- 1School of Biological Sciences, Faculty of Biology, Medicine and Health, The University of Manchester, Manchester, United Kingdom
- 2Retired Reader in Genetics, University of Manchester, Manchester, United Kingdom
- 3Ex-Kan Tong Po Professorial Fellow at the Chinese University of Hong Kong, Shatin, Hong Kong SAR, China
- 4Independent Researcher, Manchester, United Kingdom
- 5Independent Researcher, Kaysersberg, France
- 6Kääpä Biotech Oy, Lohja, Finland
We are all familiar with the episodes in the deep time history of Earth that enabled life to emerge in such abundance. Episodes like the formation of a Moon large enough and near enough to cause tides in the Earth’s waters and rocks, a core of sufficient iron with sufficient angular momentum to generate a protective magnetosphere around Earth, and assumption of a planetary axis angle that generates the ecological variation of our seasonal cycles. The living things that did arise on this planet have been modifying their habitats on Earth since they first appeared. Modifications that include the greening of Earth by photosynthetic organisms, which turned a predominantly reducing atmosphere into an oxidising one, the consequent precipitation of iron oxides into iron ore strata, and the formation of huge deposits of limestone by calcifying organisms. The episodes on which we wish to concentrate are 1) the frequent involvement of marine calcifiers (coccolithophores, foraminifera, molluscs, crustacea, corals, echinoderms), that have been described as ecosystem engineers modifying habitats in a generally positive way for other organisms, and 2) the frequent involvement of humans in changing the Earth’s biosphere in a generally negative way for other organisms. The fossil record shows that ancestral marine calcifiers had the physiology to cope with both acidified oceans and great excesses of atmospheric CO2 periodically throughout the past 500 million years, creating vast remains of shells as limestone strata in the process. So, our core belief is that humankind must look to the oceans for a solution to present-day climate change. The marine calcifiers of this planet have a track record of decisively modifying both oceans and atmospheres but take millions of years to do it. On the other hand, humanity works fast; in just a few thousand years we have driven scores of animals and plants to extinction, and in just a few hundred years we have so drastically modified our atmosphere that, arguably, we stand on the verge of extinction ourselves. Of all Earth’s ecosystems, those built around biological calcifiers, which all convert organic carbon into inorganic limestone, are the only ones that offer the prospect of permanent net removal of CO2 from our atmosphere. These are the carbon-removal biotechnologies we should be seeking to exploit.
Introduction: Emulating Earth’s prebiotic history with engineering solutions for atmospheric carbon decrease
The first 4 billion years of our planet’s history is informally called the Precambrian; it extends from the formation of Earth about to the start of the Cambrian Period, about 550 million years ago. The first definitive fossils of hard-shelled animals are abundant in the Cambrian. Life we know about probably arose on Earth after the Moon-forming impact because this event made Earth absolutely sterile for a couple of million years, with Earth covered in a deep ocean of magma and enveloped in an atmosphere of rock vapor. When the mantle solidified, steam in the atmosphere condensed and rained out, taking with it the abundant gaseous NaCl of that atmosphere into hot (about 250°C) salty oceans. At the time the atmospheric pressure was about 100 times that of our present atmosphere, and was composed mostly of H2O, CO2, and N2 (Zahnle et al., 2010). While the greenhouse gas CO2 remained a dominant feature of Earth’s atmosphere the impossibly hot conditions on the surface would have persisted.
Zahnle et al. (2010) describe the thick primordial CO2 atmosphere, liquid water ocean, and freshly formed basalt mantle crust as “…a highly reactive trinity…” It is thought that the CO2 reacted with the newly formed rocks of the seafloor forming carbonates that were subducted into the mantle over a period of 20–100 million years. Removal of CO2 from the atmosphere allowed the Earth’s surface to cool so much that ice covered the ocean and “Snowball Earth” resulted.
This was Earth’s first experience of the runaway greenhouse even though the Sun at this distant time radiated much less than it does today. It is also an indicator for a way of dealing with our present-day CO2 excess, and what might be called the industrial engineering approaches to carbon capture and storage (Petros et al., 2021; and see next section) all seek to react captured atmospheric CO2 with deep mantle rocks. In the present day a carbon sequestration solution that is fast-gaining attention amongst wealthier nations is the application of CO2 capture processes to flue gases of power facilities, and other heavy industries like cement and steel producers.
Most of these “aggressive emission decrease” processes focus on integrating new technologies to capture CO2 from power plant flue gasses; these being responsible for about 80% of global CO2 emissions (Romano et al., 2013). “Hydrate-based processing” methods, based on exposing flue gas to aqueous solutions under controlled conditions, is a promising and high efficiency technology for CO2 capture, though the high cost of maintaining suitable conditions for hydrate formation is preventing wide industrial application of the technology (Li et al., 2019). Unfortunately, aside from their expense, there are other aspects of these engineering “solutions” relating to their governance and real-world impact that argue against their general use for atmospheric carbon decrease. For one thing, these methods can only decrease further emissions, they do not decrease present CO2 accumulations.
The current global industrial trend towards adoption of carbon capture and storage (CCS) technologies focuses on key CSS technologies, such as flue gas CCS injection facilities in fossil fuel and other heavy industry facilities (where the “others” include steel, concrete and fertilizer production). Current climate policies and industry trends are directing and incentivizing the increase of industrial CCS as central technology for reaching climate change targets. Whilst CCS is essential in meeting the emissions targets, as already stated by the IPCC in 2005 (Metz et al., 2005), complications have arisen in putting all our eggs in that basket. To date, the developed carbon emissions market along with major heavy industry players have integrated and adopted a major CCS solution that allows for a “business as usual” approach (Aronoff, 2020).
Industrial carbon dioxide capture, utilization and storage
Industrial, or artificial, carbon capture and storage is usually considered essential to meeting climate goals. However, the potential negative implications of widespread adoption of certain artificial carbon capture and utilization (CCU) and carbon capture and storage (CCS) solutions (under the overall acronym CCUS) are not discussed very often. Technology being developed now, which is likely to be constructed over the next few years, with the expectation of operating for at least 10 years to become economically viable, will place enormous unforeseen burdens on all aspects of activities into the short-term. This is particularly worrisome given the very short (decadal) timeframes which are implicit in the climate models describing future greenhouse gas (GHG) emissions to the atmosphere and consequential climate change used by the IPCC and other expert bodies that (allegedly) describe the climatic paths we may already be heading into due to historic rates of GHG emissions.
Carbon dioxide capture, utilization and storage is, in many ways, a 21st century technological marvel as a climate solution. A major reason for CCUS being so readily considered is its mitigation potential of significantly large amounts of CO2 from point sources. As a brief background of its inception, the IPCC 2005 meeting on climate change first brought CCS into global attention in a weighty expert reviewed special report on Carbon Dioxide Capture and Storage (Metz et al., 2005), which outlined the technology, the costs, the benefits, the complications and the potential for playing a significant role in climate change mitigation.
The UN Framework Convention on Climate Change agreed upon CCS as a Clean Development Mechanism (CDM), which under Article 12 of the Kyoto Protocol, allows:
“…a country with an emission-reduction or emission-limitation commitment under the Kyoto Protocol (Annex B Party) to implement an emission-reduction project in developing countries. Such projects can earn saleable certified emission reduction (CER) credits, each equivalent to one tonne of CO2, which can be counted towards meeting Kyoto targets.”1
CCUS has a key role in achieving the goals of the Paris Climate Agreement targets and are deemed to be vital emissions decreasing technologies by both the IPCC and International Energy Agency (IEA). An important question that is raised as the cost of CCUS roll-outs increases is simply this: is it really worth it? The answers given to that question are certainly not a unanimous “yes” because recent innovations in biotechnological solutions could provide better alternatives, such as improved energy efficiency, renewable energy, or biotechnological innovations.
Before we go further with that proposition, we should establish exactly what CCS is. According to the IPCC 2005 Special report on Carbon Capture and Storage (Metz et al., 2005), CCS is a process consisting of the separation of CO2 from industrial and energy-related sources, transportation to a specified storage location and long-term storage and isolation from the atmosphere. This is currently considered to be the primary tool for mitigation and stabilization of atmospheric greenhouse gas concentrations. The utilization aspect of GHG emissions, or CCU, has more recently been developed as a better practice as compared to CCS due to the utilization of the emissions as a secondary resource rather than solely storing them (there are many industrial uses for captured CO2, such as refrigeration and carbonation of beverages). CCU is therefore more closely suited to a circular economy, but the captured CO2 does return to the atmosphere after such usage.
The capture of CO2 and other GHG emissions via CCUS is applicable to large industrial facilities, where the emissions can be compressed for transport and storage in suitable geological formations, in the ocean, in bedrock as mineral carbonates, or for use in further industrial processes (Metz et al., 2005). According to Zevenhoven and Fagerlund (2010), CCS involves injecting CO2 into host rocks or employing an ex situ application step, reacting huge volumes of CO2 into carbonate minerals, and storing these in geological formations. The initial steps involve capturing the CO2 emissions, followed by transportation and injection. Each step can involve variations in physical and chemical processes, each major CCS project utilising different solutions of varying efficiencies. The end results are nonetheless similar; CO2 either in liquified or mineralized form which is now available for either utilization or direct storage in geological underground pockets.
A more recent review (Hills et al., 2020) discusses mineralization in geologically derived minerals and industrial wastes, emphasizing the manufacture of products with value. The authors suggest that this sort of CCUS technology can manage significant quantities of CO2. Leakage and escape of injected CO2 has been a topic of major concern over the last 2 decades and many pilot experimental studies by expert geologist teams have attempted to allay these concerns. Larkin et al. (2019) listed 29 potential hazards in a risk assessment of CCS injection and storage activities, suggesting that for 0–50 years, 51–499 years and >500 years time periods, the likelihood is approximately 0.1% of the occurrence of major leakage from CCS storage resulting in “…measurable negative effects on human health or the environment…”
Here, we also note the enormously wide uncertainties involved with CCS leakage potential, such as uncertainties in worldwide saline aquifer storage capacity (0.1–76,000 Gt), uncertainties of ultimate percentage CO2 storage capacity in solution in a deep saline aquifer (that ranged 0.2%–76%), and uncertainties in the distances affected by salt precipitation (1–175 m). This last is when salt crystals form during CCS and inhibit the well’s pores, thereby decreasing CO2 holding capacity and consequently decreasing storage capacity and increasing possibilities for permeability and leakage from the well (Ho and Tsai, 2020). Most CCS projects that have been successful to date are site-specific, either pilot or small-to-medium-scale and have yet to reach annual expected injection capacities. Put simply, there is not enough historical data on long-term, wide-ranging, and large-scale CCS to really gauge the impact of potential hazards to be comfortable about global-scale CCS implementation. Confidence in the technology continues to be expressed, however. Miocic et al. (2019) calculated leakage rates from a 420,000 year old naturally occurring, but faulted, CO2 reservoir in Arizona, United States. Surface travertine (CaCO3) deposits provide evidence of vertical CO2 leakage which can be dated by uranium-thorium decay. The data show that leakage varies along faults, and that individual seeps have lifespans of up to 200,000 years. Time-averaged leakage equated to a linear rate of less than 0.01% y−1.
Friedmann et al. (2020) estimate that 85 Gt of CO2 must be captured and stored from coal-fired power generation alone between 2030 and 2050 to be consistent with a 1.5°C climate outcome (Cui et al., 2019; Friedmann et al., 2020). If that 85 Gt reservoir leaks back into the atmosphere at a rate of about 0.01% y−1, the reservoir’s total content of sequestered CO2 will be returned to the atmosphere in 10,000 years. In comparison with the human lifetime, 10,000 years is an unimaginable length of time, but it is totally insignificant compared with the length of time (252 million years) that atmospheric CO2 has remained sequestered in, for example, coccolithophore limestone layers laid down in the Triassic Period. Due to the sheer size and capacities anticipated for CCS storage sinks, assuming the current global trend for fossil fuel use with CCS continues, even tiny error margins could result in thousands of tonnes of CO2 leaking back into terrestrial and coastal ecosystems with potential for environmental damage along the same lines as contaminating leachates from historic landfills or mines implemented by our engineering forefathers.
Whilst the economic and energy-system risks due to potential CCS leakage are arguably modelled with confidence (Liu et al., 2016; Deng et al., 2017), it is our environmental ecosystems that are calling for more attention. Industrial CCS has small risks, but huge consequences for our environment. The key question is “what if?” Once the gas is in storage, there is no going back, and the environmental risks can only be managed after complications arise. CCS technology is arguably the most significant and powerful carbon sequestration tool we have that can serve as a point-source, “brute-force” carbon sink solution. Although relatively few sites, globally, are suitable for CCS (because the geological characteristics must be perfect), several sites have been found and classified as having the giga tonnage (Gt) CO2-storage potential required to meet Paris Agreement climate goals.
The Global CCS Institute is the leading organization and knowledge-base on CCS projects for industry as well as research and development (https://www.globalccsinstitute.com/). According to this Institute’s website, current CCS projects either in operation or under procurement or construction have been estimated to sequester CO2 at rates from 100,000 to 30 million tonnes per annum, per CCS project site. Operational lifetimes are expected to be at least 25 years. As an example, the CarbonNet Project located in South Gippsland, Victoria, Australia is working towards establishing a commercial scale CCS network with storage at the project’s Pelican site in Bass Strait, off the Southeast coast of Australia’s “Ninety Mile Beach.”
The site is projected to sequester up to 5 million t of CO2 annually: a significant quantity of CO2 gas. On a molar mass basis, carbon represents 27.29% of the mass of CO2. Consequently, that 5 million t of CO2 corresponds to 1,364,500 t of carbon removed from the atmosphere annually by the individual Pelican Site CCS facility. The key consideration here is that these large point-source quantities of CO2 are, for the most part, found in heavy industrial plant sites. Artificial CCUS solutions include but are not limited to CO2 injection or subsurface mineralization, CO2 flooding and enhanced oil recovery (EOR), deep sea storage (such as deep water pressurized storage conveyed by pipe), which are the major solutions. Less impactful, but equally innovative are: Direct Air capture and storage (DAC), Dry Ice Emissions capture (e.g., DecarbonIce™; https://cero2050.es/en/decarbonice) or capturing CO2 from hydrogen production (e.g., CryoCap™; https://tinyurl.com/mn6crpn4).
Although sceptics have raised significant concern for the environmental risks involved with CCS projects, the science has (so far) proved its safety and efficacy, albeit, at very small pilot field trials scales. As a result of stricter government policies towards fossil fuel use and of heavy GHG emissions in general, the major CO2 emitters (namely fossil fuel companies) have sought to invest into CCS as a business solution to become carbon neutral. In turn, the highest quantifiable CCU/CCS technologies are capitalizing on a new market demand created by government policy, where major heavy industries and GHG emitters are needing to protect themselves and their financiers against possible future sanctions.
The 2019 report of the US National Academies of Sciences, Engineering, and Medicine entitled Negative Emissions Technologies and Reliable Sequestration: A Research Agenda (NASEM, 2019) describes negative emissions technologies, or NETs, for mitigating climate change. These have a biotechnological component that removes and sequesters CO2 from the atmosphere. Though they have received less attention than industrial technologies, NETs are promising alternatives to industrial methods that focus on decreasing the level of future CO2 emissions by limiting fossil fuel consumption, because this requires massive deployment of low-carbon technologies and agricultural land-use change between now and the target date of 2050. One key point here is that CCUS is more useful for achieving zero or carbon neutral operations, not negative, especially when the CCUS-facilitated plant is not processing biological or waste resources (also known as “BECCS,” Bioenergy with CCS; https://tinyurl.com/mwfxwkfp).
According to the Global Carbon Project, about 37 billion tonnes of CO2 gas was emitted globally by heavy industries in 2019.2 The number of heavy emitting industrial facilities is rising, particularly in Asia. To date there are more than 5,000 large facilities globally that produce CO2 emissions above 1 million tonnes per year. Again, due to recent industrial development in Asia and lacking regulatory action or initiative, this number continues to grow significantly. Interestingly, the number of CCS industrial facilities under development between 2010 and 2017 lessened, though this was followed by a recent resurgence in development of the technology. To date, close to 40 CO2 injection facilities have been brought into operation (mostly in the United States) and many more are in development. This activity is monitored by the Center for Climate and Energy Solutions,3 an independent, nonpartisan, nonprofit organization which claims to be “… working to forge practical solutions to climate change…”4
For those already in operation CCS is implemented as an add-on or retrofit to heavy industrial facilities; particularly in the oil and gas industries and fossil fuel energy generators, but also cement, steel, and fertilizer producers, though the technologies are generally applicable to any CO2 emitting facility. The CCS system captures CO2 produced directly from the industrial plant’s output flue gases and pumps it underground into deep saline pockets under cap rock. Although injection into sedimentary basins has been commonly conducted for enhancing oil recovery from certain wells (Enhanced Oil Recovery is one of the business goals of CSS), it has been proved that basaltic cap rock pockets provide much more safety and encapsulation for mineralized CCS storage into stone (with pioneer work laid out via pilot studies in Iceland.5 Possibly the most exotic carbon storage plan is that which intends to convert captured CO2 to methane (CH4) and use that to make diamonds.6
The costs of CCS adoption were discussed in the Special Report Carbon Dioxide Capture and Storage prepared by Working Group III of the Intergovernmental Panel on Climate Change (Metz et al., 2005). According to Kheshgi et al. (2012) the publication of this report:
“…raised the profile of CCS…among the expert community dealing with international climate policy (Clarke et al., 2009; IEA, 2010; Meadowcroft and Langhelle, 2011).”
We illustrate costs of CCS adoption in Table 1, for which we have recalculated the cost ranges given in the original 2005 publication using the Consumer Price Index inflation calculator of the US Bureau of Labor Statistics as featured on Ian Webster’s website.7
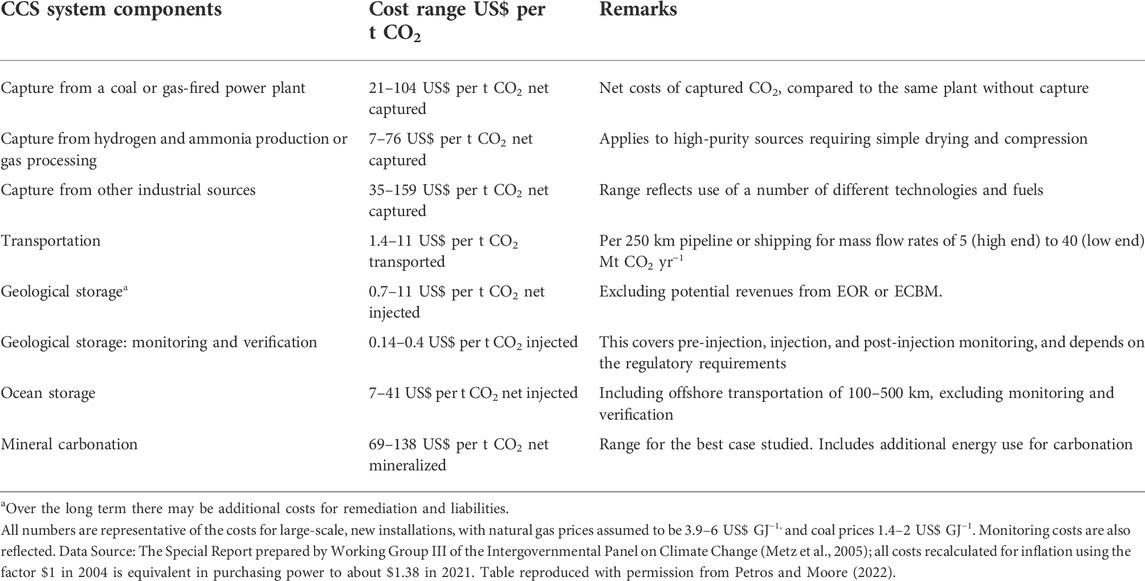
TABLE 1. Cost ranges for the components of a CCS system as applied to a given type of power plant or industrial source.
Despite the potential economic advantages of CCUS apparent from Table 1, the technologies face several practical and economic barriers that must be overcome before they can be deployed on a sufficiently large scale, and over a sufficiently long time interval, to make serious inroads into the atmosphere’s accumulated fossil-CO2 burden. The main economic and environmental hurdles in sight are:
• the significantly large capital investment and hard infrastructure required for implementation, very long term operation and maintenance (many thousands of years as a minimum); and
• the extremely energy-intensive processing required for carbon utilisation (CU) or sequestration (CS).
Those two points identify the most important disincentive to CSS implementation: its cost. This was foreshadowed in IPCC’s special report on CCS, which stated that fossil fuel-based power facilities equipped with CCS for mineralised subsurface injection, will require 60%–180% more energy (more energy = more cost) than a power plant without CCS (Metz et al., 2005).
Table 2 shows the total costs of CCS and electricity generation for three power systems with pipeline transport and two geological storage options. Again, the data is sourced from the Special Report Carbon Dioxide Capture and Storage prepared by Working Group III of the Intergovernmental Panel on Climate Change (Metz et al., 2005), with costs adjusted for inflation as described in Table 1.
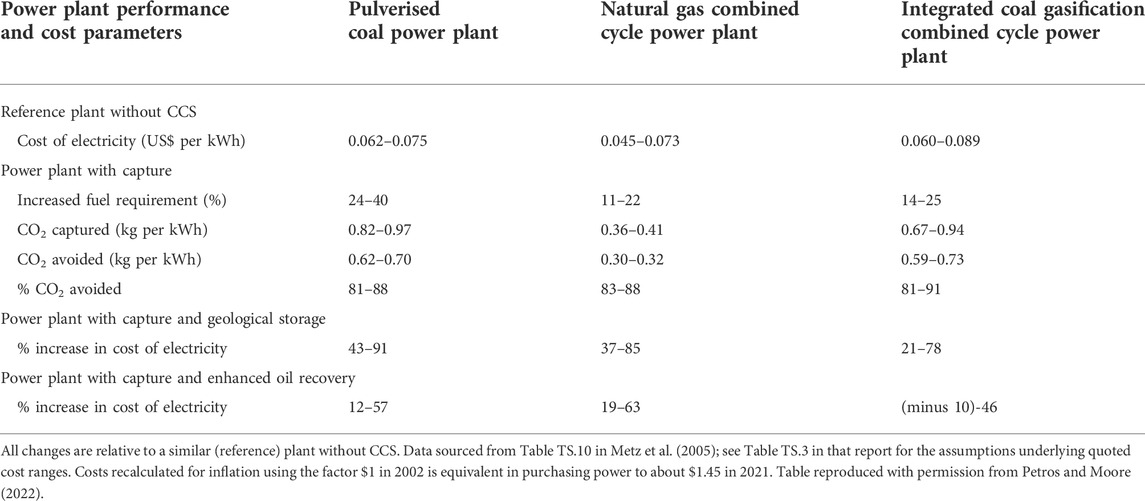
TABLE 2. The costs of CO2 capture, transport and geological storage for new power facilities using bituminous coal or natural gas.
The early recognition of this energy penalty may well be the reason for the relatively late uptake of CSS technology by the power generation industries, as compared with gas-processing industries. Though, of course, the scale of the infrastructure required by power generation facilities and the long lead times required for its design and implementation must also have contributed to the marked differences evident between the industries. We have assembled a summary of cost estimates of CCUS technologies, and their CO2 removal rates in Table 3 (see Petros and Moore, 2022 for comparative discussion).
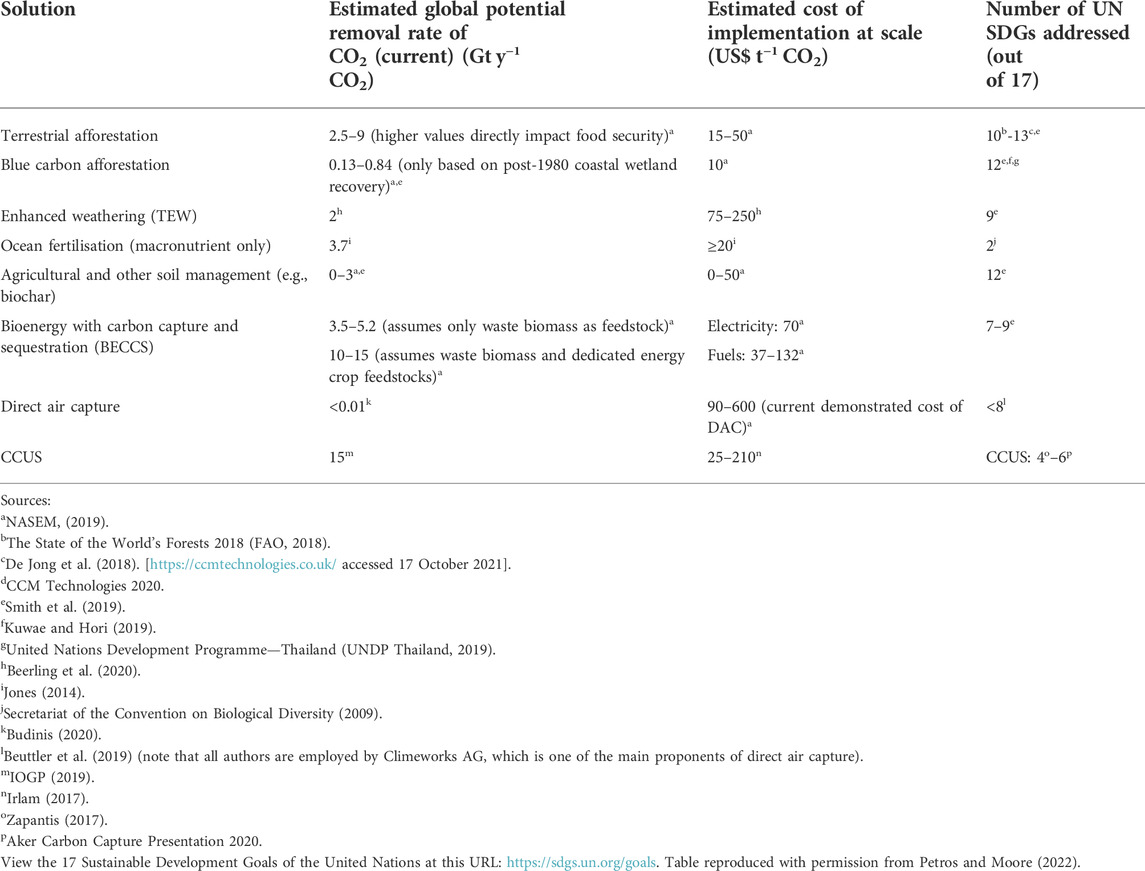
TABLE 3. Summary of CCUS solutions including cost estimates, CO2 removal rate estimates and UN Sustainable Development Goals (UN SDGs) addressed.
The UN Sustainable Development Goals (SDGs8) are shown in the final column of Table 3 because in pursuance of the Paris Climate targets through climate change mitigation technologies (artificial or bio-based), we must consider both the opportunities and risks associated with such solutions that remove GHGs from the atmosphere. Such an approach is helpful in determining the true sustainability of potential solutions because value factors such as land and water use, cultural and land heritage as well as biodiversity and nutrient stocks are given significant weighting. Smith et al. (2019) also explored this for land-based solutions and their impact on ecosystem services.
Especially for solutions that help conserve or improve natural ecosystem services, the valued benefits usually go far beyond what project engineering or financial models would normally include. Meaning, we should be placing even higher-than-usual value on natural capital and global environmental health improvement indicators on current and future decision-making.
There are some other issues that seem to be held in the background of the CCS arena, though common in the business world. These result in some ambiguity in regards to how climate is to be managed, raising the questions: where does the controlling influence and interest lie, and who are the major stakeholders? These are robust questions that need to be asked, especially in a situation where CCUS is most wholeheartedly backed by the major fossil fuel-based enterprises themselves. A quick analysis of the Global CCS Institute’s current (December 2020) 88 members,9 at least 48 out of 88 members rely on or have direct business interests in fossil fuel use. A further 17 members currently rely on fossil fuel industries either indirectly or partially, leaving only 22 of the 88 members with no immediate evidence of business reliance or connection to fossil fuel use. However, it is important to keep in mind that these members might also have significant shareholdings or be subsidiaries of upper tier companies who do have vested interests in continued fossil fuel use (e.g., CCM Technologies at https://ccmtechnologies.co.uk/). Here, we looked only as far as each company’s web page or available Wikipedia descriptions in late 2021.
The Global CCS Institute recognises the IPCC’s latest targets in a September 2020 report (Friedmann et al., 2020) these certain actions are:
• A 50% decrease in CO2 emissions is needed to achieve net-zero climate goals by 2030.
• A rapid implementation of climate mitigating infrastructure is needed urgently, including the expansion of CO2 pipelines from the current 8,000 to 43,000 km by 2030.
• Urgent development and implementation of clear climate policies to optimise financial and regulatory risk mitigation for CCS infrastructure.
43,000 km of CO2 pipeline is a lot of hard infrastructure. So, let us assume that by 2030 we achieve a decline in fossil fuel usage and then ask ourselves: will that not make some of these pipelines redundant? We must not ignore the fact that retrofitting conventional fossil-fuel industrial facilities with CCS serves not only to assist in climate change mitigation, but also to create redundant hard infrastructure for future generations, not to mention the enormous continual efforts required to monitor and manage the thousands of highly concentrated CO2 sinks that come with this direction.
Of course, some industrial facilities may be able to convert to biomass-use instead of total decommissioning, but the costs of conversion will usually outweigh the construction of a whole new facility, particularly given the likelihood of more cost-effective and optimised designs, construction/manufacturing materials and technological services that will be available decades from now. The scenario can be seen as similar to the legacy of ponds for mine-tailings; we are now seeing more and more closed mining sites requiring growing amounts of risk management, primarily environmental.
On another important note, Krüger (2017) published an interesting piece on the conflicts over CCS in international climate governance, postulating two theses:
• That the future of climate governance is contingent on decisions about the continued use of fossil fuels.
• That CCS-conflicts have an unpredictable influence that could lead to implications and cracks within the paradigm of ecological modernisation and thus could politicise international climate policy.
Krüger (2017) discusses the consequences of allowing private business interests to determine the direction of humanity’s future. The problem, however, is one of necessity. On the one hand, Carbon Capture Utilisation and/or Storage (CCUS) is a power-house technology that could play a central role in deciding where humanity ends up by the end of the 21st century. On the other hand, because it is desired most by fossil fuel-reliant enterprises to safeguard their own business, CCUS is tainted with contention. It may be the magical release from our worst nightmares; or it could be the Poisoned Apple which will send us into the Sleeping Death of our times.
Artificial CCS solutions are researched, developed, and engineered to address specifically the question of “how can we prevent GHG emissions entering our atmosphere?” However, if these artificial CCS solutions are continuously implemented, unchecked rapidly and widely, they could result in serious implications and even more problems for our future generations of scientists and engineers. As we see it, the problem is that CCUS has attracted market-trading, but without the optimal regulatory framework and market rules that would alleviate mistrust, misguidance, and corruption. The carbon trading schemes that have been opened in many nations to date have yielded both positive and negative results in relation to the problem posed by climate change. As the initial goal of carbon sequestration is to decrease atmospheric CO2 levels, the primary goal of a carbon market or carbon trading scheme is to sequester the most carbon. As a result, industries and corporations have started to look at technologies that will sequester the most carbon, and that aligns with their future business plans. These are the methods of carbon sequestration best supported by fossil fuel companies and are therefore not the ideal solutions for our environment and its ecosystems. It is the technology that secures the industry’s business plan and market position heading forward into the future, rather than the technology that is best for planet Earth.
The implications emerge more clearly when we understand how the carbon market works and who are the current big players. It is also important to remember that money is the key hurdle for change and in this case, where the money is channeled and what it is directed towards.
The carbon market
The importance of carbon sequestration will be increasingly significant as we proceed further into the 21st century. Not only is carbon sequestration an environmental and atmospheric issue, but it is also now considered an economic market, whereby carbon credits are offered by legislators and a carbon market continues to be expanded and refined. Nations currently have a monetary value assigned to the quantity of carbon directly emitted into the atmosphere. By doing this we have created the greenhouse gas (GHG) emissions market or emission trading systems (ETSs). As such, we have “put a price on carbon” and from this point on we will call it simply “the carbon market.”
Described as a unique environmental commodity, the carbon market was created out of the Kyoto Protocol. This international treaty extends the 1992 United Nations Framework Convention on Climate Change (UN, 1992), committing nations to limit greenhouse gas emissions, based on the scientific consensus that global warming is occurring and is most likely caused by human-made CO2 emissions. The Kyoto Protocol, completed in December 1997, required industrialized countries to lower their total greenhouse gas emissions to 5.2% below 1990 levels (Jacobson, 2001). As listed in Annex A of the Protocol, developed countries must limit all GHG emissions, which are carbon dioxide (CO2), methane (CH4), nitrous oxide (N2O), hydrofluorocarbons (HFCs), perfluorocarbons (PFCs), and sulfur hexafluoride (SF6).
This is a point worth remembering: ultimately, regulatory policy has the power to assign value and create economic markets, no matter what the value-assigned object might be (a service, a chemical, object or organism, an environment or a pollutant). The markets or ETSs that trade Environmental Commodities emerged as a way to buy and sell the right to pollute. The question that needs to be asked is whether the future of humanity on this planet would be better served by markets based on Global Health rather than Global Pollution? Many would agree that after more than 2 decades since adoption of the Kyoto Protocol, ETSs and the 16 compliance carbon markets in operation across the world have failed in their primary objective of ensuring significant decreases in GHG emissions to curtail anthropogenic-inputs and mitigate rising atmospheric GHG input (Pearse and Böhm 2014).
More clearly as of late, has been the misguided allocation of carbon credits and carbon offsets in the name of business, rather than in the name of climate change; meaning, in short, that the rich and powerful win more than poorer nations. Carbon credits are being used increasingly to finance nature-based solutions but are of varying quality, with some being of doubtful permanence and/or having little regard for social and ecological factors (Girardin et al., 2021). Accounting needs to be improved as well. An analysis of forest carbon accounting indicates that the common carbon market mantra “plant a tree to save the atmosphere/biodiversity/world” is based on commercial forest carbon protocols that have overestimated the carbon trading value of forest carbon by about 2½ times for more than 2 decades (Marino and Bautista, 2022). Whilst Cziesielski et al. (2021) comment that sustainable ocean management and “…inclusive governance will be central to ensuring equitable and just development of the blue economy…” Though we share this opinion, we do not wish to develop this political narrative here; suffice to say in summary that the current rules and regulations built by policy-makers have created a flawed carbon market in order to solve the climate change crisis, albeit with good initial intentions. So, what is the alternative?
In short, we consider that redefining market value is the key. An ideal, possibly utopian, scenario might be one where the market focuses primarily on improving and sustaining global environmental health and secondly on GHG emissions cutbacks (though the latter would be a significantly weighted factor). Global health fundamentally relies on:
• raising environmental awareness,
• continuous educated decision-making,
• sympathetic planning protocols,
• timely action,
• full implementation,
• extensive monitoring,
• conservation of environmental systems.
Whereas GHG emissions and carbon trading, by definition, can be produced, lowered, moved around, traded and sequestered, global health cannot and should not be passed around. The policies would ideally settle on any management body or agency holding responsibility for their local environment and the global environmental impact of their businesses. Doing anything like this effectively requires great improvements in today’s over-simplified biological models so that they provide robust and useful standards by which “environmental health” can be judged.
If value is assigned to global health, then global markets must be regulated with rules that uphold the natural capital values that the Earth’s natural ecosystems offer as services (also known as ecosystem services). Such a move would fundamentally shift us towards planning and implementing true circular economy with our planet and a healthy and harmonious relationship from market to industrial and commercial ventures to communities. As we all know, increasing carbon emissions, atmospheric GHG levels and global warming result from a complex system of biogeochemical processes affected by many anthropogenic practices with, potentially, many different tariffs. Because of this, rather than a carbon trading market, it would make more sense to introduce a global environmental health market that offers traders and participating industries and businesses, alongside the carbon credits, trading credits that could be equally important contributors to our attempts to avert global warming. For example, biodiversity credits, ecosystem service credits, and biomimicry-of-technology credits.
That’s not what we have. Instead of introducing a fully environmentally sensitive carbon market, we only have a carbon market. What is concerning about current practices is that removal of carbon from the atmosphere is the only environmental concern and those other global environmental health concerns are not at the forefront of any aspect of the carbon trading market. The value is placed on removal of carbon from the atmosphere at almost any cost. Consequently, the money (what little is left of it after successive traders have taken their top slice) therefore, goes to carbon credits, not environmental credits (listen to the BBC World Service podcast Does big money really believe green is good?10).
We would rather see a market, that consists of rules and regulations based on a global environmental health market focused on altering the root anthropogenic causes that have resulted not only in global warming, but in active destruction of ecosystems by over-exploitation, global loss of biodiversity, and anthropogenic species extinctions at rates not seen since the darkest days of the planet’s geological history (Hannah, 2021). The carbon market is already established, with the ebb and flow of supply and demand circulating. But it is important, as we make more serious attempts to ameliorate the damage our industrial activities have already done to the atmosphere, that rather than concentrating solely on the symptomatic results of unsustainable anthropogenically-raised GHG emissions, we do not forget those broader anthropogenic mistakes that should be change-incentivized towards restoring and maintaining the natural circular economies of healthy environmental ecosystems. Between the additional energy required for industrial CCS, the CO2 emissions during the process and the leakage during storage (which certainly increases with the years), it seems that twice as much oil and gas would have to be extracted to store the CO2 emitted simply by the current use of these fossil fuels. Widespread use of CSS would be like being blindfolded on the edge of a precipice and taking a big step forward!11. Rather than using renewable energy to power industrial CCS, renewable energy should be used directly for human consumption. Then neither the original fossil fuel extraction nor the related CCS is needed.
Hard carbon sequestration solutions
The “hard” carbon sequestration solutions available to us include the following processes (some of which have been introduced above):
• CCUS and mineralisation; in the latter part of this combined process, exposed surface rocks, such as peridotite and basaltic lava, react with CO2 in ambient air, or in the subsurface where concentrated CO2 streams are injected into rocks to mineralise in their pores.
• In direct air capture (DAC), chemical processes concentrate CO2 from ambient air for injection into a storage reservoir or use it in secondary industries.
• Bioenergy with carbon capture and sequestration (BECCS) involves using plant biomass as an energy source, primarily to produce electricity by one of two methods combustion or conversion. Combustion uses the biomass directly as a furnace fuel for conventional electricity generation or for other furnace-based industrial applications (cement, paper pulping, waste incineration, petrochemicals and steel and iron production). Emitted CO2 is captured from the flue gas stream resulting from combustion. Conversion of biomass involves digestion (“composting”) or fermentation to produce gaseous or liquid fuels. Fermentation is used widely to produce pharmaceuticals, alcoholic beverages, and foods like mushrooms, cheeses and salami. The main liquid fuel is bioethanol, which produces almost pure CO2 during fermentation, and the main gaseous fuel is methane, generated by anaerobic digestion of biomass, including household food, and garden and farm wastes. The subsequent combustion of the biofuel or biogas by the end user also produces CO2, of course, which, if not stored, is returned to the atmosphere by the end user. The advantage of BECCS derives from the fact that bioethanol and biogas are produced using this year’s crop and food wastes, so it is this year’s atmospheric CO2 that is recycled to the atmosphere, resulting in a net saving in emissions of fossil fuel-derived CO2. The emissions amelioration is in proportion to the ratio between biofuel and fossil fuel used, which is dependent on the combustion engineering of the end user machines. In 2019 there were five BECCS facilities around the world, collectively capturing approximately 1.5 million tonnes of CO2 per year (Mt y−1). BECCS is a way to avoid use of fossil fuels, in addition to its capture and storage aspects. The biomass feedstock can be derived from a waste material (e.g., sugarcane wastes which are widely used for bioethanol) or dedicated energy crops (e.g., fast-growing tree species) planted purely as an energy-production feedstock. At the present time, biomass feedstock supply for energy generation by burning is dominated by forest management schemes (Consoli, 2019). The fact that farming fast-growing trees for fuel on scarce agricultural land conflicts with use of that land for growing food is a major issue which has not been adequately addressed. The overall BECCS process can provide a net decrease of CO2 in the atmosphere if combined with capture and sequestration of CO2. Industry opinion of BECCS is essentially that it is the best solution to decarbonise emission-intensive industries. However, public perceptions of this technology are variable and seem to be linked to the regulatory policies by which its use is incentivised (Bellamy et al., 2019). Payments based on the amount of CO2 removed from the atmosphere were approved but guarantees of higher prices for producers selling energy derived from BECCS were strongly opposed. It remains to be seen whether the recently (19 April 2021) announced winners of the $20M NRG COSIA Carbon XPRIZE, a prize that set out to convert CO2 emissions into valuable products, can change these public perceptions, at least as far as production of traditional concrete is concerned. Concrete being “the world’s most abundant human-made material …[accounting]… for seven percent of all global CO2 emissions,”12 both $7.5M grand prize winners developed technologies focused on decarbonising concrete and converted the most CO2 into products with the highest value, while minimizing their overall CO2 footprint, land use, water use, and energy use.
• CarbonCure Technologies produce concrete with a lesser water and carbon footprint without sacrifice to the material’s reliability by injecting a precise dosage of CO2 into a concrete plant’s reclaimer system, which contains the water used to wash out concrete trucks and mixers. In tests under industrial conditions, 25 tonnes of CO2 per day supplied by the flue gasses from an adjacent natural gas-fired power plant was converted to a permanently embedded mineral with strength-enhancing properties which can be incorporated into new concrete mixes. Overall, the technology decreases the material costs and increases profitability for concrete producers (view: https://www.carboncure.com/, https://www.carbonbuilt.com/).
• The Los Angeles-based UCLA CarbonBuilt developed technology that lowers the carbon footprint of concrete by more than 50% while reducing raw material costs and increasing profitability. The CarbonBuilt concrete formulation significantly decreases the need for ordinary Portland cement by direct injection of CO2 from flue gas streams during the curing process of concrete mixtures. In this process, also, the CO2 is mineralised and permanently stored (view: https://www.carbonbuilt.com/).
Additionally, the NRG COSIA Carbon XPRIZE awarded X-Factor awards to two finalists that created other valuable products from waste CO2:
• Carbon Upcycling-NLT13 produces nanoparticles with applications in various industries, particularly concrete, construction and plastics.
• Carbon Corp14 transforms CO2 into carbon nanotubes, with applications such as lightweight, ultra-strong and cost-effective replacements for metals; stronger cement-composite building materials; and expanding applications in industrial catalysis, batteries, and nanoelectronics.
• Enhanced weathering or accelerated weathering refers to methods of CO2 removal from the atmosphere that use natural or artificially created minerals which absorb CO2 and transform it into other substances through aqueous chemical reactions.15
• Ocean fertilisation has also been suggested as a CO2 removal technique involving tipping iron filings or other nutrients (e.g., urea) into the seawater in areas that have low photosynthetic production to stimulate phytoplankton growth. The idea is that the new phytoplankton will absorb atmospheric CO2 and, when the phytoplankton die, the carbon is expected to be sequestered “as they sink to the ocean floor.” Over the last 30 years there have been at least 13 ocean iron fertilisation experiments. However, studies have shown that the amount of carbon exported to the deep sea is either very low and/or undetectable because much of the carbon is released again through the food chain (view: https://www.geoengineeringmonitor.org/2018/05/ocean-fertilization/).
CCS technologies promise the sequestration of atmospheric carbon but, inevitably, without proof of their permanence. CCU technologies promise removal of CO2 from the atmosphere for its use in other processes, but the sequestration aspect is then left in the hands of the end-user of the solid or compressed gas, and inevitably (again) most of that gas will simply vent back into the atmosphere. CCUS technologies provide captured CO2, nothing more (although, admittedly, CCU can provide you with a cold, sparkling drink while you watch the climate change). In contrast, nature-based solutions perform other crucial ecosystem services in addition to sequestration of atmospheric carbon. This is true even of those nature-based processes that offer only temporary carbon sequestration. And there are some nature-based solutions that offer numerous ecosystem services alongside the certainty of carbon sequestration for geological periods of time. The sections below briefly outline the nature-based (or “soft”) alternative solutions. We will look at each solution holistically and from a sustainable infrastructure point of view, including consideration of the capital value offered by each solution to society as a whole.
The comparison with “soft” (nature-based) carbon sequestration
Key climate-focused actions are required now in order to avoid climate catastrophe. As we progress into the third decade of the 21st century, climate records proved that “…2011–2020 was the warmest decade on record, with the warmest 6 years all being since 2015…” (WMO, 2020), while the Copernicus Climate Change Service satellite data showed that 2020 was statistically at dead heat with 2016 as the world’s warmest year on record. These data are gathered by a constellation of Sentinel satellites, called Copernicus, that monitor the Earth from orbit, together with ground level measurements. Temperature data from the system shows that 2020 was 1.25°C warmer globally than the average from 1850 to 1900, a time often described as the “pre-industrial” period. (https://climate.copernicus.eu/). Furthermore, the Carnegie Climate Governance Initiative report (Mace et al., 2021) makes clear that: “…it is no longer sufficient to reduce emissions alone. Instead, CO2 will also need to be removed from the atmosphere, on a scale never previously attempted…” But, while a number of reporting rules and accounting practices are already in place with direct applicability to the implementation of carbon dioxide removal options, many governance gaps remain. From their analysis of why private and public sectors must invest in protecting, preserving, and enhancing the blue natural capital of the Red Sea, Cziesielski et al. (2021) conclude that: “…communication, participation, and transparency of all involved parties are required to successfully build a blue economy that thrives with its natural resources…”
Soft carbon sequestration solutions include all the nature-based negative emissions technologies (NB-NETs), which differ from “hard” solutions mainly in terms of natural capital. The “hard” solutions (CCUS and direct air capture in particular) lack natural capital, primarily biomimicry-of-technology functionality, and ecosystem services. These aspects are provided by the “soft” NB-NETs. As described elsewhere (Moore et al., 2021a), these NB-NETs are of low to medium expense (US$100 t−1 CO2 or less) and offer ample capacity for safe scale-up from current levels of operation. Griscom et al. (2017) provide a succinct overview of natural climate solutions (NCSs), which encompass “soft” carbon sequestration potential. According to the study: “…NCSs can provide over one-third of the cost-effective climate mitigation needed between now and 2030…” to satisfy the IPCC’s “below 2°C model.” However, this can only be achieved via aggressive fossil fuel emissions decreases, which, if achieved, can allow NCSs to offer a powerful set of solutions for Paris Climate Agreement nations. As an added natural capital benefit, “soft” solutions help improve soil health and productivity, clean air and water and help restore and maintain biodiversity and healthy nutrient flow. They showed that most NCSs, when implemented effectively, offer additional benefits such as water filtration, flood risk reduction, improved soil health, improved habitat biodiversity, and enhanced climate resilience, and they concluded by noting the need for:
“…immediate global action to improve ecosystem stewardship as a major solution to climate change…” (Griscom et al., 2017).
Another valuable source of detailed information is the 2019 report of the US National Academies of Sciences, Engineering, and Medicine entitled Negative Emissions Technologies and Reliable Sequestration: A Research Agenda (NASEM, 2019). The Committee on Developing a Research Agenda for Carbon Dioxide Removal and Reliable Sequestration, which produced this report, was created to recommend a detailed development plan for research into negative emissions technologies (NETs) that remove CO2 from the atmosphere into sinks to mitigate climate change. NETs have received much less attention than the “hard” technologies, but this report concludes that deploying NETs may be less expensive and less disruptive than cutting some emissions, such as a substantial portion of agricultural and land-use emissions and some transportation emissions. NETs are envisaged by this Committee to:
• “…use biological processes to increase carbon stocks in soils, forests, and wetlands,
• produce energy from biomass, while capturing and storing the resulting CO2 emissions,
• use chemical processes to capture CO2 directly from the air and then sequester it in geologic reservoirs,
• enhance geologic processes that capture CO2 from the atmosphere and permanently bind it with rocks…” (quoted from NASEM, 2019).
Several conclusions that outline the main thrust of the research agenda this report goes on to develop are listed in its summary. Their Conclusion 2 highlights some negative emissions technologies that are said to be ready for large-scale deployment: “…afforestation/reforestation, changes in forest management, and uptake and storage by agricultural soils…” (quoted from NASEM, 2019).
Because photosynthetic carbon capture by trees and other plants is so widely believed to be an effective strategy to limit the rise of CO2 concentrations in the atmosphere by sequestering carbon in the plant body, this is possibly the report’s most conventional aspect. The Intergovernmental Panel on Climate Change Special Report of 2018 (Masson-Delmotte et al., 2019) suggested that an increase of 1 billion hectares of forest (= about 1–2.5 trillion additional trees) “…will be necessary to limit global warming to 1.5°C by 2050…”16 All of these proposals involve agricultural land being used for planting trees, changes in managing existing forests, or shifts in agricultural practices that enhance carbon storage in agricultural soils. Yet, we have noted before (Moore et al., 2020) that the land surface of planet Earth is equivalent to about 0.25 ha of farmland per person, but only about 0.12 ha per person of farmland is suitable for producing grain crops. The existing state of affairs is that Earth does not have enough land for all its human inhabitants to enjoy an affluent diet as that is presently defined (and see Tables 1, 2 in Miller and Gardiner, 2003). This is our primary reason for advocating greater use of the planet’s oceans for both sustainable food production and sustainable carbon sequestration.
The authors like trees (and other plants) and we are in favor of planting more of them, but they should be planted for their intrinsic ecosystem value, because there are negative aspects to relying on them so heavily as a way to sequester carbon from the atmosphere on the long term basis required for full and lasting benefit (Moore et al., 2021a; and see the next section). Tree planting schemes could make a major contribution to improving our atmosphere, but the rate and scale of their urgent implementation is enormous because “tree numbers have declined to nearly half since the start of human civilization and over 15 billion trees are lost on an annual basis” (Crowther et al., 2015). Even greater losses seem likely in view of the regular news stories of recent years of increasingly serious wild fires around the world caused by climate change. The Trillion Tree Initiative is a World Economic Forum initiative, designed to support the UN Decade on Ecosystem Restoration 2021–2030, led by the United Nations Environment Programme (UNEP) and the Food and Agriculture Organization of the United Nations (FAO).17 This, and the parallel programme Trillion Trees, which is a joint venture between BirdLife International, Wildlife Conservation Society (WCS) and the World Wide Fund for Nature (WWF)18 sometimes seem to be the only nature-centric solutions catching the attention of mainstream media.
Biotechnology: Photosynthetic organisms are not the solution
From discussions aimed at finding some way of combating climate change, proposals have been made to develop biological methods that would pull carbon dioxide out of Earth’s atmosphere and sequester it in some way on a long-term basis. One frequently recommended approach is to remove CO2 from the atmosphere with activities such as reforestation and changing forest management and agricultural practices to enhance soil carbon storage. However, it is also noted that such activities would limit land for food production and negatively affect biodiversity. Furthermore, decay of dead wood and fallen leaves in natural forests releases huge quantities of CO2 and other greenhouse gases back into the atmosphere, even in the same year the carbon was sequestered. Trees are widely cultivated for the timber they produce, and many people now expect this timber to sequester carbon from the atmosphere. Indeed, photosynthetic carbon capture by trees is widely considered to be possibly our most effective strategy to limit the rise of CO2 concentrations in the atmosphere, and there are several ambitious targets to promote forest conservation, afforestation, and atmosphere restoration on a global scale.
As mentioned above, the Intergovernmental Panel on Climate Change Special Report of 2018 (Masson-Delmotte et al., 2019) suggested that an increase of 1 billion hectares of forest will be necessary to limit global warming to 1.5°C by 2050 (typical tree densities range from 1,000 to 2,500 trees per hectare). In the same publication year, Bastin et al. (2019) estimated that the world’s ecosystems could support an additional 0.9 billion (0.9 × 109) hectares of continuous forest (which represents an increase of over 25% of the presently forested area) and that such a change has the potential to cut the atmospheric carbon pool by about 25%.
However, an increasing number of recent studies have warned against too great a reliance on tree planting. For example, Boysen et al. (2017) noted that because they are likely to be monocultures of fast-growing species quite different from the native species, plantations cultivated to sequester carbon would potentially diminish biodiversity, and are likely to occupy agricultural land that might otherwise be used for primary food production. These authors cast doubt on the viability of tree planting as a method of long-term sequestration of carbon from the atmosphere, concluding:
“…that this strategy of sequestering carbon is not a viable alternative to aggressive emission reductions…” (Boysen et al., 2017).
Friggens et al. (2020) recorded a 58% decrease in soil organic carbon stocks 12 years after birch trees (Betula pubescens) had been planted in moorland, so planting trees on peatland will not help either. This decline was not compensated by gains in carbon contained in the growing trees. This was part of a long term study of the effects of planting widely distributed native tree species (Betula pubescens and Pinus sylvestris), in Calluna vulgaris moorland in Scotland. The study demonstrated that 39 years after planting, the carbon sequestered into tree biomass did offset the carbon lost from the soil but, crucially, there was no overall increase in carbon sequestered in the ecosystem (Friggens et al., 2020).
The peatland ecosystem (also called bogs, mires, moors, or muskegs) is the most efficient terrestrial carbon sink on the planet because they are waterlogged. The resultant anoxic conditions below the surface vegetation results in the annual rate of biomass production being greater than the rate of decomposition in natural peatlands. It is the mosses, typically species of Sphagnum, that thrive in peatlands that retain rainwater, so in addition to carbon sequestration, an important function of peatlands is the stabilization of water flows to descrease the risk of flash flooding. Peat bogs also filter and clean catchments around domestic water reservoirs. But the mosses grow slowly, and although 1 ha of healthy peatland holds as much carbon as 1 ha of tropical rainforest, they offer only limited promise for carbon sequestration. It takes thousands of years for peatlands to reach their full potential. In the northern hemisphere, peatlands presently cover an area of about 3.7 million km2; half being permafrost. These northern peatlands are estimated to store around 415 billion metric tons of carbon, in deposits of peat which have average depths of 1.5–2.3 m in the boreal [northern] peatlands. Hugelius et al. (2020) projected that global warming will in time cause the northern peatlands to become a major source of methane, carbon dioxide and nitrous oxide emissions into the atmosphere. In Sphagnum moss bogs the water table must be maintained close to the surface to maintain the deeper layers of peat as a stable carbon sink. If they are drained, eroded or disturbed (and they have traditionally been mined as a source of domestic fuel, and more recently horticultural composts) the deeper layers are oxidized, and historical CO2 returns to the atmosphere. It comes down to deciding how much of your land you want to be permanently waterlogged, and preferably frozen.
This is a global problem. The UK’s Office for National Statistics (ONS, 2016) estimated that in 2007 UK soils contained approximately 4 million tonnes of carbon, of which 57% was the carbon stored in peat soils, but as the majority of UK peatlands are degraded (Natural England, 2010), they are a highly significant source of greenhouse gas emissions. Consequently, the aim of peatland restoration is to lower the extent of these emissions as a contribution to the “net zero future” (Natural Capital Committee, 2020). The authors of the Natural Capital Committee report refer to the huge publicity given to the UK’s plans for planting 11 million trees to sequester carbon emissions, but they warn that conserving carbon in soils is equally or more important.
The UK’s countryside charity CPRE has warned that emissions from UK peatland could cancel out all carbon decreases achieved through new and existing forests, in their August 2020 report entitled “Net-zero virtually impossible without more ambition on peatlands.”19 Similar concerns about issues caused by “the wrong trees in the wrong places” have been expressed in studies of ecosystems as far apart as Chile (Heilmayr et al., 2020) and China (Hong et al., 2020). Indeed, it has been suggested that there is firm evidence that current projections of global forest carbon sink persistence are too optimistic because the increased growth rates of trees caused by increased levels of CO2 in the atmosphere may shorten the lifespan of forest trees (Brienen et al., 2020). The overall conclusion seems to be that current plans for tree planting on a massive scale are not the panaceas that many believe. Putting such plans into effect could do more harm than good (Elgin, 2020; Friggens et al., 2020; Goswami, 2020; Heilmayr et al., 2020; Hong et al., 2020; Natural Capital Committee, 2020; and listen to the BBC World Service podcast Have we planted too much faith in trees?20
Unfortunately, present forests suffer from the effects of the climate changes that have already occurred. Forests around the world are dying due to drought, often amplified by destructive wildfires, and virulent, newly emerged, and invasive pests and diseases (Demeude and Gadault, 2020). Triggered by climate change, some invasive bark and ambrosia beetle/fungus symbioses are shifting from non-pathogenic saprotrophy in their native ranges to a prolific tree-killing in invaded ranges (Moore et al., 2020). Duffy et al. (2021) project an even more dramatic future. They estimate that the terrestrial carbon sink currently mitigates about 30% of anthropogenic carbon emissions but as global warming progresses, respiration rates will continue to rise in contrast to sharply declining rates of photosynthesis; they expect the land carbon sink to be halved by as early as 2040 under business-as-usual emissions.
China was responsible for approximately 27% of global fossil fuel emissions in 2017 and is currently the world’s single largest emitter of CO2. A pattern of rapid afforestation has been established in many regions, with provincial forest areas increasing by between 0.04 million and 0.44 million hectares per year during the past 10–15 years (Wang et al., 2020). Such a large expansion of fast-growing forest plantations is estimated to correspond to a land biosphere sink equivalent to about 45% of annual anthropogenic emissions in China over that period. Though this sound extremely encouraging, Wang et al. (2020) also state that the afforestation effort: “…contributes to timber exports and the domestic production of paper…,” which means that the carbon sequestration is only temporary because if these products are rapidly discarded, burnt, or composted, the sequestered carbon they represent will be returned to the atmosphere.
Despite these gloomy observations regarding trees and other photosynthetic organisms, there remains some hope that better management of forests and their carbon stocks can help improve overall terrestrial carbon cycle management (Soudzilovskaia et al., 2019; Domeignoz-Horta et al., 2020; Manrique and Franco, 2020) although the fact remains that we cannot rely on terrestrial vegetation to mitigate the effects of climate change for the simple reason that such a prospect expects too much of them. Even when discussing CO2 absorbed and stored in coastal and oceanic ecosystems, most people tend to consider only kelp forests, mangroves, seagrass meadows, and salt marshes or tidal marshes as potential carbon sequestering ecosystems to which the title “blue carbon” can be attached and tend to dismiss the potential of calcifying organisms. As with terrestrial forests and moorlands, conservation, restoration and general encouragement of these marine ecosystems offers many benefits, but the fundamental problem with carbon capture by all photosynthetic organisms is that it is temporary. Whether terrestrial or aquatic, photosynthetic organisms only sequester atmospheric carbon while they are alive; there is no net removal of CO2 from the atmosphere in the truly long term. When the plants die the carbonaceous plant debris is subject to aerobic decay and digestion that releases the bulk its CO2 back to the atmosphere very quickly. For example, globally, completely natural decomposition of dead wood in the world’s forests recycles billions of tons of CO2 to the atmosphere each year, similar in magnitude to the annual CO2 emissions from fossil fuel combustion (Rinne-Garmston et al., 2019). When a tree dies its entire biomass is digested within a few decades, the carbon being released back into the atmosphere as respiratory CO2 in a global total of 10.9 billion metric tons per year (Seibold et al., 2021). And when a kelp forest dies, it is digested and respired by a host of animals and microbes.
The only hope for relatively longer-term sequestration of the organic carbon produced by these “blue carbon” photosynthetic organisms is for those fragments of them that come to lie in anoxic sediments. If the anoxia can be maintained for sufficient lengths of time, and the attentions of anaerobic microbes minimized, sequestered organic carbon may be lithified (e.g., terrestrial coal measures and peat deposits), the blue organic carbon becoming the veins and inclusion fossils of the limestone layers made by calcifying organisms. Williamson and Gattuso (2022a) and Williamson and Gattuso (2022b) conclude that “Since the scale of long-term carbon removal and storage by blue carbon habitats is so uncertain, it is too risky to rely on as a means of offsetting continued emissions” (Williamson and Gattuso, 2022b).
Of all natural ecosystems, net, permanent, removal of CO2 from the atmosphere is only achieved by calcifiers which convert organic carbon into the inorganic CaCO3 stored in the shells, coccoliths, or foram tests that are left when they die. To recognize the ecosystems we should exploit to remove CO2 from the atmosphere we need to look further into Earth’s history and recognize what natural ways of controlling atmospheric greenhouse gasses the planet has employed in its past.
Biotechnological sequestration into the oceanic carbon sink: Marine calcifier organisms
In particular, we suggest that humanity should look to the oceans for a solution and properly harness the ability of marine calcifier organisms (molluscs, crustacea, corals, foraminifera and coccolithophore algae) to remove permanently CO2 from the atmosphere into solid (crystalline) CaCO3. This also has an ancient historical and entirely natural precedent (Moore et al., 2021b; Moore, 2021). At intervals over the past 500 million years the fossil record shows that the distant ancestors of today’s marine calcifiers had the physiological tools to cope with both acidified oceans and great excesses of atmospheric CO2 and still create vast remains of shells made from crystalline CaCO3. These organisms have dealt with excess atmospheric CO2 before and have a fine record for environmental engineering, while industrial humans have a track record for getting things done quickly. As we explain below, working together we could cure the atmosphere on which we all depend (Moore et al., 2022a).
Ultimately, the CO2 for the shell of shellfish and those other calcifiers comes from the atmosphere, but the shells of dead shellfish are chemically stable for geological periods of time, so effectively, this CO2 is removed from the atmosphere, permanently.
• Intact shellfish shells are excavated regularly from the middens associated with coastal communities of early humans, from around 120,000 years ago (Moore et al., 2021a; Moore et al., 2021b; Moore and Heilweck, 2022).
• Intact shellfish shells abound in deep-water cores of ancient coastal sediments of hundreds of thousands of years ago.
• And remember the fossils from deep time? Ammonites (65 to 240 million years ago), trilobites (520 million years ago), brachiopods (550 million years ago), shellfish all. Certainly, these fossil shells are changed considerably in chemistry by now (over extended time periods carbonates can recrystallize into calcite, or exchange with silica or iron sulphide in the surrounding rock matrix), but the shell carbonates survive over geological time.
The carbonates in shells are neither digested nor degraded. High temperatures are required to release the CO2 from carbonates (industrially, to produce quicklime)—ask the cement industry, which uses fossiliferous limestone as a feedstock for cement production (cement production accounts for about 8% of the fossil CO2 emissions from industrial sources).
The sedimentary limestone rocks derive all their calcium carbonate from the biological activities of bryozoa, corals, crinoids, microscopic algae, foraminifera in the plankton and/or benthos of the day, as well as shellfish shells. It is often claimed that “ocean acidification” has been shown experimentally to cause decreased shell formation in calcifiers but these have all used experimental pH levels that are not projected to be reached in the oceans until the next century or later; today’s oceans, despite recent changes, are alkaline in pH (Moore et al., 2022b). Ocean acidification is not a concern for today’s calcifiers providing we put them to the task of remediating the atmosphere without further delay. Even chemical precipitation, which is an important method by which limestones form, depends on solution of biologically produced CaCO3 as water currents agitate grains of sand and shell fragments together. Calcium carbonate is essentially insoluble in surface sea waters today, so warm, shallow waters can be saturated with CaCO3, which re-crystallises as aragonite on nuclei formed from shell fragments and builds up in concentric layers to form small multilayered spheres called ooids.
In the natural world, the carbonates of shells are only likely to release their CO2 when/if they encounter volcanic conditions. In the deep ocean, shells of dead calcareous plankton occur throughout the water column above the Calcite Compensation Depth (CCD). This is located at ocean depths of about 3,500 to 5,000 m and separates calcareous from non-calcareous sediments, with the “calcareous ooze,” which accretes into a type of limestone or chalk, being restricted roughly to the shallower half of the deep-sea floor. This is because calcium carbonate solubility increases dramatically with depth and pressure and at the depth of the CCD all calcium carbonate dissolves to form bicarbonate ions according to this equation:
Note that CO2 is taken up in this reaction and the carbonate ion (-CO3–) remains intact. If the seabed is above the CCD, bottom sediments consist of calcareous ooze. If the exposed seabed is below the CCD, CaCO3 will dissolve before reaching this depth, preventing deposition of calcareous sediment, and the sea floor sediment will be a layer of siliceous ooze or abyssal clay (Berger, 2016). The power of biogenic carbonate in planetary engineering of planet Earth is illustrated by the global paleoceanographic reorganizations of carbonate accumulation and dissolution from the Cretaceous to the Miocene (between 125 and 9 million years ago) that resulted from variations in surface ocean productivity and oceanographically driven variations in seafloor dissolution (van Andel et al., 1975; van Andel et al., 1977; Preiß-Daimler et al., 2021).
These natural fluctuations have been kept in balance over the years by the Earth’s Global Carbon Cycle that maintains a normal balance, keeping Earth’s temperature relatively stable over long periods of time. This normally operates over timescales of a few hundred thousand years, being a slow part of the overall carbon cycle. Over shorter time periods (ten thousand to a hundred thousand years) the CO2 content of the atmosphere, and consequently the temperature of Earth, can quite naturally vary and this is thought to be a contributory cause for the Earth fluctuating between ice ages and warmer interglacial periods over such time scales. The Global Carbon Cycle was almost exactly in equilibrium for several thousand years while humans were evolving and taking their long trek out of Africa. But then industrial humans intervened by burning fossil fuels, thereby returning to the atmosphere CO2 that the Earth’s natural processes had stockpiled in the rocks long before. The rapid pace of the human technological revolution has been imposed upon the slow-paced natural Carbon Cycle, causing such a dramatic increase in atmospheric CO2 in recent times that, if not corrected, could result in climate change so extreme as to be catastrophic for humanity. Adding greenhouse gases into the atmosphere results in warmer temperatures on Earth and consequential climate change.
The problem with which humanity is trying to deal at the present time is that continued increase in the amount of CO2 in the atmosphere will inevitably cause a “runaway greenhouse” effect that will generate catastrophic increase to the Earth’s surface temperatures. The consequence is today’s emphasis on decreasing emissions of CO2 This has happened before in the history of the planet and has been corrected by the Earth’s own processes. Perhaps we should look to those natural processes to find the cure for our present predicament.
We prefer to suggest as an alternative biotechnology that we employ a proven draw down process to return atmospheric carbon to the neo-fossil state. Change the focus by turning away from photosynthetic organisms (but still plant, restore and conserve them; they are significant to us in so many biological, ecological, social and cultural ways) and concentrate on marine calcifiers for really long-term carbon sequestration (Moore, 2020; Moore, 2021; Moore et al., 2022a).
How do you engineer a planet’s atmosphere when you are living on the planet?
The slick answer to the question posed in this subhead is: “very carefully.” There are two crucial principles that must be applied to the application of any potential solution to the current problems with our atmosphere, namely:
• Address the root cause of the problem, rather than the symptoms.
• Do not attempt any solution that has the potential to cause more problems.
Our suggestion is that we should apply the calcifier physiology we have just mentioned to solving our present problem with excess atmospheric CO2 by cultivating the calcifiers on a massive global scale to sequester that excess atmospheric CO2 into the ocean’s sediments. Our approach harnesses the ability of calcifying marine organisms to remove permanently CO2 from the atmosphere into solid (crystalline) CaCO3. This calcification reaction:
is often described by marine chemists as “returning CO2 to the atmosphere.” However, within overall CO2 budgets, shell production results in less CO2 in the ecosystem because one bicarbonate carbon ends up on the ocean floor as solid limestone. You will also note that Reaction 2 (calcification) is the reverse of Reaction 1 (dissolution at high hydrostatic pressure). The atmosphere is not directly involved in either reaction direction. The interpretation by today’s marine chemists (that calcification returns CO2 to the atmosphere) is neatly encapsulated in the following quotation: “Calcification is therefore a CO2-releasing process that can make water in equilibrium with the atmosphere degas, against the initial pCO2 gradient” (Gattuso et al., 1999). For several decades, this interpretation has influenced scientific advice about the biotechnological potential of calcifying organisms for permanent carbon sequestration, and, indeed, has removed them from consideration by the carbon offset markets. Our interpretation of the open water chemistry of calcification can be found in our recent preprint (Moore et al., 2022d). The number and range of reactions taking place in the atmosphere and ocean is enormous, so reactions 1 and 2 (above) only describe a minute proportion of this total and alternative schemes are inevitably simplified and bounded by approximations. However, Eqs 1, 2 (above) describe open water chemistry but because calcification is a biological process, it follows that the equilibriums that are happening in the oceans will not interlink with equilibriums happening in organisms.
It is the fundamental nature of biological systems that they carry out their processes within phospholipid membrane boundaries evolved specifically to separate the life processes from the open water environment. Biological calcification takes place on the surfaces of enzymatic polypeptides, within organelles that have phospholipid membranes, contained in a cell enclosed within another phospholipid bilayer membrane. Biological calcification is far removed from “water in equilibrium with the atmosphere.” Ignoring what is known about the biology, physiology, and molecular cell biology of living organisms, calcifiers of all types especially, leads to erroneous conclusions and deficient advice about the potential for calcifier biotechnology to contribute to atmosphere remediation (Moore et al., 2022d).
We know that some marine scientists are unconvinced that shell biomineralisation is effective in carbon sequestration, but we believe that the simplified biology indicated here (and further discussed by Moore, 2020, Moore, 2021; Moore et al., 2021a and Moore et al., 2021b; Moore et al., 2022b) demonstrate that the scientific evidence shows it is an effective carbon sink providing overall CO2 budgets in biologically natural conditions are considered, rather than individual reactions in open water conditions. This is demonstrated in the most recent life cycle assessments (LCA) of mussel, oyster and clam farming in Mediterranean waters that describe the activity as a sustainable aquaculture practice as well as a carbon sink (Tamburini et al., 2019; Tamburini et al., 2020; Turolla et al., 2020; Martini et al., 2022; Tamburini et al., 2022).
To the above we would add the observation that anyone who has ever enjoyed a meal of shellfish knows from personal experience that, at the conclusion of the meal, diners are left with a bowl of discarded shells. Consequently, IT DOESN’T MATTER which version of the marine chemistry mantra you believe (“calcification is/is not a CO2-releasing process”), it doesn’t matter that the shellfish spend their lives “exhaling” respiratory CO2 (we all do that!), it doesn’t matter that the boats burn diesel fuel to CO2 day in-day out, or that shore facilities are not carbon neutral. It doesn’t matter BECAUSE the fact is that consumption of every ton of freshly harvested shellfish leaves behind (on average) half a ton of freshly precipitated limestone in the shell. Most importantly, the shell material is 95% INORGANIC calcium carbonate which remains sequestered for millions of years (unless someone treats the shells as “food waste” and incinerates it).
We also emphasize that this CaCO3 not only sequesters atmospheric carbon but has the bio-circular economic potential for use as a sustainable biomaterial in a wide variety of different ways, and that the activity has enormous potential for sustainable aquaculture, conservation, and restitution of marine ecosystems (Moore, 2022a; Moore et al., 2022a; Moore, 2022b; Moore and Heilweck, 2022). Put simply, we advocate accelerating implementation of a historically proven natural solution to the Earth’s climate crisis by actively cultivating oceanic calcifiers—using engineering and technology to assist and guide natural systems on a path to planetary harmony.
We argue that if the level of finance and global effort that are readily foreseen for forest management (two trillion trees) and flue gas treatments (returned to the atmosphere in 10,000 years) were to be applied to expansion of shellfish and coccolithophore cultivation (Moore, 2022b) around the world, significant amounts of carbon dioxide could be permanently removed from the atmosphere within the timescale that is currently envisaged for carbon capture by afforestation. There’s money in it; Alonso et al. (2021) estimate that the CO2 sequestration potential of bivalve aquaculture, using the current value of 1 metric tonne of CO2 in the carbon market is over 25 €, which would represent a value of around 125 to 175 million € y−1 to the European Union’s bivalve aquaculture industry alone. The overwhelming advantage of our action plans (Heilweck and Moore, 2021; Petros et al., 2021) is that the excess atmospheric CO2 released by our use of fossil fuels will be returned to the place it belongs—as a present day fossil safe to the distant future. With the additional advantages of improved natural capital value (including food security), and ecosystem services (many of the organisms involved are natural habitat engineers). Further, as a nature-based solution, there is a minimum of hard infrastructure and consequently faster implementation (we could start tomorrow) and lower investment risk (many of the organisms that will sequester carbon in their shells are saleable food animals). Carbon sequestration through shellfish cultivation is much more permanent, being secured for geological periods of time, rather than for the few years or decades secured by planting trees or by industrial carbon capture and storage, both of which can only be considered as temporary solutions (Petros and Moore, 2022). So, we suggest cultivating shellfish for their shells.
A considerable proportion of shellfish biomass is represented by the shells of the animals, and shellfish shell is made by converting atmospheric CO2 into crystalline calcium carbonate which is stable for geological periods of time. Every shellfish farm that harvests 10,000 ton of fresh shellfish a year is producing approximately 5,000 t of calcium carbonate, which sequesters (permanently) 1,606 t of atmospheric CO2 (one farm, each year). Shellfish shell is chemically stable (to over 1,000°C) and not digested by any microbe, plant, or animal in the ocean.
Shellfish cultivation is an easy technology that has been practiced for millennia, and has an extensive literature (e.g., Xiang, 2015; Hai et al., 2018; Goddek et al., 2019; Lucas et al., 2019; Gökoğlu, 2021). Our goal is to enhance shellfish cultivation globally on an industrial scale with the same drive and determination that destroyed the huge oyster reefs in US and European waters in the 18th and 19th centuries (Moore et al., 2021b; Heilweck and Moore, 2021; Heilweck 2022). Implementation employing Integrated Multi-Trophic Aquaculture (IMTA) is planned to avoid the worst effects of monoculture cultivations (Heilweck and Moore, 2021; Moore et al., 2022c; Heilweck, 2022). Heilweck (2022) states: “…long lines mussel farming is by far the world’s most productive breeding method, currently yielding 60 to 70 metric tonnes of mussel flesh, per hectare per year. To put these figures into perspective, beef production is only around 0.340 tonne per hectare per year, around two hundred times less!”
Our biggest challenge, though, is to change the attitudes of today’s aquaculture industry, which is focused on this year’s food crop and trading conditions for a human food delicacy. The industry does not anticipate activity beyond this. We must change the paradigm from cultivating shellfish for food to cultivating shellfish for their shells. What we most urgently need is to have shellfish cultivation established as a carbon offsetting scheme for other people’s carbon footprints. CO2 producers (from holiday jets to heavy industry) could then fund cultivation of shell as a permanent removal from the atmosphere, with shellfish meat-protein taken as a profitable by-product. A working demonstration is probably needed to convince conservative farmers that what we advocate is a worthwhile proposition, though currently operating shellfish farms are themselves practical demonstrations at the kiloton carbon removal per year level.
Avdelas et al. (2020) provide data for mussel farms (2,720 enterprises) using four cultivation methods, across eight EU countries in 2010–2016:
• Assets value = €700,000, turnover = €384,000 (per enterprise).
• Mussels production cost = 870 € t−1.
• Farm gate value of 1 t fresh mussels = 1,080 €.
• 1 t fresh mussels = 0.5 t of shell.
• 0.5 t shell = 0.22 t atmospheric CO2, which cost 870 € to be converted to a permanent sink.
• Plus, nutritious meat sales value of 1,080 € by farms with no negative environmental impact but providing several highly beneficial ecosystem services.
More data bearing on life cycle assessments of the “shellfish for carbon sequestration” proposition have emerged in recent years. Tamburini et al. (2020) made a life cycle assessment of Manila Clam farming in the Po River Delta, Northern Italy, finding that: annual production of 1,000 kg fresh ready-to-sell clams sequestered in their shells 444.55 kg of CO2, 1.54 kg of nitrogen and 0.31 kg of phosphorus y−1.
The megaton scale of carbon sequestration is demonstrated by current global shellfish production. Globally, FAO “State of World Fisheries and Aquaculture 2020” reports that in 2018, shelled mollusks production was 17.3 million t. Scientific literature reports average contribution of shell to total bivalve body (fresh) weight varies from 70% to 95%. A reasonable guestimate is that shell represents an average 50% of the aquaculture harvest mass. So, in these FAO statistics total shellfish-shell produced globally was around 8.65 million t of shell y−1.
If the shell is assumed to be made entirely from CaCO3 then, on a molar mass basis, carbon = 12% of the mass of CaCO3, and 8.65 million t of shell per year = 1 million t of carbon per year being sequestered from the atmosphere by current world aquaculture. We estimate that this megaton scale can be doubled in a few years if shellfish cultivation is integrated into carbon offset programs. Could we increase shellfish production to a level that would achieve very significant sequestration of atmospheric CO2? We suggest so (Moore et al., 2022c; Petros and Moore, 2022). Double production of molluscs and crustaceans each year and from the 14th year we could be removing 10.7 billion t of carbon from the atmosphere annually (plus producing 90 billion t of shellfish meat). GLOBAL carbon emissions from fossil fuel use were 9.8 billion t in 2014.
Sustained annual doubling may not be realistic; but this simple calculation suggests that if we create the infrastructure and management, and have the commitment, it is doable. It has been done with terrestrial farming. Every day, the world’s terrestrial farmers produce 1 million tonnes of meat (actual global meat production figure was 341.16 million tonnes in 201821). In ten or 20 years’ time if we could say the same for aquaculture (“every day the world’s aquaculture farmers produce 1 million tonnes of shellfish meat”) we would be able to add that, by so doing, those aquaculture farmers were permanently removing 321,000 tonnes of CO2 from that future atmosphere every day (= 117 million tonnes annually). Another positive characteristic of shellfish is that they present no conflict between using land to grow food crops and using land to grow trees. Or, for that matter, between growing trees for biofuel and growing native trees to repair and re-establish natural forest ecosystems. There is not enough agricultural land on the planet to accomplish all these things.
In contrast, farming shellfish uses the shoreline and continental shelf and there is enormous scope for the shellfish sector to grow in those regions, let alone in the open sea. The Views of the World website states the total length of coastlines in the world as between 1.16 million and 1.63 million km.22 Continental shelves cover an area of about 32 million km2, which, according to the Blue Habitats website23 is only about 9% of the surface area of the world’s oceans. About 70% of the Earth’s surface is covered by water, we might as well use it to rescue the atmosphere.24
Aquaculture is far more scalable than agriculture, from subsistence-level farms to ocean-going factory ships, factory installations based on disused oil rigs on seamounts and even untended farms suspended from all wind turbines and other offshore structures (Maar et al., 2009; Stenberg et al., 2010; Blanchard, 2020; Heilweck, 2022). Further, farming shellfish for food can be combined with restoration and conservation of overfished fisheries and usually involves so little intervention (beyond provision of habitats and, where necessary, protection of larvae and juveniles from predation in “nurseries”) that there is no inevitable conflict with other activities (Moore et al., 2021b; Heilweck and Moore, 2021).
Ecosystem services of bivalve molluscs add value to their environment beyond their food and carbon sequestration values. These are listed in the literature as: ocean turbidity lessening by filtration; biodeposition of organics as algal nutrients; denitrification associated with organic deposition; provision of structural habitats (reefs) promoting diversity of fish, crustacea and other organisms; habitat and shoreline stabilization. The following advantages of shellfish farming should be added to this list: shellfish build food security; shellfish don’t require feeding; shellfish don’t require irrigation; shellfish don’t require agricultural land; bivalve welfare is not as serious a concern as it is for terrestrial farm animals. Our claims are not limited to bivalve molluscs, however; cultivation of coccolithophores (Flynn et al., 2016; Kenny and Flynn, 2017; Moore, 2022b), crustacea, corals, and molluscs (Moore et al., 2022a) on a massive scale would make a huge and continued ameliorating contribution to climate change on this planet. An important feature of what we are suggesting is that the calcifier farming exercise should be seen as a means of producing CaCO3. In which case even farms located in toxic waters, like those hit by harmful algal blooms (HABs), could be made profitable.
One of the Key Messages of the IPBES global assessment report on biodiversity and ecosystem services (Díaz et al., 2019) is that:
“Nature can be conserved, restored and used sustainably while other global societal goals are simultaneously met through urgent and concerted efforts fostering transformative change…” (Díaz et al., 2019).
We believe we have highlighted the potential of how the unique capabilities of marine calcifiers could be used to engineer this planet’s atmosphere into a less hazardous state, cultivate nutritious meats for human food and animal feeds, improve biodiversity and conservation throughout the oceans, and repair most of the damage that industrial humans have inflicted on planet Earth; potentially realizing the UN’s Sustainable Development Goal 14 (Life below water), Goal 2 (Zero hunger) together with Goal 13 (Climate action) (Petros et al., 2021; Moore et al., 2022a; Moore et al., 2022c).
Those who think mere humans could not accomplish what is needed in reasonable time, should consider the oil well story. When the first oil well was drilled in 1859, in Titusville, Pennsylvania, the locals called the operation “Drake’s Folly” and the driller “Crazy Drake,” but soon the well “could produce in a few days the same amount of oil as a whaling ship on a 4-year voyage” (https://todayinconservation.com/2018/07/august-27-first-oil-well-drilled-1859/). Now look where Crazy Drake has got us!
Data availability statement
The original contributions presented in the study are included in the article/supplementary material, further inquiries can be directed to the corresponding author.
Author contributions
DM coordinated the whole effort and composed the majority of the text. PP contributed most of the text on engineering aspects, and MH added insight into open ocean activities. All authors agree to be accountable for the content of the work.
Funding
The work was funded entirely from the personal resources of the authors. Any philanthropist who would like to contribute to the implementation of these ideas should contact the corresponding author at ZGF2aWRAZGF2aWRtb29yZS5vcmcudWs=.
Acknowledgments
We acknowledge with sincere thanks numerous discussions with Dr. Rebecca J. Moore during the preparation of this and other manuscripts.
Conflict of interest
Author PP is employed by Kääpä Biotech Oy, Finland.
The remaining authors declare that the research was conducted in the absence of any commercial or financial relationships that could be construed as a potential conflict of interest.
Publisher’s note
All claims expressed in this article are solely those of the authors and do not necessarily represent those of their affiliated organizations, or those of the publisher, the editors and the reviewers. Any product that may be evaluated in this article, or claim that may be made by its manufacturer, is not guaranteed or endorsed by the publisher.
Author disclaimer
Despite the many improvements resulting from these discussions, the opinions expressed here are those of the listed authors and are not necessarily shared by their advisors.
Footnotes
1View: https://cdm.unfccc.int/index.html
2Global Carbon Project website: https://www.globalcarbonproject.org/
3Center for Climate and Energy Solutions website: https://www.c2es.org/
4View: https://www.c2es.org/content/carbon-capture/
5View: https://www.carbfix.com/
6View: https://skydiamond.com/
7Ian Webster’s website: https://www.in2013dollars.com/us/inflation/
8View: https://sdgs.un.org/goals
9View: https://www.globalccsinstitute.com/membership/our-members/
10TheClimateQuestion-20210214-DoesBigMoneyReallyBelieveGreenIsGood.mp3: BBC World Service broadcast 15 February 2021. Download from https://www.bbc.co.uk/programmes/w3ct0xbd.
11View: https://thefishsite.com/articles/can-bivalve-aquaculture-prevent-the-widespread-institutional-failure-of-our-attempts-to-tackle-climate-change
12View: https://www.xprize.org/prizes/
13View: https://carbonupcycling.com/
14View: http://carboncorp.org/
15View: https://en.wikipedia.org/wiki/Enhanced_weathering
16View: https://thefishsite.com/articles/can-bivalve-aquaculture-prevent-the-widespread-institutional-failure-of-our-attempts-to-tackle-climate-change
17View: https://www.1t.org/
18View: https://trilliontrees.org/
19View: https://www.cpre.org.uk/about-us/cpre-media/net-zero-for-land-virtually-impossible-without-more-ambition-on-peatlands/
20BBC World Service podcast [TheClimateQuestion-20210221-HaveWePlantedTooMuchFaithInTrees.mp3] broadcast 22 February 2021. Download from https://www.bbc.co.uk/programmes/w3ct0xbf
21View: https://ourworldindata.org/meat-production#global-meat-production
22View: http://www.viewsoftheworld.net/?p=5340
23View: https://www.bluehabitats.org/?page_id=1660
24View: https://thefishsite.com/articles/can-bivalve-aquaculture-prevent-the-widespread-institutional-failure-of-our-attempts-to-tackle-climate-change
References
Alonso, A. A., Álvarez-Salgado, X. A., and Antelo, L. T. (2021). Assessing the impact of bivalve aquaculture on the carbon circular economy. J. Clean. Prod. 279, 123873. doi:10.1016/j.jclepro.2020.123873
Aronoff, K. (2020). Carbon capture is not a climate savior. The New Republic Website. Available at: https://newrepublic.com/. (Accessed October 17, 2021)..
Avdelas, L., Avdic-Mravlje, E., Borges Marques, A. C., Cano, S., Capelle, J. J., Carvalho, N., et al. (2020). The decline of mussel aquaculture in the European union: Causes, economic impacts and opportunities. Rev. Aquac. 13, 91–118. doi:10.1111/raq.12465
Bastin, J.-F., Finegold, Y., Garcia, C., Mollicone, D., Rezende, M., Routh, D., et al. (2019). The global tree restoration potential. Science 365, 76–79. doi:10.1126/science.aax0848
Beerling, D. J., Kantzas, E. P., Lomas, M. R., Wade, P., Eufrasio, R. M., Renforth, P., et al. (2020). Potential for largescale CO2 removal via enhanced rock weathering with croplands. Nature 583, 242–248. doi:10.1038/s41586-020-2448-9
Bellamy, R., Lezaun, J., and Palmer, J. (2019). Perceptions of bioenergy with carbon capture and storage in different policy scenarios. Nat. Commun. 10, 743. doi:10.1038/s41467-019-08592-5
Berger, W. H. (2016). “Calcite compensation depth (CCD),” in Encyclopedia of marine geosciences. Editors J. Harff, M. Meschede, S. Petersen, and J. Thiede (Dordrecht: Springer Nature), 71–73. Encyclopedia of Earth Sciences Series. doi:10.1007/978-94-007-6238-1
Beuttler, C., Charles, L., and Wurzbacher, J. (2019). The role of Direct Air Capture in mitigation of anthropogenic greenhouse gas emissions. Front. Clim. 1, 10. doi:10.3389/fclim.2019.00010
Blanchard, S. (2020). Colocation of aquaculture and wind farm in Liverpool Bay: Effective incorporation of finfish aquaculture to the Gwynt y Mor wind farm. Chisinau, Moldova: LAP Lambert Academic Publishing, 112. Available at: https://tinyurl.com/2msjf8ff.
Boysen, L. R., Lucht, W., Gerten, D., Heck, V., Lenton, T. M., and Schellnhuber, H. J. (2017). The limits to global-warming mitigation by terrestrial carbon removal. Earth's. Future 5, 463–474. doi:10.1002/2016EF000469
Brienen, R. J. W., Caldwell, L., Duchesne, L., Voelker, S., Barichivich, J., Baliva, M., et al. (2020). Forest carbon sink neutralized by pervasive growth-lifespan trade-offs. Nat. Commun. 11, 4241. doi:10.1038/s41467-020-17966-z
Budinis, S. (2020). Going carbon negative: What are the technology options? Paris: International Energy Agency. IEA Direct Air Capture Tracking report. Available at: https://www.iea.org/reports/direct-air-capturehttps://tinyurl.com/2khsp36j.
Clarke, L., Böhringer, C., and Rutherford, T. F. (Editors) (2009). “International, U.S., and E.U. climate change control scenarios: Results from EMF 22,” Energy economics. (Amsterdam), 31,2 S63–S305. Available at: https://tinyurl.com/yynrdr8a.
Consoli, C. (2019). Bioenergy and carbon capture and storage. Washington, DC, USA: The Global CCS Institute. 14 pp. Available at: https://tinyurl.com/2834jtv6.
Crowther, T. W., Glick, H. B., Covey, K. R., Bettigole, C., Maynard, D. S., Thomas, S. M., et al. (2015). Mapping tree density at a global scale. Nature 525, 201–205. doi:10.1038/nature14967
Cui, R. Y., Hultman, N., Edwards, M. R., He, L., Sen, A., Surana, K., et al. (2019). Quantifying operational lifetimes for coal power plants under the Paris goals. Nat. Commun. 10, 4759. doi:10.1038/s41467-019-12618-3
Cziesielski, M. J., Duarte Carlos, M., Aalismail, N., Al-Hafedh, Y., Anton, A., Baalkhuyur, F., et al. (2021). Investing in blue natural capital to secure a future for the Red Sea ecosystems. Front. Mar. Sci. 7, 603722. doi:10.3389/fmars.2020.603722
De Jong, W., Pokorny, B., Katila, P., Galloway, G., and Pacheco, P. (2018). Community forestry and the sustainable development goals: A two way street. Forests 9, 331. doi:10.3390/f9060331
Demeude, H., and Gadault, T. (2020). Le cri de détresse des forêts françaises confrontées aux crises écologiques. Website of The GoodPlanet Info magazine. Available at: https://tinyurl.com/yrufh2vr. (Accessed October 17, 2021).
Deng, H., Bielicki, J. M., Oppenheimer, M., Fitts, J. P., and Peters, C. A. (2017). Leakage risks of geologic CO2 storage and the impacts on the global energy system and climate change mitigation. Clim. Change 144, 151–163. doi:10.1007/s10584-017-2035-8
Díaz, S., Settele, J., Brondízio, E. S., Ngo, H. T., Guèze, M., Agard, J., et al. (eds). (2019). The global assessment report on biodiversity and ecosystem services. Summary for policymakers. 56 pp. Bonn: Germany by the Secretariat of the Intergovernmental Science-Policy Platform on Biodiversity and Ecosystem Services IPBES. Available at: https://tinyurl.com/75w5pww8.
Domeignoz-Horta, L. A., Pold, G., Liu, X.-J. A., Frey, S. D., Melillo, J. M., and DeAngelis, K. M. (2020). Microbial diversity drives carbon use efficiency in a model soil. Nat. Commun. 11, 3684. doi:10.1038/s41467-020-17502-z
Duffy, K. A., Schwalm, C. R., Arcus, V. L., Koch, G. W., Liang, L. L., and Schipper, L. A. (2021). How close are we to the temperature tipping point of the terrestrial biosphere? Sci. Adv. 7, eaay1052. doi:10.1126/sciadv.aay1052
Elgin, B. (2020). These trees are not what they seem. How the Nature Conservancy, the world’s biggest environmental group, became a dealer of meaningless carbon offsets. Blog on the Bloomberg Green website. Available at: https://www.bloomberg.com. (Accessed October 17, 2021)
FAO (2018). The State of the World’s Forests 2018 - forest pathways to sustainable development. Rome: Food and Agriculture Organization of the United Nations. 139 pp. Available at: http://www.fao.org/3/I9535EN/i9535en.pdf.
Flynn, K. J., Clark, D. R., and Wheeler, G. (2016). The role of coccolithophore calcification in bioengineering their environment. Proc. R. Soc. B 283, 20161099. doi:10.1098/rspb.2016.1099
Friedmann, S. J., Zapantis, A., Page, B., Consoli, C., Fan, Z., Havercroft, I., et al. (2020). Net-zero and geospheric return: Actions today for 2030 and beyond. Published by The Global CCS Institute and The Center on Global Energy Policy of Columbia University, USA. 55 pp. Available at: https://tinyurl.com/46u79y9j.
Friggens, N. L., Hester, A. J., Mitchell, R. J., Parker, T. C., Subke, J.-A., and Wookey, P. A. (2020). Tree planting in organic soils does not result in net carbon sequestration on decadal timescales. Glob. Chang. Biol. 26, 5178–5188. doi:10.1111/gcb.15229
Gattuso, J.-P., Frankignoulle, M., and Smith, S. V. (1999). Measurement of community metabolism and significance of coral reefs in the CO2 source-sink debate. Proc. Natl. Acad. Sci. U. S. A. 96, 13017–13022. doi:10.1073/pnas.96.23.13017
Girardin, C. A. J., Jenkins, S., Seddon, N., Allen, M., Lewis, S. L., Wheeler, C. E., et al. (2021). Nature-based solutions can help cool the planet - if we act now. Nature 593, 191–194. doi:10.1038/d41586-021-01241-2
Goddek, S., Joyce, A., Kotzen, B., and Burnell, G. M. (2019). Aquaponics food production systems: Combined aquaculture and hydroponic production technologies for the future. Switzerland: Springer Nature. Available at: https://link.springer.com/book/10.1007/978-3-030-15943-6.
Gökoğlu, N. (2021). Shellfish processing and preservation. Switzerland: Springer Nature, 334. Available at: https://link.springer.com/book/10.1007/978-3-030-60303-8.
Goswami, A. (2020). Relying on forests to achieve net zero targets not a good idea, and scientists agree. Blog on the DownToEarth website. Available at: https://www.downtoearth.org.in/. (Accessed October 17, 2021).
Griscom, B. W., Adams, J., Ellis, P. W., Houghton, R. A., Lomax, G., Miteva, D. A., et al. (2017). Natural climate solutions. Proc. Natl. Acad. Sci. U. S. A. 114, 11645–11650. doi:10.1073/pnas.1710465114
Hai, F. I., Visvanathan, C., and Boopathy, R. (2018). Sustainable aquaculture (applied environmental science and engineering for a sustainable future). Switzerland: Springer Nature, 335. Available at: https://link.springer.com/book/10.1007/978-3-319-73257-2.
Hannah, M. (2021). Extinctions: Living and dying in the margin of error. Cambridge, UK: Cambridge University Press, 263.
Heilmayr, R., Echeverría, C., and Lambin, E. F. (2020). Impacts of Chilean forest subsidies on forest cover, carbon and biodiversity. Nat. Sustain. 3, 701–709. doi:10.1038/s41893-020-0547-0
Heilweck, M., and Moore, D. (2021). Saving the planet with appropriate biotechnology: 3. The high seas solution. Mex. J. Biotech. 6, 92–128. doi:10.29267/mxjb.2021.6.1.92
Heilweck, M. (2022). “The high seas solution,” in Aquaculture: Ocean blue carbon meets UN-SDGS. Editors D. Moore, M. Heilweck, and P. Petros (Cham: Springer), 97–130. Chapter 4. A volume in the Sustainable Development Goals Series. doi:10.1007/978-3-030-94846-7_4
Hills, C. D., Tripathi, N., and Carey, P. J. (2020). Mineralization technology for carbon capture, utilization, and storage. Front. Energy Res. 8, 142. doi:10.3389/fenrg.2020.00142
Ho, T. H. M., and Tsai, P. A. (2020). Microfluidic salt precipitation: Implications for geological CO2 storage. Lab. Chip 20, 3806–3814. doi:10.1039/D0LC00238K
Hong, S., Yin, G., Piao, S., Dybzinski, R., Cong, N., Li, X., et al. (2020). Divergent responses of soil organic carbon to afforestation. Nat. Sustain. 3, 694–700. doi:10.1038/s41893-020-0557-y
Hugelius, G., Loisel, J., Chadburn, S., Jackson, R. B., Jones, M., MacDonald, G., et al. (2020). Large stocks of peatland carbon and nitrogen are vulnerable to permafrost thaw. Proc. Natl. Acad. Sci. U. S. A. 117, 20438–20446. doi:10.1073/pnas.1916387117
IEA (2010). Energy technology perspectives 2010. Paris: OECD/IEA. Available at: https://www.iea.org/reports/energy-technology-perspectives-2010.
IOGP (2019). The potential for CCS and CCU in Europe. IOGP, 47. Report to the thirty second meeting of the European Gas Regulatory Forum, Coordinated by the International Association of Oil and Gas Producers (IOGP). Available at: https://ec.europa.eu/info/sites/info/files/iogp_-_report_-_ccs_ccu.pdf.
Irlam, L. (2017). Global costs of carbon capture and storage. An update published by The Global CCS Institute. Available at: https://tinyurl.com/2p8cn877.
Jacobson, H. K. (2001). “Climate policy: International,” in International encyclopedia of the social and behavioral sciences. Editors N. J. Smelser, and P. B. Baltes. (Elsevier, Amsterdam), 2011–2016. doi:10.1016/B0-08-043076-7/04487-9
Jones, I. S. F. (2014). The cost of carbon management using ocean nourishment. Int. J. Clim. Chang. Strateg. Manag. 6, 391–400. doi:10.1108/IJCCSM-11-2012-0063
Kenny, P., and Flynn, K. J. (2017). Physiology limits commercially viable photoautotrophic production of microalgal biofuels. J. Appl. Phycol. 29, 2713–2727. doi:10.1007/s10811-017-1214-3
Kheshgi, H., de Coninck, H., and Kessels, J. (2012). Carbon dioxide capture and storage: Seven years after the IPCC special report. Mitig. Adapt. Strateg. Glob. Change 17, 563–567. doi:10.1007/s11027-012-9391-5
Krüger, T. (2017). Conflicts over carbon capture and storage in international climate governance. Energy Policy 100, 58–67. doi:10.1016/j.enpol.2016.09.059
Kuwae, T., and Hori, M. (2019). “The future of blue carbon: Addressing global environmental issues,” in Blue carbon in shallow coastal ecosystems: Carbon dynamics, policy, and implementation. Editors T. Kuwae, and M. Hori (Springer Nature Singapore Pte Ltd), 347–373. Chapter 13. doi:10.1007/978-981-13-1295-3_13
Larkin, P., Gracie, R., Shafiei, A., Dusseault, M., Sarkarfarshi, M., Aspinall, W., et al. (2019). Uncertainty in risk issues for carbon capture and geological storage: Findings from a structured expert elicitation. Int. J. Risk Assess. Manag. 22, 429–463. doi:10.1504/IJRAM.2019.103335
Li, A., Wang, J., and Bao, B. (2019). High-efficiency CO2 capture and separation based on hydrate technology: A review. Greenh. Gas. Sci. Technol. 9, 175–193. doi:10.1002/ghg.1861
Liu, L.-C., Li, Q., Zhang, J.-T., and Cao, D. (2016). Toward a framework of environmental risk management for CO2 geological storage in China: Gaps and suggestions for future regulations. Mitig. Adapt. Strateg. Glob. Change 21, 191–207. doi:10.1007/s11027-014-9589-9
Lucas, J. S., Southgate, P. C., and Tucker, C. S. (Editors) (2019). Aquaculture: Farming aquatic animals and plants. 3rd edition (Hoboken, NJ, USA: Wiley-Blackwell), 666. Available at: https://tinyurl.com/3emffyku.
Maar, M., Bolding, K., Petersen, J. K., Hansen, J. L. S., and Timmermann, K. (2009). Local effects of blue mussels around turbine foundations in an ecosystem model of Nysted off-shore wind farm, Denmark. J. Sea Res. 62, 159–174. doi:10.1016/j.seares.2009.01.008
Mace, M. J., Fyson, C. L., Schaeffer, M., and Hare, W. L. (2021). Governing large-scale carbon dioxide removal: Are we ready? - an update. New York, USA: Carnegie Climate Governance Initiative C2G. Available at: https://climateanalytics.org/media/c2g2-2018-cdr-governance-1_1_1.pdf.
Manrique, S. M., and Franco, J. (2020). Tree cover increase mitigation strategy: Implications of the “replacement approach” in carbon storage of a subtropical ecosystem. Mitig. Adapt. Strateg. Glob. Chang. 25 (8), 1481–1508. doi:10.1007/s11027-020-09930-5
Marino, B. D. V., and Bautista, N. (2022). Commercial forest carbon protocol over-credit bias delimited by zero-threshold carbon accounting. Trees, For. People 7, 100171. doi:10.1016/j.tfp.2021.100171
Martini, A., Calì, M., Capoccioni, F., Martinoli, M., Pulcini, D., Buttazzoni, L., et al. (2022). Environmental performance and shell formation-related carbon flows for mussel farming systems. Sci. Total Environ. 831, 154891. doi:10.1016/j.scitotenv.2022.154891
Masson-Delmotte, V., Zhai, P., Pörtner, H.-O., Roberts, D., Skea, J., and Shukla, P. R. Editors (2019). “IPCC, 2018: Global warming of 1.5°C,” An IPCC Special Report on the impacts of global warming of 1.5°C above pre-industrial levels and related global greenhouse gas emission pathways, in the context of strengthening the global response to the threat of climate change, sustainable development, and efforts to eradicate poverty. New York, USA. Open access at this Available at: https://www.ipcc.ch/sr15/and for PDF download https://www.ipcc.ch/sr15/download/.
Meadowcroft, J., and Langhelle, O. (Editors) (2011). Caching the carbon: The politics and policy of carbon capture and storage (Cheltenham, UK: Edward Elgar Publishing Ltd.), 320.
Metz, B., Davidson, O., de Coninck, H., Loos, M., and Meyer, L. (Editors) (2005). “Carbon dioxide capture and storage,” in Special report prepared by working group III of the intergovernmental panel on climate change. (Cambridge, UK: Cambridge University Press), 431. Available at: https://www.ipcc.ch/.
Miller, R. W., and Gardiner, D. T. (2003). Soils in our environment. 10th edition. Upper Saddle River, NJ: Pearson/Prentice Hall Publishers.
Miocic, J. M., Gilfillan, S. M. V., Frank, N., Schroeder-Ritzrau, A., Burnside, N. M., and Haszeldine, R. S. (2019). 420, 000 year assessment of fault leakage rates shows geological carbon storage is secure. Sci. Rep. 9, 769–9. doi:10.1038/s41598-018-36974-0
Moore, D. (2020). A biotechnological expansion of shellfish cultivation could permanently remove carbon dioxide from the atmosphere. Mex. J. Biotech. 5, 1–10. doi:10.29267/mxjb.2020.5.1.1
Moore, D. (2021). Saving the planet with appropriate biotechnology: 4. Coccolithophore cultivation and deployment. Mex. J. Biotech. 6, 129–155. doi:10.29267/mxjb.2021.6.1.129
Moore, D. (2022a). “Farming giant clams in 2021: A great future for the ‘blue economy’ of tropical islands,” in Aquaculture: Ocean blue carbon meets UN-SDGS. Editors D. Moore, M. Heilweck, and P. Petros (Cham: Springer), 131–153. Chapter 5. A volume in the Sustainable Development Goals Series. doi:10.1007/978-3-030-94846-7_5
Moore, D. (2022b). “Coccolithophore cultivation and deployment,” in Aquaculture: Ocean blue carbon meets UN-SDGS. Editors D. Moore, M. Heilweck, and P. Petros (Cham: Springer), 155–176. Chapter 6. A volume in the Sustainable Development Goals Series. doi:10.1007/978-3-030-94846-7_6
Moore, D., and Heilweck, M. (2022). “Aquaculture: Prehistoric to traditional to modern,” in Aquaculture: Ocean blue carbon meets UN-SDGS. Editors D. Moore, M. Heilweck, and P. Petros (Cham: Springer), 65–95. Chapter 3. A volume in the Sustainable Development Goals Series. doi:10.1007/978-3-030-94846-7_3
Moore, D., Heilweck, M., Fears, W. B., Petros, P., Squires, S. J., Tamburini, E., et al. (2022d). An assessment of the potential value for climate remediation of ocean calcifiers in sequestration of atmospheric carbon. Berlin. ScienceOpen Preprints (Climate Change: Open Access collection). doi:10.14293/S2199-1006.1.SOR-.PPGSZEY.v2
Moore, D., Heilweck, M., and Petros, P. (2021a). Saving the planet with appropriate biotechnology: 1. Diagnosing the problems. Mex. J. Biotech. 6, 1–30. doi:10.29267/mxjb.2021.6.1.1
Moore, D., Heilweck, M., and Petros, P. (2021b). Saving the planet with appropriate biotechnology: 2. Cultivate shellfish to remediate the atmosphere. Mex. J. Biotech. 6, 31–91. doi:10.29267/mxjb.2021.6.1.31
Moore, D., Heilweck, M., and Petros, P. (2022a). Aquaculture: Ocean blue carbon meets UN-SDGs. Cham, Switzerland: Springer Nature Switzerland AG, 253. A volume in the series Sustainable Development Goals. Available at: https://link.springer.com/book/10.1007/978-3-030-94846-7.
Moore, D., Heilweck, M., and Petros, P. (2022b). “Cultivate shellfish to remediate the atmosphere,” in Aquaculture: Ocean blue carbon meets UN-SDGS. Editors D. Moore, M. Heilweck, and P. Petros (Cham: Springer), 35–63. Chapter 2. A volume in the Sustainable Development Goals Series. doi:10.1007/978-3-030-94846-7_2
Moore, D., Heilweck, M., and Petros, P. (2022c). “What should Be done,” in Aquaculture: Ocean blue carbon meets UN-SDGS. Editors D. Moore, M. Heilweck, and P. Petros (Cham: Springer), 217–242. Chapter 4. A volume in the Sustainable Development Goals Series. doi:10.1007/978-3-030-94846-7_8
Moore, D., Robson, G. D., and Trinci, A. P. J. (2020). 21st century guidebook to fungi. Second Edition. Cambridge, UK: Cambridge University Press.
NASEM (2019). Negative emissions technologies and reliable sequestration: A research agenda. Washington, DC: National Academies of Sciences, Engineering, and Medicine of the United States of AmericaThe National Academies Press. Open access. 10.17226/25259.
Natural Capital Committee (2020). Natural capital committee advice on reaching net zero by 2050: Nature based interventions. Open access Available at: https://tinyurl.com/4ch4hwc4. (Accessed October 17, 2021).
Natural England (2010). England’s peatlands: Carbon storage and greenhouse gases. York, England. Report NE257. Open access and PDF download at http://publications.naturalengland.org.uk/publication/30021.
ONS (2016). UK Natural Capital: Experimental carbon stock accounts, preliminary estimates. Available at: https://tinyurl.com/5xfbazrt. (Accessed October 17, 2021)..
Pearse, R., and Böhm, S. (2014). Ten reasons why carbon markets will not bring about radical emissions reduction. Carbon Manag. 5, 325–337. doi:10.1080/17583004.2014.990679
Petros, P., Heilweck, M., and Moore, D. (2021). Saving the planet with appropriate biotechnology: 5. An action plan. Mex. J. Biotech. 6, 1–60. doi:10.29267/mxjb.2021.6.2.1
Petros, P., and Moore, D. (2022). “Comparing industrial and biotechnological solutions for carbon capture and storage,” in Aquaculture: Ocean blue carbon meets UN-SDGS. Editors D. Moore, M. Heilweck, and P. Petros (Cham: Springer), 177–216. Chapter 7. A volume in the Sustainable Development Goals Series. doi:10.1007/978-3-030-94846-7_7
Preiß-Daimler, I., Zarkogiannis, S. D., Kontakiotis, G., Henrich, R., and Antonarakou, A. (2021). Paleoceanographic perturbations and the marine carbonate system during the middle to late Miocene carbonate crash – A critical review. Geosciences 2021, 94. doi:10.3390/geosciences11020094
Rinne-Garmston (Rinne), K. T., Peltoniemi, K., Chen, J., Peltoniemi, M., Fritze, H., Peltoniemi, M., et al. (2019). Carbon flux from decomposing wood and its dependency on temperature, wood N2 fixation rate, moisture and fungal composition in a Norway spruce forest. Glob. Change Biol. 25, 1852–1867. doi:10.1111/gcb.14594
Romano, M. C., Anantharaman, R., Arasto, A., Ozcan, D. C., Ahn, H., Dijkstra, J. W., et al. (2013). Application of advanced technologies for CO2 capture from industrial sources. Energy Procedia 37, 7176–7185. doi:10.1016/j.egypro.2013.06.655
Secretariat of the Convention on Biological Diversity (2009). Scientific synthesis of the impacts of ocean fertilization on marine biodiversity. 53 pp. Published by The Secretariat of the Convention on Biological Diversity: Montreal, Canada. CBD Technical Series No. 45. Available at: https://www.cbd.int/doc/publications/cbd-ts-45-en.pdf.
Seibold, S., Rammer, W., Hothorn, T., Seidl, R., Ulyshen, M. D., Lorz, J., et al. (2021). The contribution of insects to global forest deadwood decomposition. Nature 597, 77–81. doi:10.1038/s41586-021-03740-8
Smith, P., Adams, J., Beerling, D. J., Beringer, T., Calvin, K. V., Fuss, S., et al. (2019). Land-management options for greenhouse gas removal and their impacts on ecosystem services and the sustainable development goals. Annu. Rev. Environ. Resour. 44, 255–286. doi:10.1146/annurev-environ-101718-033129
Soudzilovskaia, N. A., van Bodegom, P. M., Terrer, C., Zelfde, M. V., McCallum, I., McCormack, M. L., et al. (2019). Global mycorrhizal plant distribution linked to terrestrial carbon stocks. Nat. Commun. 10, 5077. doi:10.1038/s41467-019-13019-2
Stenberg, C., Christoffersen, M., Mariani, P., Krog, C., Dolmer, P., Maar, M., et al. (2010). Offshore wind farms and their potential for shellfish aquaculture and restocking. France: National Institute of Aquatic Resources, ICES Council Meeting Nantes. Available at: https://www.ices.dk/sites/pub/CM%20Doccuments/CM-2010/O/O1210.pdf.
Tamburini, E., Fano, E. A., Castaldelli, G., and Turolla, E. (2019). Life cycle assessment of oyster farming in the Po Delta, northern Italy. Resources 8, 170. doi:10.3390/resources8040170
Tamburini, E., Turolla, E., Fano, E. A., and Castaldelli, G. (2020). Sustainability of Mussel (Mytilus galloprovincialis) farming in the Po River Delta, Northern Italy, based on a life cycle assessment approach. Sustainability 12, 3814. doi:10.3390/su12093814
Tamburini, E., Turolla, E., Lanzoni, M., Moore, D., and Castaldelli, G. (2022). Manila clam and mediterranean mussel aquaculture is sustainable and a net carbon sink. Sci. Total Environ. 848, 157508. doi:10.1016/j.scitotenv.2022.157508
Turolla, E., Castaldelli, G., Fano, E. A., and Tamburini, E. (2020). Life Cycle Assessment (LCA) proves that Manila Clam farming (Ruditapes philippinarum) is a fully sustainable aquaculture practice and a carbon sink. Sustainability 12, 5252. doi:10.3390/su12135252
UN (1992). The united nations framework convention on climate change. New York, USA. Report FCCC/INFORMAL/84 GE.05-62220 (E) 200705. Available for download: http://unfccc.int/resource/docs/convkp/conveng.pdf.
UNDP Thailand (2019). Blue Carbon Society and UNDP team up on a ‘masterplan’ for Phetchaburi’s mangroves and its coastal ecosystems. Thailand: The United Nations Development Programme. Available at: https://www.th.undp.org/.
van Andel, T. H., Thiede, J., Sclater, J. G., and Hay, W. W. (1977). Depositional history of the South Atlantic Ocean during the last 125 million years. J. Geol. 85, 651–698. doi:10.1086/628357
van Andel, T. H., Heath, G. R., and Moore, T. C. (1975). Cenozoic history and paleoceanography of the central equatorial Pacific Ocean. Mem. Geol. Soc. Am. 143, 1–223. doi:10.1130/MEM143-p1
Wang, J., Feng, L., Palmer, P. I., Liu, Y., Fang, S., Bösch, H., et al. (2020). Large Chinese land carbon sink estimated from atmospheric carbon dioxide data. Nature 586, 720–723. doi:10.1038/s41586-020-2849-9
Williamson, P., and Gattuso, J.-P. (2022a). Carbon removal using coastal blue carbon ecosystems is uncertain and unreliable, with questionable climatic cost-effectiveness. Front. Clim. 4, 853666. doi:10.3389/fclim.2022.853666
Williamson, P., and Gattuso, J.-P. (2022b). Climate change: Why we can’t rely on regrowing coastal habitats to offset carbon emissions. Online article on The Conversation website. Available at: https://tinyurl.com/ks2te9ub. (Accessed August 10, 2022).
WMO (2020). State of the global climate 2020: Provisional report. Geneva: The World Meteorological Organization. 38 pp. Available at: https://tinyurl.com/yjhrvhnf.
Xiang, J. (2015). Recent major advances of biotechnology and sustainable aquaculture in China. Curr. Biotechnol. 4, 296–310. doi:10.2174/2211550105666151105190012
Zahnle, K., Schaefer, L., and Fegley, B. (2010). Earth’s earliest atmospheres. Cold Spring Harb. Perspect. Biol. 2, a004895. doi:10.1101/cshperspect.a004895
Zapantis, A. (2017). Benefits of CCS presented at significant conferences. Global CCS Institute Insight website news item. Available at: https://www.globalccsinstitute.com/news-media/insights/. (Accessed October 17, 2021)..
Keywords: climate, atmosphere, carbon, bioengineering, nature-based, blue-carbon, calcifiers
Citation: Moore D, Heilweck M and Petros P (2022) Planetary bioengineering on Earth to return and maintain the atmospheric carbon dioxide to pre-industrial levels: Assessing potential mechanisms. Front. Astron. Space Sci. 9:797146. doi: 10.3389/fspas.2022.797146
Received: 18 October 2021; Accepted: 24 August 2022;
Published: 15 September 2022.
Edited by:
Zhonghua Yao, Institute of Geology and Geophysics (CAS), ChinaReviewed by:
Kevin John Flynn, Plymouth Marine Laboratory, United KingdomAnirban Akhand, Hong Kong University of Science and Technology, Hong Kong SAR, China
Copyright © 2022 Moore, Heilweck and Petros. This is an open-access article distributed under the terms of the Creative Commons Attribution License (CC BY). The use, distribution or reproduction in other forums is permitted, provided the original author(s) and the copyright owner(s) are credited and that the original publication in this journal is cited, in accordance with accepted academic practice. No use, distribution or reproduction is permitted which does not comply with these terms.
*Correspondence: David Moore, ZGF2aWRAZGF2aWRtb29yZS5vcmcudWs=
†These authors have contributed equally to this work