- 1Integrative Biotechnology, University of Applied Sciences, Kaiserslautern, Germany
- 2Department of Geography, Ludwig-Maximilians-University, Munich, Germany
- 3Faculty of Geography, Laboratory for Climatology and Remote Sensing, Philipps-University of Marburg, Marburg, Germany
- 4Institute of Biology, Laboratory of Soil Biodiversity, University of Neuchâtel, Neuchâtel, Switzerland
- 5Institute of Biology, University of Graz, Graz, Austria
- 6GEMA Center for Genomics, Ecology & Environment, Universidad Mayor, Santiago, Chile
- 7Instituto de Geografía & Centro UC Desierto de Atacama, Pontificia Universidad Católica de Chile, Santiago, Chile
- 8Corporación Nacional Forestal (CONAF), Santiago, Chile
- 9Plant Ecology Group University of Tübingen, Tübingen, Germany
The grit crust is a recently discovered, novel type of biocrust made of prokaryotic cyanobacteria, eukaryotic green algae, fungi, lichens and other microbes that grow around and within granitoid stone pebbles of about 6 mm diameter in the Coastal Range of the Atacama Desert, Chile. The microbial community is very well adapted towards the extreme conditions of the Atacama Desert, such as the highest irradiation of the planet, strong temperature amplitudes and steep wet-dry cycles. It also has several other striking features making this biocrust unique compared to biocrusts known from other arid biomes on Earth. It has already been shown that the grit crust mediates various bio-weathering activities in its natural habitat. These activities prime soil for higher organisms in a way that can be envisioned as a proxy for general processes shaping even extra-terrestrial landscapes. This mini-review highlights the potential of the grit crust as a model for astrobiology in terms of extra-terrestrial microbial colonization and biotechnological applications that support human colonization of planets.
Introduction
Terrestrial extremophile microorganisms have ever since inspired hypotheses about life strategies in extra-terrestrial environments, e.g., on Mars, and about their applications in biotechnology-driven space activities. Such extremophiles usually include several hetero- and chemoautotrophic bacteria, archaea and fungi as well as photoautotrophic cyanobacteria and eukaryotic algae (Liu et al., 2008; Beblo-Vranesevic et al., 2018; Sundarasami et al., 2019; Coleine and Delgado-Baquerizo, 2022). A specific aspect of extreme environments has frequently been overlooked in this context: the symbiotic lifestyle, which is perfectly exemplified by biological soil crusts (hereafter: biocrusts). Moreover, the close coexistence and interacting of the biocenosis in a confined space also supports the response to sudden abiotic environmental fluctuations or changes and gives the community overall better resilience through different physiological use and response to change. These symbiotic associations of microorganisms are mainly found in hot and cold deserts across the globe, where they are often the main biological component colonizing the soil’s surface (Weber et al., 2022). Soil particles are aggregated through the presence and activity of this often extremo-tolerant biota that desiccates regularly. The resulting living crust mantels the ground’s surface as a coherent layer. It was estimated that biocrusts cover about 12% of the Earth’s terrestrial surface and about 30% of all dryland soils (Rodriguez-Caballero et al., 2018). In these extreme environments, the microbial consortium regularly faces long periods of desiccation, intense UV radiation, and severe nutrient and resource limitations (Belnap et al., 2008; Munzi et al., 2019).
To overcome environmental limitations biocrust organisms have developed several strategies such as extracellular polymeric substances (EPS) that help them to retain water (Mugnai et al., 2018), UV-protecting pigments such as scytonemin (Miralles et al., 2017) or specific compounds including sucrose and trehalose (Xin et al., 2015; Baubin et al., 2021) that minimize metabolic desiccation stress. Most of these strategies have not yet been fully explored, and a plethora of novel genes and metabolites is expected from biocrust-forming microorganisms from extreme environments which can be used to obtain a wide variety of products using biotechnological processes (Lakatos and Strieht, 2017). Cyanobacteria and green algae are already optimal candidates for present biotechnological application on Earth due to low needs for maintenance (Schweiger et al., 2022). Therefore, this micro-algal-based technology could also be part of regenerative life support systems in space.
Beside such applications, biocrust-forming microorganisms as early terrestrial microbial associations on Earth can also help to address ancient humanity’s questions, such as whether we are alone or how and where did life appear and evolve? This again has to do with their extremo-tolerant character, and the early time of their origins or their ability to interact with each other. Cyanobacteria, for example, which evolved during the late Archaean (Sánchez-Baracaldo and Cardona, 2020), can be found in virtually any environment on Earth and in symbiosis with fungi (Rikkinen, 2015) and plants (Chang et al., 2019). These microorganisms evolved at a time where the atmospheric composition of our planet and radiation income of the Sun were different. The Archaean Earth was devoid of oxygen and enriched by greenhouse gases (Hessler et al., 2004) including an increased exposition to higher fluxes of UV-B (280–320 nm) and UV-C (200–280 nm) radiation (Cockell, 2002). However, cyanobacteria could not only cope with these conditions (Shih, 2015), they also supposedly led to the Great Oxygenation Event during which they changed Earth’s atmosphere and became ancestral to all other eukaryotic photosynthetic organisms such as algae and plants (Sánchez-Baracaldo and Cardona, 2020). This implies that cyanobacteria (and other microorganisms) resemble concepts of simple life forms that have a high adaptation potential to extreme conditions. Thus, we imagine that relatable life forms might be expected beyond Earth even if the abiotic conditions differ. Whether they are alive or extinct, they have and they still left their footprints on Earth, and we can learn to interpret the pattern they left as biosignatures to support the search for life on other planets at least in the Solar System and particular on planets such as Mars. Cyanobacteria, for example, are well known for their ability to form stromatolites on shores and lakes ranging in height from millimeters to tens of meters and extending laterally from small lenses to aggregates of hundreds of kilometers wide as mineralized domes (Neilan et al., 2002). If cyanobacteria alone leave such landscape-dominating traces behind in aquatic environments over millennia, how pronounced must then their traces be when they are an integral part of biocrusts together with lichens, green algae and other microorganisms that cover 12% of the Earth’s terrestrial surface?
Recently, a novel type of biocrust was discovered in the Atacama Desert, one of the world’s oldest and driest deserts, covering most of its floor: the grit crust (Jung et al., 2020a). The grit crust’s microbial community is unique amongst biocrusts found in all other arid parts of the world because cyanobacteria, eukaryotic green algae, lichens and specific fungi grow on, in, and around small granitoid quartz pebbles called grits (locally called “maicillo”) of about 6 mm in diameter. These structures can be seen with naked eyes and cause a blackish, landscape filling pattern, on the otherwise bare ground. The Atacama Desert is extremely interesting in that sense as it resembles an environment close to what is expected to be found on other planetary bodies, such as Mars, in terms of bio-geochemistry, nutrient composition or topological similarities - or at least mimics particular characters (Navarro-González, et al., 2003; Schmidt, et al., 2018). This can be explained by the unique combination of its environmental extremes such as its long lasting history of hyper-aridity, the highest UV radiation levels on Earth, oxidizing soils and other factors that can be compared with the conditions present on Mars (Azua-Bustos et al., 2022). For these reasons, an increasing number of experiments spanning from testing technologies over to experiments in the life sciences have been conducted at the Atacama Desert (Sobron et al., 2012; Abarca et al., 2013; Gunes-Lasnet et al., 2014; Schulze-Makuch et al., 2018). Thus, studying the survival and microbial diversity of the grit crust of the Atacama Desert during future studies will provide valuable insights into understanding the evolution of life, the habitability beyond Earth, and biotechnological applications of these microorganisms that could support space exploration missions.
Currently, the research project “Grit Life” funded by the German Research Foundation, aims to untangle the microbiota of the grit crust based on metabarcoding data applied to a recovery experiment over several years. This will not only give detailed insights into the diversity of bacteria, eukaryotes, and fungi but also tracks their re-colonization potential. The DNA-based approach is accompanied by monitoring climate and micro-climate data of the grit crust environment so that the extremophile character of the microbiota can be determined. In addition, fungi, eukaryotic green algae, and cyanobacteria of the grit crust will successively be isolated and curated in the context of a microbial archive in order to guarantee future investigations, e.g., on the microbe’s biotechnological potential. As such, this project is the first of its kind that will generate a microbial archive of biocrusts where the extremophile properties of the microorganisms are characterized, allowing for transfer into biotechnological approaches. Exploiting this unique biocenosis will be discussed in the following in terms of the search for life beyond Earth based on novel potential fossilization structures known from the grit crust (Figure 1), and the utilization of the biocenosis’ microorganisms for biotechnological approaches in space (Figure 2).
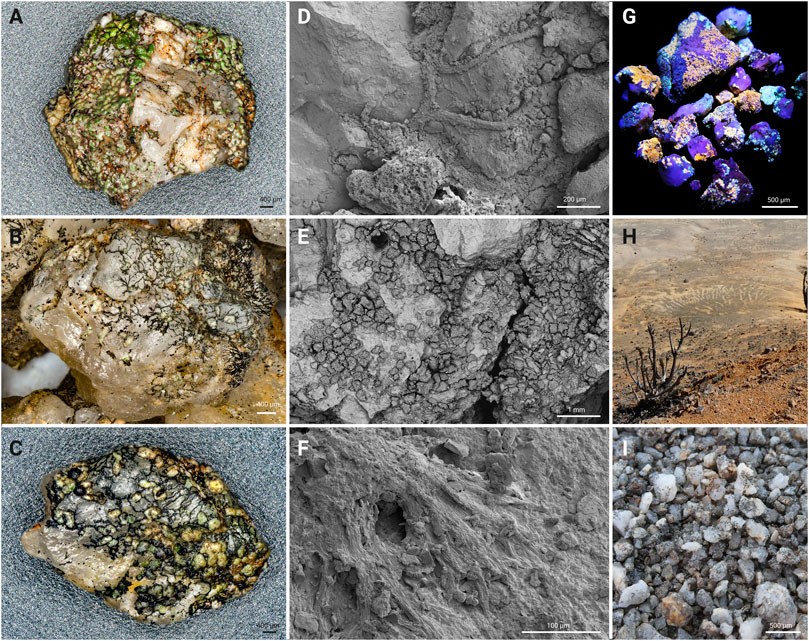
FIGURE 1. Potential fossilization traces of the grit crust depicted by various techniques. (A–C) micrographs of single grit stones in their hydrated state with lichens and fungi creating a vein-like network of micro-structures on the grit’s surface. (D–F) scanning electron microscopy showing fungal trench lines in (D), lichen squamules covered by mineral particles on grit stones in (E) and lichen pro-thallus in (F). (G–I) photographs of the grit crust with glowing lichens in G caused by reflectance of secondary lichen substances under UV light, erosion rifts in (H) and a grit crust overview image with grits overgrown by various concatenating microorganisms in (I).
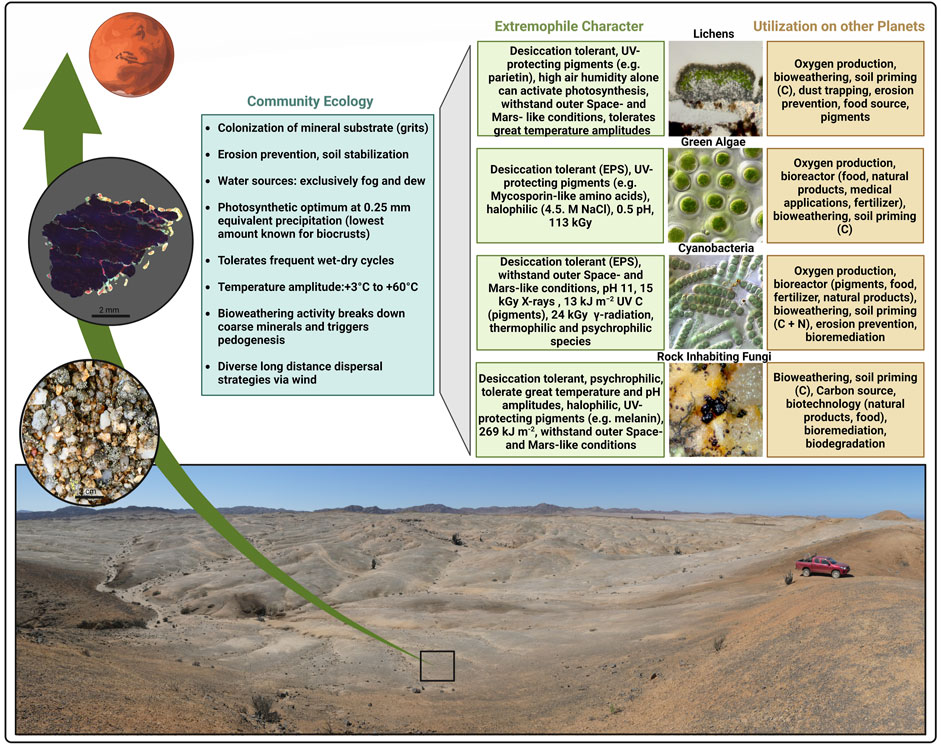
FIGURE 2. Ecology, extremophile character and possible applications of the grit crust in the context of astrobiology. The bottom picture shows the landscape of the coastal Atacama Desert with blackish patterns on the ground formed by a high microbial colonization degree. The bottom circle on the left shows a close-up photography of the ground with the grits colonized by diverse microbes such as lichens. The top circle shows a thin section of a single grit under the microscope using autofluorescence where fungal mycelium (lichens) appear whitish while algae appear red, which also colonize inner structures of the stone.
Suitability of the grit crust’s ecology for the colonization of other planets
The grit crust covers a roughly 40 km broad strip, 2.5 km off the Coastal Range of the southern Atacama Desert (∼26°S), spanning over 1.500 km to the northern Atacama Desert. In these areas, it forms blackish patterns on the desert’s ground caused by a high degree of microbial colonization of the otherwise whitish granitoid quartz pebbles of approximately 6 mm diameter. These blackish patterns are so rich in organic material that they are well visible by the naked eye in the landscape. The reason for this is the cyclic appearance of fog and dew water input that leads to biomass growth equivalent to about 140 mg chlorophylla+b m−2 and more (Jung et al., 2020a). These high biomass stocks are reached in a xeric environment where soil temperature can exceed 60 °C during summer days and falls below 3 °C during winter times. Average water input from dew is only 0.025–0.088 mm per day, water input from fog is 0.38–1.25 mm per day, and intense irradiation, with maxima of more than 1,500 μmol photons m2 s−1 is frequent (Jung et al., 2020a). In general, the Atacama Desert is considered as a radiation hotspot where the highest irradiation on the planet was reported (Rondanelli et al., 2015). Constituents of the microbial community are diverse micro-lichens (Buellia spp., Pleopsidium chlorophanum, Tetramelas pulverulentus, Aspicilia spp., Diploschistes spp.), with green algal photobionts (Trebouxia spp., Myrmecia spp.), free living eukaryotic green algae (Stichococcus spp., Pseudostichococcus spp., Chlorella spp., Klebsormidium spp.) and cyanobacteria (Nostoc spp., Microcoleus vaginatus, Kastovskya adunca, Myxacorys chilensis, Pleurocapsa spp.), as well as melanized micro-colonial fungi (Constantinomyces spp., Lichenothelia spp.) (Jung et al., 2020a). These harsh conditions under which they thrive may indicate that the microbial community building the grit crust - or at least individual strains contained therein - might be suitable candidates for testing (their) vitality in outer Space or under Martian conditions (Coleine and Delgado-Baquerizo, 2022). Here, it is also worth to mention that unicellular life forms evolved early in the Earth’s history. On the other hand, the climate of the Atacama Desert is considered to be stable for at least 150 million years (Hartley at al., 2005), which leads to the idea that the microbial life forms that jointly build the grit crust of the Atacama Desert are well adapted to extreme environments. Although other microbial consortia are known from hyper-arid regions of the Atacama Desert (e.g. Schulze-Makuch et al., 2018), the grit crust is exposed to low amounts of fog in areas devoid of any higher vegetation (Jung et al., 2020a) and it is the only microbial assemblage which fills entire landscapes of hundreds of square kilometers (Jung et al., 2020a). This demonstrates the ecophysiological adaptation potential of the grit crust on the large scale environment which might be beneficial for microbial colonization of other planets with relatable conditions.
Surviving prolonged desiccation is a common feature of all organisms mentioned here, but in addition, it has been shown that the photosynthetic activity of the grit crust is strongly inhibited when more than the equivalent of 0.25 mm water is present in the organisms (Jung et al., 2020a). This amount also marks the range in which the net photosynthesis of the microbial community is at its optimum. At the same time, this is the lowest amount of water for optimum photosynthesis detected for biocrusts on Earth (Jung et al., 2020a). Amongst biocrusts, the grit crust from the Atacama Desert is also unique in its adaptation to wet-dry cycles caused by several fog events during a single day because these cyclic conditions have been shown to be harmful for other biocrusts (Belnap et al., 2004; Reed et al., 2012). This implies that the grit crust’s microbial community could be ideal for biotechnological applications outside its natural environment, such as humanly induced microbial colonization of other planets, where cyclic artificial wetting will for sure be essential, but in the case of the grit crust - compared to other biocrusts - not lethal due to their specialized desiccation tolerance.
One of the biggest challenges during human colonization of other planets has been the formation of soil as a weathering product of mainly unweathered rocks found on other planets. Access to nutrient rich soil would subsequently allow the growth of microorganisms and/or plants in order to support human life. The grit crust is the first biocrust detected on a pure mineral, coarse substrate, while most other biocrust types are established on soil, a product of a later stage in the weathering of minerals (Weber et al., 2022). This resembles another benefit of the grit crust compared with other biocrusts because coarse minerals are the predominant substrate found on, e.g., Mars and the Earth’s moon. Mars’ surface consists mostly of coarse regolith of largely weathered basalt (McSween et al., 2009), which contains the basic macro elements (C, H, O, N, P, S, K, Mg, Na, and Ca) and minor elements (Mn, Cr, Ni, Mo, Cu, Fe, and Zn) essential for life (Greenwood et al., 2007; Stern et al., 2015; Verseux et al., 2016). However, due to the lack of organic carbon, the basaltic regolith substrate has a poor water-holding capacity and limited nutritional bioavailability (Wamelink et al., 2014).
Despite colonization of the quartz pebbles’ surface, the grit crust microorganisms also inhabit the undersurface and naturally occurring cracks where they are protected from harsh UV radiation but can still perform photosynthesis due to the transparent to translucent character of the stones. It has also been shown that these microorganisms actively mediate bio-weathering activities regarding nutrient acquisition, which can lead to the formation of a terrestrial protopedon as initial pedogenesis (Jung et al., 2020b). This terrestrial protopedon is a fine substrate enriched in nitrogen from cyanobacterial nitrogen fixation and carbon from photosynthesis that primes the substrate for the establishment of other organisms and leaches into deeper layers (Dojani et al., 2007; Young et al., 2022). Triggering this succession is a well-known feature of biocrusts worldwide (Belnap and Weber, 2013; Barger et al., 2016; Bao et al., 2019), but the grit crust is the only biocrust type on Earth where minerals are the starting material and not (the more weathered) sand or soil. At the same time, the leaked or extracellular polymeric substances (EPS) of cyanobacteria, for example, together with the filamentous nature of some algae, the lichens and fungi concatenate the first millimeters of the substrate that protects it from erosion by, e.g., wind (Chamizo et al., 2017). This leads to the idea that the grit crust might have the potential to enrich mineral substrates of other planets over the long term and protects it from strong wind erosion, which is crucial on some Solar System bodies, e.g. Mars, where wind speeds can be immense (White, 1979; Viúdez-Moreiras et al., 2019). However, such strong winds could also be beneficial for colonizing great landscapes on other planets by the grit crust organisms, as these winds produce a lot of dust that can be transported over wide distances (e.g., Ferri et al., 2003), on which microbes can hitchhike. The Martian atmosphere is full of dust, with loads that greatly fluctuate with the year’s season (Toigo and Richardson, 2000), so aeolian transport, including microbes, is a likely scenario that could support the microbial colonization of other planets that support human life. In the Atacama Desert, for example, it has been shown that certain soil-borne microorganisms were transported more than 100 km off the coast towards the hyper-arid core of the Atacama (Azua-Bustos et al., 2019).
Microalgae, lichens and fungi of the grit crust as models for astrobiology
On Earth, phototrophic extremophiles have been found in hostile environments, such as hot and cold deserts, hot, acidic, or alkaline springs, caves, or Antarctic sub-glacial lakes considered as Earth analogs of Mars and the icy moons of Jupiter and Saturn (Martins et al., 2017; Coleine and Delgado-Baquerizo, 2022). One of the most interesting microalgae occupying various ecological niches of such extreme habitats is the prokaryotic cyanobacterial order Chroococcidiopsidales, which has been a focal point for astrobiology research. For specific strains of the genus Chroococcidiopsis sensu lato, for example, it has been shown that they tolerate at least 4 years of air-drying (Billi, 2009; Fagliarone et al., 2017), up to 13 kJ m−2 of UV C radiation (Baqué et al., 2013), 15 kGy of X-rays (Billi et al., 2000) and 12 kGy of γ-radiation (Verseux et al., 2016; Verseux et al., 2017). They even have an enhanced radiation resistance, coping with 30 kJ m−2 of a simulated Martian UV flux (Cockell et al., 2005), 24 kGy of γ-radiation and 2 kGy of Fe ions (Verseux et al., 2016). In their dried state, these strains also survived 2.5 years of exposure to Mars and Space-like conditions (Billi, 2019). Also a comparative analysis of whole-genome sequences showed no increased variant numbers in the space-derivate compared to triplicates of the reference strain maintained on the ground (Napoli et al., 2022). Closely related species that also belong to the order Chroococcidiopsidales, such as Aliterella chasmolithica (Jung et al., 2020c) and Gloeocapsopsis diffluens (Jung et al., 2021) were found in the grit crust environment, and a relative of the latter has been shown to produce great amounts of trehalose (145 µg per mg dry weight) and sucrose (64 µg per mg dry weight) as desiccation protection along with copious amounts of EPS (Azua-Bustos et al., 2019). This implies that these organisms have strong capabilities to protect themselves from damage during prolonged desiccation periods, a great characteristic for exposure to Space-like conditions during travel or terraformation approaches. Although these results were achieved for several strains of the order Chroococcidiopsidales, they likely also apply to other cyanobacteria—especially if they were isolated from desert environments such as the grit crust, where most of these extreme conditions come together. In addition to Chroococcidiopsidales, other cyanobacteria such as Pleurocapsa spp., the endemic Kastovskya adunca, Myxacorys chilensis and several Nostoc species have been found to be part of the biocenosis of the grit crust (Jung et al., 2019) and are expected to possess similar desiccation resistance functions.
In contrast to the great insights on the survivability of prokaryotic cyanobacteria exposed to Space- or Mars-like conditions, little is known about these capabilities for eukaryotic green algae. One of the most notorious green algae is the spherical and highly abundant genus Chlorella which can adapt to a range of extreme and harsh terrestrial conditions (Krienitz et al., 2015). Members of this genus isolated from desert sand crusts can resist intense UV radiation and γ-rays (Treves et al., 2013). In contrast to cyanobacteria, experiments, where Chlorella from desert environments was exposed to a Mars-like near-space environment showed that they survived but got significantly damaged on a sub-cellular and physiological level (Wang et al., 2021). We already detected several Chlorella and Chlorella-like types as frequent constituents of the grit crust, including Pseudochlorella spp. or Pseudostichococcus spp. which share similar characteristics. However, Chlorella is already used in large-scale bioreactors on Earth for commercial interests due to its ability to quickly adapt to cultivation conditions, because of the significant amounts of biomass generated in a short time, its CO2 capturing ability and role as a food source (Coronado-Reyes et al., 2020). For these reasons, there are various ideas and concepts that suggest such green algae to be an integral part of lunar bases (Detrel, 2021) or on space stations (Helisch et al., 2016; Niederwieser et al., 2018), where a photobioreactor volume of 482 L has been shown to be suitable to ensure a daily CO2 reprocessing for one crew member (Helisch et al., 2020).
Another component of the grit crust from the Coastal Range of the Atacama Desert are lichens, which -in general- represent a micro-ecosystem built by a fungus and an algal photobiont partner, including a vast microbiome (Hawksworth and Grube, 2020). Several lichen species have already been detected in the grit crust belonging to the genera Aspicilia, Buellia and Pleopsidium (Jung et al., 2020a), including some micro-colonial fungi of the genera Lichenothelia and Constantinomyces that are poly-extremotolerant (Gostinčar et al., 2012; Grube et al., 2013) and can facultatively be in symbiotic relationship with free-living eukaryotic green algae (Muggia et al., 2013). The lichen Pleopsidium chlorophanum, also present in the grit crust, was exposed uninterruptedly to simulated conditions of protected Martian surface niche conditions (269 kJ m−2) for 34 days. The lichen not only survived and remained photosynthetically active but even adapted physiologically by increasing its photosynthetic activity over 34 days (de Vera et al., 2014). Similar results have been achieved for numerous other lichens (de Vera, 2012; Meeßen et al., 2013; Brandt et al., 2015; De la Torre Noetzel et al., 2017). In addition, black micro-colonial fungi (MCF), also referred to as rock-inhabiting fungi (RIF) (Sterflinger, 1998; Gorbushina, 2003), can cope with extremes of temperatures, acidity, osmotic stress and salinity, dehydration, solar and UV radiation and even radioactivity (Sterflinger 1998; Gorbushina, 2003; Onofri et al., 2007), with some of them being able to survive even real space exposure and simulated Martian conditions (Onofri et al., 2007). This indicates that lichens, their photobionts and RIFs as extremophile organisms, in general, can be great indicators to determine and prime the habitability of a planet and for the search of possible life-supporting surroundings on planets like Mars (Armstrong, 2019; Joseph et al., 2020).
Not only the singular microbes of the grit crust represent promising candidates for astrobiology but also their ecology as a community can give new impulses: the grit crust creates unique micro- and macroscopic patterns that can potentially get fossilized over time and that have until now not been included in the search for life on other planets. Paying attention to these structures is crucial and can complement investigations of fossilized microbial structures on other bodies of the Solar System or exoplanets. The structures of the grit crust microbes such as lichen thalli and fungal- or algal filaments, for example, can trap mineral particles rich in calcium carbonate that can fossilize as a venous network (Figures 1A–C). A similar pattern might be created by trench lines generated by fungi that etch the quartz surface (Figure 1D). The later is a consequence of bio-weathering activity which has already been shown for the grit crust (Jung et al., 2020b). In addition, water erosion based on occasional heavy rain events play a crucial role in the Atacama Desert (Alcayaga et al., 2022). As the organisms concatenate the mineral substrate, the grits can create small gutters where excess water runs off, forming a slightly wavy micro-topography (Figure 1H). These “waves” are quite stable due to high contents of calcium carbonate that concatenates the waves which can later be fossilized over time. These and other structures can be visualized by various techniques such as simple photography using the full light spectrum or UV-radiation, scanning electron microscopy or stereoscopic micrographs (Figure 1), all of which are common for space missions (Joseph et al., 2020).
Concluding remarks
The Coastal Range of the Atacama Desert has recently been identified as the home to an outstanding biocenosis called grit crust, with a tremendous potential for various aspects of astrobiology. The ecology of the grit crust, including its extremophile microbial constituents, are unique amongst biocrusts on Earth that can be beneficial for human colonization of other planets or rock bodies such as the Earth’s Moon or Mars. The new research project Grit Life aims to untangle the full microbial biodiversity of this grit environment, including isolating prominent microorganisms that will support future research in the context of astrobiology and space sciences. During these studies, the grit crust’s extremophiles can be used to test their suitability during mass cultivation in photobioreactors (food-, oxygen source for crewed missions), screenings for Chlorophyll f (allows photosynthesis of cyanobacteria in low light environments such as on other planets) and symbiotic interactions (expands the knowledge on ancient life forms). For these reasons, the grit crust as a consortium of various microorganisms on a mineral substrate opens up a new opportunity to test hypotheses and ideas in the context of astrobiology that surpasses other biocrusts known on Earth.
Author contributions
PJ prepared the first draft of the manuscript and the figures. All authors revised the manuscript and approved the final.
Funding
The project “Grit Life” is funded by the German Research Foundation (DFG) with the grant number JU 3228/1-1. ML was funded by the Ministry of Science and Health Rhineland-Palatinate (PhytoBioTech, 724-0116#2021/004-1501 15405) and by the Federal Ministry of Education and Research (W2V-Strategy2Value, 03WIR4502A). FA and CR were supported by FONDECYT-1220358 and FONDECYT-11200789 respectively, Agencia Nacional de Investigación y Desarrollo (ANID), Chile.
Acknowledgments
The authors want to thank CONAF (Corporación Nacional Forestal) for their help in the context of this research project and the kind contributions of the rangers. Images were prepared with the great help of Biorender.
Conflict of interest
The authors declare that the research was conducted in the absence of any commercial or financial relationships that could be construed as a potential conflict of interest.
Publisher’s note
All claims expressed in this article are solely those of the authors and do not necessarily represent those of their affiliated organizations, or those of the publisher, the editors and the reviewers. Any product that may be evaluated in this article, or claim that may be made by its manufacturer, is not guaranteed or endorsed by the publisher.
References
Abarca, M., Diaz, A., de las Casas, H., Lazarte, A., Trujillo, S., and Onchi, E. (2013). Establishment of a Mars analogue research station in pampas de la Joya (Atacama Desert in southern Peru). Lakewood, Colorado, U.S: The Mars Society.
Alcayaga, H., Soto-Alvarez, M., Laronne, J. B., Caamaño, D., Mao, L., and Urrutia, R. (2022). Runoff volume and sediment yield from an endorheic watershed generated by rare rainfall events in the Atacama Desert. Geomorphology 400, 108107. doi:10.1016/j.geomorph.2021.108107
Armstrong, R. A. (2019). The lichen symbiosis: Lichen “extremophiles” and survival on Mars. J. Astrobiol. Space Sci. Rev. 1, 378–397.
Azua-Bustos, A., González-Silva, C., and Fairén, A. G. (2022). The Atacama Desert in northern Chile as an analog model of Mars. Front. Astron. Space Sci. 8, 810426. doi:10.3389/fspas.2021.810426
Azua-Bustos, A., González-Silva, C., Fernández-Martínez, M. Á., Arenas-Fajardo, C., Fonseca, R., Martín-Torres, F. J., et al. (2019). Aeolian transport of viable microbial life across the Atacama Desert, Chile: Implications for Mars. Sci. Rep. 9 (1), 1–11. doi:10.1038/s41598-019-47394-z
Bao, T., Zhao, Y., Gao, L., Yang, Q., and Yang, K. (2019). Moss-dominated biocrusts improve the structural diversity of underlying soil microbial communities by increasing soil stability and fertility in the Loess Plateau region of China. Eur. J. Soil Biol. 95, 103120. doi:10.1016/j.ejsobi.2019.103120
Baqué, M., Viaggiu, E., Scalzi, G., and Billi, D. (2013). Endurance of the endolithic desert cyanobacterium Chroococcidiopsis under UVC radiation. Extremophiles 17, 161–169. doi:10.1007/s00792-012-0505-5
Barger, N. N., Weber, B., Garcia-Pichel, F., Zaady, E., and Belnap, J. (2016). “Patterns and controls on nitrogen cycling of biological soil crusts,” in Biological soil crusts: An organizing principle in drylands (Cham: Springer), 257–285.
Baubin, C., Ran, N., Siebner, H., and Gillor, O. (2021). The response of desert biocrust bacterial communities to hydration-desiccation cycles. SOIL Discuss., 1–48.
Beblo-Vranesevic, K., Bohmeier, M., Perras, A. K., Schwendner, P., Rabbow, E., Moissl-Eichinger, C., et al. (2018). Lack of correlation of desiccation and radiation tolerance in microorganisms from diverse extreme environments tested under anoxic conditions. FEMS Microbiol. Lett. 365 (6), fny044. doi:10.1093/femsle/fny044
Belnap, J., Phillips, S. L., Flint, S., Money, J., and Caldwell, M. (2008). Global change and biological soil crusts: Effects of ultraviolet augmentation under altered precipitation regimes and nitrogen additions. Glob. Change Biol. 14 (3), 670–686. doi:10.1111/j.1365-2486.2007.01509.x
Belnap, J., Phillips, S. L., and Miller, M. E. (2004). Response of desert biological soil crusts to alterations in precipitation frequency. Oecologia 141 (2), 306–316. doi:10.1007/s00442-003-1438-6
Belnap, J., and Weber, B. (2013). Biological soil crusts as an integral component of desert environments. Ecol. Process. 2 (1), 11–12. doi:10.1186/2192-1709-2-11
Billi, D., Friedmann, E. I., Hofer, K. G., Grilli Caiola, M., and Ocampo-Friedmann, R. (2000). Ionizing-radiation resistance in the desiccationtolerant cyanobacterium Chroococcidiopsis. Appl. Environ. Microbiol. 66, 1489–1492. doi:10.1128/aem.66.4.1489-1492.2000
Billi, D. (2009). Subcellular integrities in Chroococcidiopsis sp. CCMEE 029 survivors after prolonged desiccation revealed by molecular probes and genome stability assays. Extremophiles 13, 49–57. doi:10.1007/s00792-008-0196-0
Billi, D. (2019). Desert cyanobacteria under space and planetary simulations: A tool for searching for life beyond Earth and supporting human space exploration. Int. J. Astrobiol. 18 (5), 483–489. doi:10.1017/s147355041800037x
Brandt, A., de Vera, J. P., Onofri, S., and Ott, S. (2015). Viability of the lichen Xanthoria elegans and its symbionts after 18 months of space exposure and simulated Mars conditions on the ISS. Int. J. Astrobiol. 14 (3), 411–425. doi:10.1017/s1473550414000214
Chamizo, S., Rodríguez-Caballero, E., Román, J. R., and Cantón, Y. (2017). Effects of biocrust on soil erosion and organic carbon losses under natural rainfall. Catena 148, 117–125. doi:10.1016/j.catena.2016.06.017
Chang, A. C. G., Chen, T., Li, N., and Duan, J. (2019). Perspectives on endosymbiosis in coralloid roots: Association of cycads and cyanobacteria. Front. Microbiol. 10, 1888. doi:10.3389/fmicb.2019.01888
Cockell, C. S., Schuerger, A. C., Billi, D., Friedmann, E. I., and Panitz, C. (2005). Effects of a simulated martian UV flux on the cyanobacterium, Chroococcidiopsis sp. 029. Astrobiology 5, 127–140. doi:10.1089/ast.2005.5.127
Cockell, C. S. (2002). Photobiological uncertainties in the Archaean and post-Archaean world. Int. J. Astrobiol. 1 (1), 31–38. doi:10.1017/s1473550402001003
Coleine, C., and Delgado-Baquerizo, M. (2022). Unearthing terrestrial extreme microbiomes for searching terrestrial-like life in the Solar System. Trends Microbiol. 30, 1101–1115. doi:10.1016/j.tim.2022.04.002
Coronado-Reyes, J. A., Salazar-Torres, J. A., Juarez-Campos, B., and Gonzalez-Hernandez, J. C. (2020). Chlorella vulgaris, a microalgae important to be used in biotechnology: A review. Food Sci. Technol.
de la Torre Noetzel, R., Miller, B., Cubero, A. Z., Sancho, L. G., Jordão, L., Rabbow, E., and de Vera, J. P. (2017). Survival of lichens on the ISS-II: Ultrastructural and morphological changes of circinaria gyrosa after space and mars-like conditions.
de Vera, J. P. (2012). Lichens as survivors in space and on Mars. Fungal Ecol. 5 (4), 472–479. doi:10.1016/j.funeco.2012.01.008
de Vera, J. P., Schulze-Makuch, D., Khan, A., Lorek, A., Koncz, A., Möhlmann, D., et al. (2014). Adaptation of an Antarctic lichen to Martian niche conditions can occur within 34 days. Planet. Space Sci. 98, 182–190. doi:10.1016/j.pss.2013.07.014
Detrell, G. (2021). Chlorella vulgaris photobioreactor for oxygen and food production on a moon base—potential and challenges. Front. Astron. Space Sci. 8, 700579. doi:10.3389/fspas.2021.700579
Dojani, S., Lakatos, M., Rascher, U., Wanek, W., Lüttge, U., and Büdel, B. (2007). Nitrogen input by cyanobacterial biofilms of an inselberg into a tropical rainforest in French Guiana. Flora - Morphol. Distribution Funct. Ecol. Plants 202 (7), 521–529. doi:10.1016/j.flora.2006.12.001
Fagliarone, C., Mosca, C., Ubaldi, I., Verseux, C., Baqué, M., Wilmotte, A., et al. (2017). Avoidance of protein oxidation correlates with the desiccation and radiation resistance of hot and cold desert strains of the cyanobacterium Chroococcidiopsis. Extremophiles 21, 981–991. doi:10.1007/s00792-017-0957-8
Ferri, F., Smith, P. H., Lemmon, M., and Rennó, N. O. (2003). Dust devils as observed by Mars pathfinder. J. -Geophys. -Res. 108 (E12). doi:10.1029/2000je001421
Gorbushina, A. (2003). Microcolonial fungi: Survival potential of terrestrial vegetative structures. Astrobiology 3 (3), 543–554. doi:10.1089/153110703322610636
Gostinčar, C., Muggia, L., and Grube, M. (2012). Polyextremotolerant black fungi: Oligotrophism, adaptive potential, and a link to lichen symbioses. Front. Microbiol. 3, 390. doi:10.3389/fmicb.2012.00390
Greenwood, J. P., Blake, R. E., Barron, V., and Torrent, J. (2007). in Phosphorous geochemistry of Mars: Evidence for an early acidic hydrosphere (Pasadena, California: Seventh International Conference on Mars).
Grube, M., Muggia, L., and Gostinčar, C. (2013). “Niches and adaptations of polyextremotolerant black fungi,” in Polyextremophiles (Dordrecht: Springer), 551–566.
Gunes-Lasnet, S., Kisidi, A., van Winnendael, M., Josset, J. L., Ciarletti, V., Barnes, D., and Chong Diaz, G. (2014). Safer: The promising results of the Mars mission simulation campaign in Atacama, Chile.
Hartley, A. J., Chong, G., Houston, J., and Mather, A. E. (2005). 150 million years of climatic stability: Evidence from the Atacama Desert, northern Chile. J. Geol. Soc. Lond. 162, 421–424. 10.1144/0016-764904-071.
Hawksworth, D. L., and Grube, M. (2020). Lichens redefined as complex ecosystems. New Phytol. 227 (5), 1281–1283. doi:10.1111/nph.16630
Helisch, H., Keppler, J., Bretschneider, J., Belz, S., Henn, N., Fasoulas, S., et al. (2016). Preparatory ground-based experiments on cultivation of Chlorella vulgaris for the ISS experiment PBR@ LSR. 46th. Int. Conf. Environ. Syst.
Helisch, H., Keppler, J., Detrell, G., Belz, S., Ewald, R., Fasoulas, S., et al. (2020). High density long-term cultivation of Chlorella vulgaris SAG 211-12 in a novel microgravity-capable membrane raceway photobioreactor for future bioregenerative life support in SPACE. Life Sci. Space Res. 24, 91–107. doi:10.1016/j.lssr.2019.08.001
Hessler, A. M., Lowe, D. R., Jones, R. L., and Bird, D. K. (2004). A lower limit for atmospheric carbon dioxide levels 3.2 billion years ago. Nature 428, 736–738. doi:10.1038/nature02471
Joseph, R. J., Graham, L., Büdel, B., Jung, P., Kidron, G. J., Latif, K., and Schild, R. (2020). Mars: Algae, lichens, fossils, minerals, microbial mats, and stromatolites in gale crater. J. Astrobiol. Space Sci. Rev. 3 (1), 40–111.
Jung, P., Azua-Bustos, A., Gonzalez-Silva, C., Mikhailyuk, T., Zabicki, D., Holzinger, A., et al. (2021). Emendation of the coccoid cyanobacterial genus Gloeocapsopsis and description of the new species Gloeocapsopsis diffluens sp. nov. And Gloeocapsopsis dulcis sp. nov. Isolated from the coastal range of the Atacama Desert (Chile). Front. Microbiol. 12, 671742. doi:10.3389/fmicb.2021.671742
Jung, P., Baumann, K., Emrich, D., Springer, A., Felde, V. J., Dultz, S., et al. (2020b). Lichens bite the dust–A bioweathering scenario in the Atacama Desert. Iscience 23 (11), 101647. doi:10.1016/j.isci.2020.101647
Jung, P., Baumann, K., Lehnert, L. W., Samolov, E., Achilles, S., Schermer, M., et al. (2020a). Desert breath—how fog promotes a novel type of soil biocenosis, forming the coastal Atacama Desert’s living skin. Geobiology 18 (1), 113–124. doi:10.1111/gbi.12368
Jung, P., Mikhailyuk, T., Emrich, D., Baumann, K., Dultz, S., and Büdel, B. (2020c). Shifting boundaries: Ecological and geographical range extension based on three new species in the cyanobacterial genera Cyanocohniella, Oculatella, and, Aliterella. J. Phycol. 56 (5), 1216–1231. doi:10.1111/jpy.13025
Jung, P., Schermer, M., Briegel‐Williams, L., Baumann, K., Leinweber, P., Karsten, U., et al. (2019). Water availability shapes edaphic and lithic cyanobacterial communities in the Atacama Desert. J. Phycol. 55 (6), 1306–1318. doi:10.1111/jpy.12908
Krienitz, L., Huss, V. A., and Bock, C. (2015). Chlorella: 125 years of the green survivalist. Trends plant Sci. 20 (2), 67–69. doi:10.1016/j.tplants.2014.11.005
Lakatos, M., and Strieht, D. (2017). Terrestrial microalgae—novel concepts for biotechnology and applications. Progress in Botany 79, 269–312.
Liu, Y., Cockell, C. S., Wang, G., Hu, C., Chen, L., and De Philippis, R. (2008). Control of lunar and martian dust—Experimental insights from artificial and natural cyanobacterial and algal crusts in the desert of inner Mongolia, China. Astrobiology 8 (1), 75–86. doi:10.1089/ast.2007.0122
Martins, Z., Cottin, H., Kotler, J. M., Carrasco, N., Cockell, C. S., de la Torre Noetzel, R., et al. (2017). Earth as a tool for astrobiology – A European perspective. Space Sci. Rev. 209, 43–81. doi:10.1007/s11214-017-0369-1
McSween, H. Y., Taylor, G. J., and Wyatt, M. B. (2009). Elemental composition of the Martian crust. Science 324 (5928), 736–739. doi:10.1126/science.1165871
Meeßen, J., Sánchez, F. J., Brandt, A., Balzer, E. M., de la Torre, R., Sancho, L. G., et al. (2013). Extremotolerance and resistance of lichens: Comparative studies on five species used in astrobiological research I. Morphological and anatomical characteristics. Orig. Life Evol. Biosph. 43 (3), 283–303. doi:10.1007/s11084-013-9337-2
Miralles, I., Jorge-Villar, S. E., van Wesemael, B., and Lázaro, R. (2017). Raman spectroscopy detection of biomolecules in biocrusts from differing environmental conditions. Spectrochimica Acta Part A Mol. Biomol. Spectrosc. 171, 40–51. doi:10.1016/j.saa.2016.07.035
Muggia, L., Gueidan, C., Knudsen, K., Perlmutter, G., and Grube, M. (2013). The lichen connections of black fungi. Mycopathologia 175 (5), 523–535. doi:10.1007/s11046-012-9598-8
Mugnai, G., Rossi, F., Felde, V. J. M. N. L., Colesie, C., Büdel, B., Peth, S., et al. (2018). Development of the polysaccharidic matrix in biocrusts induced by a cyanobacterium inoculated in sand microcosms. Biol. Fertil. Soils 54 (1), 27–40. doi:10.1007/s00374-017-1234-9
Munzi, S., Varela, Z., and Paoli, L. (2019). Is the length of the drying period critical for photosynthesis reactivation in lichen and moss components of biological soil crusts? J. Arid Environ. 166, 86–90. doi:10.1016/j.jaridenv.2019.04.019
Napoli, A., Micheletti, D., Pindo, M., Larger, S., Cestaro, A., de Vera, J. P., et al. (2022). Absence of increased genomic variants in the cyanobacterium Chroococcidiopsis exposed to Mars-like conditions outside the space station. Sci. Rep. 12 (1), 8437–8439. doi:10.1038/s41598-022-12631-5
Navarro-González, R., Rainey, F. A., Molina, P., Bagaley, D. R., Hollen, B. J., de la Rosa, J., et al. (2003). Mars-like soils in the Atacama Desert, Chile, and the dry limit of microbial life. Science 302 (5647), 1018–1021. doi:10.1126/science.1089143
Neilan, B. A., Burns, B. P., Relman, D. A., and Lowe, D. R. (2002). Molecular identification of cyanobacteria associated with stromatolites from distinct geographical locations. Astrobiology 2 (3), 271–280. doi:10.1089/153110702762027853
Niederwieser, T., Kociolek, P., and Klaus, D. (2018). Spacecraft cabin environment effects on the growth and behavior of Chlorella vulgaris for life support applications. Life Sci. space Res. 16, 8–17. doi:10.1016/j.lssr.2017.10.002
Onofri, S., Selbmann, L., De Hoog, G. S., Grube, M., Barreca, D., Ruisi, S., et al. (2007). Evolution and adaptation of fungi at boundaries of life. Adv. Space Res. 40 (11), 1657–1664. doi:10.1016/j.asr.2007.06.004
Reed, S. C., Coe, K. K., Sparks, J. P., Housman, D. C., Zelikova, T. J., and Belnap, J. (2012). Changes to dryland rainfall result in rapid moss mortality and altered soil fertility. Nat. Clim. Chang. 2 (10), 752–755. doi:10.1038/nclimate1596
Rikkinen, J. (2015). Cyanolichens. Biodivers. Conservation 24 (4), 973–993. doi:10.1007/s10531-015-0906-8
Rodriguez-Caballero, E., Belnap, J., Büdel, B., Crutzen, P. J., Andreae, M. O., Pöschl, U., et al. (2018). Dryland photoautotrophic soil surface communities endangered by global change. Nat. Geosci. 11 (3), 185–189. doi:10.1038/s41561-018-0072-1
Rondanelli, R., Molina, A., and Falvey, M. (2015). The Atacama surface solar maximum. Bull. Am. Meteorological Soc. 96 (3), 405–418. doi:10.1175/bams-d-13-00175.1
Sánchez‐Baracaldo, P., and Cardona, T. (2020). On the origin of oxygenic photosynthesis and Cyanobacteria. New Phytol. 225 (4), 1440–1446. doi:10.1111/nph.16249
Schmidt, S. K., Gendron, E. M. S., Vincent, K., Solon, A. J., Sommers, P., Schubert, Z. R., et al. (2018). Life at extreme elevations on Atacama volcanoes: The closest thing to Mars on Earth? Antonie Leeuwenhoek 111 (8), 1389–1401. doi:10.1007/s10482-018-1066-0
Schulze-Makuch, D., Wagner, D., Kounaves, S. P., Mangelsdorf, K., Devine, K. G., de Vera, J. P., et al. (2018). Transitory microbial habitat in the hyperarid Atacama Desert. Proc. Natl. Acad. Sci. U. S. A. 115 (11), 2670–2675. doi:10.1073/pnas.1714341115
Schweiger, A. H., Ullmann, G. M., Nürk, N. M., Triebel, D., Schobert, R., and Rambold, G. (2022). Chemical properties of key metabolites determine the global distribution of lichens. Ecol. Lett. 25 (2), 416–426. doi:10.1111/ele.13930
Shih, P. M. (2015). Photosynthesis and early Earth. Curr. Biol. 25 (19), R855–R859. doi:10.1016/j.cub.2015.04.046
Sobron, P., Lefebvre, C., Leveille, R., Koujelev, A., Haltigin, T., Du, H., et al. (20122013). Geochemical profile of a layered outcrop in the Atacama analogue using laser‐induced breakdown spectroscopy: Implications for Curiosity investigations in Gale. Geophys. Res. Lett. 40 (10), 1965–1970. doi:10.1002/grl.50261
Sterflinger, K. (1998). Temperature and NaCl-tolerance of rock-inhabiting meristematic fungi. Antonie Leeuwenhoek 74 (4), 271–281. doi:10.1023/a:1001753131034
Stern, J. C., Sutter, B., Freissinet, C., Navarro-Gonza´lez, R., McKay, C. P., Archer, P. D., et al. (2015). Evidence for indigenous nitrogen in sedimentary and aeolian deposits from the Curiosity rover investigations at Gale crater, Mars. Proc. Natl. Acad. Sci. U. S. A. 112 (14), 4245–4250. Epub 20150323. doi:10.1073/pnas.1420932112
Sundarasami, A., Sridhar, A., and Mani, K. (2019). Halophilic archaea as beacon for exobiology: Recent advances and future challenges. Adv. Biol. Sci. Res., 197–214.
Toigo, A. D., and Richardson, M. I. (2000). Seasonal variation of aerosols in the Martian atmosphere. J. Geophys. Res. 105, 4109–4121. doi:10.1029/1999je001132
Treves, H., Raanan, H., Finkel, O. M., Berkowicz, S. M., Keren, N., Shotland, Y., et al. (2013). A newly isolated Chlorella sp. from desert sand crusts exhibits a unique resistance to excess light intensity. FEMS Microbiol. Ecol. 86 (3), 373–380. doi:10.1111/1574-6941.12162
Verseux, C., Baqué, M., Cifariello, R., Fagliarone, C., Raguse, M., Moeller, R., et al. (2017). Evaluation of the resistance of Chroococcidiopsis spp. to sparsely and densely ionizing irradiation. Astrobiology 17, 118–125. doi:10.1089/ast.2015.1450
Verseux, C., Baque´, M., Lehto, K., de Vera, J-P. P., Rothschild, L. J., and Billi, D. (2016). Sustainable life support on Mars—The potential roles of cyanobacteria. Int. J. Astrobiol. 15, 65–92. doi:10.1017/s147355041500021x
Viúdez-Moreiras, D., Gómez-Elvira, J., Newman, C. E., Navarro, S., Marin, M., Torres, J., et al. (2019). Gale surface wind characterization based on the Mars Science Laboratory REMS dataset. Part II: Wind probability distributions. Icarus 319, 645–656. doi:10.1016/j.icarus.2018.10.010
Wamelink, G. W., Frissel, J. Y., Krijnen, W. H., Verwoert, M. R., and Goedhart, P. W. (2014). Can plants grow on Mars and the moon: A growth experiment on Mars and moon soil simulants. PLoS One 9 (8), e103138. Epub 2014/08/27PMID: 25162657; PubMed Central PMCID:PMC4146463. doi:10.1371/journal.pone.0103138
Wang, B., Ye, T., Li, X., Bian, P., Liu, Y., and Wang, G. (2021). Survival of desert algae Chlorella exposed to Mars-like near space environment. Life Sci. Space Res. 29, 22–29. doi:10.1016/j.lssr.2021.02.003
Weber, B., Belnap, J., Büdel, B., Antoninka, A. J., Barger, N. N., Chaudhary, V. B., et al. (2022). What is a biocrust? A refined, contemporary definition for a broadening research community. Biol. Rev. 97, 1768–1785. doi:10.1111/brv.12862
White, B. R. (1979). Soil transport by winds on Mars. J. Geophys. Res. 84, 4643–4651. doi:10.1029/jb084ib09p04643
Xin, Z. H. A. O., Yong, S. H. I., Yang, L. I. U., Rong-Liang, J. I. A., and Xin-Rong, L. I. (2015). Osmotic adjustment of soil biocrust mosses in response to desiccation stress. Pedosphere 25 (3), 459–467. doi:10.1016/s1002-0160(15)30013-8
Keywords: grit crust, astrobiology, Atacama, Mars, cyanobacteria, lichens, green algae
Citation: Jung P, Lehnert LW, Bendix J, Lentendu G, Grube M, Alfaro FD, Rio Cd, Gutiérrez Alvarado JL, van den Brink L and Lakatos M (2022) The grit crust: A poly-extremotolerant microbial community from the Atacama Desert as a model for astrobiology. Front. Astron. Space Sci. 9:1052278. doi: 10.3389/fspas.2022.1052278
Received: 23 September 2022; Accepted: 26 October 2022;
Published: 09 November 2022.
Edited by:
Alberto Fairén, Center for Astrobiology (CSIC), SpainReviewed by:
Roberto Barbieri, University of Bologna, ItalyJean-Pierre Paul de Vera, German Aerospace Center (DLR), Germany
Copyright © 2022 Jung, Lehnert, Bendix, Lentendu, Grube, Alfaro, Rio, Gutiérrez Alvarado, van den Brink and Lakatos. This is an open-access article distributed under the terms of the Creative Commons Attribution License (CC BY). The use, distribution or reproduction in other forums is permitted, provided the original author(s) and the copyright owner(s) are credited and that the original publication in this journal is cited, in accordance with accepted academic practice. No use, distribution or reproduction is permitted which does not comply with these terms.
*Correspondence: Patrick Jung, UGF0cmlja19qdW5nOTBAd2ViLmRl