher, M. L., Jung, D. O., Samarkin, V. A., Joye, V. A., and Madigan, M. T. (2015). A halophilic Bacterium Inhabiting the Warm, CaCl2-Rich Brine of the Perennially Ice-Covered Lake Vanda, McMurdo Dry Valleys, Antarctica. Appl. Environ. Microbiol. 81, 1988–1995.
van der Meer, F. D., van der Werff, H. M. A., van Ruitenbeek, F. J. A., Hecker, C. A., Bakker, W. H., Noomen, M. F., et al. (2012). Multi- and Hyperspectral Geologic Remote Sensing: A Review. Int. J. Appl. Earth Observation Geoinformation 14, 112–128. doi:10.1016/j.jag.2011.08.002
Vaniman, D. T., Bish, D. L., Ming, D. W., Bristow, T. F., Morris, R. V., Blake, D. F., et al. (2014). Mineralogy of a Mudstone at Yellowknife Bay, Gale Crater, Mars. Science. doi:10.1126/science.1243480
Vick-Majors, T. J., Priscu, J. C., and A Amaral-Zettler, L. (2014). Modular Community Structure Suggests Metabolic Plasticity during the Transition to Polar Night in Ice-Covered Antarctic Lakes. ISME J. 8, 778–789. doi:10.1038/ismej.2013.190
Vick-Majors, T. J., and Priscu, J. C. (2019). Inorganic Carbon Fixation in Ice-Covered Lakes of the McMurdo Dry Valleys. Antarctic Sci. 31, 123–132. doi:10.1017/S0954102019000075
Wang, F., Bowen, B. B., Seo, J.-H., and Michalski, G. (2018). Laboratory and Field Characterization of Visible to Near-Infrared Spectral Reflectance of Nitrate Minerals from the Atacama Desert, Chile, and Implications for Mars. Am. Mineral. 103, 197–206. doi:10.2138/am-2018-6141
Wani, A. A., Surakasi, V. P., Siddharth, J., Raghavan, R. G., Patole, M. S., Ranade, D., et al. (2006). Molecular Analyses of Microbial Diversity Associated with the Lonar Soda lake in India: An Impact Crater in a basalt Area. Res. Microbiol. 157, 928–937. doi:10.1016/j.resmic.2006.08.005
Warren-Rhodes, K., Weinstein, S., Dohm, J., Piatek, J., Minkley, E., Hock, A., et al. 2007). Searching for Microbial Life Remotely: Satellite-To-Rover Habitat Mapping in the Atacama Desert, Chile. J. Geophys. Res. 112, a–n. doi:10.1029/2006JG000283
Weand, B. L. (1975). THE CHEMICAL LIMNOLOGY OF LAKE BONNEY, ANTARCTICA WITH EMPHASIS ON TRACE METALS AND NUTRIENTS. Dissertation. Virginia Polytechnic Institute and State University.
Wierzchos, J., Ascaso, C., and McKay, C. P. (2006). Endolithic Cyanobacteria in Halite Rocks from the Hyperarid Core of the Atacama Desert. Astrobiology 6, 415–422. doi:10.1089/ast.2006.6.415
Wilhelm, M. B., Davila, A. F., Eigenbrode, J. L., Parenteau, M. N., Jahnke, L. L., Liu, X.-L., et al. (2017). Xeropreservation of Functionalized Lipid Biomarkers in Hyperarid Soils in the Atacama Desert. Org. Geochem. 103, 97–104. doi:10.1016/j.orggeochem.2016.10.015
Williams, A. J., Eigenbrode, J., Floyd, M., Wilhelm, M. B., O'Reilly, S., Johnson, S. S., et al. (2019). Recovery of Fatty Acids from Mineralogic Mars Analogs by TMAH Thermochemolysis for the Sample Analysis at Mars Wet Chemistry Experiment on the Curiosity Rover. Astrobiology 19, 522–546. doi:10.1089/ast.2018.1819
Wood, S. A., Rueckert, A., Cowan, D. A., and Cary, S. C. (2008). Sources of Edaphic Cyanobacterial Diversity in the Dry Valleys of Eastern Antarctica. ISME J. 2, 308–320. doi:10.1038/ismej.2007.104
Wordsworth, R. D. (2016). The Climate of Early Mars. Annu. Rev. Earth Planet. Sci. 44, 381–408. doi:10.1146/annurev-earth-060115-012355
Wordsworth, R., Forget, F., Millour, E., Head, J. W., Madeleine, J.-B., and Charnay, B. (2013). Global Modelling of the Early Martian Climate under a Denser CO2 Atmosphere: Water Cycle and Ice Evolution. Icarus 222, 1–19. doi:10.1016/j.icarus.2012.09.036
Wright, S. (2014). “Lonar Crater, India: Analog for Mars in the Field and in the Laboratory,” in Eighth International Conference on Mars, 1450.
Keywords: astrobiology, terrestrial analogs, remote sensing, coupling scales, Mars, biosignatures
Citation: Harris CM, Maclay MT, Lutz KA, Nathan V, Ortega Dominguez NA, Leavitt WD and Palucis MC (2022) Remote and in-Situ Characterization of Mars Analogs: Coupling Scales to Improve the Search for Microbial Signatures on Mars. Front. Astron. Space Sci. 9:849078. doi: 10.3389/fspas.2022.849078
Received: 05 January 2022; Accepted: 30 March 2022;
Published: 26 April 2022.
Edited by:
Margarita Safonova, Indian Institute of Astrophysics, IndiaReviewed by:
Akos Kereszturi, Hungarian Academy of Sciences (MTA), HungaryRoberto Barbieri, University of Bologna, Italy
Copyright © 2022 Harris, Maclay, Lutz, Nathan, Ortega Dominguez, Leavitt and Palucis. This is an open-access article distributed under the terms of the Creative Commons Attribution License (CC BY). The use, distribution or reproduction in other forums is permitted, provided the original author(s) and the copyright owner(s) are credited and that the original publication in this journal is cited, in accordance with accepted academic practice. No use, distribution or reproduction is permitted which does not comply with these terms.
*Correspondence: Marisa C. Palucis, TWFyaXNhLkMuUGFsdWNpc0BkYXJ0bW91dGguZWR1
†These authors have contributed equally to this work and share first authorship
‡These authors have contributed equally to this work and share senior authorship