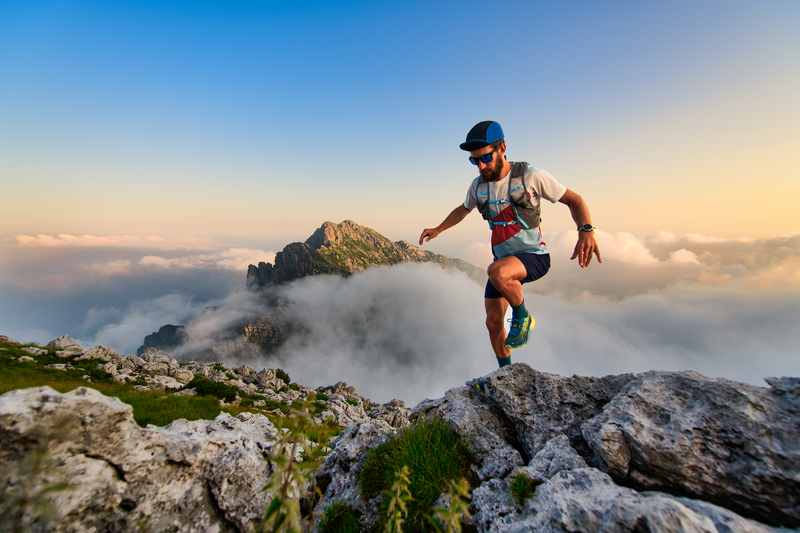
95% of researchers rate our articles as excellent or good
Learn more about the work of our research integrity team to safeguard the quality of each article we publish.
Find out more
REVIEW article
Front. Astron. Space Sci. , 08 March 2022
Sec. Astrobiology
Volume 9 - 2022 | https://doi.org/10.3389/fspas.2022.841211
Human space exploration cannot occur without reliable provision of nutritious and palatable food to sustain physical and mental well-being. This ultimately will depend upon efficient production of food in space, with on-site manufacturing on space stations or the future human colonies on celestial bodies. Extraterrestrial environments are by their nature foreign, and exposure to various kinds of plant stressors likely cannot be avoided. But this also offers opportunities to rethink food production as a whole. We are used to the boundaries of the Earth ecosystem such as its standard temperature range, oxygen and carbon dioxide concentrations, plus diel cycles of light, and we are unfamiliar with liberating ourselves from those boundaries. However, space research, performed both in true outer space and with mimicked space conditions on Earth, can help explore plant growth from its ‘first principles’. In this sense, this perspective paper aims to highlight fundamental opportunities for plant growth in space, with a new perspective on the subject. Conditions in space are evidently demanding for plant growth, and this produces “stress”. Yet, this stress can be seen as positive or negative. With the positive view, we discuss whether plant production systems could proactively leverage stresses instead of always combatting against them. With an engineering view, we focus, in particular, on the opportunities associated with radiation exposure (visible light, UV, gamma, cosmic). Rather than adapting Earth conditions into space, we advocate on rethinking the whole issue; we propose there are opportunities to exploit space conditions, commonly seen as threats, to benefit space farming.
Space exploration has intrigued humans for decades; with much of our wonder stemming from the unknown. If human beings ever visit or live for a prolonged time on another planet, they will need enough food for the complete journey, produced in a bioregenerative life support system (BLSS; Figure 1). One astronaut on a 9-month one-way trip to Mars requires an estimated 1 tonne of food (Dunbar, 2007). The estimated cost of preparing, launching, and storing this food is US$50M; a 3-year round trip would cost substantially more.
FIGURE 1. Principal relationships in a bioregenerative life support system to support human habitation of remote outposts in space. Plants play an integral role by regenerating the atmosphere and recycling water while providing food for the crew.
Significant limitations for long-term missions include the limited number of pharmaceuticals/vitamins that adequately make it to a year of shelf-life due to degradation (Kast et al., 2017). NASA has spent years perfecting thermo-stabilised or freeze-dried foods for astronauts on the International Space Station (ISS), from scrambled eggs to chicken teriyaki. “The meals are meant to last, but they would not survive the long journey to Mars”, says Julie Robinson, the chief scientist for the International Space Station (Koren, 2019). On the ISS, and proposed mission vehicles, there is very limited refrigeration present, constraining delivery or storage potential of fresh foods. Accordingly, sustainable “life-environment crop-growth ecosystems” need to be developed in conjunction with the science of how plants grow in space (Figure 1). This provides the opportunity to propel scientific discovery by interdisciplinary paradigms such as microgravity, cosmic ray irradiation, and “Unearth” gas atmospheres, temperatures, and light patterns, which are referred to as “plant stressors” in this review.
Space farming studies have been made since the early years of space launches (Harvey and Zakutnyaya, 2011) and have become steady since the ISS commenced establishment in 1998, which is humankind’s sole laboratory above Earth at present (Figure 2). However, this will soon be joined the low Earth orbit Chinese Tiangong space station currently being assembled in space (Shen et al., 2018) and the ISS is currently scheduled for staged replacement by the Axiom Lunar Gateway from Axiom Space onwards (Ekblaw, 2020). The need for adequate and sustainable farming systems is a pressing issue as sights are set on upcoming Mars missions (History and timeline of t, 2019). The importance of plant growth and development in outer space is critical for crewed missions, not only from a nutrients’ perspective but also from a psychological one (Zabel et al., 2016; History and timeline of t, 2019).
FIGURE 2. Leafy greens grown on the International Space Station (Meggs et al., 2010).
This review is conceptualised from an engineers’ perspective rather than aiming for a complete microbiology and crop science overview. More precisely, it represents the analysis of system engineers, who are willing to cross borders, in particular to plant biology and crop-relevant photochemistry and–physics; all under the auspices of space stressing. We will give an inventory of plant stressors and how they may be overcome or utilised in outer space. We will propose what kind of stress is strictly to be avoided and requires adequate shielding, and which kind of stress (stressors) might positively promote plant growth in harsh space conditions and assist with their domestication for space (Figure 3). In particular, we focus on the issues surrounding radiation (e.g., light, UV, gamma, cosmic). These aspects are then combined to discuss the combination of stressors to which plants will be exposed to. We will conclude with what opportunities are provided in space compared to Earth-grown plants, and how Earth horticulture can be further advanced with such learning, especially for the harsh environments on Earth, e.g., in the Arctic and Antarctica.
FIGURE 3. Stress and eustress for plant growth in space, and the role of modelling to make forecasts.
Tropisms are directional movement responses in plants that occur in response to a directional stimulus (e.g., gravity, touch, temperature, light, water, salt, chemicals). The comprehension of the pathways that control tropisms are at various levels of maturity, but what its clear is that tropisms are usually controlled by a network of internal signals including plant hormones (Weyers and Paterson, 2001). Tropisms can be a stress response, but equally could be harnessed for benefit under controlled conditions. Controlled Environment Agriculture (CEA) can be developed as closed, environmental systems, to mitigate stress from extreme environments on Earth. In specialised containers, plant growth functions can be maintained even in the Arctic, Antarctica, or at high altitudes in mountains. The aim and perspective given in this review, however, is not entirely to avoid stress, but to seek opportunities to use stress to become the standard environment for plant growth in space. Eustress, in general terms, means beneficial stress, either being psychological, physical, or biochemical/radiological. The question then is if plants can adapt themselves to the stress in space in a positive way to grow even better; possibly supported by genetic or other alteration.
Plant stressors will first be considered in isolation with reference to previous works before a discussion of the potential effects of combined stressors on plant performance. Two categories of plant stress are considered relation to biotic- or abiotic- origin.
Biotic stressors on Earth derive from the interaction of biological vectors with plants, including bacteria, viruses, fungi, or insects, which result in infection, diseases, damage, or a reduction in growth (Balachandran et al., 1997). In space, pathogens can be introduced in different ways. For example, several bacteria including Pseudomonas aeruginosa, Burkholderia cepacia, and Erwinia sp., are pathogenic to both plants and animal (Burkholder, 1950; Starr and Chatterjee, 1972; Goldmann and Klinger, 1986). However, of these only P. aeruginosa, has been shown to infect leaves and roots of the model plant Arabidopsis thaliana resulting in mortality (Plotnikova et al., 2000; Walker et al., 2004). Similarly, Staphylococcus aureus is carried by humans and can infect the leaves and roots of Arabidopsis thaliana resulting in lesions, chlorotic tissue and eventual mortality (Prithiviraj et al., 2005). Therefore, astronauts can serve as the host, carrying viruses and bacteria into space (Barringer and Abadie, 2013). Alternatively, pests and pathogens may be transported in growth medium, on tools or receptacles or in the spacecraft from Earth.
The mechanisms to help plants overcome biotic stress may be based on their natural resilience (as developed on Earth), or through the maintenance of a clean environment or treatments to minimise any threat. Plant natural defence mechanisms to biotic stress can include morphological and structural adjustments, chemical compounds, proteins or enzyme production (Song et al., 2021). In space, we may rely on and further developed human-made defence strategies for plants, i.e., management practices to limit the severity or even fully prevent biotic stress. As such, an integrated pest management (IPM) program is required to prevent, mitigate, and eliminate both insect pests and disease outbreaks in space-based plant-growing systems (Schuerger, 2021). This can include measures such as disinfecting tools and receptacles, cultivating plants in a closed system, or applying natural predators or chemical repellants.
One of the main mechanisms to minimise biotic stress is the cultivation in a closed system, which is a doubly closed one, since a “space greenhouse” will be part of a space station or lunar/Martian habitat (Kiss, 2014; Wamelink et al., 2021). Yet, that strategy bears both danger and opportunity. The danger is that if a biotic stressor is “imported” to a space station or habitat, it will stay there, potentially propagate, and present a high density (per space volume) of biotic stressor. Secondly, a closed system may also present a unique combination of stressors that facilitate disease spread (Stressors’ Impact on Plants Across Diverse Scales). The costly, high-tech closed systems of space environments present an opportunity to be run under with a under conditions similar to a clean room, facilitated by their relatively small footprint. Admittedly, such instrumentation is costly and energy-intensive. Nonetheless, if one manages to keep biotic stress completely absent, plants might grow in a way not seen on Earth, focusing their energies used formerly for biotic shielding now for growth.
Abiotic stresses known on Earth include cold stress, heat stress, drought stress, waterlogging/anoxia, salt (salinity) stress, and to a lesser extent light stress (Figure 4) (Mittler, 2006). These stressors are expected to also be present in space, yet with potential differences in severity. There are also abiotic stresses that are specific for space, and thus not found on Earth including stress induced by microgravity and radiation (cosmic). Light stress is primarily considered here as well, since plants are adapted to solar radiation as filtered through the Earth’s atmosphere. The impact of those space-specific stressors is less known than for the Earth-bound stresses. The following sections will discuss each of these stressors in turn.
FIGURE 4. Abiotic stresses on Earth and in space. Light stress is assigned to space, albeit known as well on Earth for reasons given in the respective text.
Temperatures in space vary from very cold to hot when considering the largely fluctuating temperatures on celestial bodies such as the Moon and Mars. Similarly, environment of a spacecraft may vary, although is expected to remain more stable relative to the outside environment. As space is concerned, the scope of temperatures on the surface of the planets within our solar system is vast, ranging from −230 to 470°C with many of the planets experiencing their own significant range at varying points throughout the day and night (Table 1) (Solar System Temperatures, 2018). For instance, Mars’ surface temperature ranges from −143 to 35°C (Karnasuta et al., 2007). Similarly, Earth’s temperature ranges from −89.2 to 59.8°C depending on the location, time, and season in which temperature was measured (Court, 1949). Temperature cycles in space do not follow the day (warm) and night (cold) cycles on Earth. This raises the question of which temperature settings may give optimal morphological and developmental responses of current crops or even new space crops.
Plants can only grow within a certain temperature range (Pisek et al., 1973). Temperature can directly affect plant growth through metabolic processes such as photosynthesis, respiration, and transpiration (Holding and Streich, 2013). Indirectly, temperature can impact soil temperature and air temperature, altering the absorption and transport of water and fertiliser through the plant (Hawkes et al., 2008). Under cold stress, Enzyme activities are reduced and photosynthetic genes are down-regulated. Cold stress can be exacerbated by high light to stimulate photoprotection pathways, with corresponding impact on the cellular function of many plant species (Demmig-Adams et al., 2012). Additionally, protein stability and solubility are altered (Eric et al., 2009). Depending on the species and location, trees and other higher plants exhibit a lower thermal limit of 5°C for plant growth (for arctic/Antarctic species; noting they can withstand freezing), with restricted growth at 6–7°C. As the temperature increases, plant growth gradually accelerates, and grows fastest at 20–30°C. But if the temperature continues to increase beyond that, plant growth will gradually slow down or even stop growing. High or low temperature during the reproductive phase can often lead to flower abortion, infertility, and low yield (Blum, 2011).
The optimal thermal range is highly species and photosynthetic pathway dependent. C4 plants generally perform better in hot, dry climates and are often more efficient than C3 in terms of light, water and nitrogen use. By contrast, C4 plants are generally more susceptible than C3 when it comes to cold stress (Long, 1983). C4 plants have a 60–80% reduction in the quantity of the photosynthetic enzyme Rubisco under cold temperatures, meaning that photosynthesis catalysis is unable to match the prevailing CO2 levels (Sage, 2002). Conversely, C3 plants are able to reduce the photorespiration with decreasing temperature, thus reducing the net energy required to fix CO2 under cooler conditions (Ripley et al., 2007).
Plants can adapt to differences in temperature, where thermal acclimation refers to the suite of morphological and architectural changes induced by variation in temperature. The effect of temperature stress on plant growth is exemplified via the physiological changes of Bromeliad seedlings, a large family of tropical plants, shown in Figure 5. Plant hormones including auxin, early flowering complex (ELF4), and the precursor gibberellin 12 (GA12) mediate growth response to ambient temperature changes. Under exposure to high temperatures (25–32°C), plants keep their roots at lower temperatures than the leaves through auxin-regulated thermonasty of leaves and associated hypocotyl elongation combined with GA12—mediated root thermomorphogensis. In comparison, plants under cold stress (10°C) maintain roots at a higher temperature than the leaves due to ELF4’s mobility, which is increased to modulate root circadian rhythms under cold temperatures.
FIGURE 5. Temperature distribution of bromeliads exposed to varying temperatures: (left) exposed to 25–32°C for 7 days, and (right) exposed to 10°C for 10 days. Solid and dashed lines indicate higher and reduced transmission of signaling molecules: auxin, early flowering complex (ELF4), and the precursor gibberellin 12 (GA12) respectively, which mediate growth response to ambient temperature changes (Nievola et al., 2017).
Alternative signalling pathways are employed by other species to respond to high temperature. In many plants, cells perceive heat in the plasma membrane and utilise signaling pathways involving ABA and calcium ion (Ca2+) exchange to trigger thermal acclimation mechanisms. Also, the activation state of the major photosynthetic enzyme, Rubisco activase, can change in response to temperature as well as light, CO2 concentration, and other environmental factors (Parry et al., 2008). Whilst Rubisco itself is heat stable, Rubisco activase is highly sensitive to temperature (Eckardt and Portis Jr, 1997). This leads to an optimal thermal range for the photosynthetic function, with differences in temperature acclimation between species (Hikosaka et al., 2006).
One way to avoid temperature stress is a change in the mechanism of photosynthesis that plants can undergo. The normal photosynthesis mechanism is performed by C3 plants; without going here into details of what this denotes.
Cold stress is an expected threat in space due to the low temperatures of the solar system. Whilst cold (or heat) stress is prevalent on Earth, the characteristics of the stress will also differ. For example, a day on the Moon is roughly equivalent to 27 days on the Earth, of which 13.5 days are daytime, and 13.5 days are nights. On the Moon, the highest temperature possible is 120°C, under direct sunlight during the day whilst night-temperatures can be as low as 150°C or even −180°C due to a lack of atmosphere. Mars also has seasonal changes like Earth but are approximately twice the length. This highlights the importance of the stress periods to which plants may be exposed; with the magnitude and length of stress likely differing in space relative to Earth. To some extent, thermal stress in space will be mitigated through engineering solutions to provide suitable plant growth habitats. However, depending on energy availability and habitat specification, the exact temperatures at which these can be maintained may differ compared to growth habitats on Earth.
Worldwide, drought e.g. lack of water, is one of the major constraints limiting crop production (Aroca, 2012a). The effect of drought will depend upon the length of water scarcity, the available water supply, if any, the developmental stage at which it occurs and the adaptation of the plant under study. Under reduced water availability, stomatal closure will reduce photosynthesis, enzyme activity will be disrupted, cell division and expansion will be reduced, and membranes or cell structure may be damaged. There is a range of possible responses to drought including morphological, molecular, or biochemical adjustments (Aroca, 2012). An important response of plants is a decline in shoot growth, which helps to reduce metabolic demand. Other morphological adjustments include increased root length, reduced leaf area, or succulent leaves (Farooq et al., 2009). During prolonged drought stress, protective compounds will be synthesised to help prepare the plant for osmotic adjustment and several signalling pathways are activated, altering gene expression and the plant metabolome (Stagnari et al., 2016). Due to negative interactions between different stressors, water stress will also increase the severity of any other abiotic stressors effecting plants.
As water is limited in space, the probability of drought causing water stress can be expected. This indicates that all water requirements must be supplied for plant growth. Within a closed-loop agricultural system, water can be recycled by re-condensing the water lost through transpiration; thus reducing overall requirements compared to an open growth system (Maiwald et al., 2021). Closed-loop systems also enable water to be supplied in appropriate amounts, thus reducing the overall risk of stress. Thus the potential stress to plants comes more from obtaining enough water in the first place. The ISS is able to recycle much of its water in the walls of the spacecraft using chemicals, however, astronauts still rely on shipments of water from Earth to provide safe drinking water, and for plant growth. This journey takes approximately 6 h from Earth to the ISS, whereas a similar shipment of water to Mars would take ∼9 months (O’Callaghan, 2020). Whilst alternative methods to purify and recycle water is being looked at (e.g., project BIOWYSE) (Guarnieri et al., 2020) another solution would be to obtain water elsewhere in the solar system in what NASA term “in situ resource utilisation”. It is widely accepted that historically there was abundant water on Mars, however low pressure resulting from a reduced atmosphere means that under current conditions, surface liquid water is highly limited (Nazari-Sharabian et al., 2020). As such, the majority of water exists as ice, with ice also found on the surface of the Moon. Theoretically, this ice could be converted into drinking water and as such, future Mars missions are identifying the optimal landing spot based on location of near surface water ice (Piqueux et al., 2019). Recent surveys have also shown the presence of under-ice saltwater lakes at the South pole of Mars, however, for the water to be in liquid form they are expected to contain a high concentration of salt (Lauro et al., 2021). Thus, water retrieved from these under-ice lakes will require de-salinisation, however, current scientific interest revolves around the potential for these lakes to harbour life forms.
Water shortages could be overcome in part by reducing water requirements through the growth of plants in aeroponics that typically needs 98% less water than field-grown plants (Spinoff, 2006), or growing cultivars that have high water use efficiency. Aeroponics, also have additional benefits of improved nutrient use efficiency, and higher growth rates due to higher oxygen availability at roots plus the ability to alter misting frequency and the nutrient composition to match the optimal conditions for each plant species (Lakhiar et al., 2018).
Salt stress affects plant growth and yield worldwide due to ionic toxicity, osmotic stress, plus alterations to the soil ionic balance and water status (Munns and Gilliham, 2015). Salinity leads to an increase in the concentration of sodium chloride (NaCl) in the cytoplasm and chloroplasts of higher plants, affecting growth rate and energy capture (Bose et al., 2017). When salt in the growing medium reaches a significant level, which may only be the equivalent of 3 dSm−1, the chlorophyll content of susceptible plants, such as potato, tomato, and pea, decreases, limiting the potential to capture light and photosynthesise, limiting growth (Parida and Das, 2005). When salt concentrations are high in the soil, the osmotic potential is larger than that of plant cells, so limiting the ability of plants to absorb water and minerals such as K+ or Ca2+. The overall plant function is, therefore, restricted (Munns and Gilliham, 2015). In comparison, in salt tolerant plants such as wheat, pearl millet and mustard, chlorophyll content increases under salt stress (Sudhir and Murthy, 2004; Acosta-Motos et al., 2017).
Salt stress further impacts photosynthesis through stomatal limitation, stomatal closure, inhibition of the chloroplast pigment-protein complex photosystem (PS) II, degradation of photosynthetic membrane proteins, membrane instability, decreased electron transport activities, a reduction in the content of the carbon-fixation enzyme Ribulose-1,5-bisphosphate carboxylase-oxygenase (Rubisco). This can lead to a reduction in photosynthetic rate or, in some instances, complete inhibition of assimilation (He et al., 2014). Salt stress also enhances the oxygenase activity of Rubisco whilst preventing carboxylase activity leading to an increase in photorespiration, reducing the efficiency of energy capture (Sivakumar et al., 2000).
Plants can be classed as glycophytes, halophytes or euhalophytes depending upon their level and mechanism of tolerance. Glycophytes, which includes most crop plants, have low tolerance; their growth is inhibited or even completely prevented by NaCl concentrations of 100–200 mM, resulting in mortality even under short-term exposure (Munns and Termaat, 1986; Hernández and Almansa, 2002). In contrast, halophytes can survive in the presence of high NaCl concentrations (300–500 mM) due to adaptive mechanisms (Parida and Das, 2005; Flowers and Colmer, 2015). This tolerance occurs through a series of morphological, physiological and biochemical changes (Acosta-Motos et al., 2017). Examples include: increases in the root/canopy ratio; increase in chlorophyll content; salt exclusion, elimination or storage; plus corresponding to changes in leaf anatomy (Larcher, 2003). These characteristics ultimately lead to preventing leaf ion toxicity, thus maintaining the water status.
It is currently unknown what effect salt stress may have in plants cultivated space. As plant growth will occur in a closed environment, the level of salt input to the system is likely to be regulated. However, plant growth will, in part, be dependent upon recycled water from human waste or from in situ resource utilisation such as under-ice salt water lakes (discussed above), and so salt-tolerant plants could be adopted to function as an osmoticum. Many studies have assessed the potential for using saline water on conventional crops and forages, however their low tolerance limits productivity (Jones et al., 2019; Romero-Trigueros et al., 2020). Thus, water desalinisation or application of irrigation water with a high salt concentration requires selection of appropriate species and genotypes (Gómez-Bellot et al., 2021). For example, Crithmum maritimum (sea fennel) and Atriplex halimus (Mediterranean saltbush) have recently been shown to tolerate irrigation by recycled wastewater and reverse osmosis brine but through differential accumulation of ions mediated by different hormonal signalling pathways (Gómez-Bellot et al., 2021). Both species can be of use for humans but C. maritimum, may be of particular use on space missions an additional food source, and with leaves exhibiting aromatic and medicinal purposes as a tonic and diuretic (Ruberto et al., 2000).
Plants evolved under gravity, and thus all aspects of plant growth, development, and morphology are affected by this force (Vandenbrink et al., 2014). In addition, gravity underlies many physical phenomena like buoyancy, convection, and sedimentation. In turn, this indirectly determines plant growth such as gas exchange, cellular respiration, and photosynthesis, each of which are influenced by changed in buoyancy (Braun et al., 2018). Plants have a known and consistent response to gravity (gravitropism). However, gravity is consistent across much of Earth and does not vary significantly. Mount Everest is the highest point on Earth at 8,848 m above sea level, and has an acceleration due to gravity of 9.773 m/s2, relative to an average of 9.800 m/s2 at sea level. Thus, only a 0.3% variation in gravity is observed on Earth. In comparison, Microgravity is considered to be the most important of all abiotic stresses in space (Zheng et al., 2015). Zero gravity refers to the absence of gravity is in space; significantly different from Earth (May 2017). Gravity is reduced by 16.5% (1.620 m/s2) and 38.0% (3.721 m/s2) on the Moon or Mars, respectively (Table 2) (Mosa et al., 2017).
On Earth, microgravity can be simulated in specialty machinery such as random position machine or clinostat, which move around a specific, typically random, trajectories in order to cancel out gravity (Hauslage et al., 2017). Magnetic forces have also proven to be a useful ground-based proxy for microgravity (Geim et al., 1999; Hammer et al., 2009). They can levitate cellular organelles, such as statoliths in roots (Kuznetsov and Hasenstein, 1996), hypocotyls (Kuznetsov and Hasenstein, 1997), rhizoids (Kuznetsov and Hasenstein, 2001), and bacteria (Dijkstra et al., 2011). However, these platforms do not reduce gravity, per se, but constantly change its direction (Kiss et al., 2019). This currently allows the investigation of altered gravity for small samples such as seedlings or emulsions indicating potential effects of microgravity on plant growth.
Microgravity can lead to mechanical changes in crops in terms of their plant growth, cell atrophy, and overall mass and mass distribution (Zheng et al., 2015; Vandenbrink and Kiss, 2016). This may cause bending stresses and other non-random mechanostimulation (John and Hasenstein, 2011), as well as dynamic effects (Kiss et al., 2019). Further, as there is no convection in zero gravity, this becomes an issue for gas build up around tissues leading to issues for gas exchange. An example of a gravity effect is provided by the stored food (starch) in Amyloplasts, which are involved in the gravity perception. Amyloplasts are denser than the cytoplasm and fall to lower cell surfaces. Starchless mutants have been prepared which respond sluggishly to gravity (Figure 6).
FIGURE 6. Schematic of cell-internal structure in the root tip of a normal plant with gravity-sensing Amyloplasts containing starch (A) and root tip of a starchless mutant plant under microgravity (B) (Kiss, 2000; Morita, 2010).
Since the 1960s, experiments conducted in space stations and research rockets have shown that plants can grow normally in microgravity provided factors such as confinement, lack of ventilation and elevated radiation levels are controlled. However, other experiments indicate a plethora of changes of plants grown under microgravity. This includes changes at the cell/molecular level, alterations to the cell cycle and the cell wall structure (Matía et al., 2010; Johnson et al., 2015). Altered gravity has also been shown to influence gene expression (Correll et al., 2013; Johnson et al., 2017; Choi et al., 2019) and facilitates altered phototropic responses (Millar et al., 2010; Kiss et al., 2012; Vandenbrink et al., 2016). The majority of these studies have been performed on Arabidopsis and thus the full extent of microgravity on plant growth for a variety of species is not known. However, seeds produced in orbit appear to have a different composition and developmental stages compared to seeds grown on Earth. In terms of providing a complete diet on long space missions, hostile space conditions could provide problems for both performance and nutritional content of space seeds, and alter the flavour of plants.
To some extent, it may be possible to simulate gravity and thus reduce the magnitude of gravitational stress. For example, rotational artificial gravity can be created using centrifugal force, e.g., via rotating two habitats around each other, as is envisaged for the Stanford torus system (diameter = 1.8 km, mass
To the best of our knowledge, atmospheric pressure has not been practically exploited so far on Earth but may be a valid addition to promote plant growth in space. In space or on celestial bodies, the atmosphere is either of a different composition (regarding the proportion of the gases) to Earth or completely lacking. On the Earth, the total atmospheric gas pressure at sea level is approximately 101 kPa, with partial pressure of oxygen at 21 kPa (Zhou et al., 2017). As elevation increases, the atmospheric pressure decreases, reaching 0 kPa at an altitude of approximately 30,000 m. However, on Earth, low pressure stress, known as hypobaria, co-occurs with low-oxygen stress, known as hypoxia, since the relative pressure of O2 is consistent in the atmosphere. A pressure of 50 kPa can be considered as a boundary of moderate and severe hypobaria, and represents the natural terrestrial limit (Paul et al., 2004; Richards et al., 2006). Controlled studies that manipulate pressure and oxygen composition independently indicate altered gene expression under hypobaric conditions, which is only partially alleviated under normoxia (Zhou et al., 2017). Understanding plant growth under hypobaria is relevant to space agriculture, where hypobaric environments may reflect a favorable engineering choice for plant growth habitats, and in terrestrial crop breeding, enabling expansion of cultivation into marginal terrain and environments (Corey et al., 2002; Paul and Ferl, 2007; Wheeler, 2010).
Current evidence suggests that alteration in pressure generally has little effect on plant development. Under laboratory conditions, hypobaric environments can be created maintaining amenable temperature, humidity, and gas composition. Generally, as long as temperatures are maintained above freezing and there is sufficient water availability, higher plants appear to physiologically adapt well to hypobaric environments. However, the specific response varies greatly depending on the composition of the atmosphere and the plant species (Paul et al., 2004; Corey et al., 2002; He et al., 2003; Andre and Massimino, 1992). For example, hypobaric stress was applied to radish, wheat, and lettuce with a reduction from 98 to 5 kPa for 5 min or 1.5 kPa for 10 min (Wheeler et al., 2011). The temperature and humidity were managed to maintain at 22°C and 65% relative humidity respectively. The plants showed almost no visible effects and sensitivity analysis indicated survival following 30 min in the low-pressure vacuum-like environment. In another study, the total pressure was reduced and as well the oxygen partial pressure. Normal plant growth was observed down to 50 kPa total pressure (Figure 7A), yet reduced plant growth was found when the oxygen partial pressure was too low (2.5 kPa) (Figure 7B) (Martelaro, 2017).
FIGURE 7. The growth status of wheat in low pressure environment: 50 kPa total pressure 10.4 or 5.0 kPa oxygen partial pressure (A), and 50 kPa total pressure 2.5 kPa oxygen partial pressure (B) (Guo et al., 2008).
Similar to hypobaric stress on Earth, under space-atmospheric conditions, hypoxia is common due to the lack of natural convection. Similarly, waterlogging decreases the oxygen available to roots. Indeed, under hypobaric conditions on Earth, hypoxia is a major contributor towards plant stress and symptoms (Ferl et al., 2002). An oxygen partial pressure of 10.3 kPa in 50 kPa constitutes mild hypoxic stress, and results in no obvious change in vegetative growth of Arabidopsis plants in soil (Ramonell et al., 2001). Under more severe stress, hypoxia physiologically inhibits respiration and oxidative phosphorylation, ultimately resulting in an energy deficit in plant cells (Drew, 1997; Mustroph et al., 2010). In such conditions, plants exhibit symptoms including a decrease in protein body size (44–80% smaller for the case of brassica), abnormal vacuole appearance, and embryo degeneration (Stankovic, 2018).
Carbon dioxide (CO2) is the main phytonutrient, which affects the growth rate, development, and final yield of plants. Earth’s atmosphere is composed of approximately 0.041% CO2 by volume, equal to 410 ppm. In comparison, the majority of the Martian atmosphere is CO2, comprising 95.3% CO2, 2.7% nitrogen, 1.6% argon, and a small amount of O2. Studies on Earth indicate the benefits to yield arising from increases in CO2 concentration (Ainsworth and Long, 2005). The leaves of most plants thicken, which changes the photosynthesis, gas exchange, evaporative cooling, and sugar storage of plants [98]. This changes the carbohydrate and nitrogen metabolism of plants (Wang et al., 2009). In addition, high concentrations of CO2 will inhibit photorespiration; the wasteful process of oxygenation, mediated by Rubisco.
Ozone (O3) can also influence plant growth, both directly or indirectly dependent on position and composition. On Earth, O3 acts as a pollutant at ground level but at high altitudes in the stratosphere, it forms an essential protective layer against harmful solar ultra-violet radiation (see below). As a pollutant, O3 increases Reactive Oxygen Species (ROS), induced through stomatal closure (Ainsworth et al., 2012). Transient exposure to high O3 induces a brief stomatal closure (3–6 min), with fully recovery within 30–40 min (Kollist et al., 2007; Vahisalu et al., 2010). However, longer exposure can lead to a significant, and often irreversible, reduction in stomatal conductance, leading to a decrease in CO2 uptake and impacting on the ability of the guard cells to close in response to further stress (Eamus et al., 1990; Morgan et al., 2003; McLaughlin et al., 2007). Both Venus and Mars are known to have atmospheric O3 layers, though weaker than that of Earth’s (Montmessin and Lefèvre, 2013). Whilst ground-based O3 pollution has not been reported, O3 exposure cannot be completely ruled out for future space missions.
Radiation is a form of energy that is emitted in the form of rays, electromagnetic waves, and/or particles. Plants and animals are exposed to radiation every day on Earth, and exposure is unavoidable. Travelling on an airplane, speaking on the phone, or even heating food can expose an individual to various doses of radiation (Radiation sources and dos, 2019). However, radiation composition and severity differs greatly between Earth and Space (Table 3). In space, and on Mars, the radiation environment consists of the solar electromagnetic spectrum plus a diverse array of charged particles from both within and outside the solar system (Nelson, 2016). This results in three kinds of radiation: particles trapped in the Earth’s magnetic field; particles shot into space during solar flares (solar particle events); and galactic cosmic rays (GCRs). Due to shielding by the Earth’s magnetosphere and atmosphere, terrestrial and low Earth orbit environments are largely shielded from these sources. In fact, the ionising radiation from galactic cosmic rays are so powerful that they even penetrate through highly-engineered spacecraft (Perez, 2019). Radiation in space can ultimately disrupt the typical function at a physiological and micro-level (Ionizing Radiation and Hu, 2018). The amount of radiation is dependent on three main factors: the altitude above Earth (higher altitudes = less protection from the Earth’s atmosphere), the individual susceptibility and physiological makeup (individual response to radiation), and the solar cycle (Perez, 2019). While non-ionising radiation is damaging, it can easily be shielded out of an environment (see below). Ionising radiation, however, is much more difficult to avoid as it has the ability to move through substances and alter their composition.
Examples of ionising radiation include alpha particles (a helium atom nucleus moving at very high speeds), beta particles (high-speed electrons or positrons), gamma rays, x-rays, and GCRs. Each of which are of a different energy and intensity (see Table 3) (Reitz, 2008). Examples of non-ionising radiation include radio frequencies, microwaves, infrared, visible light, and ultraviolet (UV) light. More damage can also be created by secondary particles that are propelled into motion by the primary radiation particle. Extraterrestrial, high-energy gamma rays include the gamma-ray background produced when cosmic rays (either high speed electrons or protons) collide with ordinary matter, producing pair-production gamma rays at 511 keV. Alternatively, secondary radiation (bremsstrahlung) is produced at energies of tens of MeV or more when cosmic-ray electrons interact with nuclei of sufficiently high atomic number. GCR are heavy, high-energy ions of elements that have had all their electrons stripped away as they journeyed through the galaxy at nearly the speed of light. While non-ionising radiation is damaging, it can easily be shielded out of an environment, as given for UV radiation.
Radiation effects in space are an issue of prominence and presents one of the main factors determining success of space travel (Mousseau and Møller, 2020). Manned space travel will require safe transit of astronauts plus the cultivation of a plant-based under conditions of prolonged exposure to ionising radiation. Although there are a significant number of studies concerning the effects of acute high dose rate exposures on plant genetics, growth, and development, much less is known concerning the effects of chronic low dose irradiation. This is particularly the case for high energy protons and heavy ions that are prevalent in space. Studies on Earth in regions of low dose irradiation, such as Chernobyl, Fukushima, and areas of India, indicate plants sustain high levels of mutation, genetic damage, reduced growth rates and reduced pollen and seed viability under a mix of alpha, beta and gamma exposure (Mousseau and Møller, 2020). However, the severity of symptoms is highly taxa specific and the actual response to the radiation environment in space is largely unknown.
The following sections will discuss different forms of radiation stress and indicate where radiation has been altered to manipulate plant growth.
PAR refers to the spectral range of solar radiation that can be used by plants; between 400 and 700 nm, and constitutes visible light. This is often referred to as the photosynthetic photon flux density; or PPFD, with each quantum of light called a photon. Leaf photosynthesis responds non-linearly to changes in PAR. Under highly heterogeneous light environments, PAR intensities will vary from limited to excessive. As the most variable environmental driver PAR imposes a two-fold challenge; the need to efficiently utilise as many photons as possible whilst simultaneously preventing harm caused by excess radiation. Achieving the optimal balance between these two states is critical to maximise both productivity and mitigate radiation-induced damage (Demmig-Adams et al., 2012). Visible light stress is common on Earth where intensities in excess of those required for photosynthesis lead to a buildup of excitation energy in the photosynthetic membrane, which leads to damage of the sensitive PSII (Murchie and Niyogi, 2011). Plants can regulate the amount of light they intercept through changes in leaf area, leaf angle or chloroplast movement, or on a molecular level, through acclimatory adjustments in light harvesting complex antenna size (known as state transitions). However, if excess energy has been absorbed, it can be dissipated via a number of different routes, broadly termed photoprotection. This includes the harmless dissipation of excess energy as heat in a process called non-photochemical quenching (Murchie and Ruban, 2020). The PAR intensity that causes damage depends upon the species or variety as well as the local environmental conditions (see below). Whilst high-light adapted plants including rice or maize can tolerate relatively high PPFDs (Foo et al., 2020), lettuce-type crops grown at PPFDs above 600 μmol m−2 s−1 will exhibit destruction of the photosynthetic apparatus in their upper leaves and decrease in total crop biomass (Zhang, 2016).
Photosynthetic acclimation (also termed photoacclimation) presents another subset of morphological and biochemical adjustments by which plants use to adapt to prevailing PAR conditions; whether an increase in intensity or a decrease (Walters, 2005; Grouneva et al., 2013; Tikkanen and Aro, 2014; Maksimov et al., 2017; Townsend et al., 2018). Photoacclimation can be broadly split into two different mechanisms: developmental acclimation and dynamic acclimation (Allakhverdiev and Murata, 2004; Kono and Terashima, 2014). Developmental acclimation refers to changes occurring during leaf development which are largely irreversible whereas dynamic acclimation is the ability for fully developed leaves to change their photosynthetic capacity (Tikkanen et al., 2011; Tikhonov, 2014). The extent of the propensity to acclimate will depend on the plant’s genotype, which will, to a greater or lesser extent, match the environment to which it is adapted through evolution.
The processes of photoprotection and photoacclimation will interact together and thus the actual productivity of a plant will depend upon the balance between different states. For example, exposure to excess light levels may lead to the enhancement of photoprotective mechanisms and in turn photoprotection may place an upper limit on the capacity to acclimate (e.g., Demmig-Adams et al., 2012 (Demmig-Adams et al., 2012)). Whilst the balance between these two states can be hard to discern under natural conditions, cultivation of plants under controlled conditions affords the ability to manipulate the light environment and thus alter plant growth.
The most important characteristics of the lighting regime for plants are the PPFD (Bykov, 1970; Knight and Mitchell, 1983; Knight and Mitchell, 1988; Fu et al., 2012; Weaver et al., 2019), photoperiod (Koontz and Prince, 1986; Lefsrud et al., 2006; Ali et al., 2009; Yakovtseva et al., 2015; Fukuda et al., 2019; Elkins and van Iersel, 2020; García-Caparrós et al., 2020; Hwang et al., 2020), light spectral composition (Goins et al., 1997; Lillo and Appenroth, 2001; Tamulaitis et al., 2005; Ohashi-Kaneko et al., 2007; Wu et al., 2007; Stutte et al., 2009; Lin et al., 2013; Ouzounis et al., 2015; Ptushenko et al., 2015), and lighting mode (pulse or continuous) (Olvera-González et al., 2013; Dong et al., 2015) (Figure 8).
Ample literature exists on the impact of he use of light emitting diodes (LEDs) for manipulation of these light traits. The design of lighting systems to obtain the optimal photosynthetic photon flux density (PPFD) remains a topic of ongoing research.
The light intensity, or PPFD, required for optimal plant growth is highly species and variety specific and is partly dependent upon the local environmental conditions in which a plant is grown. For example, a plant growing closer to the equator will be acclimated to higher PPFD (2000 μmol m−2 s−1). The maximum value strongly depends on the photosynthetic pathway employed (e.g., C3 versus C4) plus the leaf area (or biomass density) of the crop (Knight and Mitchell, 1983; Knight and Mitchell, 1988). For C4 species such as Maize (Zea mays), 2000 μmol m−2 s−1 is required for full photosynthetic saturation; whereas Chinese cabbage, Brassica chinensis, can reach full saturation at 672 μmol m−2 s−1 (Bykov, 1970). The optimal PPFD also depends upon season and latitude. For example, romaine lettuce (Lactuca sativa L.) requires a PPFD of 400 μmol m−2 s−1 for optimal growth during the winter (in a greenhouse), whereas 600 μmol m−2 s−1 is optimal late spring and early autumn (Fu et al., 2012). The PPFD can be fine-tuned: leaf area can increase at low dose and short times (150 μmol m−2 s−1; 20 h), while decreasing in the opposite direction (200 μmol m−2 s−1; 24 h) (Cho et al., 2020).
The photoperiod is an integral value of the daily dose of crop irradiation. It is often referred to as daily light integral (DLI). It affects biomass accumulation, as well as accumulation of secondary metabolites in many crops; the latter mediate ecological interactions, to produce a selective advantage in terms of plant survivability or fertility. Most crops require a circadian rhythm (“sleep–wake cycle”) with a specific day length for flowering, without which, yield will not be produced. Yet, there are also crops that are more flexible to the photoperiod, and we will report about them here.
An extension of the photoperiod at constant radiation intensity can increase the growth: the weight of loose-leaf lettuce (Lactuca sativa L.) almost doubled when increasing the photoperiod from 16 to 24 h (Koontz and Prince, 1986). A DLI of 12 mol·m−2·d−1 was provided in a greenhouse over 12, 15, 18, or 21-h photoperiods using adaptive lighting control. Similar effects are given for the individual plant parts, e.g. increase in shoot dry mass of Rudbeckia fulgida (30%), root dry mass (24%), plant height (14%), leaf area (16%), and chlorophyll content index (48%) when irradiated for 21 instead of 12 h (148). Further, increased lightening impacts the shape of plant parts (leaves become broader) and molecular functions such as the antioxidant capacity and light use efficiency of lettuce (Cho et al., 2020).
Another parameter is the number of light-dark cycles in a given photoperiod. For example, an increasing number of cycles from 18 h (light)-6 h (dark) to 9 h (light)-3 h (dark) to 6(light)-2(dark) within 24 h altered the chemical composition of tomato and cucumber seedlings (García-Caparrós et al., 2020). Leaf proline content showed the highest value in the treatment with 18 h of light and 6 h of dark. The same result was found for strawberries (Yakovtseva et al., 2015). A too-short exposure (cycle time), may cause restriction in flowering or seed abortion; thus, defining a critical photoperiod threshold that must be reached to initiate developmental stages (Jackson, 2009).
In addition, the photoperiod may be manipulated in the dark cycle by overnight supplemental lighting (OSL). While this is known to increase the growth of some crops (Okushima et al., 2012; Yao et al., 2021), it also induces physiological stress, in the example of lettuce, resulting in a reduction of photosynthetic efficiency by 10% (Fukuda et al., 2019). Similarly, the light cycle may be altered: exposure to monochromatic light can be identified (misinterpreted) by plants as a high-intensity white light, since the photosynthetic apparatus and the system of specific photoreceptors are sensitive to different spectral regions.
The light spectral composition affects plant’s growth and development (Figure 9) (Ouzounis et al., 2015). The spectral composition can also affect taste components such as the aromatic intensity in coriander (Coriandrum sativum L.) (McAusland et al., 2020). As such, many horticultural-focused studies aim to determine the optimal light “recipe” (Smith et al., 2017). Changes in metabolic and signalling pathways can arise when supplying different spectral compositions to plants. Phytohormone content, the ratio of their active and inactive forms, and regulation of hormone transport plus the activity of key metabolic enzymes such as nitrate reductase can increase or decrease. Light has a complex effect, and this happens by changes of so-called transcriptional, translational, and post-translational levels, with further details given in (Lillo and Appenroth, 2001).
FIGURE 9. Effect of light wavelengths on chrysanthemum (A), tomato (B) and lettuce (C). The light absorption maxima of the intact leaves are in the bands of 400–490 nm and 660–680 nm, which is close to maximum absorption bands of a chlorophyll extract (420–460 nm and 640–660 nm) (Mortensen and Strømme, 1987).
Typically, red light (600–660 nm) and blue light (400–490 nm) wavelengths are most effectively absorbed by chlorophylls and are required as the main energy sources for photosynthesis (Goins et al., 1997; Son and Oh, 2013). The function of red and blue light differs. Red LED generally induces plant growth by increasing fresh and dry plant weight, plant height, and leaf area as well as pest stability (Wang et al., 2009; Heo et al., 2012). In comparison, blue LED influences photosynthetic function, chlorophyll formation, and chloroplast development and can influence pest and disease prevalence (Wang et al., 2009; Johkan et al., 2010). The synergetic effect of both blue and red LEDs is known to enhance the growth of various herbs and vegetables. Combined narrow-band red and blue light stimulation of growth is most pronounced low PPFD levels, below 200 μmol m−2 s−1 (Ptushenko et al., 2015). Some crops show strong positive effects in response to unilateral red light (Millar et al., 2010). Addition of far-red light (730 nm) to red light shows reduced growth and bioprotective compounds in the example of lettuce but is associated with shade avoidance strategies (Stutte et al., 2009).
Monochromatic light usually is unable to ensure normal plant growth (Goins et al., 1997). This can lead to a decrease in CO2 absorption rate and therefore reduced plant biomass accumulation, as shown for monochromatic red light irradiation (640 nm) (Tamulaitis et al., 2005). Exceptions to this are known; Komatsuna spinach produced higher biomass by monochromatic irradiation from red fluorescent lamps, as compared to control experiments with white bulbs (Ohashi-Kaneko et al., 2007). Stem length and seedling weight might increase for some plants grown under monochromatic blue light (Wu et al., 2007). A few plants grow well with dichromatic (red-blue) light, while others need a combination of more spectral bands.
Green light (500–600 nm) is also able to promote plant growth (Smith et al., 2017). This can be attributed to the greater penetration of green wavelengths through the leaf and canopy profile, contributing to carbon gain and signalling in lower cellular and canopy layers (Smith et al., 2017). In addition, the green light has been proposed to be beneficial for human-based diagnostics of biotic stress. The absence of green light may give plants unhealthy appearance. Previously NASA studies of leaves grown in bioregenerative life-support systems for space under dichromatic red and blue LEDs have grey/black appearance, which are reported to be difficult to discern any colour-related stress symptoms (Kim et al., 2005). Similarly, green LED light benefits human health through the generation of a nicer working environment (Smith et al., 2017).
The lighting mode, i.e., whether the radiation is applied continuously or pulsed, has an influence on plant performance because light-absorbing molecules in chloroplasts become inactivated after absorbing a light quantum (Tennessen et al., 1995). Therefore, continuous lighting can only be 100% efficient if operated below the value required to saturate photosynthesis. Pulsed lighting can be used to moderate the excitation of the photosynthetic membrane whilst simultaneously saving light energy and enhancing crop energy efficiency. Essential for the latter is to find a dark-light cycle optimisation; which will be species and light intensity specific.
For several crops, short (≤100 µs), high-intensity light pulses interspersed with dark periods could fulfill the energy needs at relatively low PPFD levels and can result in a higher quantum use efficiency, as compared to continuous lighting. For example, lettuce plants grown under pulsed lighting with a period of 100 ms and duty cycle (the ratio between the duration of light-on to the total light-on plus light-off period) of 50% showed higher biomass and photosynthetic rate (Kanechi et al., 2016). Similar results were also seen in potato seedlings (Jao and Fang, 2004). Pulsed LED irradiation of lettuce could save energy at a plant growth similar to that under continuous light (Son et al., 2016). In comparison, a parametric study on the duty cycle effect on the photosynthetic characteristics and productivity of wheat resulted in negligible or reduced crop yield (Dong et al., 2015). Optimal pulsed lighting may be achieved by synchronising the light frequencies to the fluorescence emission characteristics of chlorophyll, such as maximum fluorescence, minimum fluorescence, the fluorescence emission in steady-state, and maximum efficiency of the photosystem [example: 0.1 Hz to 100 kHz; 50% duty cycle, (Jao and Fang, 2004)].
Ultraviolet (UV) radiation (100—400 nm) can cause plant growth stress. UV radiation will differ in space compared to Earth and affects plant’s growth dependent on its exact spectral profile, i.e. presenting short or long wavelength spans. UV radiation can be split into three different categories based on wavelength, each of which has a differential effect on plant growth. UV-A refers to wavelengths from 315 to 400 nm; UV-B from 280 to 315 nm; and UV-C from 100 to 280 nm. That Earth-bound protection against damage via UV radiation, does not exist in space; providing a very different UV signature. UV-C rays are almost completely absorbed by Earth’s atmosphere, whilst UV-B rays are absorbed ∼95% by the ozone layer. UV-A rays account for 95 percent of UV radiation that reaches the Earth’s surface.
Whilst UV-A and -B mainly affect morphogenesis and phototropism, UV-B and -C trigger secondary metabolite production and are strongly absorbed by nuclear, mitochondrial and chloroplastic DNA (Robson et al., 2015; Vanhaelewyn et al., 2020). The exact response to UV stress is species specific. Exposure to UV-B over several days, results in increased damage to seedlings of bean (Phaseolus vulgaris) relative to barley (Hordeum vulgare) and radish (Raphanus sativus), while corn (Zea mays) was hardly influenced at all (Escobar-Bravo et al., 2017). In all plant species, the fresh weight, the leaf area, the amounts of chlorophylls, carotenoids, and the galactolipids of the chloroplasts were reduced under UV-B exposure (Murakami and Yamada, 1988). The fresh weights fell proportionally with the chlorophylls and carotenoids. All UV-B irradiated plants showed a rise in their protein content compared to the control plants as well as in the flavonoid content (for barley and radish by about 50%). The effects are more pronounced with increasing UV-B intensity. At the highest UV-B fluence rate, the flavonoid content of barley leaves rose reached 180% of the value in the control plants. Scorching appeared in the form of bronze leaf discoloration at the highest UV-B intensity (Lizana et al., 2009). Deformations on supramolecular structures such as organelles and membranes are observed as well as visible macroscopic changes on parts of the crop plants (leaves) (Tevini et al., 1981). Embryonic evidence suggests that by proper crop selection negative effects can be diminished: less leafy vegetables/plants are less damaged.
UV-C rays are generally considered to be one of the most harmful factors, causing damage to epidermal and mesophyll cells of plants (Kara, 2013). However, UV-C light can increase branching in some plants while reducing final, plant height. This effect may avoid the need to pinch off plant shoot tips or to apply plant growth regulators (Bridgen, 2016).
Alpha (α) particles have a positive charge and are identical to helium nuclei, consisting of two protons and two neutrons. They result from the radioactive decay of heavy elements such as radium, thorium, uranium, and plutonium. Because of their double-positive charge, α particles have great ionising power, but their large mass results in very little penetration. Alpha irradiation, simulated by Earth nuclear sources, has pronounced effects on Arabidopsis thalina seedlings, both morphologically and physiologically (Biermans et al., 2015). At a low dose rate, the redox balance is controlled and the biomass is stable, whereas at a high dose rate crop growth, transfer, and redox balance decline. With more detail on the dose-dependency, effects on roots and shoots have been monitored. At up to 1910–2,400
Proton radiation is, with varying intensity, found in the Inner and Outer Belt in the near-Earth related space (Table 3). Proton irradiation, simulated by Earth nuclear sources, showed a significant morphological effect on the germination of soybean growth, as well as on survival and growth of the seedlings (Figure 10) (Im et al., 2017). Although germination rate increased with the increase of proton dose rate, the survival rate decreased significantly. From a dose of 100 Gy onwards, not only the plant height but also the root and shoot weight declined significantly Figure 10). Also, abnormal branch stem, leaves, and even chlorophyll mutations have been found in plants grown under proton radiation.
FIGURE 10. Abnormal phenotypes of proton beam-irradiated soybean cultivars. (A) Abnormal leaf (239 Gy), (B) abnormal stem (117 Gy), (C) variegated leaf (365 Gy), (D) bright green leaf (239 Gy) (Im et al., 2017).
Beta radiation is, found with varying intensity in the Inner and Outer Belts in the near-Earth-related space. Simulated by Earth nuclear sources, beta radiation stimulates the growth of Phaseolus vulgaris at a low dose, but damages roots and shoots, decreases the number of branches and leaves significantly, at high dose rates (Marcu et al., 2013).
Occasionally, giant explosions called solar flares and coronal mass ejections (CME) occur on the surface of the Sun and release massive amounts of energy out into space in the form of x-rays, gamma rays, and streams of protons and electrons called solar particle events (SPE) (Jan et al., 2012). They are absorbed in the Earth’s surface but will be present in space.
Gamma rays are not necessarily detrimental to crop growth. Seedlings exposed to relatively low doses of gamma rays (1–5 Gy) developed normally (Wi et al., 2007). Yet at higher doses, the destructive nature of gamma rays becomes evident. Exposure to gamma radiation is used as mechanism to mutagenise the genetic code of plants for breeding (Beyaz and Yildiz, 2017). It is possible that radiation doses in the cosmos could be used to select for better space adapted lines to improve the suitability of crop cultivars grown in space. However, current plant species are often sensitive to gamma radiation-induced damage. At a dose of 50 Gy, the crop growth ias significantly inhibited and cell walls and their constituents become deformed (Figure 11). Chloroplasts are extremely sensitive to gamma irradiation compared to other cell organelles, particularly resulting in swelling to thylakoids.
FIGURE 11. Schematic drawn from transmission electron micrographs of cell wall structures of Empire apples, showing the transition from fresh unirradiated cells towards gamma ray-irradiated cells (1 kGy) then stored for 6 weeks (Kovács and Keresztes, 2002).
Plant stress leads to plant changes on diverse levels of scale (Figure 12). It is relevant to transform the knowledge of stressor-induced plant changes into physiological targets for plant growth in space across these scales. Depending on the stressor, some scale-relevant changes are better documented than others (Table 4). On Earth, heat-, drought-, salt- and visible-light-induced stressors are well documented; the same holds for micro-gravity-induced stressors in space. The role of other radiation stressors than visible light, or of gas composition is less documented.
FIGURE 12. Macroscopic, mesoscopic and microscopic classification of changes of plants when exposed to various kinds of plant stressors.
As space-based plant-growing systems get more complicated, maintenance of the stability of growth conditions becomes more difficult to achieve. In general, whilst closed-loop systems may be easier to maintain, size or other constraints may necessitate the transition to more open systems. This increases the probability of both abiotic and biotic stressors as open systems are exposed to microbial disease outbreaks due to interactions between the crew-habitable modules and the plant-growth modules (Schuerger 2021). Thus space farming requires consideration of multiple combined stressors as well as the identification of which stressors may be tolerated and which must be limited.
Plants on Earth are exposed to a complex set of biotic and abiotic stressors and, in response, have developed a complex set of responses. Interactions of stressor effects common, however whether the response is additive or a result of more complex behavior, e.g. synergistic, plus the magnitude of damage and/or symptoms experiences, dependent upon the stressors and species in question. For example, the effect of salt stress will vary depending on climatic conditions, light intensity, plant species or soil conditions (Tang et al., 2015). Salt stress and CO2 concentration show interactive effects. In the halophyte Aster tripolium L. combined salt and CO2 stress was shown to affect plant growth, photosynthesis, water relations, and chemical composition (Geissler et al., 2009). Visible light and CO2 concentration also show interactive effects. The light saturation point of cucumber changes with temperature and CO2 concentration [102]. Similarly, there are interactions between radiation and other environmental stressors (e.g., temperature, drought, heavy metals) that may play important roles in determining susceptibility to radiation induced stress (Mousseau and Møller, 2020). Photoprotective processes and light-induced damage become enhanced under thermal stress, particularly under chilling stress (Demmig-Adams et al., 2012). The extent of combined chilling and radiation stress on photosynthetic efficiency is species specific, with plants performing C4 photosynthesis affected to a greater extent (Osborne et al., 2008). Even within one stressor class, such as radiation (Radiation Stress), combined effects of measures are found. For example, when increasing both PPFD and DLI, different parameters of the plant growth display linear or saturating-curve relationships (Hwang et al., 2020).
Certain stressors are likely to occur in combination and elicit similar symptoms. Water deficit or drought stress restrict growth processes directly via turgor loss but also affect CO2 assimilation through stomatal closure and by reducing transpirational cooling, increasing leaf temperature (Tardieu et al., 2018). High temperatures commonly act in combination with drought, high light intensities and an increased water vapour deficit (Grossiord et al., 2020). Hypobaria and hypoxia generally occur in combination (Atmospheric Stress—low Pressure or Atmospheres of Different Composition).
In space, the combination of stressors will likely differ than those on Earth. Plants grown under microgravity are subject to combined abiotic stressors which may promote microbial growth and thus disease severity. Aboard an 8-day mission on the NASA shuttle, Discovery, Wheat (Triticum aestivum cv. Super Dwarf) developed girdling of leaf sheaths, chlorosis and necrosis of leaf and root tissues as a result of infection by fungal pathogen Neotyphodium chilense (Bishop et al., 1997). However, on Earth, symptoms were only exhibited following 4 + days growth in closed containers, plus corresponding infection was witnessed in other cereals (wheat cv. Malcolm, orchard grass, barley and maize) when grown together. Similarly, restricted airflow contributed to the development of the root fungal pathogen Phytophthora sojae on soybean plants (Glycine max cv. ‘Williams 82’) aboard the shuttle flight STS-87 (Ryba-White et al., 2001). In late 2015 aboard the ISS in the Veggie growth system, an outbreak of the fungal pathogen Fusarium oxysporum, affected the growth of Zinnia hybrida cv. ‘Profusion’ (Schuerger et al., 2021). Plants developed chlorosis, leaf curling, damaged leaves and stems plus necrosis. Pathogenicity assays on Earth indicated that symptoms matched those in situ when plants were stressed with high-water exposure, as experienced in veggie, and that Fusarium oxysporum was able to act as an opportunistic pathogen on high-water stressed plants. Together, these studies indicate the importance of combined abiotic and biotic stressors on plant growth and mortality, and the potential implications of closed systems containing pathogens with a large host range.
Whilst many of the stressors discussed in this review can be managed so as not to limit plant growth in space, large-scale tests for food production in reduced gravity are still lacking. Furthermore, a number of viable technologies for space agriculture need to be developed including optimised lighting; efficient irrigation and nutrient-delivery; and precise atmospheric controls for temperature, humidity and gas composition. Whether necessary growth resources are obtained via in situ resource utilisation or must be transported from Earth will also determine the overall design of future plant-growth habitats. Selecting the right crops to grow in space is also essential. Given the limited amount of room available on board a spacecraft, plants with reduced size but high yields need to be developed: for example, dwarf varieties of wheat, cherry tomato, rice, pepper, soybean and pea have been successfully grown in orbit and in simulated planetary habitats. The following section will discuss adaptive mechanisms and engineering approaches by which we can reduce plant stress.
A key question of this review is, can space stressors be used positively for plant growth, or do we have to accept that space is a hostile environment? With the view that stressors usually have a negative impact, and that a positive Eustress still needs to be developed, the question is how to mitigate the threat of stressors. One way is shielding by construction material, both by outer shielding of a space habitat and inner shielding by means of a biogenerative life-support system. Such shielding has been achieved to a certain degree for existing spacecrafts. For example, while the International Space Station (ISS) is able to mitigate stressor impacts by heat, air, and other control management systems, this nonetheless does not come to a zero level. Thus, conditions on the ISS and other spacecraft still differ from those on Earth.
Yet, there is some indication that some of the space stressors can be used as Eustress, or have negligible impact on plant growth as long as other conditions are maintained as witnessed for hypobaria (Atmospheric Stress—low Pressure or Atmospheres of Different Composition). This can be conceptualised with examples from radiation, the features of which differ greatly in space compared to Earth (Radiation Stress). One solution would be toeliminate radiation entirely via shielding, with growth radiation applied via LEDs. An alternative approach would be to explore how to modulate the existing space radiation to stimulate plant growth, e.g. concerning certain stages of development, earlier flowering, and lateral bud development (Molas and Kiss, 2009). The best solution will be to use the solar and visible irradiation “as is”—meaning to rely on different photoperiods, different intensities, and different spectral compositions. Plants have been shown to be flexible to adapt to this. Additional technology opportunities of modern horticulture may assist such as pulsed lightening. This may require filtering parts of the irradiation that are detrimental, and leaving parts that are considered beneficial (Baldwin et al., 2009). Nonetheless, the use of screening materials to manipulate light spectral quality can be further advanced, also known as climate screens. These materials are typically used inside greenhouses to control humidity and temperature or remove harmful wavelengths of light (Kotilainen et al., 2018). Dependent upon the type of screen used, a large difference can be seen in both the fraction of global irradiance, which can pass through the screen and the spectral irradiance of the resulting environment. This suggests the potential for engineering appropriate screens for space conditions.
Another solution is that the plant itself may acclimate to the new conditions or stress-related signalling pathways may be altered to improve resilience. The signalling of a stress event, whether abiotic or biotic has been well established and this normally takes the form of calcium, ROS, hydraulic signals (Fichman and Mittler, 2020). ABA is also involved in ROS/Ca2+ associated closure in response to very high light on both a local and long-distance scale, activating stomatal closure at a systemic level (Takahashi and Shinozaki, 2019). A considerable amount of evidence has shown that phytohormones are signals connecting root and shoot, triggering responses to external stress (Llanes et al., 2014). Pools of polyamines in plants, particularly a major group called putrescines, could be manipulated in space and on the ground to reduce stress reactions (Llanes et al., 2014). Alternatively to stress pathways, control of plant function can also serve to improve plant growth in diverse habitats. Co-ordination between distal plant parts is well known, for example the synthesis of ABA in roots in response to drying soil that leads to stomatal closure when sensed by guard cells (Takahashi and Shinozaki, 2019). Strigolactones, microRNAs, Jasmonic acid (JA), peptides and cytokinins all are involved in some form of shoot–root communication (Ko and Helariutta, 2017). Similarly, developmental and dynamic acclimation (see above) is subject to influence from systemic signals to light and CO2 (Coupe et al., 2006; Kangasjärvi et al., 2009).
These signalling mechanisms can be exploited or improved to maximise productivity under space conditions. Modern gene manipulation may have a pivotal role for photoprotective pathways to increase the speed of response to changes in light intensity and to increase the crop yield, as shown in the examples of Tobacco (Nicotinia) and rice (Oryza sativa) (Kromdijk et al., 2016; Hubbart et al., 2018).
Plant stressors will happen in space in a way we cannot predict,yet, we must rely on Earth experiences as the first and best approximation. Here, Earth stressors and known Space stressors have been summed up, and perspectives for translation to plant growth have been given. We have aimed to provide insight to above space stressor problem from the standpoint of both biologists and system engineers. Much is known about common stressors on Earth such as visible light stress, thermal stress, drought and salinity. Many of these known stressors can be mitigated by proper systems engineering towards a biogenerative life-support system in space. The unknown impact is the effect of cosmic radiation, including alpha, beta, gamma, and x-ray irradiation unknown on Earth. We discuss ways in which the combination of these stressors may influence plant growth and the signalling and metabolic pathways involved in stress perception and response. Finally, we propose potential targets to engineer and manipulate the genetic composition of plants to improve adaptation to stress in space. As a first step, it might be sufficient to select those plants which are able to do best in space, based on the limited current knowledge from the wide selection of Earth plant varieties. It might be good to expose those plant species in a large experimental test on Earth over several years to the plant stressors expected for a Moon habitat and spacecraft travel to Mars. The plants that best resist the stress may be selected, and we may use those mutated species for our space journeys and exploration.
Space farming will finally produce new crops, meaning modifications not seen on Earth. The food need of astronauts in deep space explorations and long-term stay on extraterrestrial planets will likely be different from Earth. The ‘Moon cantina’ will not rely on Earth dishes such as pizza, steak, or Sushi. Thus, space farming will finally lead to a new type of cooking and dishes.
One way to consider that change would be based on cooked food supply from the first instance. On Earth, revolutionary cooking concepts have been proposed such as the Note-by-Note Cooking (NBN). Note by Note cuisine is a style of cooking based on molecular gastronomy (This, 2006; Rossi-Wilcox, 2007; This, 2007). Dishes are made using pure compounds instead of using animal or plant tissues. There is a big advantage in terms of water requirements, as there is no need to transport it when using NBN cooking. In principle, it can fully replace traditional food, as has been shown for coma patients in hospitals. In practical terms, NBN may rely on the cultivation of pulses, and extraction of proteins and polysaccharides (starch), followed by transfer of remaining plant residues to insects, so that they can make other proteins. This may be facilitated by the cultivation of genetically modified plants in order to produce the right cocktail of nutrients, in higher concentrations, supporting the NBN cooking.
VH: Conceptualization; Methodology; Investigation; Writing—Original Draft; Supervision. SL: Investigation; Writing—Original Draft. NT: Conceptualization; Methodology; Writing—Review and Editing. ME-G: Conceptualization; Visualization; Writing—Review and Editing. OZ: Conceptualization; Methodology; Writing—Review and Editing. MK: Conceptualization; Methodology; Writing—Review and Editing. ER: Conceptualization; Methodology; Writing—Review and Editing. HT: Conceptualization; Methodology; Writing—Review and Editing. SW: Conceptualization; Methodology; Writing—Review and Editing. IF: Conceptualization; Methodology; Writing—Review and Editing. MG: Writing—Original Draft; Supervision. AB: Conceptualization; Methodology; Investigation; Writing—Original Draft; Supervision.
VH acknowledges the financial support from The Andy Thomas Centre for Space Resources (ATCSR) at The University of Adelaide, Australia. We also acknowledge the Adelaide-Nottingham joint PhD scholarship scheme.
The authors declare that the research was conducted in the absence of any commercial or financial relationships that could be construed as a potential conflict of interest.
All claims expressed in this article are solely those of the authors and do not necessarily represent those of their affiliated organizations, or those of the publisher, the editors and the reviewers. Any product that may be evaluated in this article, or claim that may be made by its manufacturer, is not guaranteed or endorsed by the publisher.
Acosta-Motos, J., Ortuño, M., Bernal-Vicente, A., Diaz-Vivancos, P., Sanchez-Blanco, M., and Hernandez, J. (2017). Plant Responses to Salt Stress: Adaptive Mechanisms. Agronomy 7 (1), 18. doi:10.3390/agronomy7010018
Ainsworth, E. A., and Long, S. P. (2005). What Have We Learned from 15 Years of Free‐air CO 2 Enrichment (FACE)? A Meta‐analytic Review of the Responses of Photosynthesis, Canopy Properties and Plant Production to Rising CO 2. New Phytol. 165 (2), 351–372. doi:10.1111/j.1469-8137.2004.01224.x
Ainsworth, E. A., Yendrek, C. R., Sitch, S., Collins, W. J., and Emberson, L. D. (2012). The Effects of Tropospheric Ozone on Net Primary Productivity and Implications for Climate Change. Annu. Rev. Plant Biol. 63, 637–661. doi:10.1146/annurev-arplant-042110-103829
Ali, M. B., Khandaker, L., and Oba, S. (2009). Comparative Study on Functional Components, Antioxidant Activity and Color Parameters of Selected Colored Leafy Vegetables as Affected by Photoperiods. J. Food Agric. Environ. 7 (3-4), 392–398.
Allakhverdiev, S. I., and Murata, N. (2004). Environmental Stress Inhibits the Synthesis De Novo of Proteins Involved in the Photodamage-Repair Cycle of Photosystem II in Synechocystis Sp. PCC 6803. Biochim. Biophys. Acta (Bba) - Bioenerg. 1657 (1), 23–32. doi:10.1016/j.bbabio.2004.03.003
Andre, M., and Massimino, D. (1992). Growth of Plants at Reduced Pressures: Experiments in Wheat - Technological Advantages and Constraints. Adv. Space Res. 12 (5), 97–106. doi:10.1016/0273-1177(92)90015-p
Balachandran, S., Hurry, V. M., Kelley, S. E., Osmond, C. B., Robinson, S. A., Rohozinski, J., et al. (1997). Concepts of Plant Biotic Stress. Some Insights into the Stress Physiology of Virus-Infected Plants, from the Perspective of Photosynthesis. Physiol. Plant 100 (2), 203–213. doi:10.1034/j.1399-3054.1997.1000201.x
Baldwin, C. M., Liu, H., McCarty, L. B., Luo, H., Wells, C. E., and Toler, J. E. (2009). Impacts of Altered Light Spectral Quality on Warm Season Turfgrass Growth under Greenhouse Conditions. Crop Sci. 49 (4), 1444–1453. doi:10.2135/cropsci2008.07.0412
Barringer, C., and Abadie, L. (2013). Microbial Creatures in Space US: National Aeronautics and Space Administration. Available from: https://www.nasa.gov/exploration/humanresearch/Microbial-Creatures-in-Space.html.
Beyaz, R., and Yildiz, M. (2017). The Use of Gamma Irradiation in Plant Mutation Breeding. Plant Eng., 33–46. doi:10.5772/intechopen.69974
Biermans, G., Horemans, N., Vanhoudt, N., Vandenhove, H., Saenen, E., Van Hees, M., et al. (2015). Biological Effects of α-radiation Exposure by 241Am in Arabidopsis thaliana Seedlings Are Determined Both by Dose Rate and 241Am Distribution. J. Environ. Radioactivity 149, 51–63. doi:10.1016/j.jenvrad.2015.07.007
Bishop, D. L., Levine, H. G., Kropp, B. R., and Anderson, A. J. (1997). Seedborne Fungal Contamination: Consequences in Space-Grown Wheat. Phytopathology 87 (11), 1125–1133. doi:10.1094/phyto.1997.87.11.1125
Blum, A. (2011). Plant Water Relations, Plant Stress and Plant Production. Plant Breeding for Water-Limited Environments: Springer, 11–52. doi:10.1007/978-1-4419-7491-4_2
Bose, J., Munns, R., Shabala, S., Gilliham, M., Pogson, B., and Tyerman, S. D. (2017). Chloroplast Function and Ion Regulation in Plants Growing on saline Soils: Lessons from Halophytes. J. Exp. Bot. 68 (12), 3129–3143. doi:10.1093/jxb/erx142
Braun, M., Böhmer, M., Häder, D-P., Hemmersbach, R., and Palme, K. (2018). Gravitational Biology I: Gravity Sensing and Graviorientation in Microorganisms and Plants: Springer.
Bykov, O. (1970). Studies on Photosynthesis of Vegetable Crops. Trud Priklad Bot. Genet. Selekc(trans, Appl. Bot. Genet. Pl-breed) 42 (3), 139–148.
Charles, W., and Lloyd, S. (2021). Space Radiation: NASA. Available from: https://www.nasa.gov/sites/default/files/atoms/files/space_radiation_ebook.pdf.
Cho, J. Y., Yoo, K. S., Kim, J., Choi, B. J., and Growth, O. W. (2020). Bioactive Compounds of Lettuce as Affected by Light Intensity and Photoperiod in a Plant Factory Using External Electrode Fluorescent Lamps.
Choi, W.-G., Barker, R. J., Kim, S.-H., Swanson, S. J., and Gilroy, S. (2019). Variation in the Transcriptome of Different Ecotypes ofArabidopsis Thalianareveals Signatures of Oxidative Stress in Plant Responses to Spaceflight. Am. J. Bot. 106 (1), 123–136. doi:10.1002/ajb2.1223
Corey, K. A., Barta, D. J., and Wheeler, R. M. (2002). Toward Martian Agriculture: Responses of Plants to Hypobaria. Life Support. Biosph. Sci. 8 (2), 103–114.
Correll, M. J., Pyle, T. P., Millar, K. D. L., Sun, Y., Yao, J., Edelmann, R. E., et al. (2013). Transcriptome Analyses of Arabidopsis thaliana Seedlings Grown in Space: Implications for Gravity-Responsive Genes. Planta 238 (3), 519–533. doi:10.1007/s00425-013-1909-x
Coupe, S. A., Palmer, B. G., Lake, J. A., Overy, S. A., Oxborough, K., Woodward, F. I., et al. (2006). Systemic Signalling of Environmental Cues in Arabidopsis Leaves. J. Exp. Bot. 57 (2), 329–341. doi:10.1093/jxb/erj033
Demmig-Adams, B., Cohu, C. M., Muller, O., and Adams, W. W. (2012). Modulation of Photosynthetic Energy Conversion Efficiency in Nature: from Seconds to Seasons. Photosynth Res. 113 (1), 75–88. doi:10.1007/s11120-012-9761-6
Dijkstra, C. E., Larkin, O. J., Anthony, P., Davey, M. R., Eaves, L., Rees, C. E. D., et al. (2011). Diamagnetic Levitation Enhances Growth of Liquid Bacterial Cultures by Increasing Oxygen Availability. J. R. Soc. Interf. 8 (56), 334–344. doi:10.1098/rsif.2010.0294
Dong, C., Shao, L., Liu, G., Wang, M., Liu, H., Xie, B., et al. (2015). Photosynthetic Characteristics, Antioxidant Capacity and Biomass Yield of Wheat Exposed to Intermittent Light Irradiation with Millisecond-Scale Periods. J. Plant Physiol. 184, 28–36. doi:10.1016/j.jplph.2015.06.012
Drew, M. C. (1997). Oxygen Deficiency and Root Metabolism: Injury and Acclimation under Hypoxia and Anoxia. Annu. Rev. Plant Physiol. Plant Mol. Biol. 48 (1), 223–250. doi:10.1146/annurev.arplant.48.1.223
Dunbar, B. (2007). Human Needs: Sustaining Life during Exploration America: NASA. Available from: ,would%20be%20put%20in%20jeopardy.&text=Pioneers%20either%20brought%20food%20and,gathered%20them%20along%20the%20wayhttps://www.nasa.gov/vision/earth/everydaylife/jamestown-needs-fs.html#:∼:text=To%20endure%20these%20long%20voyages.
Eamus, D., Barnes, J. D., Mortensen, L., Ro-Poulsen, H., and Davison, A. W. (1990). Persistent Stimulation of CO2 Assimilation and Stomatal Conductance by Summer Ozone Fumigation in Norway spruce. Environ. Pollut. 63 (4), 365–379. doi:10.1016/0269-7491(90)90141-x
Eckardt, N. A., and Portis Jr, A. R. (1997). Heat Denaturation Profiles of Ribulose-1,5-Bisphosphate Carboxylase/Oxygenase (Rubisco) and Rubisco Activase and the Inability of Rubisco Activase to Restore Activity of Heat-Denatured Rubisco. Plant Physiol. 113 (1), 243–248. doi:10.1104/pp.113.1.243
Ekblaw, A. C. (2020). Self-aware Self-Assembly for Space Architecture: Growth Paradigms for In-Space Manufacturing: Massachusetts Institute of Technology.
Elkins, C., and van Iersel, M. W. (2020). Longer Photoperiods with the Same Daily Light Integral Improve Growth of Rudbeckia Seedlings in a Greenhouse. HortScience 1 (aop), 1–7. doi:10.21273/hortsci15200-20
Eric, R., Marie Noelle, V., Alain, Z., and Vaughan, H. (2009). Chapter 2 Cold Signalling and Cold Acclimation in Plants. Adv. Bot. Res. 49, 35–150.
Escobar-Bravo, R., Klinkhamer, P. G. L., and Leiss, K. A. (2017). Interactive Effects of UV-B Light with Abiotic Factors on Plant Growth and Chemistry, and Their Consequences for Defense against Arthropod Herbivores. Front. Plant Sci. 8, 278. doi:10.3389/fpls.2017.00278
Farooq, M., Wahid, A., Kobayashi, N., Fujita, D., and Basra, S. M. A. (2009). Plant Drought Stress: Effects, Mechanisms and Management. Sustainable Agric., 153–188. doi:10.1007/978-90-481-2666-8_12
Ferl, R. J., Schuerger, A. C., Paul, A. L., Gurley, W. B., Corey, K., and Bucklin, R. (2002). Plant Adaptation to Low Atmospheric Pressures: Potential Molecular Responses. Life Support. Biosph. Sci. 8 (2), 93–101.
Fichman, Y., and Mittler, R. (2020). Rapid Systemic Signaling during Abiotic and Biotic Stresses: Is the ROS Wave Master of All Trades? Plant J. 102 (5), 887–896. doi:10.1111/tpj.14685
Flowers, T. J., and Colmer, T. D. (2015). Plant Salt Tolerance: Adaptations in Halophytes. Ann. Bot. 115 (3), 327–331. doi:10.1093/aob/mcu267
Foo, C. C., Burgess, A. J., Retkute, R., Tree-Intong, P., Ruban, A. V., and Murchie, E. H. (2020). Photoprotective Energy Dissipation Is Greater in the Lower, Not the Upper, Regions of a rice Canopy: a 3D Analysis. J. Exp. Bot. 71 (22), 7382–7392. doi:10.1093/jxb/eraa411
Fu, W., Li, P., and Wu, Y. (2012). Effects of Different Light Intensities on Chlorophyll Fluorescence Characteristics and Yield in Lettuce. Scientia Horticulturae 135, 45–51. doi:10.1016/j.scienta.2011.12.004
García-Caparrós, P., Sabio, F., Barbero, F. J., Chica, R. M., and Lao, M. T. (2020). Physiological Responses of Tomato and Cucumber Seedlings under Different Light-Dark Cycles. Agronomy 10 (7), 945. doi:10.3390/agronomy10070945
Geim, A. K., Simon, M. D., Boamfa, M. I., and Heflinger, L. O. (1999). Magnet Levitation at Your Fingertips. Nature 400 (6742), 323–324. doi:10.1038/22444
Geissler, N., Hussin, S., and Koyro, H-W. (2009). Interactive Effects of NaCl Salinity and Elevated Atmospheric CO2 Concentration on Growth, Photosynthesis, Water Relations and Chemical Composition of the Potential Cash Crop Halophyte Aster Tripolium L. Environ. Exp. Bot. 65 (2-3), 220–231. doi:10.1016/j.envexpbot.2008.11.001
Goins, G. D., Yorio, N. C., Sanwo, M. M., and Brown, C. S. (1997). Photomorphogenesis, Photosynthesis, and Seed Yield of Wheat Plants Grown under Red Light-Emitting Diodes (LEDs) with and without Supplemental Blue Lighting. J. Exp. Bot. 48 (7), 1407–1413. doi:10.1093/jxb/48.7.1407
Goldmann, D. A., and Klinger, J. D. (1986). Pseudomonas cepacia: Biology, Mechanisms of Virulence, Epidemiology. J. Pediatr. 108 (5), 806–812. doi:10.1016/s0022-3476(86)80749-1
Gómez-Bellot, M. J., Lorente, B., Ortuño, M. F., Medina, S., Gil-Izquierdo, Á., Bañón, S., et al. (2021). Recycled Wastewater and Reverse Osmosis Brine Use for Halophytes Irrigation: Differences in Physiological, Nutritional and Hormonal Responses of Crithmum Maritimum and Atriplex Halimus Plants. Agronomy 11 (4), 627. doi:10.3390/agronomy11040627
Grossiord, C., Buckley, T. N., Cernusak, L. A., Novick, K. A., Poulter, B., Siegwolf, R. T. W., et al. (2020). Plant Responses to Rising Vapor Pressure Deficit. New Phytol. 226 (6), 1550–1566. doi:10.1111/nph.16485
Grouneva, I., Gollan, P. J., Kangasjärvi, S., Suorsa, M., Tikkanen, M., and Aro, E.-M. (2013). Phylogenetic Viewpoints on Regulation of Light Harvesting and Electron Transport in Eukaryotic Photosynthetic Organisms. Planta 237 (2), 399–412. doi:10.1007/s00425-012-1744-5
Guarnieri, V., Locantore, I., Marchitelli, G., Boscheri, G., Lobascio, C., and Saverino, A. (2020). Biowyse: a Solution for Real-Time, Automated and Integrated Biocontamination Control. Environ. Eng. Manag. J. 19 (10), 1773–1780. doi:10.30638/eemj.2020.168
Guo, S., Tang, Y., Gao, F., Ai, W., and Qin, L. (2008). Effects of Low Pressure and Hypoxia on Growth and Development of Wheat. Acta Astronautica 63 (7-10), 1081–1085. doi:10.1016/j.actaastro.2008.02.006
Hammer, B. E., Kidder, L. S., Williams, P. C., and Xu, W. W. (2009). Magnetic Levitation of MC3T3 Osteoblast Cells as a Ground-Based Simulation of Microgravity. Microgravity Sci. Technol. 21 (4), 311–318. doi:10.1007/s12217-008-9092-6
Harvey, B., and Zakutnyaya, O. (2011). Russian Space Probes: Scientific Discoveries and Future Missions. Springer science & business media.
Hauslage, J., Cevik, V., and Hemmersbach, R. (2017). Pyrocystis Noctiluca Represents an Excellent Bioassay for Shear Forces Induced in Ground-Based Microgravity Simulators (Clinostat and Random Positioning Machine). npj Microgravity 3 (1), 12–17. doi:10.1038/s41526-017-0016-x
Hawkes, C. V., Hartley, I. P., Ineson, P., and Fitter, A. H. (2008). Soil Temperature Affects Carbon Allocation within Arbuscular Mycorrhizal Networks and Carbon Transport from Plant to Fungus. Glob. Change Biol. 14 (5), 1181–1190. doi:10.1111/j.1365-2486.2007.01535.x
He, C., Davies, F. T., Lacey, R. E., Drew, M. C., and Brown, D. L. (2003). Effect of Hypobaric Conditions on Ethylene Evolution and Growth of Lettuce and Wheat. J. Plant Physiol. 160 (11), 1341–1350. doi:10.1078/0176-1617-01106
He, Y., Yu, C., Zhou, L., Chen, Y., Liu, A., Jin, J., et al. (2014). Rubisco Decrease Is Involved in Chloroplast Protrusion and Rubisco-Containing Body Formation in Soybean (Glycine max.) under Salt Stress. Plant Physiol. Biochem. 74, 118–124. doi:10.1016/j.plaphy.2013.11.008
Heo, J. W., Kang, D. H., Bang, H. S., Hong, S. G., Chun, C., and Kang, K. K. (2012). Early Growth, Pigmentation, Protein Content, and Phenylalanine Ammonia-Lyase Activity of Red Curled Lettuces Grown under Different Lighting Conditions. 원예과학기술지. doi:10.7235/hort.2012.11118
Hernández, J. A., and Almansa, M. S. (2002). Short-term Effects of Salt Stress on Antioxidant Systems and Leaf Water Relations of Pea Leaves. Physiologia Plantarum 115 (2), 251–257. doi:10.1034/j.1399-3054.2002.1150211.x
H. H. Kim, R. M. Wheeler, J. C. Sager, G. Gains, and J. Naikane (Editors) (2005). Evaluation of Lettuce Growth Using Supplemental green Light with Red and Blue Light-Emitting Diodes in a Controlled Environment-A Review of Research at Kennedy Space Center. V International Symposium on Artificial Lighting in Horticulture 711.
Hikosaka, K., Ishikawa, K., Borjigidai, A., Muller, O., and Onoda, Y. (2006). Temperature Acclimation of Photosynthesis: Mechanisms Involved in the Changes in Temperature Dependence of Photosynthetic Rate. J. Exp. Bot. 57 (2), 291–302. doi:10.1093/jxb/erj049
History and timeline of the ISS ISS National Lab: NASA (2019). The ISS National Lab Is Managed by the center for the Advancement of Science in Space, under Agreement with NASA. Available from: https://www.issnationallab.org/about/iss-timeline/.
Hodkinson, P. D., Anderton, R. A., Posselt, B. N., and Fong, K. J. (2017). An Overview of Space Medicine. Br. J. Anaesth. 119 (Suppl. l_1), i143–i153. doi:10.1093/bja/aex336
Holding, D. R., and Streich, A. M. (2013). Plant Growth Processes: Transpiration, Photosynthesis, and Respiration. Lincoln, Nebraska: University of Nebraska Cooperative Extension.
Hubbart, S., Smillie, I. R. A., Heatley, M., Swarup, R., Foo, C. C., Zhao, L., et al. (2018). Enhanced Thylakoid Photoprotection Can Increase Yield and Canopy Radiation Use Efficiency in rice. Commun. Biol. 1 (1), 22–12. doi:10.1038/s42003-018-0026-6
Hwang, H., An, S., Pham, M. D., Cui, M., and Chun, C. (2020). The Combined Conditions of Photoperiod, Light Intensity, and Air Temperature Control the Growth and Development of Tomato and Red Pepper Seedlings in a Closed Transplant Production System. Sustainability 12 (23), 9939. doi:10.3390/su12239939
Im, J., Kim, W. J., Kim, S. H., and Ha, B.-K. (2017). Effects of Proton Beam Irradiation on Seed Germination and Growth of Soybean (Glycine max L. Merr.). J. Korean Phys. Soc. 71 (11), 752–757. doi:10.3938/jkps.71.752
Ionizing Radiation and Human Health (2018). Reviewing Models of Exposure and Mechanisms of Cellular DamageEnvironmental Research and Public Health. An Epigenetic Perspective, 15.
Jackson, S. D. (2009). Plant Responses to Photoperiod. New Phytol. 181 (3), 517–531. doi:10.1111/j.1469-8137.2008.02681.x
Jan, S., Parween, T., Siddiqi, T. O., and Mahmooduzzafar, F. (2012). Effect of Gamma Radiation on Morphological, Biochemical, and Physiological Aspects of Plants and Plant Products. Environ. Rev. 20 (1), 17–39. doi:10.1139/a11-021
Jao, R.-C., and Fang, W. (2004). Effects of Frequency and Duty Ratio on the Growth of Potato Plantlets In Vitro Using Light-Emitting Diodes. HortSci 39 (2), 375–379. doi:10.21273/hortsci.39.2.375
Johkan, M., Shoji, K., Goto, F., Hashida, S.-n., and Yoshihara, T. (2010). Blue Light-Emitting Diode Light Irradiation of Seedlings Improves Seedling Quality and Growth after Transplanting in Red Leaf Lettuce. horts 45 (12), 1809–1814. doi:10.21273/hortsci.45.12.1809
John, S. P., and Hasenstein, K. H. (2011). Effects of Mechanostimulation on Gravitropism and Signal Persistence in Flax Roots. Plant Signaling Behav. 6 (9), 1365–1370. doi:10.4161/psb.6.9.16601
Johnson, C. M., Subramanian, A., Edelmann, R. E., and Kiss, J. Z. (2015). Morphometric Analyses of Petioles of Seedlings Grown in a Spaceflight experiment. J. Plant Res. 128 (6), 1007–1016. doi:10.1007/s10265-015-0749-0
Johnson, C. M., Subramanian, A., Pattathil, S., Correll, M. J., and Kiss, J. Z. (2017). Comparative Transcriptomics Indicate Changes in Cell wall Organization and Stress Response in Seedlings during Spaceflight. Am. J. Bot. 104 (8), 1219–1231. doi:10.3732/ajb.1700079
Jones, E., Qadir, M., van Vliet, M. T. H., Smakhtin, V., and Kang, S.-m. (2019). The State of Desalination and Brine Production: A Global Outlook. Sci. Total Environ. 657, 1343–1356. doi:10.1016/j.scitotenv.2018.12.076
Kangasjärvi, S., Nurmi, M., Tikkanen, M., and Aro, E.-M. (2009). Cell-specific Mechanisms and Systemic Signalling as Emerging Themes in Light Acclimation of C3 Plants. Plant Cel Environ. 32 (9), 1230–1240. doi:10.1111/j.1365-3040.2009.01982.x
Kara, Y. (2013). Morphological and Physiological Effects of UV-C Radiation on Bean Plant (Phaseolus vulgaris). Biosci. Res. 10 (1), 29–32.
Karnasuta, S., Punsuvon, V., Chiemchaisri, C., and Chunkao, K. (2007). Optimization of Biodiesel Production from Trap Grease via Two-step Catalyzed Process. Asian J. Energ. Environ 8, 145–168.
Kast, J., Yu, Y., Seubert, C. N., Wotring, V. E., and Derendorf, H. (2017). Drugs in Space: Pharmacokinetics and Pharmacodynamics in Astronauts. Eur. J. Pharm. Sci. 109, S2–S8. doi:10.1016/j.ejps.2017.05.025
Kiss, J. Z. (2000). Mechanisms of the Early Phases of Plant Gravitropism. Crit. Rev. Plant Sci. 19 (6), 551–573. doi:10.1080/07352680091139295
Kiss, J. Z., Millar, K. D. L., and Edelmann, R. E. (2012). Phototropism of Arabidopsis thaliana in Microgravity and Fractional Gravity on the International Space Station. Planta 236 (2), 635–645. doi:10.1007/s00425-012-1633-y
Kiss, J. Z. (2014). Plant Biology in Reduced Gravity on the Moon and Mars. Plant Biol. J. 16, 12–17. doi:10.1111/plb.12031
Kiss, J. Z., Wolverton, C., Wyatt, S. E., Hasenstein, K. H., and van Loon, J. J. W. A. (2019). Comparison of Microgravity Analogs to Spaceflight in Studies of Plant Growth and Development. Front. Plant Sci. 10, 1577. doi:10.3389/fpls.2019.01577
Knight, S. L., and Mitchell, C. A. (1983). Enhancement of Lettuce Yield by Manipulation of Light and Nitrogen Nutrition. HortScience 108 (5), 750–754.
Knight, S. L., and Mitchell, C. A. (1988). Effects of CO2and Photosynthetic Photon Flux on Yield, Gas Exchange and Growth Rate ofLactuca SativaL. 'Waldmann's Green'. J. Exp. Bot. 39 (3), 317–328. doi:10.1093/jxb/39.3.317
Ko, D., and Helariutta, Y. (2017). Shoot-Root Communication in Flowering Plants. Curr. Biol. 27 (17), R973–R978. doi:10.1016/j.cub.2017.06.054
Kollist, T., Moldau, H., Rasulov, B., Oja, V., Rämma, H., Hüve, K., et al. (2007). A Novel Device Detects a Rapid Ozone-Induced Transient Stomatal Closure in Intact Arabidopsis and its Absence in Abi2 Mutant. Physiol. Plant 129 (4), 796–803. doi:10.1111/j.1399-3054.2006.00851.x
Kono, M., and Terashima, I. (2014). Long-term and Short-Term Responses of the Photosynthetic Electron Transport to Fluctuating Light. J. Photochem. Photobiol. B: Biol. 137, 89–99. doi:10.1016/j.jphotobiol.2014.02.016
Koontz, H. V., and Prince, R. P. (1986). Effect of 16 and 24 hours Daily Radiation (Light) on Lettuce Growth. HortScience 21 (1), 123–124.
Kotilainen, T., Robson, T. M., and Hernández, R. (2018). Light Quality Characterization under Climate Screens and Shade Nets for Controlled-Environment Agriculture. PLoS One 13, e0199628. doi:10.1371/journal.pone.0199628
Kovács, E., and Keresztes, Á. (2002). Effect of Gamma and UV-B/C Radiation on Plant Cells. Micron 33 (2), 199–210. doi:10.1016/s0968-4328(01)00012-9
Kromdijk, J., Głowacka, K., Leonelli, L., Gabilly, S. T., Iwai, M., Niyogi, K. K., et al. (2016). Improving Photosynthesis and Crop Productivity by Accelerating Recovery from Photoprotection. Science 354 (6314), 857–861. doi:10.1126/science.aai8878
Kuznetsov, O. A., and Hasenstein, K. H. (1996). Intracellular Magnetophoresis of Amyloplasts and Induction of Root Curvature. Planta 198 (1), 87–94. doi:10.1007/BF00197590
Kuznetsov, O. A., and Hasenstein, K. H. (2001). Intracellular Magnetophoresis of Statoliths in Chara Rhizoids and Analysis of Cytoplasm Viscoelasticity. Adv. Space Res. 27 (5), 887–892. doi:10.1016/s0273-1177(01)00182-x
Kuznetsov, O. A., and Hasenstein, K. H. (1997). Magnetophoretic Induction of Curvature in Coleoptiles and Hypocotyls. J. Exp. Bot. 48 (11), 1951–1957. doi:10.1093/jxb/48.11.1951
L. Okushima, N. Fukuda, N. Kanesaka, K. Sekiguchi, N. Odawara, S. Saseet al. (2012). Effect of Overnight Supplemental Lighting with Different Spectral LEDs on the Growth of Some Leafy Vegetables. VII International Symposium on Light in Horticultural Systems 956.
Lakhiar, I. A., Gao, J., Syed, T. N., Chandio, F. A., and Buttar, N. A. (2018). Modern Plant Cultivation Technologies in Agriculture under Controlled Environment: A Review on Aeroponics. J. Plant Interactions 13 (1), 338–352. doi:10.1080/17429145.2018.1472308
Larcher, W. (2003). Physiological Plant Ecology: Ecophysiology and Stress Physiology of Functional Groups. Springer Sci. Business Media.
Lauro, S. E., Pettinelli, E., Caprarelli, G., Guallini, L., Rossi, A. P., Mattei, E., et al. (2021). Multiple Subglacial Water Bodies below the South Pole of Mars Unveiled by New MARSIS Data. Nat. Astron. 5 (1), 63–70. doi:10.1038/s41550-020-1200-6
Lefsrud, M. G., Kopsell, D. A., Augé, R. M., and Both, A. J. (2006). Biomass Production and Pigment Accumulation in Kale Grown under Increasing Photoperiods. HortSci 41 (3), 603–606. doi:10.21273/hortsci.41.3.603
Lillo, C., and Appenroth, K.-J. (2001). Light Regulation of Nitrate Reductase in Higher Plants: Which Photoreceptors Are Involved? Plant Biol. 3 (5), 455–465. doi:10.1055/s-2001-17732
Lin, K.-H., Huang, M.-Y., Huang, W.-D., Hsu, M.-H., Yang, Z.-W., and Yang, C.-M. (2013). The Effects of Red, Blue, and white Light-Emitting Diodes on the Growth, Development, and Edible Quality of Hydroponically Grown Lettuce (Lactuca sativa L. Var. Capitata). Scientia Horticulturae 150, 86–91. doi:10.1016/j.scienta.2012.10.002
Lizana, X. C., Hess, S., and Calderini, D. F. (2009). Crop Phenology Modifies Wheat Responses to Increased UV-B Radiation. Agric. For. Meteorology 149 (11), 1964–1974. doi:10.1016/j.agrformet.2009.07.003
Llanes, A., Masciarelli, O., and Luna, V. (2014). Growth Responses to Sulfate and Chloride Are Related to Different Phytohormone Profiles in the Halophyte Prosopis Strombulifera. Emir. J. Food Agric. 26, 1097–1113. doi:10.9755/ejfa.v26i12.19121
Long, S. P. (1983). C4 Photosynthesis at Low Temperatures. Plant Cel Environ 6 (4), 345–363. doi:10.1111/1365-3040.ep11612141
M. Bridgen (Editor) (2016). “Using Ultraviolet-C (UV-C) Irradiation on Greenhouse Ornamental Plants for Growth Regulation,”.VIII International Symposium on Light in Horticulture 1134
M. Kanechi, A. Maekawa, Y. Nishida, and E. Miyashita (Editors) (2016). Effects of Pulsed Lighting Based Light-Emitting Diodes on the Growth and Photosynthesis of Lettuce Leaves. VIII International Symposium on Light in Horticulture 1134.
Maiwald, V., Schubert, D., Quantius, D., and Zabel, P. (2021). From Space Back to Earth: Supporting Sustainable Development with Spaceflight Technologies. Sustainable Earth 4 (1), 1–16. doi:10.1186/s42055-021-00042-9
Maksimov, E. G., Mironov, K. S., Trofimova, M. S., Nechaeva, N. L., Todorenko, D. A., Klementiev, K. E., et al. (2017). Membrane Fluidity Controls Redox-Regulated Cold Stress Responses in Cyanobacteria. Photosynth Res. 133 (1), 215–223. doi:10.1007/s11120-017-0337-3
Marcu, D., Damian, G., Cosma, C., and Cristea, V. (2013). Gamma Radiation Effects on Seed Germination, Growth and Pigment Content, and ESR Study of Induced Free Radicals in maize (Zea mays). J. Biol. Phys. 39 (4), 625–634. doi:10.1007/s10867-013-9322-z
Martelaro, N. (2017). Powering the Stanford Torus America. Stanford University. Available from: http://large.stanford.edu/courses/2016/ph240/martelaro2/.
Matía, I., González-Camacho, F., Herranz, R., Kiss, J. Z., Gasset, G., van Loon, J. J. W. A., et al. (2010). Plant Cell Proliferation and Growth Are Altered by Microgravity Conditions in Spaceflight. J. Plant Physiol. 167 (3), 184–193. doi:10.1016/j.jplph.2009.08.012
May, S. (2017). What Is Microgravity?. Washington: NASA. Available from: https://www.nasa.gov/audience/forstudents/5-8/features/nasa-knows/what-is-microgravity-58.html.
McAusland, L., Lim, M.-T., Morris, D. E., Smith-Herman, H. L., Mohammed, U., Hayes-Gill, B. R., et al. (2020). Growth Spectrum Complexity Dictates Aromatic Intensity in Coriander (Coriandrum Sativum L.). Front. Plant Sci. 11, 462. doi:10.3389/fpls.2020.00462
McLaughlin, S. B., Wullschleger, S. D., Sun, G., and Nosal, M. (2007). Interactive Effects of Ozone and Climate on Water Use, Soil Moisture Content and Streamflow in a Southern Appalachian forest in the USA. New Phytol. 174 (1), 125–136. doi:10.1111/j.1469-8137.2007.01970.x
Meggs, L., Dunbar, B., and Boen, B. (2010). Growing Plants and Vegetables in a Space Garden. Natl. Aeronautics Space Adm., 10–074.
Millar, K. D. L., Kumar, P., Correll, M. J., Mullen, J. L., Hangarter, R. P., Edelmann, R. E., et al. (2010). A Novel Phototropic Response to Red Light Is Revealed in Microgravity. New Phytol. 186 (3), 648–656. doi:10.1111/j.1469-8137.2010.03211.x
Mittler, R. (2006). Abiotic Stress, the Field Environment and Stress Combination. Trends Plant Sci. 11 (1), 15–19. doi:10.1016/j.tplants.2005.11.002
Molas, M. L., and Kiss, J. Z. (2009). Chapter 1 Phototropism and Gravitropism in Plants. Adv. Bot. Res. 49, 1–34. doi:10.1016/s0065-2296(08)00601-0
Montmessin, F., and Lefèvre, F. (2013). Transport-driven Formation of a Polar Ozone Layer on Mars. Nat. Geosci 6 (11), 930–933. doi:10.1038/ngeo1957
Morgan, P. B., Ainsworth, E. A., and Long, S. P. (2003). How Does Elevated Ozone Impact Soybean? A Meta-Analysis of Photosynthesis, Growth and Yield. Cel Environ. 26 (8), 1317–1328. doi:10.1046/j.0016-8025.2003.01056.x
Morita, M. T. (2010). Directional Gravity Sensing in Gravitropism. Annu. Rev. Plant Biol. 61, 705–720. doi:10.1146/annurev.arplant.043008.092042
Mortensen, L., and Strømme, E. (1987). Effects of Light Quality on Some Greenhouse Crops. Scientia Horticulturae 33 (1-2), 27–36. doi:10.1016/0304-4238(87)90029-x
Mosa, K. A., Ismail, A., and Helmy, M. (2017). Introduction to Plant Stresses. Plant Stress Tolerance: Springer, 1–19. doi:10.1007/978-3-319-59379-1_1
Mousseau, T. A., and Møller, A. P. (2020). Plants in the Light of Ionizing Radiation: What Have We Learned from Chernobyl, Fukushima, and Other “Hot” Places? Front. Plant Sci. 11, 552. doi:10.3389/fpls.2020.00552
Munns, R., and Gilliham, M. (2015). Salinity Tolerance of Crops - what Is the Cost? New Phytol. 208 (3), 668–673. doi:10.1111/nph.13519
Munns, R., and Termaat, A. (1986). Whole-plant Responses to Salinity. Funct. Plant Biol. 13 (1), 143–160. doi:10.1071/pp9860143
Murakami, S., and Yamada, M. (1988). Architecture of Statocytes and Chloroplasts under the Microgravity Environment. Biol. Sci. Space 2 (4), 301. doi:10.2187/bss.2.228
Murchie, E. H., and Niyogi, K. K. (2011). Manipulation of Photoprotection to Improve Plant Photosynthesis. Plant Physiol. 155 (1), 86–92. doi:10.1104/pp.110.168831
Murchie, E. H., and Ruban, A. V. (2020). Dynamic Non‐photochemical Quenching in Plants: from Molecular Mechanism to Productivity. Plant J. 101 (4), 885–896. doi:10.1111/tpj.14601
Mustroph, A., Lee, S. C., Oosumi, T., Zanetti, M. E., Yang, H., Ma, K., et al. (2010). Cross-kingdom Comparison of Transcriptomic Adjustments to Low-Oxygen Stress Highlights Conserved and Plant-specific Responses. Plant Physiol. 152 (3), 1484–1500. doi:10.1104/pp.109.151845
N. Fukuda, N. Kanesaka, L. Okushima, and S. Sase (Editors) (2019). Effects of Over-night Lighting by Red Light Emitting Diodes on the Growth and Photosynthesis of Lettuce under CO2 Enrichment. International Symposium on Advanced Technologies and Management for Innovative Greenhouses: GreenSys2019 1296.
Nazari-Sharabian, M., Aghababaei, M., Karakouzian, M., and Karami, M. (2020). Water on Mars-A Literature Review. Galaxies 8 (2), 40. doi:10.3390/galaxies8020040
Nelson, G. A. (2016). Space Radiation and Human Exposures, a Primer. Radiat. Res. 185 (4), 349–358. doi:10.1667/rr14311.1
Nievola, C. C., Carvalho, C. P., Carvalho, V., and Rodrigues, E. (2017). Rapid Responses of Plants to Temperature Changes. Temperature 4 (4), 371–405. doi:10.1080/23328940.2017.1377812
O’Callaghan, J. (2020). The Food and Water Systems Astronauts Will Need to Travel to Places like Mars. The EU Res. Innovation.
Ohashi-Kaneko, K., Takase, M., Kon, N., Fujiwara, K., and Kurata, K. (2007). Effect of Light Quality on Growth and Vegetable Quality in Leaf Lettuce, Spinach and Komatsuna. Environ. Control. Biol. 45 (3), 189–198. doi:10.2525/ecb.45.189
Olvera-González, E., Alaniz-Lumbreras, D., Ivanov-Tsonchev, R., Villa-Hernández, J., de la Rosa-Vargas, I., López-Cruz, I., et al. (2013). Chlorophyll Fluorescence Emission of Tomato Plants as a Response to Pulsed Light Based LEDs. Plant Growth Regul. 69 (2), 117–123. doi:10.1007/s10725-012-9753-8
Osborne, C. P., Wythe, E. J., Ibrahim, D. G., Gilbert, M. E., and Ripley, B. S. (2008). Low Temperature Effects on Leaf Physiology and Survivorship in the C3 and C4 Subspecies of Alloteropsis Semialata. J. Exp. Bot. 59 (7), 1743–1754. doi:10.1093/jxb/ern062
Ouzounis, T., Rosenqvist, E., and Ottosen, C.-O. (2015). Spectral Effects of Artificial Light on Plant Physiology and Secondary Metabolism: a Review. horts 50 (8), 1128–1135. doi:10.21273/hortsci.50.8.1128
Parida, A. K., and Das, A. B. (2005). Salt Tolerance and Salinity Effects on Plants: a Review. Ecotoxicology Environ. Saf. 60 (3), 324–349. doi:10.1016/j.ecoenv.2004.06.010
Parry, M. A., Keys, A. J., Madgwick, P. J., Carmo-Silva, A. E., and Andralojc, P. J. (2008). Rubisco Regulation: a Role for Inhibitors. J. Exp. Bot. 59 (7), 1569–1580. doi:10.1093/jxb/ern084
Paul, A-L., and Ferl, R. J. (2007). The Biology of Low Atmospheric Pressure–Implications for Exploration mission Design and Advanced Life Support. Gravitational and Space Research, 19.
Paul, A.-L., Schuerger, A. C., Popp, M. P., Richards, J. T., Manak, M. S., and Ferl, R. J. (2004). Hypobaric Biology: Arabidopsis Gene Expression at Low Atmospheric Pressure. Plant Physiol. 134 (1), 215–223. doi:10.1104/pp.103.032607
Perez, J. (2019). Why Space Radiation Matters USA: NASA. Available from: https://www.nasa.gov/analogs/nsrl/why-space-radiation-matters.
Piqueux, S., Buz, J., Edwards, C. S., Bandfield, J. L., Kleinböhl, A., Kass, D. M., et al. (2019). Widespread Shallow Water Ice on Mars at High Latitudesand Midlatitudes. Geophys. Res. Lett. 46 (24), 14290–14298. doi:10.1029/2019gl083947
Pisek, A., Larcher, W., Vegis, A., and Napp-Zinn, K. (1973). The normal Temperature Range. Temperature and life: Springer, 102–194. doi:10.1007/978-3-642-65708-5_4
Plotnikova, J. M., Rahme, L. G., and Ausubel, F. M. (2000). Pathogenesis of the Human Opportunistic PathogenPseudomonas Aeruginosa PA14 in Arabidopsis. Plant Physiol. 124 (4), 1766–1774. doi:10.1104/pp.124.4.1766
Prithiviraj, B., Bais, H. P., Jha, A. K., and Vivanco, J. M. (2005). Staphylococcus aureus Pathogenicity on Arabidopsis thaliana Is Mediated Either by a Direct Effect of Salicylic Acid on the Pathogen or by SA-dependent, NPR1-independent Host Responses. Plant J. 42 (3), 417–432. doi:10.1111/j.1365-313x.2005.02385.x
Ptushenko, V. V., Avercheva, O. V., Bassarskaya, E. M., Berkovich, Y. A., Erokhin, A. N., Smolyanina, S. O., et al. (2015). Possible Reasons of a Decline in Growth of Chinese Cabbage under a Combined Narrowband Red and Blue Light in Comparison with Illumination by High-Pressure Sodium Lamp. Scientia Horticulturae 194, 267–277. doi:10.1016/j.scienta.2015.08.021
Radiation sources and doses USA (2019). The United States Environmental Protection Agency. Available from: https://www.epa.gov/radiation/radiation-sources-and-doses.
Ramonell, K. M., Kuang, A., Porterfield, D. M., Crispi, M. L., Xiao, Y., McClure, G., et al. (2001). Influence of Atmospheric Oxygen on Leaf Structure and Starch Deposition in Arabidopsis thaliana. Plant Cel Environ. 24 (4), 419–428. doi:10.1046/j.1365-3040.2001.00691.x
Reitz, G. (2008). Characteristic of the Radiation Field in Low Earth Orbit and in Deep Space. Z. für Medizinische Physik 18 (4), 233–243. doi:10.1016/j.zemedi.2008.06.015
Richards, J. T., Corey, K. A., Paul, A.-L., Ferl, R. J., Wheeler, R. M., and Schuerger, A. C. (2006). Exposure ofArabidopsis Thalianato Hypobaric Environments: Implications for Low-Pressure Bioregenerative Life Support Systems for Human Exploration Missions and Terraforming on Mars. Astrobiology 6 (6), 851–866. doi:10.1089/ast.2006.6.851
Ripley, B. S., Gilbert, M. E., Ibrahim, D. G., and Osborne, C. P. (2007). Drought Constraints on C4 Photosynthesis: Stomatal and Metabolic Limitations in C3 and C4 Subspecies of Alloteropsis Semialata. J. Exp. Bot. 58 (6), 1351–1363. doi:10.1093/jxb/erl302
Robson, T. M., Klem, K., Urban, O., and Jansen, M. A. K. (2015). Re-interpreting Plant Morphological Responses to UV-B Radiation. Plant Cel Environ 38 (5), 856–866. doi:10.1111/pce.12374
Romero-Trigueros, C., Cabañero, J. J., Tortosa, P. A., Gambín, J. M., Maestre-Valero, J. F., and Nicolás, E. N. (2020). Medium-long Term Effects of saline Reclaimed Water and Regulated Deficit Irrigation on Fruit Quality of Citrus. J. Sci. Food Agric. 100 (3), 1350–1357. doi:10.1002/jsfa.10091
Rossi-Wilcox, S. M. (2007). Molecular Gastronomy: Exploring the Science of Flavor. Wiley Online Libr. doi:10.1111/j.1540-5931.2007.00472.x
Ruberto, G., Baratta, M. T., Deans, S. G., and Dorman, H. J. (2000). Antioxidant and Antimicrobial Activity of Foeniculum Vulgare and Crithmum Maritimum Essential Oils. Planta Med. 66 (08), 687–693. doi:10.1055/s-2000-9773
Ryba-White, M., Nedukha, O., Hilaire, E., Guikema, J. A., Kordyum, E., and Leach, J. E. (2001). Growth in Microgravity Increases Susceptibility of Soybean to a Fungal Pathogen. Plant Cel Physiol. 42 (6), 657–664. doi:10.1093/pcp/pce082
S. Vernov, I. Savenko, M. Teltsov, and P. Shavrin (Editors) (1965). “International Cosmic Ray Conference,” Investigation of the Outer Proton belt.
Sage, R. F. (2002). C4 Photosynthesis in Terrestrial Plants Does Not Require Kranz Anatomy. Trends Plant Sci. 7 (7), 283–285. doi:10.1016/s1360-1385(02)02293-8
Schuerger, A. C. (2021). Integrated Pest Management (IPM) Protocols for Space-Based Bioregenerative Life Support Systems (BLSS). Front. Astron. Space Sci. 8, 195. doi:10.3389/fspas.2021.759641
Schuerger, A. C., Amaradasa, B. S., Dufault, N. S., Hummerick, M. E., Richards, J. T., Khodadad, C. L., et al. (2021). Fusarium Oxysporum as an Opportunistic Fungal Pathogen on Zinnia Hybrida Plants Grown on Board the International Space Station. Astrobiology 21 (9), 1029–1048. doi:10.1089/ast.2020.2399
Shen, Y., Guo, S., Zhao, P., Wang, L., Wang, X., Li, J., et al. (2018). Research on Lettuce Growth Technology Onboard Chinese Tiangong II Spacelab. Acta Astronautica 144, 97–102. doi:10.1016/j.actaastro.2017.11.007
Sivakumar, P., Sharmila, P., and Pardha Saradhi, P. (2000). Proline Alleviates Salt-Stress-Induced Enhancement in Ribulose-1,5-Bisphosphate Oxygenase Activity. Biochem. Biophysical Res. Commun. 279 (2), 512–515. doi:10.1006/bbrc.2000.4005
Smith, H. L., McAusland, L., and Murchie, E. H. (2017). Don't Ignore the green Light: Exploring Diverse Roles in Plant Processes. J. Exp. Bot. 68 (9), 2099–2110. doi:10.1093/jxb/erx098
Solar System Temperatures (2018). NASA. Available from: https://solarsystem.nasa.gov/resources/681/solar-system-temperatures/.
Son, K.-H., Jeon, Y.-M., and Oh, M.-M. (2016). Application of Supplementary white and Pulsed Light-Emitting Diodes to Lettuce Grown in a Plant Factory with Artificial Lighting. Hortic. Environ. Biotechnol. 57 (6), 560–572. doi:10.1007/s13580-016-0068-y
Son, K.-H., and Oh, M.-M. (2013). Leaf Shape, Growth, and Antioxidant Phenolic Compounds of Two Lettuce Cultivars Grown under Various Combinations of Blue and Red Light-Emitting Diodes. horts 48 (8), 988–995. doi:10.21273/hortsci.48.8.988
Song, W., Forderer, A., Yu, D., and Chai, J. (2021). Structural Biology of Plant Defence. New Phytol. 229 (2), 692–711. doi:10.1111/nph.16906
Spinoff, N. (2006). Progressive Plant Growing Has Business Blooming. Environ. Agric. Resour. New York, 64–77. NASA Spinoff.
Stagnari, F., Galieni, A., and Pisante, M. (2016). Drought Stress Effects on Crop Quality. Water Stress Crop plants: a Sustain. approach 2, 375–392. doi:10.1002/9781119054450.ch23
Stankovic, B. (2018). A Journey of How Humans Adapt and Live in Microgravity. Plants Space Into Space, 153–170.
Starr, M. P., and Chatterjee, A. K. (1972). The Genus Erwinia: Enterobacteria Pathogenic to Plants and Animals. Annu. Rev. Microbiol. 26 (1), 389–426. doi:10.1146/annurev.mi.26.100172.002133
Stutte, G. W., Edney, S., and Skerritt, T. (2009). Photoregulation of Bioprotectant Content of Red Leaf Lettuce with Light-Emitting Diodes. horts 44 (1), 79–82. doi:10.21273/hortsci.44.1.79
Sudhir, P., and Murthy, S. D. S. (2004). Effects of Salt Stress on Basic Processes of Photosynthesis. Photosynt. 42 (4), 481–486. doi:10.1007/s11099-005-0001-6
Takahashi, F., and Shinozaki, K. (2019). Long-distance Signaling in Plant Stress Response. Curr. Opin. Plant Biol. 47, 106–111. doi:10.1016/j.pbi.2018.10.006
Tamulaitis, G., Duchovskis, P., Bliznikas, Z., Breive, K., Ulinskaite, R., Brazaityte, A., et al. (2005). High-power Light-Emitting Diode Based Facility for Plant Cultivation. J. Phys. D: Appl. Phys. 38 (17), 3182–3187. doi:10.1088/0022-3727/38/17/s20
Tang, X., Mu, X., Shao, H., Wang, H., and Brestic, M. (2015). Global Plant-Responding Mechanisms to Salt Stress: Physiological and Molecular Levels and Implications in Biotechnology. Crit. Rev. Biotechnol. 35 (4), 425–437. doi:10.3109/07388551.2014.889080
Tardieu, F., Simonneau, T., and Muller, B. (2018). The Physiological Basis of Drought Tolerance in Crop Plants: a Scenario-dependent Probabilistic Approach. Annu. Rev. Plant Biol. 69, 733–759. doi:10.1146/annurev-arplant-042817-040218
Tennessen, D. J., Bula, R. J., and Sharkey, T. D. (1995). Efficiency of Photosynthesis in Continuous and Pulsed Light Emitting Diode Irradiation. Photosynth Res. 44 (3), 261–269. doi:10.1007/bf00048599
Tevini, M., Iwanzik, W., and Thoma, U. (1981). Some Effects of Enhanced UV-B Irradiation on the Growth and Composition of Plants. Planta 153 (4), 388–394. doi:10.1007/bf00384258
This, H. (2007). Kitchen Mysteries: Revealing the Science of Cooking. Columbia University Press, 220.
Tikhonov, A. N. (2014). The Cytochrome B6f Complex at the Crossroad of Photosynthetic Electron Transport Pathways. Plant Physiol. Biochem. 81, 163–183. doi:10.1016/j.plaphy.2013.12.011
Tikkanen, M., and Aro, E.-M. (2014). Integrative Regulatory Network of Plant Thylakoid Energy Transduction. Trends Plant Sci. 19 (1), 10–17. doi:10.1016/j.tplants.2013.09.003
Tikkanen, M., Grieco, M., and Aro, E.-M. (2011). Novel Insights into Plant Light-Harvesting Complex II Phosphorylation and 'state Transitions'. Trends Plant Sci. 16 (3), 126–131. doi:10.1016/j.tplants.2010.11.006
Townsend, A. J., Retkute, R., Chinnathambi, K., Randall, J. W. P., Foulkes, J., Carmo-Silva, E., et al. (2018). Suboptimal Acclimation of Photosynthesis to Light in Wheat Canopies. Plant Physiol. 176 (2), 1233–1246. doi:10.1104/pp.17.01213
Vahisalu, T., Puzõrjova, I., Brosché, M., Valk, E., Lepiku, M., Moldau, H., et al. (2010). Ozone-triggered Rapid Stomatal Response Involves the Production of Reactive Oxygen Species, and Is Controlled by SLAC1 and OST1. Plant J. 62 (3), 442–453. doi:10.1111/j.1365-313x.2010.04159.x
Vandenbrink, J. P., Herranz, R., Medina, F. J., Edelmann, R. E., and Kiss, J. Z. (2016). A Novel Blue-Light Phototropic Response Is Revealed in Roots of Arabidopsis thaliana in Microgravity. Planta 244 (6), 1201–1215. doi:10.1007/s00425-016-2581-8
Vandenbrink, J. P., Kiss, J. Z., Herranz, R., and Medina, F. J. (2014). Light and Gravity Signals Synergize in Modulating Plant Development. Front. Plant Sci. 5, 563. doi:10.3389/fpls.2014.00563
Vandenbrink, J. P., and Kiss, J. Z. (2016). Space, the Final Frontier: A Critical Review of Recent Experiments Performed in Microgravity. Plant Sci. 243, 115–119. doi:10.1016/j.plantsci.2015.11.004
Vanhaelewyn, L., Van Der Straeten, D., De Coninck, B., and Vandenbussche, F. (2020). Ultraviolet Radiation from a Plant Perspective: The Plant-Microorganism Context. Front. Plant Sci. 11, 1984. doi:10.3389/fpls.2020.597642
Walker, T. S., Bais, H. P., Dèziel, E., Schweizer, H. P., Rahme, L. G., Fall, R., et al. (2004). Pseudomonas Aeruginosa-Plant Root Interactions. Pathogenicity, Biofilm Formation, and Root Exudation. Plant Physiol. 134 (1), 320–331. doi:10.1104/pp.103.027888
Walters, R. G. (2005). Towards an Understanding of Photosynthetic Acclimation. J. Exp. Bot. 56 (411), 435–447. doi:10.1093/jxb/eri060
Wamelink, G. W. W., Frissel, J. Y., Krijnen, W. H. J., and Verwoert, M. R. (2021). Crop Growth and Viability of Seeds on Mars and Moon Soil Simulants. Terraforming Mars, 313–329. doi:10.1002/9781119761990.ch13
Wang, H., Gu, M., Cui, J., Shi, K., Zhou, Y., and Yu, J. (2009). Effects of Light Quality on CO2 Assimilation, Chlorophyll-Fluorescence Quenching, Expression of Calvin Cycle Genes and Carbohydrate Accumulation in Cucumis Sativus. J. Photochem. Photobiol. B: Biol. 96 (1), 30–37. doi:10.1016/j.jphotobiol.2009.03.010
Weaver, G. M., van Iersel, M. W., and Mohammadpour Velni, J. (2019). A Photochemistry-Based Method for Optimising Greenhouse Supplemental Light Intensity. Biosyst. Eng. 182, 123–137. doi:10.1016/j.biosystemseng.2019.03.008
Weyers, J. D. B., and Paterson, N. W. (2001). Plant Hormones and the Control of Physiological Processes. New Phytol. 152 (3), 375–407. doi:10.1046/j.0028-646x.2001.00281.x
Wheeler, R. M. (2010). Plants for Human Life Support in Space: From Myers to Mars. Gravitational and Space Research, 23.
Wheeler, R. M., Wehkamp, C. A., Stasiak, M. A., Dixon, M. A., and Rygalov, V. Y. (2011). Plants Survive Rapid Decompression: Implications for Bioregenerative Life Support. Adv. Space Res. 47 (9), 1600–1607. doi:10.1016/j.asr.2010.12.017
Wi, S. G., Chung, B. Y., Kim, J.-S., Kim, J.-H., Baek, M.-H., Lee, J.-W., et al. (2007). Effects of Gamma Irradiation on Morphological Changes and Biological Responses in Plants. Micron 38 (6), 553–564. doi:10.1016/j.micron.2006.11.002
Wu, M., Hou, C., Jiang, C., Wang, Y., Wang, C., Chen, H., et al. (2007). A Novel Approach of LED Light Radiation Improves the Antioxidant Activity of Pea Seedlings. Food Chem. 101 (4), 1753–1758. doi:10.1016/j.foodchem.2006.02.010
Yakovtseva, M., Govorova, G., and Tarakanov, I. (2015). Photomorphogenetic Control of Growth, Development and Crop Production in Strawberry (Fragaria X Ananassa L,) Grown with Artificial Lighting. Izvestiya Timiryazev Agric. Acad. 3, 25–35.
Yao, X. C., Tu, H. Q., Wang, X. L., and Wang, J. (2021). The Effect of Supplemental LED Night Lighting on the Growth and Physiology of the Para Rubber Tree. J. Rubber Res., 1–6. doi:10.1007/s42464-021-00095-7
Zabel, P., Bamsey, M., Schubert, D., and Tajmar, M. (2016). Review and Analysis of over 40 Years of Space Plant Growth Systems. Life Sci. Space Res. 10, 1–16. doi:10.1016/j.lssr.2016.06.004
Zhang, G. (2016). Improving Productivity and Quality of Low-Potassium Lettuce in a Plant Factory with Artificial Lighting. Chiba: 千葉大学= Chiba University.
Zheng, H. Q., Han, F., and Le, J. (2015). Higher Plants in Space: Microgravity Perception, Response, and Adaptation. Microgravity Sci. Technol. 27 (6), 377–386. doi:10.1007/s12217-015-9428-y
Keywords: plant, stressors, space, farming, Earth, ecosystem
Citation: Hessel V, Liang S, Tran NN, Escribà-Gelonch M, Zeckovic O, Knowling M, Rebrov E, This H, Westra S, Fisk I, Gilliham M and Burgess A (2022) Eustress in Space: Opportunities for Plant Stressors Beyond the Earth Ecosystem. Front. Astron. Space Sci. 9:841211. doi: 10.3389/fspas.2022.841211
Received: 22 December 2021; Accepted: 15 February 2022;
Published: 08 March 2022.
Edited by:
Olga V. Khabarova, Institute of Terrestrial Magnetism Ionosphere and Radio Wave Propagation (RAS), RussiaReviewed by:
Fabrizio D'Aprile, Monash University, AustraliaCopyright © 2022 Hessel, Liang, Tran, Escribà-Gelonch, Zeckovic, Knowling, Rebrov, This, Westra, Fisk, Gilliham and Burgess. This is an open-access article distributed under the terms of the Creative Commons Attribution License (CC BY). The use, distribution or reproduction in other forums is permitted, provided the original author(s) and the copyright owner(s) are credited and that the original publication in this journal is cited, in accordance with accepted academic practice. No use, distribution or reproduction is permitted which does not comply with these terms.
*Correspondence: Volker Hessel, dm9sa2VyLmhlc3NlbEBhZGVsYWlkZS5lZHUuYXU=; Alexandra Burgess, YWxleGFuZHJhLmJ1cmdlc3NAbm90dGluZ2hhbS5hYy51aw==
Disclaimer: All claims expressed in this article are solely those of the authors and do not necessarily represent those of their affiliated organizations, or those of the publisher, the editors and the reviewers. Any product that may be evaluated in this article or claim that may be made by its manufacturer is not guaranteed or endorsed by the publisher.
Research integrity at Frontiers
Learn more about the work of our research integrity team to safeguard the quality of each article we publish.