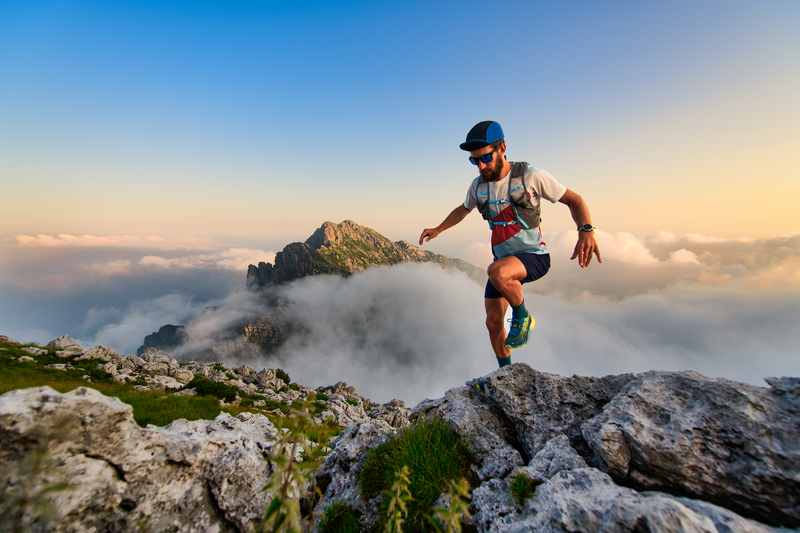
95% of researchers rate our articles as excellent or good
Learn more about the work of our research integrity team to safeguard the quality of each article we publish.
Find out more
PERSPECTIVE article
Front. Astron. Space Sci. , 15 November 2022
Sec. Space Physics
Volume 9 - 2022 | https://doi.org/10.3389/fspas.2022.1064152
This article is part of the Research Topic The Future of Space Physics 2022 View all 66 articles
The impact of regional-scale neutral atmospheric waves has been demonstrated to have profound effects on the ionosphere, but the circumstances under which they generate ionospheric disturbances and seed plasma instabilities are not well understood. Neutral atmospheric waves vary from infrasonic waves of <20 Hz to gravity waves with periods on the order of 10 min, for simplicity, hereafter they are combined under the common term Acoustic and Gravity Waves (AGWs). There are other longer period waves like planetary waves from the lower and middle atmosphere, whose effects are important globally, but they are not considered here. The most ubiquitous and frequently observed impact of AGWs on the ionosphere are Traveling Ionospheric Disturbances (TIDs), but AGWs also affect the global ionosphere/thermosphere circulation and can trigger ionospheric instabilities (e.g., Perkins, Equatorial Spread F). The purpose of this white paper is to outline additional studies and observations that are required in the coming decade to improve our understanding of the impact of AGWs on the ionosphere.
One of the main impacts of Acoustic and Gravity Waves (AGWs) on the thermosphere is the formation and development of Traveling Ionospheric Disturbances (TIDs) in the coexisting ionosphere, which has long been a challenging science question because of their immediate implications for communications, navigation and geolocation as well as the fact that they are an example of the dynamics and coupling between the thermosphere and the ionosphere. TIDs are wave-like propagating ionospheric density disturbances with horizontal scales sizes of 10–1,000 km. Some early observations of these structures were made in the 1950s (Munro, 1950; Munro and Heisler, 1956a, Munro and Heisler, 1956b), but the undetermined nature of their occurrence and lack of global measurements have complicated efforts to obtain a comprehensive understanding of how and why these features form and evolve.
The basic source of AGW driven TIDs is thought to be fairly well understood qualitatively: the TID is simply a signature of the wave disturbance in the neutral atmosphere. However, the evolution of the AGW from its source to thermospheric altitudes and the overall impact of the AGW properties on the ionosphere has not been properly quantified. Atmospheric waves have many natural and anthropogenic causes including: natural disasters (e.g., earthquakes, tsunamis, thunderstorms), nuclear detonation and other explosions, Joule heating from geomagnetic storms, and ocean sources (Djuth et al., 2010; Zabotin et al., 2016; Azeem and Barlage, 2018). In the case of AGWs driven from the Earth’s surface or troposphere, the waves are typically characterized as primary or higher order (e.g., secondary) depending on how they propagate to thermospheric altitudes. Primary AGWs propagate directly through the thermosphere and can be modeled using linear ray propagation theory. Higher order AGWs are created when primary AGWs break in the upper atmosphere; nonlinear propagation theory is required to simulate them (Vadas and Crowley, 2010). While there are documented cases of TIDs generated by primary gravity waves (Azeem et al., 2015; Huba et al., 2015; Chou et al., 2017a; Azeem and Barlage, 2018), recent studies have indicated that secondary and higher order gravity waves may be largely responsible for TIDs in the ionosphere (e.g., Vadas and Crowley, 2010; Fritts et al., 2018).
Another potential impact of AGWs on the ionosphere is the seeding of instabilities. While many TIDs are the direct result of AGW forcing, there is another type of medium scale TID that occurs at mid-latitudes, which is believed to be caused by the Perkins instability. One hypothesis is that AGWs provide the trigger for the Perkins instability, providing the necessary increase to the growth rates to generate TIDs (Chou et al., 2017b). AGWs have also been identified as a potential trigger for Equatorial Spread F (ESF) Bubbles, which are the result of a Rayleigh Taylor instability at equatorial latitudes (Krall et al., 2013; Aa et al., 2019). It is not known how frequently or under what conditions AGWs can trigger these instabilities in the ionosphere. Observational evidence of these connections has been limited thus far due to a lack of sufficient measurements.
While many of the effects of AGWs on the ionosphere are regional in nature, these local effects also contribute to the global redistribution of mass and energy in the thermosphere/ionosphere system. For example, Large Scale AGWs, which are driven by Joule heating from geomagnetic storms, are believed to be an important mechanism by which high latitude forcing modifies the low-latitude thermospheric density (Lu et al., 2016). In addition, since the resolution of many global thermosphere/whole-atmosphere models is not sufficient to resolve AGWs, their overall effect on thermospheric circulation is typically taken into account with parameterizations (Hines, 1960). As these parameterizations do not typically reflect the actual gravity wave spectrum for any given time, there are generally large uncertainties associated with them. Thus, the gravity wave parameterizations have been unable to fully quantify the effects of AGWs on the global ionosphere/system.
Understanding and predicting the physical mechanisms that drive regional ionospheric perturbations, such as TIDs, is a key challenge for the development of future space weather forecast systems. There have been recent efforts to mitigate the effects of TIDs on HF geolocation (Keller, 2012), but in order to predict TID occurrence, their generation mechanisms must be understood. The sparse nature of measurements in the ionosphere makes it unlikely that these questions can be answered via observational methods alone. Comprehensive modeling studies combined with new, multi-instrument observations will be required to obtain closure on these science questions.
What are the observable properties of TIDs driven from atmospheric waves? TIDs generated from different types of sources may exhibit similar or very different characteristics in their spatial and temporal scales, periodicities, propagation speeds and directions. Meanwhile, TIDs generated from the same type of sources may exhibit different characteristics depending on the local atmospheric or ionospheric conditions. Therefore, it remains a challenge to distinguish the generation mechanism of TIDs from ionospheric observations alone. Additional measurements and modeling studies are required to make progress on this issue.
What background conditions favor the generation and propagation of TIDs? Emerging model capabilities are demonstrating new techniques to understand the propagation of AGWs to the upper atmosphere. Early results indicate that the background thermosphere and ionosphere conditions contribute to the propagation of the AGWs and TIDs given that the amplitude of TIDs may in cases be directly proportional to the background electron density (Hunsucker, 1982). Future research should seek to better understand these conditions in order to evaluate the fidelity of different modeling techniques for atmospheric wave propagation.
How do the smaller scale atmospheric waves affect the global scale ionosphere/thermosphere density distribution? It is well known that AGWs can affect the overall thermospheric circulation. To account for these effects, neutral atmosphere or whole atmosphere models typically utilize a gravity wave parameterization (e.g., Liu et al., 2018). There are large uncertainties with this parameterization, however, and an obvious limitation is that they use a generic spectrum of gravity waves, rather than an AGW spectrum specific to a particular event (like thunderstorm convection), location (like orography) and season. Without realistic driving forces, only qualitative comparisons to the waves are possible.
When do AGW driven TIDs have electric fields and/or conjugate effects associated with them? Recent work has demonstrated that AGW driven TIDs can potentially induce a signature in the conjugate ionosphere (Huba et al., 2015; Jonah et al., 2017; Zettergren and Snively, 2019; Chou et al., 2022; Lin et al., 2022), similar to TIDs that are generated from the Perkins instability and E-F coupling. These AGW-related fluctuating polarization electric fields were also measured recently in the morning hours using the Millstone Hill incoherent scatter radar (Zhang et al., 2021). It is not understood how frequently these electrodynamic effects are observable and if they differ significantly from the electric fields associated with Perkins-instability type TIDs. In addition, gravity wave-driven polarization electric fields may contribute to the TID generation, but these effects are not well understood (Abdu et al., 2015).
How do the geopace storm magnetosphere-ionosphere-thermosphere (M-I-T) coupling process excite and impact the propagation of various TIDs? Although it is well established that the solar wind-magnetospheric energy, momentum, and particle depositions at high latitudes can excite AGWs and cause global propagation of large scale TIDs. Strong M-I-T coupling occurs at subauroral and midlatitude regions as well. Recent studies suggested that some of those midlatitude TIDs, in particular, MSTIDs, were highly correlated to subauroral electrodynamics and its potential impact on the ionospheric instabilities (Zhang et al., 2019). The subauroral electrodynamics is also connected to neutral atmospheric disturbances in winds and the associated dynamo action. This kind of storm-related MSTID studies have recently emerged, and many questions remain to be answered by observations and modeling regarding unique roles of storm-time electric fields and disturbance winds for the MSTID excitation and propagation.
How do AGWs contribute to the development of other ionospheric instabilities (e.g., Perkins instability, Equatorial Spread F)? AGWs are believed to provide triggering mechanisms for a number of different ionospheric instabilities. For example (Kelley and Makela, 2001), suggested that small-amplitude gravity waves with a phase velocity component in a particular direction can enhance the growth of the Perkins instability. While the Perkins instability coupled with sporadic E-region instabilities cause the electrified MSTIDs in midlatitude regions (Yokoyama, et al., 2009; Narayanan et al., 2018), the initial seed for such coupled instabilities is believed to be provided by AGWs. In addition, AGWs may contribute to the development of Equatorial Spread F, as well as high-latitude instabilities associated with polar cap patches and cusp irregularities. The circumstances and frequency with which these developments occur is currently unknown. Some studies have demonstrated similarities in the occurrence rate between atmospheric waves and instabilities (Takahashi et al., 2018; Takahashi et al., 2020), but additional observations and modeling is required to fully elucidate how and when AGWs seed ionospheric instabilities.
How predictable are AGWs and their effects on the ionosphere? While the potential sources of AGWs are fairly well known, the predictability of these sources and their impacts on the thermosphere and ionosphere have not yet been evaluated. For example, the spatial and temporal scales that are associated with predictable features are yet to be quantified. The effect of varying geomagnetic field orientations in constraining the interactions of AGWs and the ionosphere is not well understood. In addition, the conditions under which MSTIDs can propagate to different latitude regions needs further study.
Developing tools and metrics to evaluate model fidelity. It is imperative that the above science questions are accompanied by a definition of community tools that will be used to evaluate the fidelity of the models. The upper atmosphere community needs tools and metrics that can be used by different models, as in the numerical weather prediction communities. Many of the model validation studies thus far have been more qualitative. The community needs quantitative metrics that are connected with wave characteristics and potential operational requirements.
Significantly more observations are required to resolve these questions about how atmospheric waves affect the ionosphere. Many of the currently available direct observations of TIDs are from Global Navigation Satellite System (GNSS) signal measurements, which provide Total Electron Content (TEC) or scintillation (e.g., S4, ROTI indices) and airglow imaging observations of OI 630 nm emission during nighttimes. Since these measurements are integrated over the path between the receiver and satellite in the case of TEC and column integrated emissions in the case of airglow, they do not provide information on the vertical structure of the ionosphere or the TIDs. Existing studies indicate that there may be significant differences between TEC data and bottomside ionosphere electron density data, but significantly more measurements are needed. In addition, different measurement techniques have observed different aspects of the waves (Belehaki et al., 2020). In the ionosphere, additional measurements of electron density as a function of latitude, longitude and altitude; ion drifts and electric fields are required to make progress on this issue. This requirement can be supported by the operation of a dense network of ionosondes capable of performing soundings with a cadence less than 5 min. Another possibility is the Dynasonde technique, which provides more information about wave activity than standard ionosondes (Zabotin et al., 2017). Topside electron density observations from LEO satellites coincident with bottomside ionosonde measurements, can offer new possibilities in setting up a nowcasting system for the occurrence of ionospheric instabilities in the topside ionosphere based on the bottomside stratification (Belehaki et al., 2022).
In the neutral atmosphere, measurements of the winds, composition and temperature are crucial to resolve many of the science questions. So far, the only reasonable neutral parameters available with relative ease are the wind information around 250 km from the Fabry-Perot Interferometers, which provide information from column integrated airglow emissions. To properly understand the generation of TIDs, however, altitude-resolved measurements of neutral densities, temperature and winds are likely required. The Geospace Dynamics Constellation (Jaynes et al., 2019) will provide new in situ measurements from a series of distributed spacecraft near 400 km. During the initial phases, the spacecraft will be separated by hundreds of kilometers in similar high-latitude orbits. This will reveal new insight into the structure and propagation of TIDs. However, lower altitude measurements (100–200 km) are also required to capture the interplay between AGWs and TIDs where ion-neutral coupling is the strongest. Future missions with remote sensing of the winds and composition in this region (such as DYNAMIC) or low-altitude in situ measurements (such as ENLoTIS) can open up this new Frontier. Additionally, CubeSat missions can also play a role in exploring this region (Klenzing et al., 2020; Verkhoglyadova et al., 2021).
In the past decade, a number of high-resolution neutral models have been developed that can help answer these science questions. A partial list of these models includes MAGIC (Snively, 2017), HIAMCM (Becker and Vadas, 2020) and WACCM-X (Liu et al., 2018). These models have varying assumptions and techniques to model the evolution of the neutral atmospheric waves, but would allow for direct comparisons with observations, which is required to better understand the underlying physics. They can all be used to drive ionospheric models, which also allows direct comparisons with ionospheric quantities such as TEC and electron density (Inchin et al., 2021). By combining model studies with multi-instrument observations, significant progress can be made in resolving these questions in the next decade.
The original contributions presented in the study are included in the article/Supplementary Material, further inquiries can be directed to the corresponding author.
KZ wrote the first draft of the manuscript. All authors contributed to manuscript concept and revision, including reading and approving the submitted version.
JK and AH are supported through the Space Precipitation Impacts working group at Goddard Space Flight Center. VLN is supported by the NERC, United Kingdom grant NE/V01837X/1.
The authors acknowledge support from the International Space Science Institute as part of the “Resolving the Generation Mechanisms and Electrodynamical Effects of Medium Scale Traveling Ionospheric Disturbances (MSTIDs)” working group. KZ, MB, MD, DH, and FS acknowledge funding from the Office of Naval Research. AB acknowledges financial support provided by the AFRL grant award FA9550-19-1-7019 and by the PITHIA-NRF Horizon 2020 Grant Agreement 101007599 of the European Commission.
The authors declare that the research was conducted in the absence of any commercial or financial relationships that could be construed as a potential conflict of interest.
All claims expressed in this article are solely those of the authors and do not necessarily represent those of their affiliated organizations, or those of the publisher, the editors and the reviewers. Any product that may be evaluated in this article, or claim that may be made by its manufacturer, is not guaranteed or endorsed by the publisher.
Aa, E., Zou, S., Eastes, R., Karan, D. K., Zhang, S.-R., Erickson, P. J., et al. (2019). Coordinated ground‐based and space‐based observations of equatorial plasma bubbles. JGR. Space Phys. 125, 2019JA027569. doi:10.1029/2019JA027569
Abdu, M. A., de Souza, J. R., Kherani, E. A., Batista, I. S., MacDougall, J. W., and Sobral, J. H. A. (2015). Wave structure and polarization electric field development in the bottomside F layer leading to postsunset equatorial spread F. J. Geophys. Res. Space Phys. 120, 6930–6940. doi:10.1002/2015JA021235
Azeem, I., and Barlage, M. (2018). Atmosphere-ionosphere coupling from convectively generated gravity waves. Adv. Space Res. 61 (7), 1931–1941. doi:10.1016/j.asr.2017.09.029
Azeem, I., Yue, J., Hoffmann, L., Miller, S. D., Straka III, W. C., and Crowley, G. (2015). Multisensor profiling of a concentric gravity wave event propagating from the troposphere to the ionosphere. Geophys. Res. Lett. 42, 7874–7880. doi:10.1002/2015GL065903
Becker, E., and Vadas, S. L. (2020). Explicit global simulation of gravity waves in the thermosphere. JGR. Space Phys. 125, e2020JA028034. doi:10.1029/2020JA028034
Belehaki, A., Tsagouri, I., Altadill, D., Blanch, E., Borries, C., Buresova, D., et al. (2020). An overview of methodologies for real-time detection, characterisation and tracking of traveling ionospheric disturbances developed in the TechTIDE project. J. Space Weather Space Clim. 10, 42. doi:10.1051/swsc/2020043
Belehaki, A., Tsagouri, I., and Paouris, E. (2022). Characteristics of the effective scale height in the topside ionosphere extracted from Swarm A and Digisonde observations: Preliminary results. JGR. Space Phys. 127, e2021JA030075. doi:10.1029/2021JA030075
Chou, M.-Y., Lin, C. C. H., Yue, J., Tsai, H. F., Sun, Y. Y., Liu, J. Y., et al. (2017a). Concentric traveling ionosphere disturbances triggered by Super Typhoon Meranti (2016). Geophys. Res. Lett. 44, 1219–1226. doi:10.1002/2016GL072205
Chou, M.-Y., Lin, C. H., Yue, J., Chang, L. C., Tsai, H. F., Chen, C. H., et al. (2017b). Medium-scale traveling ionospheric disturbances triggered by Super Typhoon Nepartak (2016). Geophys. Res. Lett. 44, 7569–7577. doi:10.1002/2017GL073961
Chou, M.-Y., Yue, J., Lin, C. C. H., Rajesh, P. K., and Pedatella, N. M. (2022). Conjugate effect of the 2011 Tohoku reflected tsunami-driven gravity waves in the ionosphere. Geophys. Res. Lett. 49, e2021GL097170. doi:10.1029/2021GL097170
Djuth, F. T., Zhang, L. D., Livneh, D. J., Seker, I., Smith, S. M., Sulzer, M. P., et al. (2010). Arecibo’s thermospheric gravity waves and the case for an ocean source. J. Geophys. Res. 115, A08305. doi:10.1029/2009JA014799
Fritts, D. C., Laughman, B., Wang, L., Lund, T. S., and Collins, R. L. (2018). Gravity wave dynamics in a mesospheric inversion layer: 1. Reflection, trapping, and instability dynamics. JGR. Atmos. 123, 626–648. doi:10.1002/2017JD027440
Hines, C. O. (1960). Internal atmospheric gravity waves at ionospheric heights. Can. J. Phys. 38, 1441–1481. doi:10.1139/p60-150
Huba, J. D., Drob, D. P., Wu, T.-W., and Makela, J. J. (2015). Modeling the ionospheric impact of tsunami-driven gravity waves with SAMI3: Conjugate effects. Geophys. Res. Lett. 42, 5719–5726. doi:10.1002/2015GL064871
Hunsucker, R. D. (1982). Atmospheric gravity waves generated in the high-latitude ionosphere: a review. Rev. Geophys. 20 (2), 293–315. doi:10.1029/RG020i002p00293
Inchin, P. A., Snively, J. B., Kaneko, Y., Zettergren, M. D., and Komjathy, A. (2021). Inferring the evolution of a large earthquake from its acoustic impacts on the ionosphere. AGU Adv. 2, e2020AV000260. doi:10.1029/2020AV000260
Jaynes, A., Ridley, A., Bishop, R., Heelis, R., Zesta, E., Anderson, B., et al. (2019). NASA science and technology definition team for the geospace dynamics constellation final report. Available at: https://science.nasa.gov/heliophysics/resources/stdts/geospace-dynamics-constellation.
Jonah, O. F., Kherani, E. A., and De Paula, E. R. (2017). Investigations of conjugate MSTIDS over the Brazilian sector during daytime. J. Geophys. Res. Space Phys. 122, 9576–9587. doi:10.1002/2017JA024365
Keller, J. (2012). HFGeo ELINT program to pinpoint HF transmitters extends to advanced ionospheric modeling. Available at: https://www.militaryaerospace.com/computers/article/16719873/hfgeo-elint-program-to-pinpoint-hf-transmitters-extends-to-advanced-ionospheric-modeling (Accessed September 30, 2012).
Kelley, M. C., and Makela, J. J. (2001). Resolution of the discrepancy between experiment and theory of midlatitude f-region structures. Geophys. Res. Lett. 28 (13), 2589–2592. doi:10.1029/2000gl012777
Klenzing, J., Davidson, R. L., Jones, S. L., Martinis, C., Zawdie, K. A., Earle, G. D., et al. (2020). The petitSat mission – science goals and instrumentation. Adv. Space Res. 66 (1), 107–115. doi:10.1016/j.asr.2019.12.013
Krall, J., Huba, J. D., and Fritts, D. C. (2013). On the seeding of equatorial spread F by gravity waves. Geophys. Res. Lett. 40, 661–664. doi:10.1002/grl.50144
Lin, J.-T., Rajesh, P. K., Lin, C. C. H., Chou, M.-Y., Liu, J.-Y., Yue, J., et al. (2022). Rapid conjugate appearance of the giant ionospheric Lamb wave signatures in the northern hemisphere after Hunga-Tonga volcano eruptions. Geophys. Res. Lett. 49, e2022GL098222. doi:10.1029/2022GL098222
Liu, H.-L., Bardeen, C. G., Foster, B. T., Lauritzen, P., Liu, J., Lu, G., et al. (2018). Development and validation of the whole atmosphere community climate model with thermosphere and ionosphere extension (WACCM-X 2.0). J. Adv. Model. Earth Syst. 10, 381–402. doi:10.1002/2017MS001232
Lu, G., Richmond, A. D., Lühr, H., and Paxton, L. (2016). High-latitude energy input and its impact on the thermosphere. JGR. Space Phys. 121, 7108–7124. doi:10.1002/2015JA022294
Munro, G. H., and Heisler, L. H. (1956a). Cusp type anomalies in variable frequency ionospheric records. Aust. J. Phys. 9, 343–358. doi:10.1071/PH560343
Munro, G. H., and Heisler, L. H. (1956b). Divergence of radio rays in the ionosphere. Aust. J. Phys. 9, 359–372. doi:10.1071/PH560359
Narayanan, V. L., Shiokawa, K., Otsuka, Y., and Neudegg, D. (2018). On the role of thermospheric winds and sporadic E layers in the formation and evolution of electrified MSTIDs in geomagnetic conjugate regions. J. Geophys. Res. Space Phys. 123, 6957–6980. doi:10.1029/2018JA025261
Snively, J. B. (2017). Nonlinear gravity wave forcing as a source of acoustic waves in the mesosphere, thermosphere, and ionosphere. Geophys. Res. Lett. 44, 12020–12027. doi:10.1002/2017GL075360
Takahashi, H., Wrasse, C. M., Figueiredo, C. A. O. B., Barros, D., Abdu, M. A., Otsuka, Y., et al. (2018). Equatorial plasma bubble seeding by MSTIDs in the ionosphere. Prog. Earth Planet. Sci. 5, 32. doi:10.1186/s40645-018-0189-2
Takahashi, H., Wrasse, C. M., Figueiredo, C. A. O. B., Barros, D., Paulino, I., Essien, P., et al. (2020). Equatorial plasma bubble occurrence under propagation of MSTID and MLT gravity waves. J. Geophys. Res. Space Phys. 125, e2019JA027566. doi:10.1029/2019JA027566
Vadas, S. L., and Crowley, G. (2010). Sources of the traveling ionospheric disturbances observed by the ionospheric TIDDBIT sounder near Wallops Island on 30 October 2007. J. Geophys. Res. 115, A07324. doi:10.1029/2009JA015053
Verkhoglyadova, O. P., Bussy-Virat, C. D., Caspi, A., Jackson, D. R., Kalegaev, V., Klenzing, J., et al. (2021). Addressing gaps in space weather operations and understanding with small satellites. Space weather 19, e2020SW002566. doi:10.1029/2020SW002566
Yokoyama, T., Hysell, D. L., Otsuka, Y., and Yamamoto, M. (2009). Three‐dimensional simulation of the coupled Perkins and Es‐layer instabilities in the nighttime midlatitude ionosphere. J. Geophys. Res. 114, A03308. doi:10.1029/2008JA013789
Zabotin, N. A., Godin, O. A., and Bullett, T. W. (2016). Oceans are a major source of waves in the thermosphere. J. Geophys. Res. Space Phys. 121 (4), 3452–3463. doi:10.1002/2016JA022357
Zabotin, N., Godin, O. A., Negrea, C., Bullett, T., and Zabotina, L. (2017). Studies of wave activity in the thermosphere-ionosphere system using Dynasonde techniques, XXXIInd general assembly and scientific symposium of the international union of radio science (URSI GASS). Montreal, QC: IEEE Xplore.
Zettergren, M. D., and Snively, J. B. (2019). Latitude and longitude dependence of ionospheric TEC and magnetic perturbations from infrasonic-acoustic waves generated by strong seismic events. Geophys. Res. Lett. 46, 1132–1140. doi:10.1029/2018GL081569
Zhang, S.-R., Erickson, P. J., Coster, A. J., Rideout, W., Vierinen, J., Jonah, O., et al. (2019). Subauroral and polar traveling ionospheric disturbances during the 7-9 september 2017 storms. Space weather 17 (1), 1748–1764. doi:10.1029/2019SW002325
Keywords: ionosphere, thermosphere, plasma irregularities, space physics, waves
Citation: Zawdie K, Belehaki A, Burleigh M, Chou M-Y, Dhadly MS, Greer K, Halford AJ, Hickey D, Inchin P, Kaeppler SR, Klenzing J, Narayanan VL, Sassi F, Sivakandan M, Smith JM, Zabotin N, Zettergren MD and Zhang S-R (2022) Impacts of acoustic and gravity waves on the ionosphere. Front. Astron. Space Sci. 9:1064152. doi: 10.3389/fspas.2022.1064152
Received: 07 October 2022; Accepted: 24 October 2022;
Published: 15 November 2022.
Edited by:
Guozhu Li, Institute of Geology and Geophysics (CAS), ChinaCopyright © 2022 Zawdie, Belehaki, Burleigh, Chou, Dhadly, Greer, Halford, Hickey, Inchin, Kaeppler, Klenzing, Narayanan, Sassi, Sivakandan, Smith, Zabotin, Zettergren and Zhang. This is an open-access article distributed under the terms of the Creative Commons Attribution License (CC BY). The use, distribution or reproduction in other forums is permitted, provided the original author(s) and the copyright owner(s) are credited and that the original publication in this journal is cited, in accordance with accepted academic practice. No use, distribution or reproduction is permitted which does not comply with these terms.
*Correspondence: Kate Zawdie, a2F0ZS56YXdkaWVAbnJsLm5hdnkubWls
Disclaimer: All claims expressed in this article are solely those of the authors and do not necessarily represent those of their affiliated organizations, or those of the publisher, the editors and the reviewers. Any product that may be evaluated in this article or claim that may be made by its manufacturer is not guaranteed or endorsed by the publisher.
Research integrity at Frontiers
Learn more about the work of our research integrity team to safeguard the quality of each article we publish.