- School of Physics and Astronomy, University of Leicester, Leicester, United Kingdom
The near three decades of continuous Mars’ exploration has opened the door to the understanding of the Martian space environment, which includes the solar wind, magnetosphere, ionosphere and atmosphere, and is a complex structure with simultaneous downward and upward couplings. However, we do not yet understand many of the physical processes that drive matter and energy flow between these couplings and within the various atmospheric reservoirs (including temporal and spatial changes on short time scales). Although each coupling plays an essential role for the system, understanding the fate of the ionosphere, as a natural sink of both internal (i.e., atmospheric cycles) and external (i.e., solar wind) energy inputs, is the key for a successful future systematic exploration of Mars.
1 Introduction
The Martian space environment, which includes the solar wind, magnetosphere, ionosphere and atmosphere, is a complex system with simultaneous downward and upward couplings (e.g., Lillis et al., 2021; Sanchez-Cano et al., 2022), all of which induce large dynamics in the entire system. In the absence of a global intrinsic field at Mars (Acuna, 1999), the solar wind interaction with the planet mainly occurs at high altitude with its upper atmosphere, where the interplanetary magnetic field bends about the planet inducing a magnetosphere (e.g., Vaisberg et al., 2018). The ultimate region where the energy of the solar wind is dissipated is the ionosphere, which is the solar photoionized part of the thermosphere (upper atmosphere) at ∼100–500 km altitude. As an ionized medium, the ionosphere is sensitive to electrodynamics and magnetic fields. As a reactive medium, the ionosphere strongly interacts with the chemistry of the neutral atmosphere. This is known as the magnetosphere-ionosphere-thermosphere (M-I-T) coupled system. The solar wind is the major energy input to the M-I-T system at any of the terrestrial planets, and therefore, the major source of upper atmospheric variability. Thus, understanding how the M-I-T system evolve along the long-term variation of the solar wind with the solar cycle of activity (typically ∼11 years) is an essential factor that determines the background variability of the space environment of each planet, and in particular for Mars (e.g., Sanchez-Cano et al., 2022).
A particular feature of Mars is that the ionosphere-solar wind interaction is more complex over a region of the southern hemisphere where highly non-uniform crustal magnetic fields are located. These fields, which are of the order of a few tends to hundreds nT at ∼400 km (e.g., Langlais et al., 2019), can interact directly with the solar wind producing a “hybrid magnetosphere”, i.e., with features of both induced and intrinsic magnetospheres, which affect the whole planet because it changes as the crustal magnetic fields rotate with the planet (Fang et al., 2015). This is a unique aspect of Mars in our Solar System and means that parts of the Martian M-I-T system may behave differently and in shorter time-scales than expected. Moreover, these crustal fields play an important role in guiding plasma motion, producing hemispheric asymmetry in the magnetosphere, ionosphere, and ion escape (Vaisberg et al., 2018).
Although each region of the M-I-T coupling plays an essential role for the system, the continued exploration of Mars for almost three decades has shown that the ionosphere is a natural sink of both internal and external energy inputs. For example, as internal energy sources, the ionosphere is seasonally affected by lower atmospheric cycles such as the water or CO2 cycles during spring (e.g., Sánchez-Cano et al., 2018), as well as by regional and even sometimes global dust storms (e.g., Fang et al., 2015; Montabone, 2015), and gravity waves (e.g., England et al., 2017). As an external energy source, solar wind energetic particles precipitate into the ionosphere and ionize the middle atmosphere (a region typically not ionized) causing absorption of signals and, therefore, major radio propagation issues (e.g., Sánchez-Cano et al., 2015; Lester et al., 2022), as well as a myriad of different types of auroras (Schneider et al., 2015; Schneider et al., 2021), and gives us an estimation of the level of shielding of the surface from harmful radiations (Guo et al., 2017). Moreover, the presence of different magnetic field features within the ionosphere (either from crustal fields or from the solar wind) significantly changes the thermal balance of the ionosphere, which becomes magnetized, and ion distributions critically depend on the balance of both the thermal and magnetic pressures (e.g., Sanchez-Cano et al., 2020). All these processes are entangled between several regions of the system. Understanding their temporal and spatial variability in order to assess the differences of the processes that control the long-term dynamics of an ionosphere, including the role of the electrodynamics induced by the solar wind at Mars along the solar cycle and the motion and dynamics of the neutral atmosphere, which in turn can also create currents in the ionosphere (Riousset et al., 2014; Collinson et al., 2019), is a critical aspect for exploration of the red planet that we must resolve (Lillis et al., 2021; Sanchez-Cano et al., 2022).
The ionosphere of Mars is the layer of the upper atmosphere that plays a critical role in balancing the energy of the Martian system by coupling the neutral atmosphere with space, and can be considered as a tracer for atmospheric dynamics and also, for the solar wind-lower atmosphere coupling. The future of Mars exploration, either manned or robotic, will critically depend on a good understanding of ionospheric variability, thus the M-I-T coupling, as it determines the properties of the near-planet environment, and in turn its habitability.
2 Our gained knowledge of Mars’ ionosphere thanks to the continuous exploration of Mars
For the last almost 3 decades there has always been an active mission at Mars taking different types of ionospheric observations. This is illustrated in Figure 1, where the lifetime of different missions as well as planned and proposed missions at Mars with respect to the latest solar cycles is plotted. Before the arrival of Mars Global Surveyor (MGS) at Mars, the knowledge of Mars ionosphere was very limited and only basic information was gathered thanks to a few flybys and short-life missions. A critical discovery by MGS was the presence of crustal magnetic fields at Mars (Acuna, 1999) that changed the entire vision that we had of the planet, as well as it showed for the first time the effect of non-migrating waves in the ionosphere, a clear sign of coupling between the lower and upper atmospheres (Bougher, 2001). This, together with its long duration covering the maximum and moderate levels of solar activity in solar cycle 23 opened a new door for planetary plasma physics research. Since then, the continued exploration of Mars by several missions, but in particular, thanks first to Mars Express, and later to the Mars Atmosphere and Volatile EvolutioN (MAVEN) missions, has allowed us to start to appreciate how the ionosphere intertwine with the atmospheric layers and with the solar wind. It has also allowed us to start characterizing the basic properties of the various plasma boundaries and regions that exist in the Martian induced magnetosphere (e.g., the bow shock, magnetic pileup boundary, ionopause (e.g., Hall et al., 2019; Edberg, 2009; Mazelle, 2004; Sanchez-Cano et al., 2020), as well as processes that control the current Martian climate, from the general atmospheric circulation, to the role of photochemistry, clouds, development of dust storms (both local and global), channels of atmospheric escape (e.g., England et al., 2017; Hartwick, 2019; Farahat et al., 2021).
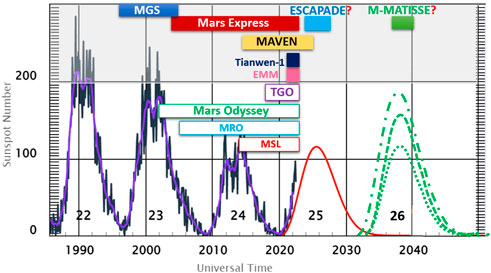
FIGURE 1. Time life of missions at Mars since 1986 with respect to solar cycles 22–26. Missions with ionospheric instrumentation are shown with filled boxes and over the grey band at the top of the Figure. Missions with complementary atmospheric or particle observations are shown with white boxes. ESCAPADE (awaiting for launch window period) and M-MATISSE (under current ESA study) are also shown as potential future missions. The sunspot numbers are plotted in black and with a running average in purple. The red line shows the current prediction for solar cycle 25. The multiple green lines show some potential possibilities for solar cycle 26 with the intention of showing the expected period of maximum solar activity. There has been an active ionospheric mission at Mars since 1996. MGS, Mars Global Surveyor; TGO, ExoMars Trace Gas Orbiter; MSL, Mars Science Laboratory; MRO, Mars Reconnaissance Orbiter; MAVEN, Mars Atmospheric and Volatile EvolutioN; EMM, Emirates Mars Mission; ESCAPADE, The Escape and Plasma Acceleration and Dynamics Explorers; M-MATISSE, Mars Magnetosphere ATmosphere Ionosphere and Space-weather SciencE. This Figure has been modified from the National Oceanic and Atmospheric Administration (NOAA) Space Weather Prediction Centre (https://www.swpc.noaa.gov/products/solar-cycle-progression).
Some remarkable findings are the characterization of the vertical structure of the dayside ionosphere and how it varies with altitude, solar zenith angle, solar flux, Sun-Mars distance, seasons, solar cycle, crustal magnetic fields, dust seasons, and water/CO2 cycles (Duru et al., 2006; Sanchez-Cano et al., 2013; Sanchez-Cano et al., 2015; Sanchez-Cano et al., 2016; Sanchez-Cano et al., 2018; Mendillo et al., 2013). These missions also found density fluctuations in the topside Martian ionosphere (Gurnett, 2010; Fowler et al., 2017), as well as large ionization layers that last for several days at the bottomside of the ionosphere after cometary dust is deposited, as seen after the flyby of comet Siding-Spring (Gurnett et al., 2015), or even highly sporadic lower magnitude layers found in the bottomside at other times less actives than during this cometary flyby (Peter et al., 2021).
We also have gained significant knowledge of the nightside ionosphere, including terminator transport (e.g., Withers et al., 2012), electron impact ionization down to 90 km (e.g., Lillis et al., 2018), as well as some insights into how the ionosphere is strongly controlled by the inclination of crustal magnetic fields (Němec et al., 2011). Moreover, we now know that the Martian induced tail is highly twisted (DiBraccio et al., 2018), mostly as a result of the complex interaction of the interplanetary magnetic field with the crustal magnetic fields and with the draped fields about the planet (e.g., Fang et al., 2015). The twisted tail may be potentially related to the large levels of electron precipitation observed in the nightside ionosphere, most of which lead to the generation of a myriad of auroras that have been observed at Mars (Schneider et al., 2015, 2021), such as: 1) “discrete auroras”, which occur typically over regions of strong vertical crustal magnetic fields (Bertaux, 2005); 2) “diffuse aurora”, which are caused by global precipitation of solar energetic particles on the nightside of Mars (e.g., Gerard et al., 2017); 3) “proton aurora”, which occurs on the dayside upper atmosphere and it is caused by solar wind proton precipitation (Deighan et al., 2018); 4) and the recently discovered “sinuous aurora” by the Emirates Mars Mission (EMM), which is characterized by elongated serpentine structures of thousands of kilometers in the nightside northern hemisphere, far from intense crustal fields (Lillis et al., 2022), and which origin is still unknown.
An important step forward in our knowledge of Mars as an integrate system has been the synergistic opportunities to use observations from different missions to investigate the variability of the ionosphere, and of the M-I-T coupling. This cooperation, mainly performed from researcher-based efforts, has opened a new door for global investigations of Mars, for the first time at other planet than earth.
3 What do we do not know about Mars’ ionosphere?
Despite the great progress in our understanding of the M-I-T coupling at Mars, there are major open questions that still need an answer, especially on the eve of Mars’ systematic robotic and human exploration. For example, a few highlights are, the fundamental nature of the plasma boundaries, as formed by systems of currents coupling the solar wind, magnetosphere, and ionosphere, is not well understood. Currents are a natural connection between different regions of a planet (Ramstad et al., 2020), and a quantitative description of their role, on both global and local scales, together with crustal fields, ionosphere, magnetosphere and particle and energy exchanges between regions is still missing (e.g., Sanchez-Cano et al., 2022).
Martian atmospheric losses to space are largely the result of thermal escape of neutral hydrogen and photochemical escape of neutral oxygen. This, together with sputtering, ion outflow, and pickup ion escape, are thought to have controlled the loss of liquid water on Mars over time (Jakosky, 2015), a critical aspect for understanding Martian habitability. However, the main atmospheric loss channels at Mars today cannot be observed because the rates of the escaping neutral hydrogen and oxygen atoms cannot be directly measured with current technology due to their low density and energy (several eV), although significant efforts have been done via different routes, such as on water vapour and hydrogen abundance observations, to get a better understanding of this escape mechanism (Holmes et al., 2021).
As mentioned before, aurora formation within the ionosphere on the nightside of Mars thanks to electron precipitation far from crustal fields is still a mystery. Energy deposition from particle precipitation can drive ionospheric structure through ionization. At earth, auroras are explained by direct cusp entry of the solar energetic particles, and by magnetospheric tail reconnection. However at Mars, this has not been confirmed, and could be related to both magnetotail field topology and poorly understood tail and cusp processes related to the localized crustal magnetic field regions.
Moving down into the lower altitudes, the bottom-side ionosphere is scarcely sampled; with practically no observations of the ionosphere below ∼70 km (only indirect observations and modelling efforts are available). Thus, a significant gap in our knowledge is present as it is believed this region to play a major role in the controls the amount of energy from the solar wind that is deposited into the atmosphere and eventually into the surface (e.g., Sanchez-Cano et al., 2021; Nakamura et al., 2022). This is particularly important when considering the radio propagation issues that low ionization creates, and therefore, being highly relevant to ground operations and future Mars exploration.
This is only a small sample of the Mars’ ionosphere unknowns, which may be essential knowledge for the future of Mars science and exploration, but also, to understand other worlds thought comparative planetology. Having good knowledge of Mars, which is relatively close to earth, easy accessible and has is near-unmagnetised in contrast with earth, is fundamental to extrapolate and compare knowledge to other worlds which may not be that accessible and limited observations can be taken.
4 Discussion: The ionosphere as key to unravel the way forward of Mars’ exploration
There are still many aspects that we do not yet understand from Mars environment, including many of the physical processes that drive matter and energy flow between and within the various atmospheric reservoirs (including temporal and spatial changes on short time scales, see Section 3), and although single-spacecraft missions provide a wealth of observations, synergetic and simultaneous multi-point measurements of the system are still missing. Simultaneous multi-point and coordinated measurements are required to determine how energy flows through the induced magnetosphere and into the ionosphere-atmosphere, causing important dynamics and energization (Sanchez-Cano et al., 2021).
From the near 60 years of space exploration at earth, we can draw the need for multi-point missions that have revolutionized our understanding of the terrestrial solar wind-magnetosphere-ionosphere coupling particularly during the last 20 years. At Mars, “ad-hoc” multi-spacecraft studies between existing individual assets have been undertaken and have demonstrated the huge potential that a coordinated mission has, but the instrument suites of existing assets are not designed for multi-point observations and opportunities for such analyses are rare. Thus, dedicated missions with multiple spacecraft having fully coordinated and simultaneous observations at different parts of the Martian system is critical to unravel the key mechanisms that strongly couple its surface with the M-I-T system and the solar wind, which requires at least two well-separated spacecraft with one in the solar wind and the other inside the system, and this is not available at the moment.
Following earth example, several multi-spacecraft missions have been proposed to fulfil this requirement, which will bring us to the next-generation of exploration at Mars where for the first time we will be able to disentangle spatial from temporal variability, and capture variations on short spatial/temporal scales that cannot be resolved from a single spacecraft. The first one of this missions is ESCAPADE (Lillis, 2020), which is a small-class NASA twin-spacecraft Mars orbiter mission which launch is still to be decided, that will provide a global picture of how solar wind energy flows through Mars’ unique hybrid magnetosphere to drive ion and sputtering escape. With views to future robotic and manned exploration of Mars, the Mars Magnetosphere Atmosphere Ionosphere and Space-weather Science (M-MATISSE) mission is currently being evaluated by ESA for the next Medium-size mission of opportunity to be launched not earlier than 2037. M-MATISSE is a two-spacecraft orbiting Mars to investigate the dynamic response of the M-I-T coupling to space weather activity (Sanchez-Cano et al., 2022). Moreover, the most ambitious of the missions concepts is the Mars Orbiters for Surface, Atmosphere, and Ionosphere Connections (MOSAIC) (Lillis et al., 2021), which is a Planetary Mission Concept Study mission from the NASA Science Decadal Survey, composed of 10 spacecraft to cover and investigate the system as a whole, with the most detailed ever coordination, covering all regions of the M-I-T coupling, including the surface and subsurface of Mars, and with a permanent monitoring of the Sun and solar wind.
All these missions have in common that the ionosphere is key in their observations. This is because Mars ionosphere is the “sponge” or “porous layer” in between the lower-middle atmosphere and space that facilitates the connection between different regions, where ultimately energy is dissipated, where the strongest dynamics occur, it is the reservoir for atmosphere escape, and it is where the larger part of the radiation filtering for the surface occurs. Moreover, the state of the ionosphere strongly controls communications with the surface and instrument operations in HF and UHF frequencies, as well as ionospheric irregularities can potentially produce scintillation in signal propagation. Therefore, the future of Mars exploration is strongly linked to the fate of the ionosphere, being the key for its future systematic exploration.
Data availability statement
The original contributions presented in the study are included in the article/Supplementary Material, further inquiries can be directed to the corresponding author.
Author contributions
BS-C has written and made the Figures of this paper.
Acknowledgments
Useful discussions concerning the main ideas of this article with Prof Mark Lester, Dr Francois Leblanc and Dr Olivier Witasse are acknowledged. The Mars Upper Atmosphere Network (MUAN) led by Dr Hermann Opgenoorth is greatly acknowledged for all the discussions and activities to bring the best science and facilitate collaborations from teams from all current missions at Mars in order to investigate the upper atmosphere of its interaction with the solar wind. BS-C acknowledges support through STFC Ernest Rutherford Fellowship ST/V004115/1.
Conflict of interest
The author declares that the research was conducted in the absence of any commercial or financial relationships that could be construed as a potential conflict of interest.
Publisher’s note
All claims expressed in this article are solely those of the authors and do not necessarily represent those of their affiliated organizations, or those of the publisher, the editors and the reviewers. Any product that may be evaluated in this article, or claim that may be made by its manufacturer, is not guaranteed or endorsed by the publisher.
References
Acuna, (1999). Magnetic field and plasma observations at Mars: Initial results of the Mars global Surveyor mission. Science 279 (5357), 1676–1680. doi:10.1126/science.279.5357.167
Bougher, (2001). Mars global Surveyor radio science electron density profiles ß neutral atmosphere implications. Geophys. Res. Lett. 28 (16), 3091–3094. doi:10.1029/2001GL012884
Collinson, G., McFadden, J., Mitchell, D., Grebowsky, J., Benna, M., Espley, J., et al. (2019). Traveling ionospheric disturbances at Mars. Geophys. Res. Lett. 46, 4554–4563. doi:10.1029/2019GL082412
Deighan, J., Jain, S. K., Chaffin, M. S., Fang, X., Halekas, J. S., Clarke, J. T., et al. (2018). Discovery of a proton aurora at Mars. Nat. Astron. 2 (10), 802–807. doi:10.1038/s41550-018-0538-5
DiBraccio, G. A., Luhmann, J. G., Curry, S. M., Espley, J. R., Xu, S., Mitchell, D. L., et al. (2018). The twisted configuration of the martian magnetotail: MAVEN observations. Geophys. Res. Lett. 45, 4559–4568. doi:10.1029/2018GL077251
Duru, F., Gurnett, D. A., Averkamp, T. F., Kirchner, D. L., Huff, R. L., Persoon, A. M., et al. (2006). Magnetically controlled structures in the ionosphere of Mars. J. Geophys. Res. 111, A12204. doi:10.1029/2006JA011975
Edberg, , 2009, Simultaneous measurements of martian plasma boundaries by Rosetta and Mars express, Planet. Space Sci., Volume 57, Issues 8–9. doi:10.1016/j.pss.2008.10.016
England, S. L., Liu, G., Yiğit, E., Mahaffy, P. R., Elrod, M., Benna, M., et al. (2017). MAVEN NGIMS observations of atmospheric gravity waves in the Martian thermosphere. J. Geophys. Res. Space Phys. 122, 2310–2335. doi:10.1002/2016JA023475
Fang, X., Ma, Y., Brain, D., Dong, Y., and Lillis, R. (2015). Control of Mars global atmospheric loss by the continuous rotation of the crustal magnetic field: A time-dependent MHD study. J. Geophys. Res. Space Phys. 120 (10926– 10), 944. doi:10.1002/2015JA021605
Fang, X., Ma, Y., Lee, Y., Bougher, S., Liu, G., Benna, M., et al. (2020). Mars dust storm effects in the ionosphere and magnetosphere and implications for atmospheric carbon loss. J. Geophys. Res. Space Phys. 125, e2019JA026838. doi:10.1029/2019JA026838
Farahat, A., Mayyasi, M., Withers, P., Dayeh, M. A., and Abuelgasim, A. (2021). Effects of the June 2018 global dust storm on the atmospheric composition of the Martian upper atmosphere as observed by MAVEN. J. Geophys. Res. Planets 126, e2021JE006868. doi:10.1029/2021JE006868
Fowler, C. M., Andersson, L., Shaver, S. R., Thayer, J. P., Huba, J. P., Lillis, R. J., et al. (2017). MAVEN observations of ionospheric irregularities at Mars. Geophys. Res. Lett. 44 (10), 854. doi:10.1002/2017GL075189
Gerard, J. C., Soret, L., Shematovich, V. I., Bisikalo, D. V., and Bougher, S. W. (2017). The Mars diffuse aurora: A model of ultraviolet and visible emissions. Icarus 288, 284–294. doi:10.1016/j.icarus.2017.01.037
Guo, J., Slaba, T. C., Zeitlin, C., Wimmer-Schweingruber, R. F., Badavi, F. F., Bohm, E., et al. (2017). Dependence of the Martian radiation environment on atmospheric depth: Modeling and measurement. J. Geophys. Res. Planets 122, 329–341. doi:10.1002/2016JE005206
Gurnett, D. A., Morgan, D. D., Persoon, A. M., Granroth, L. J., Kopf, A. J., Plaut, J. J., et al. (2015). An ionized layer in the upper atmosphere of Mars caused by dust impacts from comet Siding Spring. Geophys. Res. Lett. 42, 4745–4751. doi:10.1002/2015GL063726
Gurnett, (2010). Large density fluctuations in the martian ionosphere as observed by the Mars Express radar sounder. Icarus 206, 1. doi:10.1016/j.icarus.2009.02.019
Hall, B. E. S., Sánchez-Cano, B., Wild, J. A., Lester, M., and Holmstrom, M. (2019). The Martian bow shock over solar cycle 23–24 as observed by the Mars Express mission. J. Geophys. Res. Space Phys. 124, 4761–4772. doi:10.1029/2018JA026404
Hartwick, (2019). High-altitude water ice cloud formation on Mars controlled by interplanetary dust particles. Nat. Geosci. Vol. 12, 516–521. doi:10.1038/s41561-019-0379-6
Holmes, J. A., Lewis, S. R., Patel, M. R., Chaffin, M. S., Cangi, E. M., Deighan, J., et al. (2021). Enhanced water loss from the martian atmosphere during a regional-scale dust storm and implications for long-term water loss. Earth Planet. Sci. Lett. 571, 117109. ISSN 0012-821X. doi:10.1016/j.epsl.2021.117109
Jakosky, (2015). MAVEN Explores the martian upper atmosphere. SCIENCE 350 (6261), 643. doi:10.1126/science.aad3443
Langlais, B., Thébault, E., Houliez, A., Purucker, M. E., and Lillis, R. J. (2019). A new model of the crustal magnetic field of Mars using MGS and MAVEN. J. Geophys. Res. Planets 124, 1542–1569. doi:10.1029/2018JE005854
Lester, M., Sanchez-Cano, B., Potts, D., Lillis, R., Cartacci, M., Bernardini, F., et al. (2022). The impact of energetic particles on the Martian ionosphere during a full solar cycle of radar observations: Radar blackouts. J. Geophys. Res. Space Phys. 127, e2021JA029535. doi:10.1029/2021JA029535
Lillis, (2020). Escapade: Coordinated Multi-Point Observations Of Ion And Sputtered Escape From Mars, 51st Lunar and planetary science Conference. https://www.hou.usra.edu/meetings/lpsc2020/pdf/2470.pdf.
Lillis, R. J., Deighan, J., Brain, D., Fillingim, M., Jain, S., Chaffin, M., et al. (2022). First synoptic images of FUV discrete aurora and discovery of sinuous aurora at Mars by EMM EMUS. Geophys. Res. Lett. 49, e2022GL099820. doi:10.1029/2022GL099820
Lillis, R. J., Mitchell, D. L., Steckiewicz, M., Brain, D., Xu, S., Weber, T., et al. (2018). Ionizing electrons on the Martian nightside: Structure and variability. J. Geophys. Res. Space Phys. 123, 4349–4363. doi:10.1029/2017JA025151
Lillis, Robert J., Mitchell, David, Montabone, Luca, Heavens, Nicholas, Harrison, Tanya, Stuurman, Cassie, et al. (2021). Mosaic: A Satellite Constellation to enable Groundbreaking Mars climate system science and Prepare for human exploration. Planet. Sci. J. 10, 211. doi:10.3847/psj/ac0538
Mazelle, (2004). Bow shock and Upstream Phenomena at Mars. Space Sci. Rev. 111, 115–181. doi:10.1023/B:SPAC.0000032717.98679.d0
Mendillo, M., Marusiak, A. G., Withers, P., Morgan, D., and Gurnett, D. (2013). A new semiempirical model of the peak electron density of the Martian ionosphere. Geophys. Res. Lett. 40, 5361–5365. doi:10.1002/2013GL057631
Montabone, (2015). Eight-year climatology of dust optical depth on Mars. Icarus 251, 65–95. doi:10.1016/j.icarus.2014.12.034
Nakamura, Y., Terada, N., Leblanc, F., Rahmati, A., Nakagawa, H., Sakai, S., et al. (2022). Modeling of diffuse auroral emission at Mars: Contribution of MeV protons. J. Geophys. Res. Space Phys. 127 (1), e2021JA029914. doi:10.1029/2021JA029914
Němec, F., Morgan, D. D., Gurnett, D. A., Duru, F., and Truhlík, V. (2011). Dayside ionosphere of Mars: Empirical model based on data from the MARSIS instrument. J. Geophys. Res. 116, E07003. doi:10.1029/2010JE003789
Peter, K., Martin, P., GregorioMolina-Cuberos, J., González-Galindo, F., Olivier, W., Tellmann, S., et al. (2021). The lower dayside ionosphere of Mars from 14 years of Mars radio science observations. Icarus 359, 114213. ISSN 0019-1035. doi:10.1016/j.icarus.2020.114213
Ramstad, (2020). The global current systems of the Martian induced magnetosphere. Nat. Astron. 4, 979–985. doi:10.1038/s41550-020-1099-y
Riousset, J. A., Paty, C. S., Lillis, R. J., Fillingim, M. O., England, S. L., Withers, P. G., et al. (2014). Electrodynamics of the Martian dynamo region near magnetic cusps and loops. Geophys. Res. Lett. 41. doi:10.1002/2013GL059130
Sánchez-Cano, Beatriz, Lester, Mark, Andrews, David J., Hermann, Opgenoorth, Lillis, Robert, Leblanc, François, et al. (2021). Mars’ plasma system. Scientific potential of coordinated multipoint missions: “The next generation”. Exp. Astron. doi:10.1007/s10686-021-09790-0
Sánchez-Cano, Beatriz, Lester, Mark, Olivier, Witasse, Blelly, Pierre-Louis, Indurain, Mikel, Cartacci, Marco, et al. (2018). Seasonal, and solar cycle variations of the martian Total electron content (TEC): Is the TEC a good tracer for atmospheric cycles? J. Geophys. Res. Planets 10, 1746–1759. doi:10.1029/2018je005626
Sanchez-Cano, B., Lester, M., Leblanc, F., Andrews, D., and Opgenoorth, H. (2022). The M-MATISSE mission: Mars magnetosphere ATmosphere ionosphere and surface SciencE. 44th COSPAR Sci. Assem. Held 16-24 July 44, 421.
Sánchez-Cano, B., Lester, M., Witasse, O., Milan, S. E., Hall, B. E. S., Blelly, P.-L., et al. (2015). Evidence of scale height variations in the Martian ionosphere over the solar cycle. J. Geophys. Res. Space Phys. 120, 913–925. doi:10.1002/2015JA021949
Sánchez-Cano, B., Lester, M., Witasse, O., Milan, S. E., Hall, B. E. S., Cartacci, M., et al. (2016). Solar cycle variations in the ionosphere of Mars as seen by multiple Mars Express data sets. J. Geophys. Res. Space Phys. 121, 2547–2568. doi:10.1002/2015JA022281
Sánchez-Cano, B., Narvaez, C., Lester, M., Mendillo, M., Mayyasi, M., Holmstrom, M., et al. (2020). Mars' ionopause: A matter of pressures. J. Geophys. Res. Space Phys. 125, e2020JA028145. doi:10.1029/2020JA028145
Sanchez-Cano, , Radicella, S., Herraiz, M., Witasse, O., and Rodriguez-Caderot, G. (2013). NeMars: An empirical model of the martian dayside ionosphere based on Mars Express MARSIS data. Icarus 225 (1), 236–247. doi:10.1016/j.icarus.2013.03.021
Sánchez–Cano, B., Blelly, P.-L., Lester, M., Witasse, O., Cartacci, M., Orosei, R., et al. (2019). Origin of the extended Mars radar blackout of September 2017. J. Geophys. Res. Space Phys. 124, 4556–4568. doi:10.1029/2018JA026403
Schneider, N. M., Deighan, J. I., Jain, S. K., Stiepen, A., Stewart, A. I. F., Larson, D., et al. (2015). Discovery of diffuse aurora on Mars. Science 350 (6261), aad0313. doi:10.1126/science.aad0313
Schneider, N. M., Milby, Z., Jain, S. K., Gérard, J. C., Soret, L., Brain, D. A., et al. (2021). Discrete aurora on Mars: Insights into their distribution and activity from MAVEN/IUVS observations. J. Geophys. Res. Space Phys. 126 (10), e2021JA029428. doi:10.1029/2021JA029428
Vaisberg, O. L., Ermakov, V. N., Shuvalov, S. D., Zelenyi, L. M., Halekas, J., DiBraccio, G. A., et al. (2018). The structure of Martian magnetosphere at the dayside terminator region as observed on MAVEN spacecraft. J. Geophys. Res. Space Phys. 123, 2679–2695. doi:10.1002/2018JA025202
Keywords: mars, aeronomy, plasma, ionosphere, future exploration, article types perspective
Citation: Sánchez-Cano B (2023) Mars’ ionosphere: The key for systematic exploration of the red planet. Front. Astron. Space Sci. 9:1101945. doi: 10.3389/fspas.2022.1101945
Received: 18 November 2022; Accepted: 12 December 2022;
Published: 11 January 2023.
Edited by:
Jorge Luis Chau, Leibniz Institute of Atmospheric Physics (LG), GermanyReviewed by:
Scott England, Virginia Tech, United StatesCopyright © 2023 Sánchez-Cano. This is an open-access article distributed under the terms of the Creative Commons Attribution License (CC BY). The use, distribution or reproduction in other forums is permitted, provided the original author(s) and the copyright owner(s) are credited and that the original publication in this journal is cited, in accordance with accepted academic practice. No use, distribution or reproduction is permitted which does not comply with these terms.
*Correspondence: Beatriz Sánchez-Cano, YnNjbWRyMUBsZWljZXN0ZXIuYWMudWs=