- 1Center for Astrophysics, Harvard & Smithsonian, Cambridge, MA, United States
- 2NASA GSFC, Greenbelt, MD, United States
- 3Climate and Space Science and Engineering (CLaSP), University of Michigan, Ann Arbor, MI, United States
- 4Southwest Research Institute, San Antonio, TX, United States
- 5Department of Astrophysical and Planetary Sciences, University of Colorado, Boulder, CO, United States
- 6U.S. Naval Research Laboratory (NRL), Washington, DC, United States
- 7Predictive Sciences Inc., San Diego, CA, United States
- 8Space Sciences Laboratory, University of California Berkeley, Berkeley, CA, United States
- 9Fu Foundation School of Engineering and Applied Science, Columbia University, New York City, NY, United States
This paper outlines key scientific topics that are important for the development of solar system physics and how observations of heavy ion composition can address them. The key objectives include, 1) understanding the Sun’s chemical composition by identifying specific mechanisms driving elemental variation in the corona. 2) Disentangling the solar wind birthplace and drivers of release by determining the relative contributions of active regions (ARs), quiet Sun, and coronal hole plasma to the solar wind. 3) Determining the principal mechanisms driving solar wind evolution from the Sun by identifying the importance and interplay of reconnection, waves, and/or turbulence in driving the extended acceleration and heating of solar wind and transient plasma. The paper recommends complementary heavy ion measurements that can be traced from the Sun to the heliosphere to properly connect and study these regions to address these topics. The careful determination of heavy ion and elemental composition of several particle populations, matched at the Sun and in the heliosphere, will permit for a comprehensive examination of fractionation processes, wave-particle interactions, coronal heating, and solar wind release and energization that are key to understanding how the Sun forms and influences the heliosphere.
1 Science motivation
Since the start of space exploration in the 1960s, solar probes have measured plasma on a multitude of spatial and temporal scales at several locations around our solar system. However, we still lack a fundamental understanding of the transfer of mass and energy from the Sun to interplanetary space. Initially, mainly H and He ions were observed through the Mariner and Pioneer missions between the 1960s and 1970s. However, the launch of the ISEE-8 in 1978 and the SWICS instruments that were flown on both Ulysses and ACE as well as the CTOF on SOHO from the 1990s has enabled measurements of the most abundant minor ions in the solar wind (C, N, O, and Fe). Several decades of heavy ion measurements have provided crucial insight to the solar wind’s source region and inner heliosphere conditions that continue to shed light on fundamental questions about our star.
Key scientific questions that are important for the development of solar system physics, see Viall & Borovsky (2020) and Viall and Borovsky (2022) decadal survey white paper in connection to this paper.
1) What drives the compositional variation across coronal structures and their associated solar wind streams?
2) What are the specific drivers of solar wind release? What are the relative contributions of active regions (ARs), quiet Sun, and coronal holes to the solar wind?
3) What is the contribution and interplay of reconnection, waves, and/or turbulence driving the extended acceleration and heating of solar wind and transient plasma?
To effectively address these open questions, it is necessary to determine the thermodynamic state of solar material at its source, as it is released, and as it escapes the corona to allow for a full characterization of its formation and energization as it travels away from the Sun. Significant progress in these open science topics requires effective coupling of physically disparate regions of the solar atmosphere and heliosphere to build a complete picture of the physical mechanisms driving the continuous creation and outflow of solar wind. Tracing the active processes at different stages of evolution will require connecting observations at the solar surface, across the middle corona, and beyond (West, 2022).
Heavy ions are excellent probes to investigate the creation and evolution of solar plasma because they are highly sensitive to processes connected to the solar wind’s birth region, generation, and subsequent evolution (Cranmer & Winebarger 2019; West, 2022). Elemental and ion composition measured at the Sun and throughout the solar system can be useful to 1) connect solar and heliospheric structures, 2) provide sensitive diagnostics for the solar wind thermodynamic evolution, and 3) reveal key thermal and non-thermal processes taking place at the Sun and as the solar wind accelerates through the corona into interplanetary space. The observations will be highly beneficial to evaluating models of the corona and solar wind which remain under-constrained due to the lack of necessary observations at critical stages of formation, heating, and acceleration. Correspondingly, a more comprehensive understanding of ionization and recombination processes, characterization of collisional and radiative coronal emission, as well as fractionation processes at the Sun, are informed by the development of more detailed coronal magnetic field extrapolations and physics-based models of the corona/solar wind.
2 Heavy ions as tracers of solar origin and heating/acceleration processes in the Corona and solar wind
Elemental Composition Decades of observations have shown that the elemental composition of the corona within different structures can vary both spatially and temporally. The key processes attributed to changes in the chemical makeup of the corona are from the first ionization potential (FIP) effect, theorized to occur mainly at the chromospheric-coronal boundary where the solar atmosphere is partially ionized, and gravitational settling that can be exhibited in large coronal loops higher in the corona (Raymond et al 1997; Laming 2015). Through the FIP effect, coronal plasma is observed to be preferentially enhanced of “low” FIP elements (FIP <10eV) while having little to no changes in its “high” FIP (FIP >10 eV) elemental composition when compared to photospheric abundances. At the Sun, the FIP effect is most prominently observed in closed magnetic field structures such as ARs and quiet Sun, with the most extreme FIP values occurring in ARs, while nearly absent in open field structures, i.e., coronal holes. From in situ observations in the heliosphere, low-FIP enhanced slow speed wind has been linked to plasma originating in and near closed field structures, while persistently photospheric fast speed wind has been connected to coronal holes (Geiss et al 1995; von Steiger et al 2000). However, while we can generally specify the solar wind’s solar source, current backmapping capabilities cannot directly link the inherently time-dynamic processes of reconnection-released closed-field plasma with observations of the solar wind. Therefore, connection science and mapping techniques could be strengthened by including properties of the plasma’s chemical composition.
A leading theory to describe FIP fractionation is the ponderomotive force (Laming 2015). As detailed in Laming (2004), Laming (2012), Laming (2017), Laming (2022), a ponderomotive force directed up toward the corona can develop through the reflection and refraction of Alfvén waves at the chromosphere-corona boundary due to a steep gradient in the density. The ponderomotive force driven by these waves can act on ions, but not neutral particles, resulting in the preferential transport of elements with a lower ionization threshold into the corona, which establishes the FIP effect. In most recent studies, simulations implementing the ponderomotive force have shown that elements with a FIP value straddling the “low” and “high” FIP boundary (10eV) could provide a more sensitive constraint to solar wind origin and properties of its magnetic topology (Laming 2022). Detailed simulations of the ponderomotive force examining a range of wave energy fluxes in open and closed field structures find that the behavior of Sulfur, Carbon, and Phosphorous are predicted to shift between “high” or “low” FIP depending on the magnetic topology, magnetic field strength, and the thermodynamic state of its source. It is found that insignificant fractionation arises in S and C in coronal loops, similarly to the “high” FIP elements, while they behave like “low” FIP elements in plasma emanating from an open field. A careful determination of elemental composition of several particle populations, matched at the Sun and in the heliosphere, will allow an examination of the ponderomotive force and its connection to fractionation processes, wave-particle interactions, and coronal heating that are key to understanding coronal dynamics (Dahlburg et al 2017; Tarr 2017; Laming 2022) and provide a fundamental link to solar wind origin and formation.
Another potentially relevant fractionation effect observed at the Sun is from gravitational settling. Several studies of the elemental composition higher in the corona have found that large, long-lived helmet streamer loops can become depleted of several heavy elements, with timescales on the order of ∼1 day (Raymond et al 1997; Lenz et al., 1998). Gravitational settling manifests as mass stratified plasma in helmet streamers where their core is strongly depleted of heavy elements with increasing coronal altitude. This effect is independent of the particle’s FIP value (Feldman et al 1998; Uzzo et al 2003; Uzzo et al 2004). Heavy elemental abundance “dropouts” in connection to those reported at the Sun have also been identified in the slow speed solar wind and coronal mass ejections with in situ observations (Weberg et al 2012; Lepri and Rivera 2021; Rivera et al 2022). These dropouts, together with the FIP effect, can modify the chemical makeup of specific solar structures up to the tops of loops ∼2.5 Rsun) in the closed-field corona prior to plasma release, making them key signatures of solar wind origin and coronal lifetime important to constraining fractionation processes.
Heavy Ion Composition To understand the physical drivers behind the formation and continuous outflow of solar wind, it is essential to understand the dynamic changes in temperature, density, and outflow velocity of the plasma across the coronal-heliospheric system. As such, the plasma’s ion composition can be examined to determine the plasma’s thermodynamic state throughout its initial release and propagation away from the Sun (Kohl et al., 1997; Habbal et al., 2010a; Habbal et al., 2010b; Landi et al., 2012; Gilly & Cranmer 2020). This is because the ionization and recombination history of ions evolving in solar wind streams are governed by collisions with electrons which make them highly sensitive to the electron temperature, the electron density, and the bulk speed of the expanding plasma parcel. Within a few radii from the Sun, the density becomes too low for further ionization or recombination to continue, after which ions decouple from further heating or cooling experienced by the plasma. At the so-called “freeze-in” point, ions remain unchanged, and the ionization state of ions is uniquely shaped by the plasma’s thermodynamic evolution up to that point, as shown for several ions evolving within an expanding coronal hole in Figure 1. Given that each ion undergoes a distinctive ionization and recombination history, relative abundances of individual ions provide sensitive constraints to the heating, acceleration, and expansion of the solar wind during the early stages of its evolution prior to “freeze-in”. This evolution has been demonstrated with multi-wavelength coronal observations during solar eclipses that map Fe charge states from 1 to 3 Rsun enabling more detailed characterization of the corona and solar wind’s thermal structure (Habbal et al 2010a; Habbal et al 2010b; Boe et al 2018).
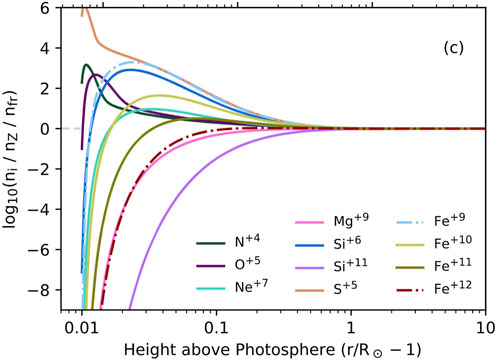
FIGURE 1. Simulation of the relative abundances to freeze-in value of several ions evolving within a coronal hole, adapted from Gilly and Cranmer, 2020.
Previous work has indicated that the inclusion of time-dependent ionization and recombination of heavy ions can provide a useful observational constraint to models of the solar wind and transients through the comparison of both remote sensing (i.e., synthetic multi-wavelength emission) and in situ observations (i.e., simulated freeze-in charge state distributions) as shown through simulated intensities and particle abundances in the top and bottom of Figure 2, respectively (Oran et al 2015; Shen et al 2017; Lionello et al 2019; Szente et al 2022). Simulations of heavy ion evolution in solar eruptions have provided critical insight to the energy deposition and non-uniform heating experienced in coronal mass ejections (CMEs) essential to constraining the energy budget (Rakowski et al 2007; Lynch et al 2011; Gruesbeck et al 2011, 2012; Rivera et at 2019a; Rivera et at 2019b) as well as important in determining the source region and dynamic processes occurring during solar wind outflow (Kohl et al., 1997; Gilly & Cranmer 2020; Rivera et al 2020). For example, anomalous signatures of heavy ion ratios (C6+/C5+) have been utilized to distinguish between waves and reconnection mechanisms as the primary source of heating and acceleration of the solar wind (Raymond et al., 2022). In another study, work examining the conditions for interchange reconnection cited the evolution of O7+/O6+ as a key marker in the ambient versus transient creation of solar wind (Scott et al 2022). Measurements of the carbon and oxygen signatures can enable further characterization of solar sources to quantify and disentangle sources of slow speed solar wind using heavy ion composition. Additionally, it can act as a distinctive compositional tracer of the magnetic origin and topology of structures measured in situ such as those for recently discovered magnetic switchbacks, or magnetic reversals, in the inner heliosphere by Parker Solar Probe (PSP) whose origin and formation remain actively debated (Bale et al 2019; Kasper et al 2019).
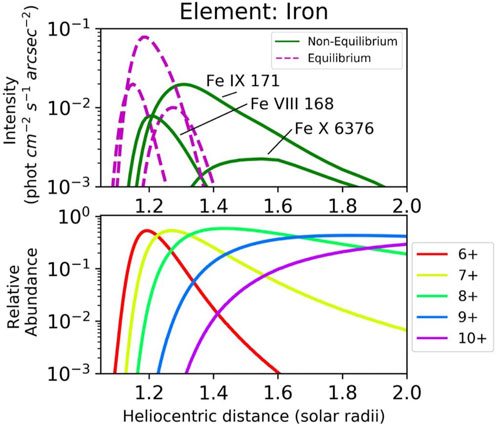
FIGURE 2. (Top) simulated intensities for equilibrium and non-equilibrium conditions and, (bottom) relative abundaces under non-equilibrium conditions within radially evolving solar plasma, adapted from Rivera et al., 2019b.
Non-thermal Solar Wind Processes There is strong evidence that departure from thermodynamic equilibrium among ions and electrons is established at low altitudes in coronal holes at the Sun (Kohl et al., 1997; Kohl et al., 1998; Landi and Cranmer 2009, and references discussed below). This suggests that non-thermal processes are closely linked to the heating and acceleration of particles out of the corona. The kinetic properties of heavy ions offer a unique window to signatures of the heating and acceleration mechanism(s) in the solar plasma that can bring insight to the governing processes taking place. For instance, velocity distribution functions (VDFs) of ions observed in situ can provide clues of non-thermal processes, such as wave-particle resonances, that develop during different stages of solar wind outflow (Cranmer et al 2017 and references therein). Most recently, in situ observations from PSP found signatures of cyclotron resonant heating in the solar wind that suggests this process could be a dominant plasma heating mechanism well beyond the low corona, proving a model to explain the observed ion kinetic properties (Bowen et al., 2022). Ion cyclotron resonant heating is a leading theory aimed at explaining the apparent quasi mass-per-charge dependency observed in the temperature and velocities of heavy ions near the Sun and in the solar wind (Esser et al 1999; Cranmer et al 1999; Landi and Cranmer 2009; Tracy et al., 2016; Berger et al., 2011). However, there may be other processes that could potentially explain those observations as well (Chandran et al., 2010). In recent studies, a statistical analysis of switchbacks suggested that their relaxation from rotational to tangential discontinuities with increasing heliocentric distance could be a source of heating and acceleration in the solar wind (Akhavan-Tafti et al 2022).
A major hindrance in the effective discrimination or associated contribution among these processes is that these studies have been carried out with remote and in situ observations that are entirely disconnected and lack continuous assessment beyond the spectrometer’s field of view or single point in the heliosphere. Significant progress in determining where, when, and how these properties arise during the radial flow, and if all solar wind types and transients behave in the same manner, can only be reached by following the progression of these processes in the plasma using coordinated remote and in situ observations.
3 Current obstacles
Current missions are attempting to close the gap between corona and heliosphere, but they continue to lack the spatial and continuous coverage of ion measurements required to address the open questions. PSP is flying through the outer tendrils of the corona to take in situ measurements of the Sun’s outer atmosphere; however, it does not carry a heavy ion composition instrument for detailed compositional studies. Solar Orbiter (SolO) carries a composition payload, the Heavy Ion Sensor (SolO/HIS) on the Solar Wind Analyser suite, however it is the only operational heavy ion composition instrument measuring the thermal solar wind in the entire inner heliosphere. In connection to ion abundances made by SolO/HIS, SolO’s SPectral Imaging of the Coronal Environment (SPICE) instrument takes spectroscopic observations of the solar disk with the objective of mapping chemical abundances between the Sun and heliosphere. However, because these two instruments are on the same spacecraft, they are not in the optimum position to effectively connect remote and in situ observations of the same source region. Therefore, while both the PSP and SolO missions sample the inner heliosphere and SolO can observe the Sun directly, their instrumentation and configuration severely limits the coordinated, long-term observations of heavy ions that can be made.
Another key aspect is that spectrometers are tuned to specific spectral ranges with limited spectral lines from only a small variety of ions at specific temperatures. In situ heavy ion composition instruments measure ions that are most abundant and most easily separated in energy-per-charge. Because of the limited overlap of the ions covered between a single remote sensing instrument and in situ instruments, 1) it inhibits a direct comparison of a large number of elemental abundances between them, and 2) largely limit comprehensive studies of composition with spectrometers to hot, dense regions of the Sun ,i.e., ARs and helmet streamers.
4 Necessary observations to meet science goals
Long-term, Matched, and Consistent Heavy Ion Measurements in the Corona and Heliosphere For a comprehensive understanding of the solar wind’s method of release and its energization, it is critical to make continuous, matched, long-term compositional measurements between the Sun and the interplanetary medium. Relevant mechanisms operate at a large range of spatial and temporal scales, from characteristic timescales of wave-particle interaction to the 11-years solar cycle and beyond, all of which are important to build a global picture of the Sun and associated solar processes. However, up to the present, there is only a small window, limited opportunities, and ecliptic-based measurements (except for Ulysses reaching higher latitudes) in which solar observatories assemble in quadrature to connect remote and in situ observations. The intermittent way this configuration occurs inhibits an in-depth temporal analysis of regions on the Sun to examine trends and signatures that develop at its source and during the plasma’s radial evolution. The lack of consistent and dedicated observations that can be linked between the Sun and heliosphere has delayed progress.
Dedicated and Complementary Remote and in situ Measurements that can be Meaningfully Compared To properly examine element and ion abundances and their variation across solar structures and solar wind streams, it is necessary to measure the same element and charge states at the Sun, the corona, and the heliosphere. As discussed in Section 2 (Elemental Composition), S has been identified as a sensitive diagnostic of fractionation processes occurring at the Sun which can provide a distinctive signature to the magnetic topology and possible release of the emerging plasma (Laming 2022). However, there are several limitations when examining S and other abundances across solar structures in the corona in connection to the solar wind. For current in situ instruments, it is difficult to determine the heliospheric densities of S ions with certainty using current time-of-flight (TOF) mass spectrometer technology. Several less abundant S ions have TOFs and energies that are close to those of Mg, Si, and Fe, making it difficult to cleanly separate their contributions. However, spectral observations such as those from EIS on Hinode, routinely use S lines to examine coronal composition, most notably high-to low-FIP S X to Si X ratio, in structures at the Sun (Brooks & Warren 2011; Baker et al 2013; Brooks et al 2015; Stansby et al 2020; Baker et al 2022). This ambiguity affects the meaningful comparison between the solar wind and its solar source in two important ways: 1) We are unable to confidently compare changes of S’s chemical composition together with other high- and low-FIP species at the Sun that may also be influenced by fractionation processes. 2) We are unable to effectively compare the S composition of the emerging plasma to its counterpart in the heliosphere. The large gap in our ability to compare overlapping heavy ion properties between the remote and in situ communities can lead to significant ambiguities and misinterpretation in scientific results. Therefore, along with advances in backmapping and connection science modeling techniques, there is a critical need for complementary heavy ion measurements between the Sun and heliosphere to properly connect and study these regions.
5 Strategy and action points
In the next decade and beyond, the solar and heliospheric community should aim to understand the source, release, and energization of the ambient and transient solar wind. This requires the consistent and effective coupling of different regions of the Sun and heliosphere that includes tracking parcels across the coronal-heliospheric system, by way of advancements in remote and in situ diagnostic techniques and technologies coupled with advances in modeling/theory.
5.1 Specific goals for the future
1) High throughput multi-wavelength UV, EUV, visible, and near-infrared spectroscopy to measure the time variation of FIP fractionation in ARs, flares, and throughout the corona that cover a complementary range of low-FIP and high-FIP elements, including S and C, with in situ instruments.
a) EUV (−10–50 nm): offers on-disk abundance measurements through bright low-FIP emission from Fe, in several stages of ionization, as well as Si and strong high-FIP emission from S as observed by Hinode/EIS (Brooks & Warren 2011).
b) UV (−50–200 nm): offers the largest coverage of high- and low-FIP emission for on and off-disk compositional measurements with strong lines of H, He, N, O, Ne, Mg, Al, Si, S, Ar, Ca, Fe, and Ni with two or more stages of ionization from Mg, Si, S, and Fe as observed by SOHO/UVCS and SolO’s SPICE (Raymond et al 1997; Brooks et al 2022). Additionally, the inclusion of the strong H I Lyman alpha line makes it possible to obtain absolute, rather than just relative abundances. Also, the neutral lines of elements such as H, He, and O together with lines of highly ionized species such as Fe XVIII through Fe XXII mean that the UV range spans a thermal range that can measure cool prominence ejecta along with 10 MK flared heating CME material.
c) Visible/near-Infrared (−200–5000 nm): offers off-disk coronal compositional diagnostics that include strong low-FIP emission from Fe, Si, Ni, and Ca and high-FIP emission from Ar and S for a set of lines with two or more ionization stages as observed during total solar eclipses and with ground-based observatories (Tomczyk et al 2008; Habbal et al 2010a; 2010b; Steven et al., 2021; Boe et al 2021). Of particular interest will be the recently deployed Upgraded COronal Multi-channel Polarimeter (UCoMP, high corona; Landi et al 2016) and Daniel K. Inouye Solar Telescope (DKIST, low corona; Rimmele et al. 2020) ground observatories that will offer more routine coverage of several visible and near-infrared lines in the corona. UCoMP is a ground-based, multi-wavelength coronagraph observing Fe X, XI, XIII (line pair), XIV, XV, and Ar XI, H alpha and He I 1083 nm lines near-simultaneously out to 2Rsun. DKIST is a five-instrument, ground-based observatory that will enable a highly detailed view of the photosphere, chromosphere, and corona. Low, off-limb observations of a small region of the corona are planned through the Cryogenic Near-IR Spectro-Polarimeter (Cyro-NIRSP) instrument. Cryo-NIRSP is planned to observe the Fe XIII line pair, He I 1083 nm, Si IX, and Si X that will be highly synergistic with UCoMP observations.
2) Charge-resolving heavy ion mass spectrometer instruments covering the inner heliosphere to measure ions and associated waves in collisionally young solar wind. In addition, the ongoing technological advancement for such instruments to resolve an extended number of heavy ions more accurately and at higher temporal resolution.
a) The primary challenges to high time resolution are instrument performance and data rate. Increases in collecting power (geometric factor) are needed to increase the number of heavy ions collected per unit time. Improvements in the total energy resolution of the solid-state detectors typically used would reduce overlap of ions in the time-of-flight (TOF)—energy (E) measurement space, therefore improving the ability to separate different ions. Development of better methods for avoiding the high flux of protons would lower the accidental coincidence rate, improving signal-to-noise and further supporting high time and species resolution. Another limitation to time resolution is the high data rates that are required to support the current standard of ground processing. Development of new algorithms and methods for on-board processing of this data could greatly reduce needed data rates. These developments would need to overcome the additional challenge that on-board processing eliminates the ability to reprocess the raw data later with improved algorithms. It has been largely for this reason that on-board processing has been avoided. The Heavy Ion Sensor on Solar Orbiter has the potential for up to 30 s normal time resolution (4 s in burst), though more work is required to reach that goal.
b) To make full use of the power of heavy ion composition, especially in boundary identification, more charge-resolving ion mass spectrometers need to be flown simultaneously. Since this would likely be achieved with small-sats, these instruments must be driven down in size, mass and power. One of the main drivers of these design parameters is the need to apply internal post-acceleration to ions to achieve workable TOF and E resolution. This necessitates larger power supplies and internal spacing. The use of energy detectors with lower energy threshold could improve this situation, though the emerging avalanche photodiodes typically lack sufficient energy resolution. There are no drop-in replacements for carbon foils so substantial innovation is required in this area to reduce high-voltage requirements.
3) Dedicated funding for theory and modeling congruent with observations to answer the key science topics discussed in the paper.
a) The main steps forward in understanding processes in the closed field corona include the development of a modeling framework that can dynamically capture time-dependent elemental fractionation between the chromosphere and corona as well as its connection to coronal heating (Dahlburg et al., 2017, and see details in Laming 2022, decadal survey white paper).
b) In the open corona, it is necessary to develop a self-consistent physical model of the heating and acceleration process that describes the thermal and non-thermal dynamics across the extended corona. This includes,
i) A model accounting for the physical conditions of solar wind streams that drive time-dependent ionization/recombination compatible with observed non-Maxwellian electron distributions to form the measured spectrum of freeze-in ionization states.
ii) A complete physical description of kinetic processes that preferentially heat and accelerate heavy ion particles out of the corona, generate temperature anisotropies, and form the non-thermal character of spectral lines and particle VDFs (see details in Cranmer et al 2015).
Data availability statement
The original contributions presented in the study are included in the article/supplementary material, further inquiries can be directed to the corresponding author.
Author contributions
The manuscript was compiled by YJR with content and editorial contributions from all co-authors.
Funding
YJR acknowledges support from the Future Faculty postdoctoral fellowship at Harvard University. JML was supported by NASA Heliophysics Guest Investigator Grant (80HQTR19T0029) and by Basic Research Funds of the Office of Naval Research. The work of EL was supported by NASA grants 80NSSC20K0185, 80NSSC21K0579, 80NSSC18K1553, 80NSSC18K0647, 80NSSC22K0750, and 80NSSC22K1015, and NSF grant AGS-2229138. NMV is supported by the NASA competed Heliophysics Internal Scientist Funding Model (HISFM) program.
Acknowledgments
A modified version of this paper was submitted to the 2024 Solar and Space Physics Decadal Survey and is published in the associated issue of the Bulletin of the American Astronomical Society.
Conflict of interest
EIM was employed by the company Predictive Sciences Inc.
The remaining authors declare that the research was conducted in the absence of any commercial or financial relationships that could be construed as a potential conflict of interest.
Publisher’s note
All claims expressed in this article are solely those of the authors and do not necessarily represent those of their affiliated organizations, or those of the publisher, the editors and the reviewers. Any product that may be evaluated in this article, or claim that may be made by its manufacturer, is not guaranteed or endorsed by the publisher.
References
Akhavan-Tafti, M., Kasper, J., Huang, J., and Thomas, L. (2022). Magnetic switchbacks heat the solar corona. Astrophys. J. Lett. 937, L39. doi:10.3847/2041-8213/ac913d
Baker, D., Brooks, D. H., Demoulin, P., van Driel-Gesztelyi, L., Green, L. M., Steed, K., et al. (2013). Astrophys. J. 778, 69. doi:10.1088/0004-637X/778/1/69
Baker, D., Green, L. M., Brooks, D. H., Demoulin, P., van Driel-Gesztelyi, L., Mihailescu, T., et al. (2022). Evolution of plasma composition in an eruptive flux rope. Astrophys. J. 924, 17. doi:10.3847/1538-4357/ac32d2
Bale, S. D., Badman, S. T., Bonnell, J. W., Bowen, T. A., Burgess, D., Case, A. W., et al. (2019). Highly structured slow solar wind emerging from an equatorial coronal hole. Nature 576, 237–242. doi:10.1038/s41586-019-1818-7
Berger, L., Wimmer-Schweingruber, R. F., and Gloeckler, G. (2011). PhRvL 106, 151103. doi:10.1103/PhysRevLett.106.151103
Boe, B., Habbal, S., Downs, C., and Druckmuller, M. (2021). The color and brightness of the F-corona inferred from the 2019 july 2 total solar eclipse. Astrophys. J. 912, 44. doi:10.3847/1538-4357/abea79
Boe, B., Habbal, S., Druckmuller, M., Landi, E., Kourkchi, E., Ding, A., et al. (2018). The first empirical determination of the Fe10+ and Fe13+ freeze-in distances in the solar corona. Astrophys. J. 859, 155. doi:10.3847/1538-4357/aabfb7
Bowen, T. A., Chandran, B. D. J., Squire, J., Bale, S. D., Duan, D., Klein, K. J., et al. (2022). In situ signature of cyclotron resonant heating in the solar wind. Phys. Rev. Lett. 129 (16), 1–26. doi:10.1103/PhysRevLett.129.165101
Brooks, D. H., Janvier, M., Baker, D., Warren, H. P., Auchère, F., and Carlsson, M., 2022, Plasma composition measurements in an active region from Solar Orbiter/SPICE and Hinode/EIS, doi:10.48550/arXiv.2210.08899
Brooks, D. H., Ugarte-Urra, I., and Warren, H. P. (2015). Full-Sun observations for identifying the source of the slow solar wind. Nat. Commun. 6, 5947. doi:10.1038/ncomms6947
Brooks, D. H., and Warren, H. P. (2011). Establishing a connection between active region outflows and the solar wind: Abundance measurements with EIS/hinode. Astrophys. J. 727, L13. doi:10.1088/2041-8205/727/1/L13
Chandran, B. D. G., Li, B., Rogers, B. N., Quataert, E., and Germaschewski, K. (2010). Perpendicular ion heating by low-frequency alfvén-wave turbulence in the solar wind. Astrophys. J. 720 (1), 503–515. doi:10.1088/0004-637X/720/1/503
Cranmer, S. R., Asgari-Targhi, M., Miralles, M. P., Raymond, J. C., Strachan, L., Tian, H., et al. (2015). Philosophical Trans. R. Soc. Lond. Ser. A 373, 2041. doi:10.1098/rsta.2014.0148
Cranmer, S. R., Field, G. B., and Kohl, J. L. (1999). Spectroscopic constraints on models of ion cyclotron resonance heating in the polar solar corona and high-speed solar wind. Astrophys. J. 518, 937–947. doi:10.1086/307330
Cranmer, S. R., Gibson, S. E., and Riley, P. (2017). Origins of the ambient solar wind: Implications for space weather. Space Sci. Rev. 212, 1345–1384. doi:10.1007/s11214-017-0416-y
Cranmer, S. R., and Winebarger, A. R. (2019). The properties of the solar corona and its connection to the solar wind. Annu. Rev. Astron. Astrophys. 57, 157–187. doi:10.1146/annurev-astro-091918-104416
Dahlburg, R. B., Laming, J. M., and Taylor, B., (2017). Ponderomotive acceleration in coronal loops. Astrophys. J. 831 (2), 12. doi:10.3847/0004-637X/831/2/160
Esser, R., Fineschi, S., Dobrzycka, D., Habbal, S. R., Edgar, R. J., Raymond, J. C., et al. (1999). Plasma properties in coronal holes derived from measurements of minor ion spectral lines and polarized white light intensity. Astrophys. J. 510, L63–L67. doi:10.1086/311786
Feldman, U., Schühle, U., Widing, K. G., and Laming, J. M. 1998. Coronal composition above the solar equator and the north pole as determined from spectra acquired by the SUMER instrument on SOHO 505 (2), 999–1006. doi:10.1086/306195
Geiss, J., Gloeckler, G., and Steiger, R. V. (1995). Origin of the solar wind from composition data. Space Sci. Rev. 72, 49–60. doi:10.1007/bf00768753
Gilly, C. R., and Cranmer, S. R. (2020). The effect of solar wind expansion and nonequilibrium ionization on the broadening of coronal emission lines. Astrophys. J. 901, 150. doi:10.3847/1538-4357/abb1ad
Gruesbeck, J. R., Lepri, S. T., and Zurbuchen, T. H. (2012). Astrophys. J. 760, 141. doi:10.1088/0004-637x/760/2/141
Gruesbeck, J. R., Lepri, S. T., Zurbuchen, T. H., and Antiochos, S. K. (2011). Astrophys. J. 730, 103. doi:10.1088/0004-637x/730/2/103
Habbal, S. R., Druckmuller, M., Morgan, H., Daw, A., Johnson, J., Ding, A., et al. (2010a). MAPPING THE DISTRIBUTION OF ELECTRON TEMPERATURE AND Fe CHARGE STATES IN THE CORONA WITH TOTAL SOLAR ECLIPSE OBSERVATIONS. Astrophys. J. 708, 1650–1662. doi:10.1088/0004-637x/708/2/1650
Habbal, S. R., Morgan, H., Druckmuller, M., and Ding, A. (2010b). ON the constancy of the electron temperature in the expanding corona throughout solar cycle 23. Astrophys. J. 711, L75–L78. doi:10.1088/2041-8205/711/2/l75
Kasper, J. C., Bale, S. D., Belcher, J. W., Berthomier, M., Case, A. W., Chandran, B. D. G., et al. (2019). Alfvénic velocity spikes and rotational flows in the near-Sun solar wind. Nature 576, 228–231. doi:10.1038/s41586-019-1813-z
Kohl, J. L., Noci, G., Antonucci, E., Tondello, G., Huber, M. C. E., Gardner, L. D., et al. (1997). Sol. Phys. 175, 613–644. doi:10.1023/A:1004903206467
Kohl, J. L., Noci, G., Antonucci, E., Tondello, J., Huber, M. C. E., Cranmer, S. R., et al. (1998). UVCS/SOHO empirical determinations of anisotropic velocity distributions in the solar corona. Astrophys. J. 501 (1), L127–L131. doi:10.1086/31143410.1088/0143-0807/27/4/007
Laming, J. M. (2012). Non-WKB Models of the First Ionization Potential Effect: The Role of Slow Mode Waves, Astrophys. J. 744, 115. doi:10.1088/0004-637x/744/2/115
Laming, J. M. (2004). A unified picture of the first ionization potential and inverse first ionization potential effects. Astrophys. J. 614, 1063–1072. doi:10.1086/423780
Laming, J. M. (2015). The FIP and inverse FIP effects in solar and stellar coronae. Living Rev. Sol. Phys. 12, 2. doi:10.1007/lrsp-2015-2
Laming, J. M. (2017). The first ionization potential effect from the ponderomotive force: On the polarization and coronal origin of Alfvén waves. Astrophys. J. 844, 153. doi:10.3847/1538-4357/aa7cf1
Landi, E., Gruesbeck, J. R., Lepri, S. T., Zurbuchen, T. H., and Fisk, L. A. (2012). Astrophys. J. 761, 48. doi:10.1088/0004-637x/761/1/48
Landi, E., Habbal, S. R., and Tomczyk, S. (2016). Coronal plasma diagnostics from ground-based observations. J. Geophys. Res. Space Phys. 121, 8237–8249. doi:10.1002/2016JA022598
Lenz, D. D., Lou, Y.-Q., and Rosner, R. (1998). Density structure in a multicomponent coronal loop. Astrophys. J. 504, 1020–1028. doi:10.1086/306111
Lepri, S. T., and Rivera, Y. (2021). Elemental abundances of prominence material inside ICMEs. Astrophys. J. 912, 51. doi:10.3847/1538-4357/abea9f
Lionello, R., Downs, A. C., and Linker, J. A., 2019, Ion Charge States in a Time-Dependent Wave-Turbulence-Driven Model of the Solar Wind, Solar Physics 294 (1), 11. doi:10.1007/s11207-019-1401-2
Lynch, B. J., Reinard, A. A., Mulligan, T., Reeves, K. K., Rakowski, C. E., Allred, J. C., et al. (2011). Astrophys. J. 740, 112. doi:10.1088/0004-637x/740/2/112
Oran, R., Landi, E., Holst, B. V. D., Lepri, S. T., Vasquez, A. M., Nuevo, F. A., et al. (2015). Astrophys. J. 806, 55. doi:10.1088/0004-637x/806/1/55
Rakowski, C. E., Laming, J. M., and Lepri, S. T. (2007). Ion Charge States in Halo Coronal Mass Ejections: What Can We Learn about the Explosion? Astrophys. J. 667, 602–609. doi:10.1086/520914
Raymond, J. C., Asgari-Targhi, M., Wilson, M. L., Rivera, Y. J., Lepri, S. T., and Shen, C., 2022, Dropouts of Fully Stripped Ions in the Solar Wind: A Diagnostic for Wave Heating versus Reconnection, doi:10.48550/arXiv.2208.12193
Raymond, J. C., Kohl, J. L., Noci, G., Antonucci, E., Tondello, G., Huber, M. C. E., et al. (1997). Sol. Phys. 175, 645–665. doi:10.1023/A:1004948423169
Rimmele, T. R., Warner, M., Keil, S. L., Goode, P. R., Knolker, M., Kuhn, J. R., et al. (2020). The Daniel K. Inouye solar telescope – observatory overview. Sol. Phys. 295, 172. doi:10.1007/s11207-020-01736-7
Rivera, Y. J., Landi, E., Lepri, S. T., and Gilbert, J. A. (2019a). Empirical modeling of CME evolution constrained to ACE/SWICS charge state distributions. Astrophys. J. 874, 164. doi:10.3847/1538-4357/ab0e11
Rivera, Y. J., Landi, E., Lepri, S. T., and Gilbert, J. A. (2020). On the production of He+ of solar origin in the solar wind. Astrophys. J. 899, 11. doi:10.3847/1538-4357/aba4a9
Rivera, Y. J., Landi, E., and Lepri, S. T. (2019b). Identifying spectral lines to study coronal mass ejection evolution in the lower corona. Astrophys. J. Suppl. Ser. 243, 34. doi:10.3847/1538-4365/ab2bfe
Rivera, Y. J., Raymond, J. C., Landi, E., Lepri, S. T., Reeves, K. K., Stevens, M. L., et al. (2022). Manifestation of gravitational settling in coronal mass ejections measured in the heliosphere. Astrophys. J. 936, 83. doi:10.3847/1538-4357/ac8873
Scott, R. B., Bradshaw, S. J., and Linton, M. G. (2022). The dynamic evolution of solar wind streams following interchange reconnection. Astrophys. J. 933, 72. doi:10.3847/1538-4357/ac7144
Shen, C., Raymond, J. C., Miki c, Z., Linker, J. A., Reeves, K. K., and Murphy, N. A. (2017). Time-dependent ionization in a steady flow in an MHD model of the solar corona and wind. Astrophys. J. 850, 26. doi:10.3847/1538-4357/aa93f3
Stansby, D., Baker, D., Brooks, D. H., and Owen, C. J. (2020). Directly comparing coronal and solar wind elemental fractionation. Astron. Astrophys. 640, 640A28. doi:10.1051/0004-6361/202038319
Steven, T., Enrico, L., Ben, B., Joan, B., Marc, C., Dennis, G., et al. (2021). First images from the upgraded coronal multi-channel polarimeter (UCoMP). Available at: https://ui.adsabs.harvard.edu/abs/2021AGUFMSH15G2089T/abstract.
Szente, J., Landi, E., and van der Holst, B. (2022). Charge state calculation for global solar wind modeling. Astrophys. J. 926, 35. doi:10.3847/1538-4357/ac3918
Tarr, L. A. (2017). On excited frequencies for Alfvén waves in a coronal arcade. Astrophys. J. 847, 1. doi:10.3847/1538-4357/aa880a
Tomczyk, S., Card, G. L., Darnell, T., Elmore, D. F., Lull, R., Nelson, P. G., et al. (2008). An instrument to measure coronal emission line polarization. Sol. Phys. 247, 411–428. doi:10.1007/s11207-007-9103-6
Tracy, P. J., Kasper, J. C., Raines, J. M., Shearer, P., Gilbert, J. A., and Zurbuchen, T. H. (2016). Constraining solar wind heating processes by kinetic properties of heavy ions. Phys. Rev. Lett. 116, 255101. doi:10.1103/PhysRevLett.116.255101
Uzzo, M., Ko, Y.-K., and Raymond, J. C. (2004). Active region streamer diagnostics 2001 september 14–16. Astrophys. J. 603, 760–775. doi:10.1086/381525
Uzzo, M., Ko, Y.-K., Raymond, J. C., Wurz, P., and Ipavich, F. M. (2003). Elemental abundances for the 1996 streamer belt. Astrophys. J. 585, 1062–1072. doi:10.1086/346132
Viall, N. M., and Borovsky, J. E. (2020). Nine outstanding questions of solar wind physics. J. Geophys. Res. Space Phys. 125, e2018JA026005. doi:10.1029/2018JA026005
von Steiger, R., Schwadron, N. A., Fisk, L. A., Gloeckler, G., Hefti, S., et al. (2000). Composition of quasi-stationary solar wind flows from ulysses/solar wind ion composition spectrometer. J. Geophys. Res. 105, 27217–27238. doi:10.1029/1999ja000358
Weberg, M. J., Zurbuchen, T. H., and Lepri, S. T. (2012). ACE/SWICS observatIONS of heavy ION dropouts within the solar wind. Astrophys. J. 760, 30. doi:10.1088/0004-637X/760/1/30
Keywords: chemical composition, solar wind, solar Corona, heavy ion, solar physics, heliophysics
Citation: Rivera YJ, Higginson A, Lepri ST, Viall NM, Alterman BL, Landi E, Spitzer SA, Raines JM, Cranmer SR, Laming JM, Mason EI, Wallace S, Raymond JC, Lynch BJ, Gilly CR, Chen TY and Dewey RM (2022) Deciphering the birth region, formation, and evolution of ambient and transient solar wind using heavy ion observations. Front. Astron. Space Sci. 9:1056347. doi: 10.3389/fspas.2022.1056347
Received: 28 September 2022; Accepted: 01 December 2022;
Published: 20 December 2022.
Edited by:
Sabrina Savage, Marshall Space Flight Center (NASA), United StatesReviewed by:
Huw Morgan, Aberystwyth University, United KingdomCopyright © 2022 Rivera, Higginson, Lepri, Viall, Alterman, Landi, Spitzer, Raines, Cranmer, Laming, Mason, Wallace, Raymond, Lynch, Gilly, Chen and Dewey. This is an open-access article distributed under the terms of the Creative Commons Attribution License (CC BY). The use, distribution or reproduction in other forums is permitted, provided the original author(s) and the copyright owner(s) are credited and that the original publication in this journal is cited, in accordance with accepted academic practice. No use, distribution or reproduction is permitted which does not comply with these terms.
*Correspondence: Yeimy. J. Rivera, eWVpbXkucml2ZXJhQGNmYS5oYXJ2YXJkLmVkdQ==