- 1Laboratory for Atmospheric and Space Physics, University of Colorado Boulder, Boulder, CO, United States
- 2Department of Atmospheric and Oceanic Sciences, College of Arts and Sciences, University of Colorado Boulder, Boulder, CO, United States
- 3Center for Space Science and Engineering Research and Bradley Department of Electrical and Computer Engineering, Virginia Tech, Blacksburg, VA, United States
- 4Northwest Research Associates Inc., Boulder, CO, United States
- 5Leibniz Institute of Atmospheric Physics, University of Rostock, Külungsborn, Germany
- 6Haystack Observatory, Massachusetts Institute of Technology, Westford, MA, United States
- 7Global Atmospheric Technologies and Sciences Inc., Newport News, VA, United States
- 8Centre for Space, Atmospheric and Oceanic Science, University of Bath, Bath, United Kingdom
- 9NASA Goddard Space Flight Center, Greenbelt, MD, United States
- 10High Altitude Observatory, National Center for Atmospheric Research, Boulder, CO, United States
- 11Department of Meteorology, Stockholm University, Stockholm, Sweden
- 12Department of Aerospace Engineering Sciences, University of Colorado Boulder, Boulder, CO, United States
- 13Naval Research Laboratory, Space Science Division, Washington, DC, United States
- 14Atmospheric Chemistry Observations and Modeling Laboratory, National Center for Atmospheric Research, Boulder, CO, United States
- 15Institute of Applied Physics and Oeschger Center for Climate Change Research, Microwave Physics, University of Bern, Bern, Switzerland
- 16Department of Physics, Catholic University of America, Washington, DC, United States
The mesospheric polar vortex (MPV) plays a critical role in coupling the atmosphere-ionosphere system, so its accurate simulation is imperative for robust predictions of the thermosphere and ionosphere. While the stratospheric polar vortex is widely understood and characterized, the mesospheric polar vortex is much less well-known and observed, a short-coming that must be addressed to improve predictability of the ionosphere. The winter MPV facilitates top-down coupling via the communication of high energy particle precipitation effects from the thermosphere down to the stratosphere, though the details of this mechanism are poorly understood. Coupling from the bottom-up involves gravity waves (GWs), planetary waves (PWs), and tidal interactions that are distinctly different and important during weak vs. strong vortex states, and yet remain poorly understood as well. Moreover, generation and modulation of GWs by the large wind shears at the vortex edge contribute to the generation of traveling atmospheric disturbances and traveling ionospheric disturbances. Unfortunately, representation of the MPV is generally not accurate in state-of-the-art general circulation models, even when compared to the limited observational data available. Models substantially underestimate eastward momentum at the top of the MPV, which limits the ability to predict upward effects in the thermosphere. The zonal wind bias responsible for this missing momentum in models has been attributed to deficiencies in the treatment of GWs and to an inaccurate representation of the high-latitude dynamics. In the coming decade, simulations of the MPV must be improved.
Introduction
While the stratospheric polar vortex has been extensively studied since the 1950s (e.g., Labitzke & Naujokat, 2000 and references therein), it was only recently documented that the polar vortex also extends well into the mesosphere (Harvey et al., 2018). Figure 1 shows that the polar vortex as depicted in the 2013 decadal survey only extends up to the stratopause. It is now known that the polar vortex broadens with increasing altitude into the upper mesosphere. High-top models such as the Whole Atmosphere Community Climate Model (WACCM) properly simulate the mesospheric polar vortex (MPV) up to middle mesospheric altitudes, but fail to reproduce observations above ∼80 km (Harvey et al., 2019; Hindley et al., 2022) especially when the vortex is strong (Harvey et al., 2022). At winter mesopause altitudes the upper-most reaches of the polar vortex can manifest as troughs in traveling planetary waves (PWs) (Harvey et al., 2021). Descent in the longitude sectors of these wave troughs into the top of the MPV can be 5 times stronger than at other longitudes. While much progress has been made in diagnosing and understanding the vortex in the mesosphere, more work is needed to fully characterize both its mean state and variability and how it is coupled to regions both above and below.
The MPV often behaves differently than the vortex in the stratosphere; the MPV can be strong when the stratospheric vortex is weak, and vice versa. It is not yet known if MPV strength could be a predictor for variability in the ionosphere and thermosphere (IT) system, but sudden stratospheric warming (SSW)-induced variability in the mesosphere has been associated with dynamical variability at stratopause altitudes (e.g., Tweedy et al., 2013; Stray et al., 2015; Limpasuvan et al., 2016; Zülicke et al., 2018) rather than at 10 hPa where SSWs are traditionally defined. This suggests that dynamical proxies defined at the base of the MPV may be a better predictor of IT variability than SSW definitions.
The energetic particle precipitation “indirect effect”
The MPV plays an important role in coupling the atmosphere-ionosphere system from the top-down. As depicted in Figure 1, the MPV acts to couple the atmosphere via the transport of nitrogen oxides (NOx) produced by energetic particle precipitation (EPP) from the mesosphere and lower thermosphere (MLT) down to the stratosphere where the NOx can destroy ozone. Understanding why models underestimate this EPP “indirect effect” was identified as a priority in the last decadal survey but has yet to be fully realized (Randall et al., 2015; Pettit et al., 2019, 2021). Underestimates in simulated NOx are likely due to a combination of erroneous transport (Siskind et al., 2015) and electron source specifications.
Since the last decadal survey, studies have focused on eliminating model underestimates in the descent of NOx in the MPV. For example, Smith-Johnsen et al. (2022) modified model dynamics by decreasing the amplitude of non-orographic gravity waves (GWs) and decreasing the Prandtl number (a measure of vertical mixing by GW breaking), both of which resulted in better agreement with nitric oxide (NO) observations in the polar winter mesosphere. In the mesosphere, NOx is primarily comprised of NO. On the other hand, Pettit et al. (2021) showed that including medium energy electron (MEE) sources of ionization in WACCM resulted in better agreement between simulated and observed NO concentrations in the polar winter mesosphere, though midlatitude NO was still underestimated in the model. A study that imposes both improved dynamics and MEE sources is long overdue.
Lower atmosphere impacts on the ionosphere and thermosphere system depend on vortex strength
It is well known that the polar vortex modulates GW and PW fluxes and tidal amplitudes and that each of these waves behaves differently during weak vs. strong polar vortex states (e.g., Pedatella & Harvey, 2022). A weakening or reversal of the polar night jet (PNJ) during SSWs leads to anomalous GW propagation and dissipation that, in turn, modifies the global residual circulation and can lead to cooling in the polar winter mesosphere (Labitzke, 1972). Tides are strongly modified during weak polar vortex states due to the changes in propagation conditions related to zonal wind variations in the stratosphere and mesosphere (Jin et al., 2012; Pedatella & Liu, 2013), due to modulation through nonlinear interactions with PWs (Lieberman et al., 2015), and due to increases in ozone in the tropical stratosphere (Goncharenko et al., 2012; Siddiqui et al., 2019). Goncharenko et al. (2010), Chau et al. (2012), and Siddiqui et al. (2015) illustrate extreme ionospheric variability during SSWs when the vortex is weak and demonstrate that changes in the strength of the polar vortex are associated with tidal modulation in the MLT region and large anomalous variations in the equatorial electrojet, vertical ion drift, total electron content and peak electron density. In the last decade, many other studies have confirmed and expanded upon these provocative results (Goncharenko et al., 2021). Variations in the low-latitude IT system are well documented and better understood; variations at middle latitudes are less studied and understood as they are produced by different competing mechanisms, and variations at high latitudes are understood the least due to the relative shortage of data and high sensitivity of polar regions to geomagnetic conditions. While much progress has been made in understanding the far-reaching effects of weak polar vortices on variability throughout the atmosphere-ionosphere system (e.g., Pedatella et al., 2018), this is only the tip of the iceberg.
The polar vortex is a source of gravity waves that can lead to traveling ionospheric disturbances
The geographic distribution of GWs in the polar winter stratosphere depends strongly on the location, strength, and stability of the PNJ that encircles the polar vortex. These waves are prevalent in the vortex jet region because 1) persistent westerlies from the surface to the mid stratosphere allow tropospheric GWs to propagate vertically without breaking, 2) GW propagation directions are focused toward faster wind speeds (Sato et al., 2009), and 3) GWs are refracted to longer vertical wavelengths, so they can grow to larger amplitudes before breaking (Whiteway et al., 1997). These provide ideal conditions for surface-generated GWs to reach the mesosphere. GWs may also be generated in-situ in the PNJ by local instabilities in the jet exit region (Plougonven and Snyder, 2007) or as secondary GWs (SGWs) generated by breaking primary GWs above the jet core (Becker and Vadas, 2018). Generation and modulation of GWs by the fast winds at the polar vortex edge has been shown to give rise to traveling ionospheric disturbances (TIDs) (Becker et al., 2022b). Frissell et al. (2016) showed that TID activity depends on vortex strength rather than geomagnetic activity, and is observable on two to 4-week time scales; subsequent studies are consistent with these results (Yasyukevich, et al., 2017; Nayak and Yiğit, 2019). Since the state of the polar vortex can be forecasted out 2 weeks with some accuracy (Domeisen et al., 2020), the vortex-TID relationship adds predictability to the ionosphere.
The problem
Unfortunately, representation of the polar vortex in the upper mesosphere is generally not accurate in state-of-the-art global models. In fact, in many models the zonal winds blow in the wrong direction in the polar winter upper mesosphere (Harvey et al., 2022 and references therein) compared to observations. Important impacts of this easterly (westward) wind bias are 1) a reduction in the vertical extent of the MPV (Harvey et al., 2019), 2) an increase in the vertical wind shear, which alters the spectrum of GWs and PWs (e.g., Chandran et al., 2013), 3) persistent negative meridional potential vorticity gradients at mid-to-high latitudes, which can generate PWs via baroclinic or barotropic instability (e.g., Charney and Stern, 1962), and 4) a reduction in the amplitude of the migrating wavenumber two semidiurnal tide (SW2) in Arctic winter (Zhang et al., 2021).
It is strongly suspected that the easterly wind bias is due to inaccurate or incomplete treatment of parameterized GWs in community models. This limits the use of such models to study the role of the MPV in constituent transport, wave-mean flow interactions, and vertical coupling mechanisms in the atmosphere-ionosphere system. An interesting aspect of the model easterly wind bias is that it varies as a function of time and is most egregious when the vortex is strong (Harvey et al., 2022). Figure 2 illustrates the relevant zonal wind and GW filtering processes during strong (left) and weak (right) polar vortices. Between 80 and 100 km the modeled and observed zonal winds blow in opposite directions when the vortex is strong, whereas there is reasonable agreement between the model and observations when the vortex is weak (during SSWs). Harvey et al. (2022) provide a detailed discussion of the GW filtering mechanisms, which is summarized in the caption of Figure 2.
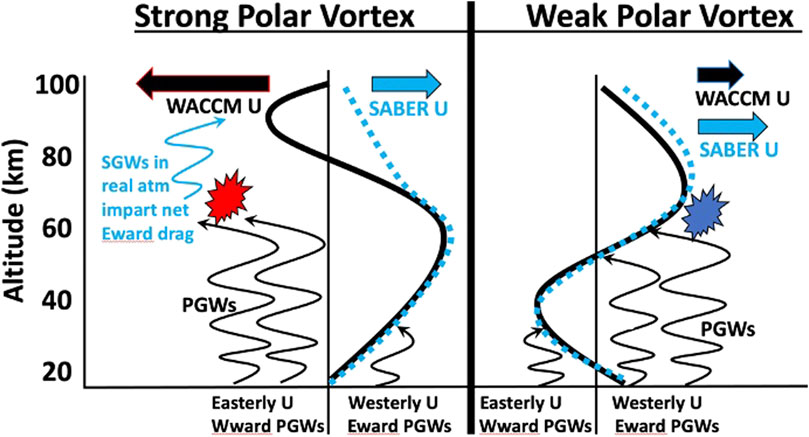
FIGURE 2. Schematic illustrating zonal winds and GW processes that modulate the easterly model wind bias in the MLT when the vortex is strong (left) and weak (right). Typical WACCM zonal wind profiles are given in thick black lines. Sounding of the Atmosphere using Broadband Emission Radiometry (SABER) observed zonal wind profiles are given in blue dashed lines. GWs with phase speeds opposite to the zonal wind propagate upward and dissipate. The red (blue) star denotes westward (eastward) GW drag due to the breaking of westward (eastward) primary GWs when the vortex is strong (weak) and zonal winds in the stratosphere are eastward (westward). PGW = Primary Gravity Wave. SGW = Secondary Gravity Wave.
One leading hypothesis for the model easterly wind bias in the MLT is that it could be due to an incomplete representation of GW effects, in particular SGWs. Becker and Vadas (2018) showed that there is a significant eastward drag from SGWs in the winter MLT (which cannot be due to primary GWs) that is absent in models, and that the easterly wind bias during strong polar vortex conditions is eliminated when SGW effects are included. Thus, missing eastward forcing from SGWs may account for the easterly wind bias in conventional high-top models. Other factors that may contribute to the easterly wind bias in the model include: the absence of oblique GW propagation (e.g., Sato et al., 2009), the need for anisotropic GW source spectra (e.g., Liu & Roble, 2002; Pramitha et al., 2020), the need to impose GW sources at all altitudes (e.g., Ribstein et al., 2022) including the tropospheric jets and the stratospheric polar vortex (e.g., Sato & Yoshiki, 2008), and the need to tune GW parameterizations according to simulated tidal variability (e.g., Becker, 2017).
Discussion
In the coming decade, more extensive wind, temperature, and constituent observations of the MPV are needed as well as scientific studies that utilize both ground-based and space-based observing techniques. In addition to observations of the MPV, spaceborne limb and nadir viewing GW observations (e.g., Kogure et al., 2020) at mid-high latitudes would also be useful to validate simulated GW distributions. Further, new frameworks of GW parameterizations are required to properly simulate the zonal wind in the polar winter upper mesosphere (e.g., Bölöni et al., 2021). Indeed, sufficient observations exist to know the modeled MPV is incorrect, but there are not sufficient observations to determine why the models are incorrect or how to fix them.
A full observational characterization of the MPV in the MLT and at all longitudes with high temporal resolution (hours) is still elusive. Typical sun-synchronous space-borne observations provide only one to two soundings per day at a given location at fixed local times (Livesey et al., 2022). This can determine the mean wind and PW activity, but renders investigations of tidal diurnal and day-to-day variability unfeasible. 24-h sampling is needed to characterize tidal evolution in tandem with the MPV, and to prevent tidal aliasing of zonal mean temperatures and balanced wind calculations. Ground-based observations provide sufficiently high time cadence to assess short-timescale variability caused by the superposition of migrating and non-migrating tides and GWs, but lack the spatial coverage to provide unambiguous PW wavenumber identification.
We propose new satellite measurements to fully characterize the MPV. Observations show that the MPV can extend to ∼30° latitude in the winter hemisphere, display a PW wavenumber one pattern in longitude, and extend to at least 80 km (Harvey et al., 2018). Manifestations of the vortex as troughs in traveling PWs also appear at 90 km (Harvey et al., 2021) and vortex signatures in geopotential height can appear as high as 100 km. Harvey et al. (2015) defined the MPV using horizontal gradients in carbon monoxide (CO) observed by the Microwave Limb Sounder (MLS). However, if the vortex extends above the top of the global residual circulation where descent and horizontal CO gradients weaken, then horizontal winds become necessary to identify the vortex edge. Therefore, both horizontal vector winds and CO are required. We propose satellite observations of these observables that span the winter hemisphere from 50–110 km with sufficient spatial and temporal sampling to characterize diurnal and SW2 variations (every 4–6 h). This temporal coverage will likely require a constellation of two or more satellites similar to the DYNAMIC mission concept outlined in the 2013 decadal survey. These new observations will allow for the unambiguous identification of the MPV as a function of longitude, latitude, altitude, and local time and this will, in turn, support a wide range of scientific studies.
The MPV needs to be accurately simulated. Increased model horizontal and vertical resolution, combined with advanced methods to parameterize sub-grid scales and SGWs, enables the explicit simulation of a new part of the GW spectrum and can eliminate the easterly wind bias (Becker & Vadas, 2018; Liu et al., 2022). However, these models are computationally expensive and GWs remain under-resolved, even at the highest model resolutions. Therefore, it is critical to improve GW parameterizations in the next decade by enhancing model physics related to processes that govern the generation, propagation, and dissipation of GWs. In addition to improved representation of the MPV, improved GW parameterizations would lead to better representation of the mean circulation, chemistry, and large-scale wave dynamics throughout the middle-upper atmosphere. Below is a non-exhaustive list of proposed improvements to current GW parameterizations.
1. Allow oblique GW propagation.
2. Test the impact of anisotropic GW source spectra on polar winter mesopause winds.
3. Include tropospheric jet exit regions and the polar vortex as GW sources.
4. Develop a new framework to better simulate the generation, propagation, and dissipation of higher order GWs.
5. Improve the simulation of GW-tidal interactions.
Model underestimates in the downward transport of EPP-NOx need to be understood and corrected. Precipitating electron source specification in models needs to include electrons with energies >30 keV. Further, we need to understand the role of the MPV in the containment of nitric oxide, how efficient it is, and over what altitude range. While there has been progress in characterizing the mean state of the MPV, more needs to be done to understand its hourly, daily, seasonal, and interannual variability. A full characterization of local and remote effects during weak and strong vortex events needs to be undertaken. Measurements and models need to be used in conjunction to fully appreciate the mechanisms governing the GW-TID relationship and its dependence on polar vortex strength. A non-exhaustive list of recommended science studies is given below.
1. Evaluate the sensitivity of the easterly wind bias to model horizontal resolution, vertical resolution, and physics-based sub-grid-scale parameterizations. Compare high-resolution GW-resolving models to models with parameterized GWs to understand how polar winter mesopause zonal winds are related to GW effects. Test the hypothesis that eastward momentum deposition from SGWs is necessary to bring models closer to observations.
2. Use simulations from high-resolution global models to identify discrepancies between resolved and parameterized GWs and their impacts on the vortex.
3. Compare observed to modelled GW momentum flux. Because observations can only observe a limited part of the GW spectrum, it is essential to sample model outputs as the observations to make like-for-like comparisons.
4. Combine new satellite observations of the MPV with observations made by ground-based array systems such as SuperDARN meteor radars to understand how the small and large scales evolve together and separately.
5. Identify the MPV as a function of longitude, latitude, altitude, and local time. When and how often does the MPV extend into the lower thermosphere? How predictable is it?
6. Determine the extent to which MPV strength is a predictor for variability in the IT system. Use long-term ionospheric records to quantify daily/weekly ionospheric predictability.
7. In current GW schemes, rapid vertical wave mixing in the MLT is likely underestimated by over an order of magnitude (Liu, 2021). Include this rapid vertical mixing due to higher order GWs into chemistry climate models (e.g., WACCM) and quantify the extent to which the EPP-NOx underestimate is alleviated.
8. Determine how the polar vortex contributes as a source of primary GWs during strong vortex states. For example, Liu (2017) and Becker et al. (2022a) observed/simulated in-situ generation of GWs by a disturbed polar vortex.
9. Quantify diffusive vs. non-local advective transport of EPP-NOx in the polar winter upper mesosphere. Resolve the controversy whereby Smith et al. (2011) showed eddy diffusion to be dominant whereas Meraner & Schmidt (2016) concluded that transport by molecular diffusion and vertical advection dominated.
How will the advances outlined above prepare the aeronomy community for future decades?
1. MLT wind measurements at 6 + local times per day will provide sufficient temporal resolution to characterize day-to-day tidal winds within which the MPV is embedded. These measurements will also provide a much-needed constraint on models in the MLT.
2. Model improvements to the representation of the MPV will have far-reaching impacts. They will enable a wide variety of scientific studies involving GWs, PWs, and tides, atmosphere-ionosphere vertical coupling and teleconnections, and constituent transport.
3. Characterization of the MPV will elucidate vertical transport of trace gases from the MLT to the stratosphere and mesosphere, will be useful for studies of wave-mean flow interaction, and will provide a meteorological context for GWs generated and modulated by wind shears at the vortex edge that lead to TID activity.
Given the need to both observe and accurately simulate the MPV, and the current inability to do so, we summarize the following plan for moving forward:
1. Solicit mission proposals to measure temperature, winds, and trace gases in the MLT. NASA critically needs a follow-on to MLS and SABER to observe MLT dynamics and chemistry, especially at high latitudes beyond the scope of ICON. Such a mission should consist of a constellation of satellites that provide sufficient sampling to quantify daily tidal variations.
2. Encourage international participation in the deployment of more ground-based observing platforms to complement satellite-based observations and provide high temporal and spatial resolution measurements of the MLT.
3. Solicit studies that explicitly simulate more of the GW spectrum, or more realistic GW generation, propagation, dissipation and higher order GW generation processes in general circulation models. Evaluate model results by comparing to available observations.
Data availability statement
The original contributions presented in the study are included in the article/supplementary material, further inquiries can be directed to the corresponding author.
Author contributions
VLH wrote the first draft of this manuscript and created Figure 2. All other authors contributed to revised versions of the text.
Funding
This research has been supported by the National Aeronautics and Space Administration (Grant Nos 80NSSC18K1046, 80NSSC190262, 80NSSC19K0834, 80NSSC20K0628, 80NSSC21K0002, 80NSSC22K1074, and 80NSSC22K0017) and the National Science Foundation (grant AGS 1651428). This material is based upon work supported by the National Center for Atmospheric Research, which is a major facility sponsored by the U.S. National Science Foundation under Cooperative Agreement 1852977. High-end computing resources were provided by NASA to run WACCMX + DART on the Pleiades supercomputer at the NASA Ames research center.
Conflict of interest
EB is employed by the Northwest Research Associates, Inc. and LLG is employed by Global Atmospheric Technologies and Sciences, Inc.
The remaining authors declare that the research was conducted in the absence of any commercial or financial relationships that could be construed as a potential conflict of interest.
Publisher’s note
All claims expressed in this article are solely those of the authors and do not necessarily represent those of their affiliated organizations, or those of the publisher, the editors and the reviewers. Any product that may be evaluated in this article, or claim that may be made by its manufacturer, is not guaranteed or endorsed by the publisher.
References
Becker, E., Goncharenko, L., Harvey, V. L., and Vadas, S. (2022b). Multi-step vertical coupling during the January 2017 sudden stratospheric warming. J. Geophys. Res. Space Phys. accepted. doi:10.1029/2022JA030866
Becker, E. (2017). Mean-flow effects of thermal tides in the mesosphere and lower thermosphere. J. Atmos. Sci. 74, 2043–2063. doi:10.1175/JAS-D-16-0194.1
Becker, E., Vadas, S. L., Bossert, K., Harvey, V. L., Zülicke, C., and Hoffmann, L. (2022a). A high‐resolution whole‐atmosphere model with resolved gravity waves and specified large‐scale dynamics in the troposphere and stratosphere. JGR. Atmos. 127. doi:10.1029/2021JD035018
Becker, E., and Vadas, S. L. (2018). Secondary gravity waves in the winter mesosphere: Results from a high‐resolution global circulation model. J. Geophys. Res. Atmos. 123, 2605–2627. doi:10.1002/2017JD027460
Bölöni, G., Kim, Y.-H., Borchert, S., and Achatz, U. (2021). Toward transient subgrid-scale gravity wave representation in atmospheric models. Part I: Propagation model including nondissipative wave–mean-flow interactions. J. Atmos. Sci. 78, 1317–1338. doi:10.1175/JAS-D-20-0065.1
Chandran, A., Collins, R. L., and Harvey, V. L. (2014). Stratosphere-mesosphere coupling during stratospheric sudden warming events. Adv. Space Res. 53 (9), 1265–1289. doi:10.1016/j.asr.2014.02.005
Chandran, A., Garcia, R. R., Collins, R. L., and Chang, L. C. (2013). Secondary planetary waves in the middle and upper atmosphere following the stratospheric sudden warming event of January 2012. Geophys. Res. Lett. 40, 1861–1867. doi:10.1002/grl.50373
Charney, J. G., and Stern, M. E. (1962). On the stability of internal baroclinic jets in a rotating atmosphere. J. Atmos. Sci. 19, 159–172. doi:10.1175/1520-0469(1962)019<0159:otsoib>2.0.co;2
Chau, J. L., Goncharenko, L. P., Fejer, B. G., and Liu, H. L. (2012). Equatorial and low latitude ionospheric effects during sudden stratospheric warming events ionospheric effects during SSW events. Space Sci. Rev. 168 (1-4), 385–417. doi:10.1007/s11214-011-9797-5
Domeisen, D. I., Butler, A. H., Charlton-Perez, A. J., Ayarzagüena, B., Baldwin, M. P., Dunn-Sigouin, E., et al. (2020). The role of the stratosphere in subseasonal to seasonal prediction: 1. Predictability of the stratosphere. J. Geophys. Res. Atmos. 125, e2019JD030920. doi:10.1029/2019JD030920
Frissell, N. A., Baker, J. B. H., Ruohoniemi, J. M., Greenwald, R. A., Gerrard, A. J., Miller, E. S., et al. (2016). Sources and characteristics of medium-scale traveling ionospheric disturbances observed by high-frequency radars in the North American sector. J. Geophys. Res. Space Phys. 121, 3722–3739. doi:10.1002/2015JA022168
Goncharenko, L., Coster, A., Plumb, R., and Domeisen, D. (2012). The potential role of stratospheric ozone in the stratosphere-ionosphere coupling during stratospheric warmings. Geophys. Res. Lett. 39, L08101. doi:10.1029/2012GL051261
Goncharenko, L. P., Chau, J. L., Liu, H. L., and Coster, A. J. (2010). Unexpected connections between the stratosphere and ionosphere. Geophys. Res. Lett. 37. doi:10.1029/2010GL043125
Goncharenko, L. P., Harvey, V. L., Liu, H., and Pedatella, N. M. (2021). “Sudden stratospheric warming impacts on the ionosphere–thermosphere system,” in Ionosphere dynamics and applications (American Geophysical Union Geophysical Monograph Series). Chapter 16, 369–400. doi:10.1002/9781119815617.ch16
Harvey, V. L., Datta-Barua, S., Pedatella, N., Wang, N., Randall, C. E., Siskind, D. E., et al. (2021). Transport of nitric oxide via Lagrangian Coherent Structures into the top of the polar vortex. J. Geophys. Res. Atmos. 126, e2020JD034523. doi:10.1029/2020JD034523
Harvey, V. L., Pedatella, N. M., Becker, E., and Randall, C. E. (2022). Evaluation of polar winter mesopause wind in WACCMX+DART. J. Geophys. Res. Atmos. 127, e2022JD037063. doi:10.1029/2022JD037063
Harvey, V. L., Randall, C. E., Becker, E., Smith, A. K., Bardeen, C. G., France, J. A., et al. (2019). Evaluation of the mesospheric polar vortices in WACCM. J. Geophys. Res. Atmos. 124, 10,626–10,645. doi:10.1029/2019JD030727
Harvey, V. L., Randall, C. E., and Collins, R. L. (2015). Chemical definition of the mesospheric polar vortex. J. Geophys. Res. Atmos. 120, 10,166–10,179. doi:10.1002/2015JD023488
Harvey, V. L., Randall, C. E., Goncharenko, L., Becker, E., and France, J. (2018). On the upward extension of the polar vortices into the mesosphere. J. Geophys. Res. Atmos. 123, 9171–9191. doi:10.1029/2018JD028815
Hindley, N. P., Cobbett, N., Fritts, D. C., Janches, D., Mitchell, N. J., Moffat-Griffin, T., et al. (2022). Radar observations of winds, waves and tides in the mesosphere and lower thermosphere over South Georgia island (54°S, 36°W) and comparison to WACCM simulations. Atmos. Chem. Phys. 22, 9435–9459. doi:10.5194/acp-22-9435-2022
Jin, H., Miyoshi, Y., Pancheva, D., Mukhtarov, P., Fujiwara, H., and Shinagawa, H. (2012). Response of migrating tides to the stratospheric sudden warming in 2009 and their effects on the ionosphere studied by a whole atmosphere- ionosphere model GAIA with COSMIC and TIMED/SABER observations. J. Geophys. Res. 117 (10). doi:10.1029/2012JA017650
Kogure, M., Yue, J., Nakamura, T., Hoffmann, L., Vadas, S. L., Tomikawa, Y., et al. (2020). First direct observational evidence for secondary gravity waves generated by mountain waves over the andes. Geophys. Res. Lett. 47. doi:10.1029/2020gl088845
Labitzke, K., and Naujokat, B. (2000). The lower arctic stratosphere in winter since 1952. SPARC Newsl. 15, 11–14. Available at: https://www.atmosp.physics.utoronto.ca/SPARC/News15/15_Labitzke.html.
Labitzke, K. (1972). Temperature changes in the mesosphere and stratosphere connected with circulation changes in winter. J. Atmos. Sci. 29, 756–766. doi:10.1175/1520-0469(1972)029<0756:tcitma>2.0.co;2
Lieberman, R. S., Fritts, D. C., Pedatella, N., Doornbos, E., and Ortland, D. A. (2015). Global observations of thermospheric lunar tidal winds. J. Atmos. Sol. Terr. Phys. 136, 126–133. doi:10.1016/j.jastp.2015.05.019
Limpasuvan, V., Orsolini, Y. J., Chandran, A., Garcia, R. R., and Smith, A. K. (2016). On the composite response of the MLT to major sudden stratospheric warming events with elevated stratopause. J. Geophys. Res. Atmos. 121, 4518–4537. doi:10.1002/2015JD024401
Liu, H.-L. (2021). Effective vertical diffusion by atmospheric gravity waves. Geophys. Res. Lett. 48, e2020GL091474. doi:10.1029/2020GL091474
Liu, H.-L. (2017), Gravity wave variation from the troposphere to the lower thermosphere during a stratospheric sudden warming event: A case study. Sci. Online Lett. Atmos. 13A. Issue Special Edition, 24–30. doi:10.2151/sola.13A-005
Liu, H.-L., Lauritzen, P., Vitt, F., and Goldhaber, S. (2022). Thermospheric and ionospheric effects by gravity waves from the lower atmosphere. Submitt. J. Geophys. Res. Space Phys. doi:10.1002/essoar.10511744.1
Liu, H.-L., and Roble, R. G. (2002). A study of a self-generated stratospheric sudden warming and its mesospheric – lower thermospheric impacts using the coupled TIME-GCM/CCM3. J. -Geophys. -Res. 107 (23), ACL 15-21–ACL 15-18. doi:10.1029/2001JD001533
Livesey, N. J., Read, W. G., Wagner, P. A., Froidevaux, L., Santee, M. L., Schwartz, M. J., et al. (2022). Version 5.0x Level 2 and 3 data quality and description document (Tech. Rep.). Pasadena, California: Jet Propulsion Laboratory.
Meraner, K., and Schmidt, H. (2016). Transport of nitrogen oxides through the winter mesopause in HAMMONIA. J. Geophys. Res. Atmos. 121, 2556–2570. doi:10.1002/2015JD024136
Nayak, C., and Yiğit, E. (2019). Variation of small-scale gravity wave activity in the ionosphere during the major sudden stratospheric warming event of 2009. J. Geophys. Res. Space Phys. 123, 470–488. doi:10.1029/2018JA026048
Pedatella, N. M., Chau, J. L., Schmidt, H., Goncharenko, L. P., Stolle, C., Hocke, K., et al. (2018). How sudden stratospheric warming affects the whole atmosphere. EoS 99. doi:10.1029/2018eo092441
Pedatella, N. M., and Harvey, V. L. (2022). Impact of strong and weak stratospheric polar vortices on the mesosphere and lower thermosphere. Geophys. Res. Lett. 49. doi:10.1029/2022GL098877
Pedatella, N. M., and Liu, H.-L. (2013). The influence of atmospheric tide and planetary wave variability during sudden stratosphere warmings on the low latitude ionosphere. J. Geophys. Res. Space Phys. 118, 5333–5347. doi:10.1002/jgra.50492
Pettit, J. M., Randall, C. E., Peck, E. D., and Harvey, V. L. (2021). A new MEPED-based Precipitating Electron data set. J. Geophys. Res. Space Phys. 126, e2021JA029667. doi:10.1029/2021JA029667
Pettit, J. M., Randall, C. E., Peck, E. D., Marsh, D. R., van de Kamp, M., Fang, X., et al. (2019). Atmospheric effects of >30‐keV energetic electron precipitation in the Southern Hemisphere winter during 2003. JGR. Space Phys. 124, 8138–8153. doi:10.1029/2019JA026868
Plougonven, R., and Snyder, C. (2007). Inertia–gravity waves spontaneously generated by jets and fronts. Part I: Different baroclinic life cycles. J. Atmos. Sci. 64, 2502–2520. doi:10.1175/JAS3953.1
Pramitha, M., Kumar, K. K., Ratnam, M. V., Praveen, M., and Rao, S. V. B. (2020). Gravity wave source spectra appropriation for mesosphere lower thermosphere using meteor radar observations and GROGRAT model simulations. Geophys. Res. Lett. 47 (19), e2020GL089390. doi:10.1029/2020GL089390
Randall, C. E., Harvey, V. L., Holt, L. A., Marsh, D. R., Kinnison, D., Funke, B., et al. (2015). Simulation of energetic particle precipitation effects during the 2003-2004 Arctic winter. JGR. Space Phys. 120, 5035–5048. doi:10.1002/2015JA021196
Ribstein, B., Millet, C., Lott, F., and de la Cámara, A. (2022). Can we improve the realism of gravity wave parameterizations by imposing sources at all altitudes in the atmosphere? J. Adv. Model. Earth Syst. 14, e2021MS002563. doi:10.1029/2021MS002563
Sato, K., Watanabe, S., Kawatani, Y., Tomikawa, Y., Miyazaki, K., and Takahashi, M. (2009). On the origins of mesospheric gravity waves. Geophys. Res. Lett. 36 (19), L19801. doi:10.1029/2009gl039908
Sato, K., and Yoshiki, M. (2008). Gravity wave generation around the polar vortex in the stratosphere revealed by 3-hourly radiosonde observations at Syowa Station. J. Atmos. Sci. 65, 3719–3735. doi:10.1175/2008JAS2539.1
Siddiqui, T. A., Maute, A., and Pedatella, N. M. (2019). On the importance of interactive ozone chemistry in earth‐system models for studying mesophere‐lower thermosphere tidal changes during sudden stratospheric warmings. JGR. Space Phys. 124 (12), 10690–10707. doi:10.1029/2019JA027193
Siddiqui, T., Stolle, C., Lühr, H., and Matzka, J. (2015). On the relationship between weakening of the northern polar vortex and the lunar tidal amplification in the equatorial electrojet. J. Geophys. Res. Space Phys. 120 (11), 10006–10019. doi:10.1002/2015JA021683
Siskind, D. E., Sassi, F., Randall, C. E., Harvey, V. L., Hervig, M. E., and Bailey, S. M. (2015). Is a high-altitude meteorological analysis necessary to simulate thermosphere-stratosphere coupling? Geophys. Res. Lett. 42, 8225–8230. doi:10.1002/2015GL065838
Smith, A. K., Garcia, R. R., Marsh, D. R., and Richter, J. H. (2011). WACCM simulations of the mean circulation and trace species transport in the winter mesosphere. J. Geophys. Res. 116, D20115. doi:10.1029/2011JD016083
Smith-Johnsen, C., Marsh, D. R., Smith, A. K., Tyssøy, H. N., and Maliniemi, V. (2022). Mesospheric nitric oxide transport in WACCM. JGR. Space Phys. 127, e2021JA029998. doi:10.1029/2021JA029998
Stray, N. H., Orsolini, Y. J., Espy, P. J., Limpasuvan, V., and Hibbins, R. E. (2015). Observations of planetary waves in the mesosphere-lower thermosphere during stratospheric warming events. Atmos. Chem. Phys. 15, 4997–5005. doi:10.5194/acp-15-4997-2015
Tweedy, O. V., Limpasuvan, V., Orsolini, Y. J., Smith, A. K., Garcia, R. R., Kinnison, D., et al. (2013). Nighttime secondary ozone layer during major stratospheric sudden warmings in specified-dynamics WACCM. J. Geophys. Res. Atmos. 118, 8346–8358. doi:10.1002/jgrd.50651
Whiteway, J. A., Duck, T. J., Donovan, D. P., Bird, J. C., Pal, S. R., and Carswell, A. I. (1997). Measurements of gravity wave activity within and around the Arctic stratospheric vortex. Geophys. Res. Lett. 24 (11), 1387–1390. doi:10.1029/97GL01322
Yasyukevich, A. S., Chernigovskaya, M. A., Mylnikova, A. A., Shpynev, B. G., and Khabituev, D. S. (2017). “Seasonal and helio-geomagnetic activity pattern of the ionospheric variability over Russia’s Eastern Siberia and Far East region from the GPS/GLONASS data,” in Proceeding of the2017 Progress In Electromagnetics Research Symposium - Spring, 22-25 May 2017 (St. Petersburg, Russia: IEEE), 2015–2022. doi:10.1109/PIERS.2017.8262081
Zhang, J., Limpasuvan, V., Orsolini, Y. J., Espy, P. J., and Hibbins, R. E. (2021). Climatological westward-propagating semidiurnal tides and their composite response to sudden stratospheric warmings in SuperDARN and SD-WACCM-X. Geophys. Res. Atmos. 126, e2020JD032895. doi:10.1029/2020JD032895
Keywords: polar vortex, gravity wave parameterization, mesospheric winds, atmosphere-ionosphere coupling, energetic electron precipitation (EEP)
Citation: Harvey VL, Randall CE, Bailey SM, Becker E, Chau JL, Cullens CY, Goncharenko LP, Gordley LL, Hindley NP, Lieberman RS, Liu H-L, Megner L, Palo SE, Pedatella NM, Siskind DE, Sassi F, Smith AK, Stober G, Stolle C and Yue J (2022) Improving ionospheric predictability requires accurate simulation of the mesospheric polar vortex. Front. Astron. Space Sci. 9:1041426. doi: 10.3389/fspas.2022.1041426
Received: 10 September 2022; Accepted: 20 October 2022;
Published: 09 November 2022.
Edited by:
Benoit Lavraud, Laboratoire d’astrophysique de Bordeaux (LAB), FranceReviewed by:
Jun Liang, University of Calgary, CanadaCopyright © 2022 Harvey, Randall, Bailey, Becker, Chau, Cullens, Goncharenko, Gordley, Hindley, Lieberman, Liu, Megner, Palo, Pedatella, Siskind, Sassi, Smith, Stober, Stolle and Yue. This is an open-access article distributed under the terms of the Creative Commons Attribution License (CC BY). The use, distribution or reproduction in other forums is permitted, provided the original author(s) and the copyright owner(s) are credited and that the original publication in this journal is cited, in accordance with accepted academic practice. No use, distribution or reproduction is permitted which does not comply with these terms.
*Correspondence: V. Lynn Harvey, bHlubi5oYXJ2ZXlAbGFzcC5jb2xvcmFkby5lZHU=