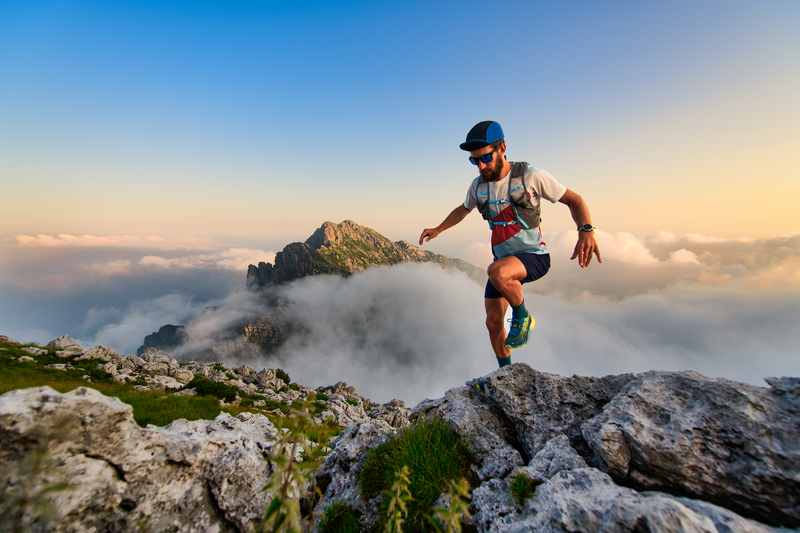
95% of researchers rate our articles as excellent or good
Learn more about the work of our research integrity team to safeguard the quality of each article we publish.
Find out more
PERSPECTIVE article
Front. Astron. Space Sci. , 01 December 2022
Sec. Space Physics
Volume 9 - 2022 | https://doi.org/10.3389/fspas.2022.1067571
This article is part of the Research Topic The Future of Space Physics 2022 View all 66 articles
Space weather refers to conditions around a star, like our Sun, and its interplanetary space that may affect space- and ground-based assets as well as human life. Space weather can manifest as many different phenomena, often simultaneously, and can create complex and sometimes dangerous conditions. The study of space weather is inherently trans-disciplinary, including subfields of solar, magnetospheric, ionospheric, and atmospheric research communities, but benefiting from collaborations with policymakers, industry, astrophysics, software engineering, and many more. Effective communication is required between scientists, the end-user community, and government organizations to ensure that we are prepared for any adverse space weather effects. With the rapid growth of the field in recent years, the upcoming Solar Cycle 25 maximum, and the evolution of research-ready technologies, we believe that space weather deserves a reexamination in terms of a “risk and resiliency” framework. By utilizing open data science, cross-disciplinary collaborations, information systems, and citizen science, we can forge stronger partnerships between science and industry and improve our readiness as a society to mitigate space weather impacts. The objective of this manuscript is to raise awareness of these concepts as we approach a solar maximum that coincides with an increasingly technology-dependent society, and introduce a unique way of approaching space weather through the lens of a risk and resiliency framework that can be used to further assess areas of improvement in the field.
Space weather is the physical and phenomenological state of space environments. The associated discipline aims through observation, monitoring, analysis, and modeling to understand and predict the state of the Sun, the interplanetary and planetary environments, and the solar and non-solar driven perturbations that affect them, as well as to forecast and nowcast the potential impacts on biological and technological systems (from COST Action 724, 20091, and in line with Temmer, 2021). As our understanding of space weather increases, so does the realization that answering the field’s most complex questions requires a new scientific approach that values convergence: The merging of innovative ideas, approaches, and technologies from a diverse range of sectors and expertise. In this article, we reflect on the current state of space weather research and discuss the next steps to advance the field during the next decade and beyond, specifically by formulating space weather in a risk and resiliency framework.
The grand challenges in heliophysics and space weather, especially those that involve complexity precluding uni-disciplinary analyses, may require new frameworks to further understand the multi-disciplinary knowledge necessary to make significant progress. A risk and resiliency framework (Scheffer et al., 2001; de Bruijn et al., 2017; Angeler et al., 2018, see Figure 1) provides a solid foundation for the evolution of our sciences beyond the next decade. Within this framework, a system is treated as a complex entity that can be defined by whether or not it can accommodate changes and reorganize itself while maintaining its unique characteristics (Scheffer et al., 2001). The framework is built on two important principles: 1) Consideration of the holistic Sun-to-society system, and 2) Quantification of the uncertainty that arises from coarse-graining and statistical simplification (McGranaghan, 2022). If space weather is approached through the lens of risk and resiliency, the domain could share a common framework with other risks such as terrestrial weather (e.g., hurricanes). This would allow researchers to conduct trans-disciplinary research into the convolved and compounding effects of space weather.
FIGURE 1. Overview of the Space Weather Risk and Resiliency Framework. Adapted from https://svs.gsfc.nasa.gov/30822 (original credit: NASA).
Space weather currently affects four main industry domains: ground infrastructure, high-frequency (HF) communications, near-Earth space assets and services, and aviation. Our nearest star creates five main space weather disturbances discussed in this article:
1. Coronal mass ejections (CMEs; e.g., Webb and Howard, 2012) are large-scale eruptions that carry huge quantities of plasma and magnetic fields into interplanetary space, occasionally in the direction of Earth. The fastest CMEs are usually the most geoeffective.
2. Solar flares (e.g., Benz, 2017) are sudden intense bursts of electromagnetic radiation from the solar atmosphere, and are associated with the impulsive release of magnetic energy via reconnection.
3. Solar energetic particle (SEP; e.g., Reames, 2013) events are associated with CMEs and flares. They cause large fluxes of high-energy relativistic protons and electrons to travel with the solar wind along the interplanetary magnetic field.
4. (Coronal-hole) high-speed streams (HSSs; e.g., Cranmer et al., 2017) are flows of fast solar wind that originate from open magnetic field lines in the Sun’s corona.
5. (Co-rotating) stream interaction regions (SIRs; e.g., Richardson, 2018) occur when HSSs overtake slower solar wind, producing regions of enhanced density and magnetic field strength.
In Section 2 of this article we provide a background of space weather and highlight areas of improvement in science and forecasting. We also share possible solutions (Section 3) using the concepts of open data and data science (Section 3.1), cross-disciplinary science and information systems (Section 3.2), as well as citizen science (Section 3.3). Concluding thoughts are summarized in Section 4.
The disturbances from the Sun mentioned in the Introduction can manifest into three main categories of space weather effects at Earth: Geomagnetic storms and substorms, radiation storms, and radio blackouts2. On Earth, space weather can lead to damaging effects with varying time scales and spatial footprints (see Eastwood et al., 2017; Hapgood et al., 2021). So-called “Carrington-scale events” refer to extreme space weather events that cause widespread infrastructure damage (Tsurutani et al., 2003; Baker et al., 2013; Riley et al., 2018; Cliver et al., 2022; Hayakawa et al., 2022). For industries, Carrington-scale events often present a “worst-case scenario.”
Although there are many affected sectors of society, within this article we will discuss space weather impacts in five industry domains, outlined below.
During radio blackouts and radiation storms, increased ionization in the atmosphere and ionosphere impacts high-frequency (HF) radio communication, which relies on ionospheric propagation for signal transmission and integrity. Signals either become degraded from distortion and scintillation or completely absorbed by the ionosphere (Kintner et al., 2007). HF radio communication is used by the aviation industry, the shipping industry, emergency responders, the amateur radio operator (“ham”) community (Frissell et al., 2022, 2019), and the military (Balch et al., 2004; Kelly et al., 2014). Mobile phone networks and global navigation satellite system (GNSS) timing services can also be affected and debilitated by solar flare radio noise (Kintner et al., 2009; Cannon et al., 2013). During minor-to-moderate space weather events, regional and global gaps in HF radio bands occur, but most critical infrastructure is designed to be resilient, i.e., capable of using multiple bands of communication or operating under expected noise. However, some infrastructure is not resilient enough: Even minor radio blackouts have caused airplanes to lose contact with ground controllers, especially over the North Atlantic (Fiori et al., 2022). Carrington-scale events may cause degraded HF communication performance for several days due to intense and prolonged radio blackouts caused by severe space weather (Cannon et al., 2013; Frissell et al., 2019).
During geomagnetic storms, substorms, and sudden impulse events, geomagnetically induced currents (GICs) in the ground may damage infrastructure, particularly power transformers, or offline certain power surge protection and fault-detection systems (Tsurutani et al., 2003; Cannon et al., 2013). These effects are most intense in the vicinity of the auroral ovals that surround Earth’s magnetic poles, but have also been observed at mid and low latitudes due to the effects of ionospheric (Pulkkinen et al., 2012; Ngwira et al., 2013; Carter et al., 2015) and magnetospheric (Russell et al., 1992; Shinbori et al., 2009) current systems. Consequently, GIC power grid impacts are widespread and have been observed in the United Kingdom (Erinmez et al., 2002; Thomson et al., 2005), Finland (Juusola et al., 2015), Sweden (Pulkkinen et al., 2005), Spain (Torta et al., 2012), the United States and Canada (Bolduc, 2002), South Africa (Lotz and Cilliers, 2015; Matandirotya et al., 2015), Japan (Watari, 2015), China (Wang et al., 2015), Australia (Marshall et al., 2011), New Zealand (Oliveira et al., 2018), and Brazil (Trivedi et al.,2007).
During geomagnetic storms, the thermosphere often expands and changes the neutral density of low-Earth-orbit altitudes (Danilov and Laštovička, 2002; Prölss, 2011; Oliveira et al., 2020). Satellites flying at these heights experience increased drag, causing a deceleration in the orbital direction and loss of altitude. Manual intervention often must be taken to ensure the nominal orbit of the spacecraft is maintained (Capon et al., 2019; Smith et al., 2019). A notable recent example of this phenomenon was in February 2022, when 38 SpaceX Starlink satellites re-entered Earth’s atmosphere after a space weather event (Dang et al., 2022; Hapgood et al., 2022). During geomagnetic storms and substorms, energetic electrons become trapped in Earth’s radiation belts, causing electrostatic charging and discharging on spacecraft, which can damage sensitive electronic equipment (Koons et al., 1998; Wrenn et al., 2002; Hapgood, 2004; Choi et al., 2011; Loto’aniu et al., 2015). Alongside short-term effects, a satellite’s performance may also degrade over time due to radiation events. Many satellite providers use our current understanding of the climatology of the radiation environments to determine the expected total dose over their satellite’s lifetime and include a safety margin to ensure resiliency. As a consequence, complete satellite losses are rare.
Earth is constantly bombarded by high-energy charged particles either from the Sun (i.e., SEPs) or from interstellar space (known as galactic cosmic rays or GCRs). During strong radiation storms, SEPs can be accelerated to relativistic energies (thus penetrating Earth’s magnetosphere) and secondary particles can be detected even on the ground by neutron monitors, a phenomenon known as a ground level enhancement (GLE; e.g., Nitta et al., 2012). Due to open magnetic field lines at Earth’s poles, particle fluxes and the resulting background radiation environment are highest at high latitudes (Compton, 1933; O’Brien et al., 1996; Mertens et al., 2010). This ionizing radiation can have biological impacts on aircrew (Dyer and Truscott, 1999; Lindborg et al., 2004). While unlikely, during severe space weather events crew radiation dose limits may be reached and airlines may choose to reroute high-risk flights (Jones et al., 2005). In geospace, humans lose radiation protection from the atmosphere, receive higher overall dose rates (Dachev et al., 2017), and are greatly affected by radiation storms (Berrilli et al., 2014), potentially leading to adverse health effects over time (Cucinotta, 2014). In interplanetary space and on the Moon, humans lose all magnetic or atmospheric shielding and are exposed to even higher radiation levels (Reitz et al., 2012). On Mars, due to the planet’s thinner atmosphere and weaker magnetic field, dose rates are much higher than on Earth (Guo et al., 2021). The space radiation environment will present challenges to upcoming crewed lunar and martian missions.
Single event upsets (SEUs) occur when ionizing radiation changes the internal voltages in electronics, leading to the corruption of stored or transmitted data (Dodd and Massengill, 2003; Oates, 2015). During radiation storms, highly energetic particles cause increased rates of SEUs in electronics (Campbell et al., 2002; Lohmeyer and Cahoy, 2013). SEUs affect all electronics, regardless of altitude. During the 2003 Halloween storms, about 10% (47 out of 450) of satellites experienced anomalies, one scientific satellite was lost, and 10 satellites were non-operational for over 1 day (Balch et al., 2004). Effects from these events were observed even at Mars, the most dramatic outcome being the loss of a radiation monitor aboard the 2001 Mars Odyssey satellite (Zeitlin et al., 2010). SEUs also affect avionics instruments (Taber and Normand, 1993), although almost all commercial aircraft contain mitigation techniques to limit disruptions3, and ground electronics, although critical electronics have error-correcting mechanisms in most cases (Normand, 1996).
Space weather simultaneously affects these five domains and creates complex and challenging situations for forecasters and industry managers. Our scientific understanding of these industry-specific risks to space weather is growing, but there are still gaps in knowledge. Several international efforts are underway to identify and reduce these gaps, as well as to explore ways to improve our readiness for harmful space weather impacts (e.g., Schrijver et al., 2015; Opgenoorth et al., 2019; Tsurutani et al., 2020; Lilensten et al., 2021), but are we fully prepared for a Carrington-scale event (Riley and Love, 2017)? The answer to this question depends on who is being asked, and information regarding the ways in which industries respond to space weather is not readily publicly available. As we prepare for the next decade of space weather, including the Solar Cycle 25 maximum, there are a multitude of avenues by which we can improve forecasting and research to achieve clearer scientific understanding and well-integrated inter-disciplinary collaboration.
Adopting a new systems-science approach for space weather, utilizing, e.g., open data and citizen science, will cultivate cross-disciplinary collaborations that help solve challenging problems in unique ways.
As the amount of space weather data increases, data science projects and principles will enable new scientific discoveries via open access and collaboration.
As the number of models, data, and model–data fusion products increases with the growth of the space weather field, so does our recognition that powerful new opportunities for scientific discovery are made possible by utilizing data science optimized for large data volumes. Data science in regards to space weather refers to scalable architectural approaches, techniques, software, and algorithms that alter the paradigm by which data are collected, managed, analyzed, and communicated (McGranaghan et al., 2017).
Data science-driven transformations in related fields such as Earth science (Yue et al., 2016) and climate research (Carleton and Hsiang, 2016) are a testament to the immense potential of leveraging these new ideas, and similar efforts are beginning to take root in space weather.
The NSF EarthCube project, Assimilative Mapping of Geospace Observations (AMGeO4; Matsuo et al., 2021) demonstrates the potential of implementing data science best practices. The project deploys a collaborative data science platform to investigate the constantly changing conditions of high-latitude ionospheric electrodynamics. AMGeO connects geospace observational datasets from NSF-funded facility programs (e.g., SuperDARN, AMPERE, and SuperMAG) to form a coherent specification of ionospheric electrodynamics. AMGeO does this through open-source Python software and an online interface that facilitates data acquisition and pre-processing. The project streamlines data access, collection, and integration, and its software is designed to be transparent, expandable, and interoperable to encourage collaboration and engagement within the geospace community. The newly-formed “near-Earth space data infrastructure for e-science” (ESPAS; Belehaki et al., 2016) is a similar project with a special emphasis of standardized vocabulary and expandability to improve long-term sustainability. The Python in Heliophysics Community (PhYC5) effort promotes open data through a knowledge base for performing heliophysics research in Python.
Given the breadth of the space weather field—spanning from solar physics to geology—inter-disciplinary science connecting phenomena from the Sun to Earth may require datasets from many organizations, instruments, and agencies. Making observations and data open source reduces the barrier to starting research and can help accelerate needed discoveries—the “democratization” of science. While the needs of specific industries may vary from country to country, these specific perspectives help scientists understand why these regional variations exist. Making these data available, translatable, and accessible to a worldwide audience will help foster international collaboration, a critical step in reacting to and developing technology for the regional and global nature of space weather phenomena.
Understanding space weather from a broad perspective is important for industries to communicate their needs to scientists, and vice-versa. Common scientific understanding improves the utility of discovery and unifies efforts.
An information system is a technology that provides the structure to collect, store, process, and integrate data. The Sun-to-industry information system is an important part of creating industries resilient to space weather, and coordinating collaboration across disciplines improves scientific knowledge. Specifically, information currently flows mainly in one direction: from space weather to industry, but this should not be the case. Bidirectional communication improves scientists’ understanding of what industries need (e.g., real-time maps of GICs used by power grids). Communication also improves trust in space weather models, which helps clarify risk. Additionally, coordinating across scientific communities is an important step in developing a “systems science” approach to space weather. In order for the observation, forecasting, and modeling of space weather to improve, a knowledge commons shared between disciplines should be created. This sharing of information will standardize the glossary and semantics of space weather, fostering collaboration without current communication barriers, while also preventing the duplication of effort through the gathering and sharing of knowledge across groups. Optimizing the information flow “from Sun to mud” means that we can create more refined and polished plans for how industries prepare for and respond to space weather.
Citizen science is a field that connects scientists to the public, enabling discovery especially at disciplinary boundaries. Successful projects in heliophysics and related fields make citizen science capable of shedding light on some of the most challenging space weather mysteries.
Citizen science is a rapidly growing, recently formalized field that is fueled by the concept of cognitive surplus, i.e., that small amounts of volunteered time by many people can contribute to a larger scientific goal (Shirky, 2010). Projects that incorporate citizen science have the potential to engage and motivate broad, global audiences to drive new scientific discoveries, while still maintaining data quality. Citizen science also centers around creating open-data frameworks, making data accessible to the interested public. Citizen science projects are frequent and well-established in astronomy (e.g., Globe at Night), and within the past solar cycle, a number of projects have emerged to study space weather. One such project, Aurorasaurus (MacDonald et al., 2015), utilizes manual reports as well as aggregate Twitter sightings of aurora to develop a more accurate nowcast prediction of the auroral ovals. In some instances, citizen science reports map the aurora more realistically in real time than operational models (Case et al., 2016). The Aurorasaurus community has also contributed to discoveries relating to the STEVE (Strong Thermal Emission Velocity Enhancement) phenomenon, using citizen scientists’ aurora photographs (Gallardo-Lacourt et al., 2018; MacDonald et al., 2018; Chu et al., 2020; Grandin, 2020; Hunnekuhl and MacDonald, 2020; Semeter et al., 2020). Other notable citizen science projects include Solar Stormwatch (Jones et al., 2017), Solar Jet Hunter (Musset et al., 2021), and NOAA’s CrowdMAG (Nair et al., 2014), which has proven capable of detecting geomagnetic fields (Robinson et al., 2021). Citizen science works in tandem with initiatives focusing on systems science, open data, and international collaboration. Projects also serve educational purposes, helping to create a more well-informed public that is aware of space weather. MUSICS (Archer et al., 2018) and Space Weather UnderGround (Smith, 2022) are recent notable examples. In space weather, it enables novel ways to monitor conditions on the ground in real time, and contributes to scientific endeavors that require the analysis of large datasets. Together with developing data aggregates and knowledge commons, citizen science projects may be developed to leverage the large amount of data linking industry and science.
Adapting a risk and resiliency framework for space weather is crucial for tackling heliophysics challenges during the next decade and beyond. Utilizing open data and data science is one route to shape existing data products, tools, and software to be more easily accessible. The “democratization of science” will only happen if we promote existing projects and develop new efforts to converge information into a knowledge commons that can be accessed by scientists, industries, the public, and other end-users. Cross-disciplinary efforts will be crucial for advancing space weather. Specifically, adopting information systems where knowledge is shared between scientists, space weather forecasters, and affected industries will improve our readiness for space weather threats. Working towards this goal will require dedicated efforts to convene scientists, community members, and industry representatives (e.g., cross-disciplinary workshops and industry test-bed scenarios). Finally, citizen science takes these concepts and puts them into practice, by employing science–public partnerships, community-building, data sovereignty and accessibility, reciprocity efforts, and inter-disciplinary science to advance the field of space weather. Most importantly, citizen science projects are able to quickly evolve to answer emerging science questions. Agency-specific recommendations reflecting these sentiments can be found in the white paper of the same title submitted to the 2024–2033 Heliophysics Decadal Survey (Ledvina et al., 2022).
Over the next decade, the challenges of the next solar cycle maximum coinciding with an increasingly technology-dependent society will demand new technologies, collaborations, and innovative research methods to bridge knowledge gaps in science, operations, and industry responses to space weather. Creating a risk and resiliency framework for space weather will ensure that we can approach these problems prepared and adapt to create resilient and responsive systems.
The original contributions presented in the study are included in the article/Supplementary Material, further inquiries can be directed to the corresponding authors.
VEL led the organization of this article. All authors listed have made a substantial, direct, and intellectual contribution to the work and approved it for publication.
EP acknowledges NSF’s PREEVENTS program (Grant No. ICER-1854790). AJF was supported by the Space Precipitation Impacts project at Goddard Space Flight Center through the Heliophysics Internal Science Funding Model. CD was supported by Princeton Plasma Physics Laboratory through the Laboratory Directed Research and Development (LDRD) Program under DOE Prime Contract No. DE-AC02-09CH11466. NAF acknowledges the support of NSF AGS-2045755.
The authors are pleased to acknowledge the organizers and participants of the 3rd Eddy Cross Disciplinary Symposium (https://cpaess.ucar.edu/meetings/eddy-symposium-2022), held during June 6–10, 2022 in Vail (CO), United States, from which discussion on the Space Weather Risk and Resiliency Framework originated.
VEL, EP, EIM, and PR were employed by Predictive Science Inc. RMM was employed by Orion Space Solutions. JC was employed by 2i2c. JK was employed by Jufa Intermedia—Capture North.
The remaining authors declare that the research was conducted in the absence of any commercial or financial relationships that could be construed as a potential conflict of interest.
All claims expressed in this article are solely those of the authors and do not necessarily represent those of their affiliated organizations, or those of the publisher, the editors and the reviewers. Any product that may be evaluated in this article, or claim that may be made by its manufacturer, is not guaranteed or endorsed by the publisher.
1https://swe.ssa.esa.int/what-is-space-weather.
2https://www.swpc.noaa.gov/noaa-scales-explanation.
3https://www.faa.gov/aircraft/air_cert/design_approvals/air_software/media/TC-15-62.pdf.
Angeler, D. G., Allen, C. R., Garmestani, A., Pope, K. L., Twidwell, D., and Bundschuh, M. (2018). Resilience in environmental risk and impact assessment: Concepts and measurement. Bull. Environ. Contam. Toxicol. 101, 543–548. doi:10.1007/s00128-018-2467-5
Archer, M. O., Hartinger, M. D., Redmon, R., Angelopoulos, V., Walsh, B. M., and Students, E. H. S. Y. P. (2018). First results from sonification and exploratory citizen science of magnetospheric ulf waves: Long-lasting decreasing-frequency poloidal field line resonances following geomagnetic storms. Space weather. 16, 1753–1769. doi:10.1029/2018SW001988
Baker, D. N., Li, X., Pulkkinen, A., Ngwira, C. M., Mays, M. L., Galvin, A. B., et al. (2013). A major solar eruptive event in July 2012: Defining extreme space weather scenarios. Space weather. 11, 585–591. doi:10.1002/swe.20097
Balch, C., Murtagh, B., Zezula, D., Combs, L., Nelson, G., Tegnell, K., et al. (2004). Intense space weather storms october 19–november 07, 2003, service assessment. NOAA, U. S. Dep. Commer. Silver Spring 106, 1–47.
Belehaki, A., James, S., Hapgood, M., Ventouras, S., Galkin, I., Lembesis, A., et al. (2016). The ESPAS e-infrastructure: Access to data from near-Earth space. Adv. Space Res. 58, 1177–1200. doi:10.1016/j.asr.2016.06.014
Berrilli, F., Casolino, M., Del Moro, D., Di Fino, L., Larosa, M., Narici, L., et al. (2014). The relativistic solar particle event of May 17th, 2012 observed on board the International Space Station. J. Space Weather Space Clim. 4, A16. doi:10.1051/swsc/2014014
Bolduc, L. (2002). GIC observations and studies in the Hydro-Québec power system. J. Atmos. Sol. Terr. Phys. 64, 1793–1802. doi:10.1016/S1364-6826(02)00128-1
Campbell, A., Buchner, S., Petersen, E., Blake, B., Mazur, J., and Dyer, C. (2002). SEU measurements and predictions on MPTB for a large energetic solar particle event. IEEE Trans. Nucl. Sci. 49, 1340–1344. doi:10.1109/TNS.2002.1039664
Cannon, P., Angling, M., Barclay, L., Curry, C., Dyer, C., Edwards, R., et al. (2013). Extreme space weather: Impacts on engineered systems and infrastructure. London, United Kingdom: Royal Academy of Engineering.
Capon, C. J., Smith, B., Brown, M., Abay, R., and Boyce, R. R. (2019). Effect of ionospheric drag on atmospheric density estimation and orbit prediction. Adv. Space Res. 63, 2495–2505. doi:10.1016/j.asr.2019.01.013
Carleton, T. A., and Hsiang, S. M. (2016). Social and economic impacts of climate. Science 353, aad9837. doi:10.1126/science.aad9837
Carter, B. A., Yizengaw, E., Pradipta, R., Halford, A. J., Norman, R., and Zhang, K. (2015). Interplanetary shocks and the resulting geomagnetically induced currents at the equator. Geophys. Res. Lett. 42, 6554–6559. doi:10.1002/2015GL065060
Case, N. A., MacDonald, E. A., and Viereck, R. (2016). Using citizen science reports to define the equatorial extent of auroral visibility. Space weather. 14, 198–209. doi:10.1002/2015SW001320
Choi, H.-S., Lee, J., Cho, K.-S., Kwak, Y.-S., Cho, I.-H., Park, Y.-D., et al. (2011). Analysis of GEO spacecraft anomalies: Space weather relationships. Space weather. 9, 06001. doi:10.1029/2010SW000597
Chu, X., Wolter, L., Malaspina, D., Andersson, L., Connors, M., Chatfield, C., et al. (2020). Morphological characteristics of strong thermal emission velocity enhancement emissions. JGR. Space Phys. 125, e2020JA028110. doi:10.1029/2020JA028110
Cliver, E. W., Schrijver, C. J., Shibata, K., and Usoskin, I. G. (2022). Extreme solar events. Living Rev. Sol. Phys. 19, 2. doi:10.1007/s41116-022-00033-8
Compton, A. H. (1933). A geographic study of cosmic rays. Phys. Rev. 43, 387–403. doi:10.1103/PhysRev.43.387
Cranmer, S. R., Gibson, S. E., and Riley, P. (2017). Origins of the ambient solar wind: Implications for space weather. Space Sci. Rev. 212, 1345–1384. doi:10.1007/s11214-017-0416-y
Cucinotta, F. A. (2014). Space radiation risks for astronauts on multiple international space station missions. PLoS ONE 9, e96099. doi:10.1371/journal.pone.0096099
Dachev, T. P., Bankov, N. G., Tomov, B. T., Matviichuk, Y. N., Dimitrov, P. G., Häder, D. P., et al. (2017). Overview of the ISS radiation environment observed during the ESA EXPOSE-R2 mission in 2014-2016. Space weather. 15, 1475–1489. doi:10.1002/2016SW001580
Dang, T., Li, X., Luo, B., Li, R., Zhang, B., Pham, K., et al. (2022). Unveiling the space weather during the Starlink satellites destruction event on 4 february 2022. Space weather. 20, e2022SW003152. doi:10.1029/2022SW003152
Danilov, A., and Laštovička, J. (2002). Effects of geomagnetic storms on the ionosphere and atmosphere. Int. J. Geomag. Aeron. 7, 278–286.
de Bruijn, K., Buurman, J., Mens, M., Dahm, R., and Klijn, F. (2017). Resilience in practice: Five principles to enable societies to cope with extreme weather events. Environ. Sci. Policy 70, 21–30. doi:10.1016/j.envsci.2017.02.001
Dodd, P. E., and Massengill, L. W. (2003). Basic mechanisms and modeling of single-event upset in digital microelectronics. IEEE Trans. Nucl. Sci. 50, 583–602. doi:10.1109/TNS.2003.813129
Dyer, C., and Truscott, P. (1999). Cosmic radiation effects on avionics. Microprocess. Microsyst. 22, 477–483. doi:10.1016/S0141-9331(98)00106-9
Eastwood, J. P., Biffis, E., Hapgood, M. A., Green, L., Bisi, M. M., Bentley, R. D., et al. (2017). The economic impact of space weather: Where do we stand? Risk Anal. 37, 206–218. doi:10.1111/risa.12765
Erinmez, I. A., Kappenman, J. G., and Radasky, W. A. (2002). Management of the geomagnetically induced current risks on the national grid company’s electric power transmission system. J. Atmos. Sol. Terr. Phys. 64, 743–756. doi:10.1016/S1364-6826(02)00036-6
Fiori, R. A. D., Kumar, V. V., Boteler, D. H., and Terkildsen, M. B. (2022). Occurrence rate and duration of space weather impacts on high-frequency radio communication used by aviation. J. Space Weather Space Clim. 12, 21. doi:10.1051/swsc/2022017
Frissell, N. A., Kaeppler, S. R., Sanchez, D. F., Perry, G. W., Engelke, W. D., Erickson, P. J., et al. (2022). First observations of large scale traveling ionospheric disturbances using automated amateur radio receiving networks. Geophys. Res. Lett. 49, e2022GL097879. doi:10.1029/2022GL097879
Frissell, N. A., Vega, J. S., Markowitz, E., Gerrard, A. J., Engelke, W. D., Erickson, P. J., et al. (2019). High-frequency communications response to solar activity in september 2017 as observed by amateur radio networks. Space weather. 17, 118–132. doi:10.1029/2018SW002008
Gallardo-Lacourt, B., Nishimura, Y., Donovan, E., Gillies, D. M., Perry, G. W., Archer, W. E., et al. (2018). A statistical analysis of steve. JGR. Space Phys. 123, 9893–9905. doi:10.1029/2018JA025368
Grandin, M. (2020). Small-scale optical atmospheric emissions discovered using citizen science photography. AGU Adv. 1, e2020AV000268. doi:10.1029/2020AV000268
Guo, J., Zeitlin, C., Wimmer-Schweingruber, R. F., Hassler, D. M., Ehresmann, B., Rafkin, S., et al. (2021). Radiation environment for future human exploration on the surface of Mars: The current understanding based on MSL/RAD dose measurements. Astron. Astrophys. Rev. 29, 8. doi:10.1007/s00159-021-00136-5
Hapgood, M., Angling, M. J., Attrill, G., Bisi, M., Cannon, P. S., Dyer, C., et al. (2021). Development of space weather reasonable worst case scenarios for the UK national risk assessment. Space weather. 19, e2020SW002593. doi:10.1029/2020SW002593
Hapgood, M., Liu, H., and Lugaz, N. (2022). SpaceX—sailing close to the space weather? Space weather. 20, e2022SW003074. doi:10.1029/2022SW003074
Hapgood, M. (2004). SEDAT Report on spacecraft anomalies analysis.Report on demonstration of correlation of spacecraft anomalies with environment data
Hayakawa, H., Nevanlinna, H., Blake, S. P., Ebihara, Y., Bhaskar, A. T., and Miyoshi, Y. (2022). Temporal variations of the three geomagnetic field components at colaba observatory around the Carrington storm in 1859. Astrophys. J. 928, 32. doi:10.3847/1538-4357/ac2601
Hunnekuhl, M., and MacDonald, E. (2020). Early ground-based work by auroral pioneer carl størmer on the high-altitude detached subauroral arcs now known as “STEVE”. Space weather. 18, e2019SW002384. doi:10.1029/2019SW002384
Jones, J. B. L., Bentley, R. D., Hunter, R., Iles, R. H. A., Taylor, G. C., and Thomas, D. J. (2005). Space weather and commercial airlines. Adv. Space Res. 36, 2258–2267. doi:10.1016/j.asr.2004.04.017
Jones, S. R., Barnard, L. A., Scott, C. J., Owens, M. J., and Wilkinson, J. (2017). Tracking CMEs using data from the Solar Stormwatch project; observing deflections and other properties. Space weather. 15, 1125–1140. doi:10.1002/2017SW001640
Juusola, L., Viljanen, A., van de Kamp, M., Tanskanen, E. I., Vanhamäki, H., Partamies, N., et al. (2015). High-latitude ionospheric equivalent currents during strong space storms: Regional perspective. Space weather. 13, 49–60. doi:10.1002/2014SW001139
Kelly, M. A., Comberiate, J. M., Miller, E. S., and Paxton, L. J. (2014). Progress toward forecasting of space weather effects on UHF SATCOM after Operation Anaconda. Space weather. 12, 601–611. doi:10.1002/2014SW001081
Kintner, P. M., Ledvina, B. M., and de Paula, E. R. (2007). GPS and ionospheric scintillations. Space weather. 5, 09003. doi:10.1029/2006SW000260
Kintner, P. M., O’Hanlon, B., Gary, D. E., and Kintner, P. M. S. (2009). Global Positioning System and solar radio burst forensics. Radio Sci. 44, RS0A08. doi:10.1029/2008RS004039
Koons, H. C., Mazur, J. E., Selesnick, R. S., Blake, J. B., Fennell, J. F., Roeder, J. L., et al. (1998). The impact of the space environment on space systems. Proc. 6th Spacecr. Charging Conf., 7–11.
Ledvina, V. E., McGranaghan, R. M., Palmerio, E., Halford, A. J., Brandt, L., Thayer, A., et al. (2022). How open data and interdisciplinary collaboration improve our understanding of space weather: A risk & resiliency perspective. Bull. Am. Astron. Soc. in press.
Lilensten, J., Dumbović, M., Spogli, L., Belehaki, A., Van der Linden, R., Poedts, S., et al. (2021). Quo vadis, European space weather community? J. Space Weather Space Clim. 11, 26. doi:10.1051/swsc/2021009
Lindborg, L., Bartlett, D., Beck, P., McAulay, I., Schnuer, K., Schraube, H., et al. (2004). Cosmic radiation exposure of aircraft crew: Compilation of measured and calculated data. Radiat. Prot. Dosim. 110, 417–422. doi:10.1093/rpd/nch232
Lohmeyer, W. Q., and Cahoy, K. (2013). Space weather radiation effects on geostationary satellite solid-state power amplifiers. Space weather. 11, 476–488. doi:10.1002/swe.20071
Loto’aniu, T. M., Singer, H. J., Rodriguez, J. V., Green, J., Denig, W., Biesecker, D., et al. (2015). Space weather conditions during the Galaxy 15 spacecraft anomaly. Space weather. 13, 484–502. doi:10.1002/2015SW001239
Lotz, S. I., and Cilliers, P. J. (2015). A solar wind-based model of geomagnetic field fluctuations at a mid-latitude station. Adv. Space Res. 55, 220–230. doi:10.1016/j.asr.2014.09.014
MacDonald, E. A., Case, N. A., Clayton, J. H., Hall, M. K., Heavner, M., Lalone, N., et al. (2015). Aurorasaurus: A citizen science platform for viewing and reporting the aurora. Space weather. 13, 548–559. doi:10.1002/2015SW001214
MacDonald, E. A., Donovan, E., Nishimura, Y., Case, N. A., Gillies, D. M., Gallardo-Lacourt, B., et al. (2018). New science in plain sight: Citizen scientists lead to the discovery of optical structure in the upper atmosphere. Sci. Adv. 4, eaaq0030. doi:10.1126/sciadv.aaq0030
Marshall, R. A., Smith, E. A., Francis, M. J., Waters, C. L., and Sciffer, M. D. (2011). A preliminary risk assessment of the Australian region power network to space weather. Space weather. 9, S10004. doi:10.1029/2011SW000685
Matandirotya, E., Cilliers, P. J., and Van Zyl, R. R. (2015). Modeling geomagnetically induced currents in the South African power transmission network using the finite element method. Space weather. 13, 185–195. doi:10.1002/2014SW001135
Matsuo, T., Kilcommons, L., Mirkovich, W., Ruohoniemi, J. M., Chakraborty, S., Anderson, B., et al. (2021). “Assimilative mapping of geospace observations (AMGeO): Data science tools for collaborative geospace systems science,” in AGU fall meeting abstracts. SA11A–08.
McGranaghan, R. M., Bhatt, A., Matsuo, T., Mannucci, A. J., Semeter, J. L., and Datta-Barua, S. (2017). Ushering in a new frontier in geospace through data science. JGR. Space Phys. 122, 590. doi:10.1002/2017JA024835
McGranaghan, R. M. (2022). The evolution of heliophysics: Complexity, community, and open science. Front. Astron. Space Sci. 9, 951411. doi:10.3389/fspas.2022.951411
Mertens, C. J., Kress, B. T., Wiltberger, M., Blattnig, S. R., Slaba, T. S., Solomon, S. C., et al. (2010). Geomagnetic influence on aircraft radiation exposure during a solar energetic particle event in October 2003. Space weather. 8, S03006. doi:10.1029/2009SW000487
Musset, S., Glesener, L., Fortson, L., Kapsiak, C., Ostlund, E., Alnahari, S., et al. (2021). “Solar Jet hunter: A citizen science investigation of coronal solar jets,” in AGU fall meeting abstracts. SA32A–07.
Nair, M. C., Boneh, N., and Chulliat, A. (2014). “CrowdMag - crowdsourcing magnetic data,” in AGU fall meeting abstracts. ED53A–3470.
Ngwira, C. M., Pulkkinen, A., Wilder, F. D., and Crowley, G. (2013). Extended study of extreme geoelectric field event scenarios for geomagnetically induced current applications. Space Weather 121, 121–131. doi:10.1002/swe.20021
Nitta, N. V., Liu, Y., DeRosa, M. L., and Nightingale, R. W. (2012). What are special about ground-level events? Flares, CMEs, active regions and magnetic field connection. Space Sci. Rev. 171, 61–83. doi:10.1007/s11214-012-9877-1
Normand, E. (1996). Single event upset at ground level. IEEE Trans. Nucl. Sci. 43, 2742–2750. doi:10.1109/23.556861
Oates, A. (2015). “Reliability of silicon integrated circuits,” in Reliability characterisation of electrical and electronic systems. Editor J. Swingler (Oxford, United Kingdom: Woodhead Publishing). 115–141. doi:10.1016/B978-1-78242-221-1.00007-1
O’Brien, K., Friedberg, W., Sauer, H. H., and Smart, D. (1996). Atmospheric cosmic rays and solar energetic particles at aircraft altitudes. Environ. Int. 22, 9–44. doi:10.1016/S0160-4120(96)00086-4
Oliveira, D. M., Arel, D., Raeder, J., Zesta, E., Ngwira, C. M., Carter, B. A., et al. (2018). Geomagnetically induced currents caused by interplanetary shocks with different impact angles and speeds. Space weather. 16, 636–647. doi:10.1029/2018SW001880
Oliveira, D. M., Zesta, E., Hayakawa, H., and Bhaskar, A. (2020). Estimating satellite orbital drag during historical magnetic superstorms. Space weather. 18, e2020SW002472. doi:10.1029/2020SW002472
Opgenoorth, H. J., Wimmer-Schweingruber, R. F., Belehaki, A., Berghmans, D., Hapgood, M., Hesse, M., et al. (2019). Assessment and recommendations for a consolidated European approach to space weather - as part of a global space weather effort. J. Space Weather Space Clim. 9, A37. doi:10.1051/swsc/2019033
Prölss, G. W. (2011). Density perturbations in the upper atmosphere caused by the dissipation of solar wind energy. Surv. Geophys. 32, 101–195. doi:10.1007/s10712-010-9104-0
Pulkkinen, A., Bernabeu, E., Eichner, J., Beggan, C., and Thomson, A. W. P. (2012). Generation of 100-year geomagnetically induced current scenarios. Space Weather 10, 04003. doi:10.1029/2011SW000750
Pulkkinen, A., Lindahl, S., Viljanen, A., and Pirjola, R. (2005). Geomagnetic storm of 29-31 October 2003: Geomagnetically induced currents and their relation to problems in the Swedish high-voltage power transmission system. Space weather. 3, S08C03. doi:10.1029/2004SW000123
Reames, D. V. (2013). The two sources of solar energetic particles. Space Sci. Rev. 175, 53–92. doi:10.1007/s11214-013-9958-9
Reitz, G., Berger, T., and Matthiae, D. (2012). Radiation exposure in the moon environment. Planet. Space Sci. 74, 78–83. doi:10.1016/j.pss.2012.07.014
Richardson, I. G. (2018). Solar wind stream interaction regions throughout the heliosphere. Living Rev. Sol. Phys. 15, 1. doi:10.1007/s41116-017-0011-z
Riley, P., Baker, D., Liu, Y. D., Verronen, P., Singer, H., and Güdel, M. (2018). Extreme space weather events: From cradle to grave. Space Sci. Rev. 214, 21. doi:10.1007/s11214-017-0456-3
Riley, P., and Love, J. J. (2017). Extreme geomagnetic storms: Probabilistic forecasts and their uncertainties. Space weather. 15, 53–64. doi:10.1002/2016SW001470
Robinson, J., Saltus, R., and Liao, A. (2021). “Can a smartphone magnetometer capture space weather?,” in AGU fall meeting abstracts. ED35A–0556.
Russell, C. T., Ginskey, M., Petrinec, S., and Le, G. (1992). The effect of solar wind dynamic pressure changes on low and mid-latitude magnetic records. Geophys. Res. Lett. 19, 1227–1230. doi:10.1029/92GL01161
Scheffer, M., Carpenter, S., Foley, J. A., Folke, C., and Walker, B. (2001). Catastrophic shifts in ecosystems. Nature 413, 591–596. doi:10.1038/35098000
Schrijver, C. J., Kauristie, K., Aylward, A. D., Denardini, C. M., Gibson, S. E., Glover, A., et al. (2015). Understanding space weather to shield society: A global road map for 2015-2025 commissioned by cospar and ilws. Adv. Space Res. 55, 2745–2807. doi:10.1016/j.asr.2015.03.023
Semeter, J., Hunnekuhl, M., MacDonald, E., Hirsch, M., Zeller, N., Chernenkoff, A., et al. (2020). The mysterious green streaks below STEVE. AGU Adv. 1, e2020AV000183. doi:10.1029/2020AV000183
Shinbori, A., Tsuji, Y., Kikuchi, T., Araki, T., and Watari, S. (2009). Magnetic latitude and local time dependence of the amplitude of geomagnetic sudden commencements. J. Geophys. Res. 114. doi:10.1029/2008JA013871
Smith, B., Capon, C., and Brown, M. (2019). Ionospheric drag for satellite formation control. J. Guid. Control Dyn. 42, 2590–2599. doi:10.2514/1.G004404
Smith, C. W. (2022). Professional development, mentoring, and outreach in scientific research. Perspect. Earth Spac. 3, e2022CN000188. doi:10.1029/2022cn000188
Taber, A., and Normand, E. (1993). Single event upset in avionics. IEEE Trans. Nucl. Sci. 40, 120–126. doi:10.1109/23.212327
Temmer, M. (2021). Space weather: The solar perspective. Living Rev. Sol. Phys. 18, 4. doi:10.1007/s41116-021-00030-3
Thomson, A. W. P., McKay, A. J., Clarke, E., and Reay, S. J. (2005). Surface electric fields and geomagnetically induced currents in the Scottish Power grid during the 30 October 2003 geomagnetic storm. Space weather. 3, S11002. doi:10.1029/2005SW000156
Torta, J. M., Serrano, L., Regué, J. R., SáNchez, A. M., and RoldáN, E. (2012). Geomagnetically induced currents in a power grid of northeastern Spain. Space weather. 10, S06002. doi:10.1029/2012SW000793
Trivedi, N. B., Vitorello, C., Kabata, W., Dutra, S. L. G., Padilha, A. L., Bologna, M. S., et al. (2007). Geomagnetically induced currents in an electric power transmission system at low latitudes in Brazil: A case study. Space weather. 5, 04004. doi:10.1029/2006SW000282
Tsurutani, B. T., Gonzalez, W. D., Lakhina, G. S., and Alex, S. (2003). The extreme magnetic storm of 1-2 September 1859. J. Geophys. Res. 108, 1268. doi:10.1029/2002JA009504
Tsurutani, B. T., Lakhina, G. S., and Hajra, R. (2020). The physics of space weather/solar-terrestrial physics (STP): What we know now and what the current and future challenges are. Nonlin. Process. Geophys. 27, 75–119. doi:10.5194/npg-27-75-2020
Wang, K.-R., Liu, L.-G., and Li, Y. (2015). Preliminary analysis on the interplanetary cause of geomagnetically induced current and its effect on power systems. Chin. Astronomy Astrophysics 39, 78–88. doi:10.1016/j.chinastron.2015.01.003
Watari, S. (2015). Estimation of geomagnetically induced currents based on the measurement data of a transformer in a Japanese power network and geoelectric field observations. Earth Planets Space 67, 77. doi:10.1186/s40623-015-0253-8
Webb, D. F., and Howard, T. A. (2012). Coronal mass ejections: Observations. Living Rev. Sol. Phys. 9, 3. doi:10.12942/lrsp-2012-3
Wrenn, G. L., Rodgers, D. J., and Ryden, K. A. (2002). A solar cycle of spacecraft anomalies due to internal charging. Ann. Geophys. 20, 953–956. doi:10.5194/angeo-20-953-2002
Yue, P., Ramachandran, R., Baumann, P., Khalsa, S. J. S., Deng, M., and Jiang, L. (2016). Recent activities in Earth data science [technical committees]. IEEE Geosci. Remote Sens. Mag. 4, 84–89. doi:10.1109/MGRS.2016.2600528
Keywords: space weather, risk and resiliency, solar activity, solar storms, geospace, Sun and society, open data, open science
Citation: Ledvina VE, Palmerio E, McGranaghan RM, Halford AJ, Thayer A, Brandt L, MacDonald EA, Bhaskar A, Dong C, Altintas I, Colliander J, Jin M, Jain RN, Chatterjee S, Shaikh Z, Frissell NA, Chen TY, French RJ, Isola B, McIntosh SW, Mason EI, Riley P, Young T, Barkhouse W, Kazachenko MD, Snow M, Ozturk DS, Claudepierre SG, Di Mare F, Witteman A and Kuzub J (2022) How open data and interdisciplinary collaboration improve our understanding of space weather: A risk and resiliency perspective. Front. Astron. Space Sci. 9:1067571. doi: 10.3389/fspas.2022.1067571
Received: 12 October 2022; Accepted: 15 November 2022;
Published: 01 December 2022.
Edited by:
Jaroslav Chum, Institute of Atmospheric Physics (ASCR), CzechiaReviewed by:
Anna Belehaki, National Observatory of Athens, GreeceCopyright © 2022 Ledvina, Palmerio, McGranaghan, Halford, Thayer, Brandt, MacDonald, Bhaskar, Dong, Altintas, Colliander, Jin, Jain, Chatterjee, Shaikh, Frissell, Chen, French, Isola, McIntosh, Mason, Riley, Young, Barkhouse, Kazachenko, Snow, Ozturk, Claudepierre, Di Mare, Witteman and Kuzub. This is an open-access article distributed under the terms of the Creative Commons Attribution License (CC BY). The use, distribution or reproduction in other forums is permitted, provided the original author(s) and the copyright owner(s) are credited and that the original publication in this journal is cited, in accordance with accepted academic practice. No use, distribution or reproduction is permitted which does not comply with these terms.
*Correspondence: Vincent E. Ledvina, dmluY2VudGxlZHZpbmFAZ21haWwuY29t; Chuanfei Dong, ZGNmeUBwcmluY2V0b24uZWR1
Disclaimer: All claims expressed in this article are solely those of the authors and do not necessarily represent those of their affiliated organizations, or those of the publisher, the editors and the reviewers. Any product that may be evaluated in this article or claim that may be made by its manufacturer is not guaranteed or endorsed by the publisher.
Research integrity at Frontiers
Learn more about the work of our research integrity team to safeguard the quality of each article we publish.