- Leibniz Institute of Atmospheric Physics, Kühlungsborn, Germany
The scope of this paper is to present some progress being made in the last few decades regarding some aspects of physical processes in the mesosphere/lower thermosphere and to point to some open questions. This summary is presented from a personal perspective, i.e., this is not a review of a certain science topic. Most citations reflect my own work or are representative examples only. They are not meant to be complete or comprehensive.
1 Introduction
The (upper) mesosphere and lower thermosphere (MLT) is a scientifically exciting region since it comprises the transition between the Earth’s atmosphere and space. More specifically, several physical processes change their general characteristics in this region. The mean free path of molecules (and atoms) increases with altitude and approaches macroscopic dimensions in the MLT. This implies that objects entering from space ‘feel’ the Earth’s atmosphere here for the first time. This is relevant for the reentry of satellites (including space shuttle etc.) but also for meteors. This also means that the Knudsen number (Kn = λ/ℓ) is on the order of unity, i.e., the description of flows changes from fluid dynamics to molecular kinetics (λ = mean free path of molecules; ℓ = typical macroscopic dimension, e.g., ℓ = 0.1 m). For insitu-based measurements, e.g., sensors on sounding rockets, this imposes a significant challenge when correcting for ram effects.
We note that parameters describing molecular diffusion processes increase exponentially with altitude because they are roughly proportional to 1/n (n = atmospheric number density). In the MLT, more precisely at the turbopause, the mixing effect by molecular diffusion (Dm) reaches turbulent mixing described by the eddy diffusion cofficient (K): Dm ≈ K. In the MLT a major part of solar EUV and UV (and particles) gets absorbed which contributes to the fact that the ionosphere is located above approximately 60–70 km. Furthermore, geomagnetically induced variations can be significant.
Since atmospheric density is decreasing with height, the time between collisions of molecules decreases. In the MLT this time gets close to the lifetime of excited states due to spontaneous emissions for some relevant molecular excitations, e.g., in carbon dioxide. This implies that radiation and atmospheric matter are no longer in thermodynamic equilibrium for those transitions (non-LTE: non local thermodynamic equilibrium). Therefore, the corresponding radiation cannot readily be taken to derive temperatures which imposes a significant challenge for some satellite retrievals of temperatures.
2 The thermal structure of the MLT
The thermal structure of the MLT is of specific importance since it reflects the energy balance in this atmospheric region and is therefore a good indicator for the physical processes involved. This is particularly relevant for the summer mesopause region at middle and polar latitudes which is the coldest place in the terrestrial atmosphere (T ∼130 K), much colder than in winter (see Figure 1). On the other hand, it is rather difficult to measure temperatures in the MLT region because of aerodynamical and non-LTE effects mentioned above. Furthermore, the heat capacity of the atmosphere is too low to alow for direct classical temperature measurements by thermistors etc. One of very few methods to directly derive high altitude resolution temperature profiles quasi-permanently (depending on weather conditions) is to determine the Doppler broadened line-width of atomic transitions by resonance lidars (Fricke and von Zahn, 1985; von Zahn and Höffner, 1996). Lidars typically measure at one location only. However, recent developments promise to cover a substantial horizontal region [VAHCOLI = Vertical And Horizontal COverage by LIdar (Lübken and Höffner, 2021)].
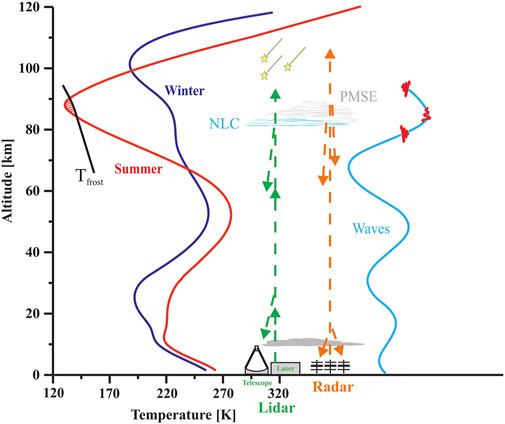
FIGURE 1. Temperature profiles at high latitudes (70°N) for summer (red) and winter (dark blue), respectively. In summer, frost temperatures (black) in the mesopause region are larger than atmospheric temperatures. This allows for the existence of ice particles which are known as noctilucent clouds (NLC), and which are crucial for radar echoes known as polar mesosphere summer echoes (PMSE). The thermal structure in the MLT is mainly caused by a residual circulation driven by the interaction of the mean flow with gravity waves and tides.
In the late 1990s a climatology of temperatures was deduced from density measurements by falling spheres (FS) which is frequently being used as a ‘standard’ for comparison with other observations and with models (Lübken, 1999). The idea to measure densities and deduce temperature profiles assuming hydrostatic equilibrium is also used by other instruments like the rocket-borne CONE ionization gauge or by ground-based Rayleigh lidars (Strelnikov et al., 2013).
Although temperatures in the summer MLT are difficult to measure, they can easily be checked for consistency due to the presence of ice particles known as NLC (noctilucent clouds) or related phenomena (PMSE, polar mesosphere summer echoes, see below): mean temperatures must be below the frost point temperature of water vapor, Tfrost, which is on the order of 150–145 K at 82–88 km, respectively [see Figure 1 and reference (Lübken et al., 1996), the abundance of water vapor plays a minor role for Tfrost; 82 and 88 km are typical heights for the lower and upper edge of NLC/PMSE, respectively]. Typical temperatures from the FS climatology mentioned above are 150 and 135 K at 82 and 88 km, respectively, which are consistent with the presence of ice particles. Note that PMSE are present nearly permanently at polar latitudes, i.e., temperatures must be smaller than Tfrost nearly permanently and not just sporadically.
Energy budget considerations employing observational campaigns and modelling should be contemplated again, taking into account also non-local processes. A significant contribution to the energy budget comes from observations of turbulence on sounding rockets demonstrating that heating of the atmosphere due to the dissipation of turbulent motions caused by wave breaking can be up to 10–20 K/day which is on the same order of magnitude as other energy budget contributions (Lübken, 1997). Remarkably, this heating is largest around the summer mesopause at polar latitudes, i.e., at the coldest place in the Earth’s atmosphere. Heating in the MLT by turbulent dissipation of parameterized or resolved gravity waves is occasionally taken into account in global models, although often incomplete, inconsistent, or tuned by fudge factors [see discussion in (Becker, 2003)].
A long-standing and still unresolved problem in the MLT relates to non-LTE and its relevance for the energy budget in that region. The coefficient for the deactiviation (and activation) of excited carbon dioxide molecules by atomic oxygen is on the order of k(CO2-O) ∼1.5–6 × 10−12 cm3/sec. Unfortunately, values for k(CO2-O) as used in satellite retrieval algorithms are different from values obtained from laboratory measurements (Feofilov and Kutepov, 2012). This coefficient has a major influence on radiative heating/cooling and therefore on background temperatures. Non-LTE is therefore of high relevance for MLT science and deserves to be studied further in the future.
In the summer mesopause region dynamical processes drive the thermal state of the atmosphere away from an otherwise balanced state by up to 100 K (Figure 2). This is the largest impact of dynamics on the thermal state in the Earth’s atmosphere. The forcing comes from wave-induced residual circulation leading to up- and downwelling and associated cooling and heating, respectively. This situation should therefore be used as a benchmark test for models describing this dynamical control, and, more general, of studying the energy balance of the atmosphere.
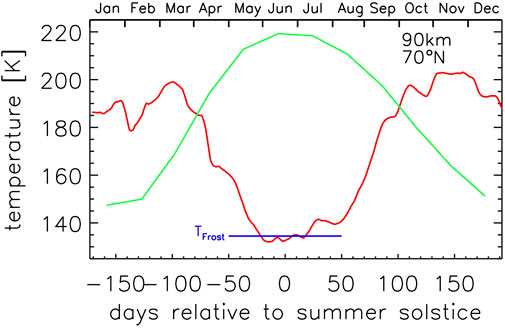
FIGURE 2. Temperatures as function of season at the summer mesopause (90 km), as measured by an Fe-lidar at ALOMAR (red) and from a model (green) where the impact of waves is itentionally eliminated. The blue line indicates the frost point temperature for water ice. The data are provided by Josef Höffner (lidar) and Erich Becker (model).
Winds are also crucial when evaluating the physics of the MLT, including the energy and momentum balance. Measuring background winds in the MLT is realized by radars which since recently cover a substantial horizontal region (Chau et al., 2019). The observational gap of measuring winds on a continuous basis between the (middle) stratosphere and mesosphere is nowadays filled by sophisticated lidars measuring line-of-sight winds (Baumgarten, 2010; Lübken et al., 2016).
3 Layers in the MLT: NLC, PMSE, PMWE
Ice layers in the MLT can be observed by naked eye, by lidars, or from satellites, all of which are here referred to as ‘noctilucent clouds’, NLC (Russell et al., 2009; Fiedler et al., 2017). Polar mesosphere summer echoes (PMSE) are large radar echoes from the summer MLT which require the presence of (charged) ice particles, but also neutral air turbulence. The main physics aspects of PMSE are well understood, i.e., they can now be used as a diagnostic tool to study the atmospheric background, in particular temperatures and turbulence (Rapp and Lübken, 2003).
When using PMSE as an indicator for neutral air turbulence, fossile turbulence can play a role: Fluctuations in the plasma may still exist although neutral air turbulence has already decayed (Rapp and Lübken, 2003). This effect increases with increasing Schmidt number, Sc = D/ν (D = diffusion coefficient for plasma irregularities, ν = kinematic viscosity), i.e., with increasing mass of the (charged) ice (or dust) particles, mice. If mice is small, Sc ∼1 and fossile turbulence plays no role. This is the case in the upper altitude range of PMSE but also for ‘polar mesosphere winter echoes’, PMWE. The latter are weaker compared to PMSE and are observed in the lower and middle mesosphere mainly outside the summer seasons (Lübken et al., 2006; Latteck et al., 2021). They are mainly caused by neutral air turbulence. Although (charged) dust can support the creation of PMWE, their presence is of secondary importance. Note that PMSE and PMWE also occur at middle latitudes like Kühlungsborn (54°N).
PMSE and PMWE should be used to deduce the morphology of neutral air turbulence (occurrence rate, intermittency, etc.) which is important to judge the significance of snapshot observations performed by sounding rockets. Of course, other prerequisites, like ice particles (for PMSE) and sufficient electron number densities (for PMSE and PMWE) must also be fulfilled.
NLC and PMSE also allow to study the microphysics of ice particle formation, growth, and sublimation in a unique environment, including the frostpoint temperature of water vapor over ice at very low temperatures, or the charging of ice and dust particles in a weakly ionized plasma, or the impact of gravity waves and instabilities on the formation of ice (Fritts et al., 2014). NLC are also considered as an indicator for climate change (see Section 5).
4 Waves, turbulence, and transport
4.1 Gravity waves and tides
Gravity wave (GW) and tidal forcing of the background atmosphere is absolutely essential to understand the thermal structure of the MLT. Unfortunately, the physics of gravity wave forcing of the atmosphere is rather complex regarding sources, propagation, generation of higher harmonic waves, dissipation of momentum and energy, all of which in a time-varying background. Mesopause jumps in the southern hemisphere, first observed in 2010 by resonance lidars, are a nice example for a chain of processes of GW forcing of the MLT involving nearly the entire height range from the troposphere to the lower thermosphere (Lübken et al., 2017). They rely on an early breakdown of the winter polar vortex which only takes place in the southern hemisphere. Mesopause jumps should be used as a benchmark test for GW models since they exhibit a distinct and unique signature. Note that in 1998 we performed falling sphere observations of temperatures from Rothera (68°S), the first scientific rocket launchings in Antarctica (Lübken et al., 1999a). The polar stratospheric vortex breakdown in that season occurred rather late, i.e., temperatures in the southern hemisphere MLT were very similar to the northern hemisphere in that season.
Lidars offer the only method to measure GW and tides quasi-continuously (clear sky conditions provided) from the troposphere to the lower thermosphere, both in temperatures (potential energy) and in winds (kinetic energy). Recent developments in lidar technology promise to extend this capability from local to regional scales (Lübken and Höffner, 2021). Note that the morphology of gravity waves can only be treated correctly if the background wind field is known, which is nowadays the case for some lidars (Baumgarten, 2010; Lübken and Höffner, 2021).
Recent lidar observations of GW temperatures and winds have shown that a substantial part of GW propagate downward which confirmed earlier model predictions (Becker and Vadas, 2018; Strelnikova et al., 2020). This mechanism is not taken into account when parameterizing GW in models. Other deficiencies concern non-oblique propagation, unjustifiably assuming an instantaneous impact, ignoring refraction in a time-varying background etc [see, e.g., (Senf and Achatz, 2011)]. Continuous measurements of GW in the stratosphere and lower mesosphere over a period of 10 days showed some unexpected variations on timescales of days to weeks which are presumably caused by the interaction of GW and tides (Baumgarten et al., 2018). In the upper mesosphere such variations of tides are frequently observed by radar (Kero et al., 2019).
Thousends of hours of lidar observations are now available at ALOMAR (69°N) and Kühlungsborn (54°N) which were used to derive a climatology of gravity waves (Strelnikova et al., 2021). The seasonal variation of potential energy (Epot) is more than an order of magnitude smaller compared to a gravity wave resolving model [KMCM, Kühlungsborn Meachnistic Circulation Model (Becker et al., 2022)]. Currently, there is no straight forward explanation of this discrepancy since 1) GW in KMCM are self-generated and cannot easily be ‘tuned’, and b) any change of GW forcing in KMCM will presumably change the background atmosphere, in particular temperatures at the summer mesopause. This discrepancy between observations and modelling provides a promising field for further studies.
A crucial task for the future will be to describe the complex morphology of GW (and tides) in models in a background which varies in time and space and to correctly model the interaction between waves and the mean flow. It is obvious that the creation/propagation of GW in the troposphere and stratosphere are also very important in this context. Up-to-date observations by ground based instruments (lidars, radars, etc.) and by sounding rockets and satellites should be used to constrain these concepts and to provide hints for an adequate description.
4.2 Turbulence
Our early approach to study turbulence around the turbopause at ∼100 km concentrated on the effect of mixing/demixing by turbulence and molecular diffusion, respectively. We evaluated the ratio of Argon to Nitrogen number densities, nAr/nN2, measured by the BUGATTI mass spectrometer (von Zahn et al., 1990). It turned out that other processes may substantially contribute to a constant mixing ratio (i.e., vertical winds), and that it may take a long time (up to weeks) for demixing. This implies that the mixing ratio nAr/nN2 may not result in an adequat description of turbulence. To study the abundance and vertical transport of water vapor we later developed a tunable diode laser spectrometer on sounding rockets called MASERATI, which was, however, rather complicated and too costly for frequent applications (Lübken et al., 1999b).
It should be noted that the transport of tracers from the thermosphere to the stratosphere is not yet fully understood (Smith et al., 2011), but is an important coupling mechanism between the MLT and lower altitudes. This topic requires more attention in the future.
Realizing the limitations outlined above we decided to use relative neutral air density fluctuations Δn/n as a passive tracer for turbulent motions. The magnitude of this effect is on the order of Δn/n ∼ (ωB ⋅ vturb)/g ∼0.1–1% (depending on ωB and vturb; ωB = Brunt-Väisälä frequency; vturb = turbulent velocity ∼1 m/s,; g = Earth’s acceleration). Indeed, we observed spectra of fluctuations consistent with inertial subrange turbulence (ISR). However, deducing turbulent energy dissipation rates, ϵ, is hampered by several uncertain parameters, for example, the dissipation rate of fluctuations (Thrane et al., 1994). A more precise method relies on obtaining the transition between the ISR and the viscous subrange (VSR) in the spectra, which only depends on ϵ, and on the kinematic viscosity, ν, which can easily be derived from atmospheric background parameters. This method imposes a challenge for the experimental method since spectra have to be measured with high temporal resolution (few milli-seconds on a sounding rocket) and high precision (0.1–1%). For this purpose we developed the CONE instrument which was successfully applied in many flights (Lübken, 1992; Lübken et al., 1993). The idea to derive ϵ from the ‘break’ in the spectrum is nowadays frequently applied on sounding rockets and on balloons, and has also been compared to direct numerical simulations (Lehmacher et al., 2006; Theuerkauf et al., 2011; Triplett et al., 2018; Strelnikov et al., 2022).
A climatology for ϵ at 69°N (Andoya Rocket Range) was derived from CONE observations showing large turbulent heating rates of up to ∼20 K/d at the summer mesopause, the coldest place in the Earth’s atmosphere (Lübken, 1997). Obviously, other processes have to compensate for this heating.
Turbulence in the MLT can also be derived from the spectral broadening of radar backscattering which, however, is associated with some uncertainties, e.g., regarding non-turbulent spectral broadening processes (Hocking, 1996; Lübken, 2014).
5 Trends and solar cycle
Negative temperature trends in the strato-, meso-, and thermosphere are meanwhile established in models and are partly confirmed by observations (Roble, 1995; Berger and Lübken, 2011). There is one exception, namely the summer mesopause region at middle and polar latitudes, where temperature trends are positive which is partly due to an enhanced radiative coupling of the cold mesopause to the ‘warm’ stratopause. Unfortunately, this implies that trends are very small in the NLC/PMSE region. On the other hand, ice particles are very sensitive to temperatures and may therefore still be used to study long term trends of temperatures and/or water vapor (Lübken et al., 2021).
Trends in mesospheric ice layers are controversially disputed in the literature (Thomas, 1996; von Zahn, 2003). Model studies by LIMA/MIMAS show that cooling in the stratosphere/lower mesosphere due to an increase of CO2 reduces the (geometric) heights of NLC because of atmospheric shrinking. The decrease of NLC pressure altitudes is much smaller (Berger and Lübken, 2011; Lübken et al., 2021). On the other hand an increase of H2O (due to an increase of CH4 which oxidizes nearly completely to H2O in the mesosphere) leads to an enhancement of NLC brightness and other related parameters, e.g., ice water content (IWC). Note that trends in dynamics have been ignored in these studies. The results from LIMA/MIMAS are consistent with rather limited observations, for example with phase height measurements and with the NLC record at ALOMAR (Bremer and Berger, 2002; Fiedler et al., 2017). Regarding an unambiguous confirmation of trends in NLC or PMSE, the available observations are still not long and comprehensive enough, and they compete with natural variability. A recent extension of LIMA/MIMAS applying future scenarios of greenhouse gas emissions shows that NLC are likely to absorb a significant part of solar radiation, in particular in the UV. This will presumably affect photochemical processes in the stratosphere and MLT.
The upper atmosphere also affects climate on Earth’s surface. Empirical studies indicate a significant modulation of regional temperatures by solar cycle, but the mechanism of the coupling from the MLT (where radiation with largest solar cycle variation is absorbed) to the surface is not yet understood (Seppälä et al., 2009; Gray et al., 2010). Presumably, a complicated chain of processes is involved which includes the transport of photochemically active species, the influence of waves (GW, tides, and planetary waves), and the modification of the background circulation. There is no reasonable doubt that anthropogenic activity is the main reason for global climate change in the troposphere. Still, a climate impact from higher altitudes (and from the Sun) should be considered, at least on regional scales.
Little is known regarding trends in dynamics, including GW (Shepherd, 2014). To repeat: 1) the polar summer mesopause region is the strongest manifestation of GW forcing, and 2) mesopheric ice layers (NLC, PMSE) show rather small long term changes. This imposes a major constraint on any model results or speculations about GW trends. Long term observations of radar winds have shown trends in GW (Hoffmann et al., 2011). It is not clear yet, why this trend has not resulted in an accompanying change of MLT temperatures and NLC/PMSE parameters. This topic requires further attention.
6 Concluding remarks and outlook
The mesosphere/lower thermosphere is a unique and stimulating region for applying various and partly competing physics concepts from rather different fields. More specifically, this region provides an impressive example for an impact of dynamics on the background thermal structure. Some of the involved processes are also relevant for regional climate change.
From my experience I recommend publishing (hopefully) unique observations even without interpretation by modelling, since they can trigger new physical insights. If observations do not agree with models, this should be taken as a motivation to investigate the physical reasons (provided that mistakes in the observations can virtually be excluded) and in the ideal case to modify the models. On the other hand, it is crucial to understand the underlying physics and to consider local/regional processes in the view of global impacts. This can only be acquired by appropriate simulations and modelling. A more recent example of such interaction of observations and modelling comes from our improvements in understanding PMSE/PMWE which were subsequently applied to study phenomena such as interhemispheric coupling and mesopause jumps. I think that science in the MLT can easily stimulate and motivate young scientists, also because they can achieve visibility in the science community more quickly than in many other fields of physics. I’m grateful that such a unique opportunity was provided to me.
Data availability statement
The datasets presented in this study can be found in online repositories. The names of the repository/repositories and accession number(s) can be found at doi: 10.22000/705.
Author contributions
The author confirms being the sole contributor of this work and has approved it for publication.
Funding
This work was partly supported by the Leibniz SAW project FORMOSA (K227/2019) and by the TIMA project of the BMBF research initiative ROMIC (FZ 01LG 1902A). More details of funding sources used in the last decades are provided in the references.
Acknowledgments
I’m grateful to numerous friends and colleagues who inspired and triggered this research over many years. I also thank various funding organizations such as DLR, BMBF, and DFG who not only supported individual projects, but also initiated larger programs such as CAWSES (DFG) and ROMIC (BMBF) which involved several institutes.
Conflict of interest
The author declares that the research was conducted in the absence of any commercial or financial relationships that could be construed as a potential conflict of interest.
Publisher’s note
All claims expressed in this article are solely those of the authors and do not necessarily represent those of their affiliated organizations, or those of the publisher, the editors and the reviewers. Any product that may be evaluated in this article, or claim that may be made by its manufacturer, is not guaranteed or endorsed by the publisher.
References
Baumgarten, G. (2010). Doppler Rayleigh/Mie/Raman lidar for wind and temperature measurements in the middle atmosphere up to 80 km. Atmos. Meas. Tech. 3, 1509–1518. doi:10.5194/amt-3-1509-2010
Baumgarten, K., Gerding, M., Baumgarten, G., and Lübken, F. J. (2018). Temporal variability of tidal and gravity waves during a record long 10 day continuous lidar sounding. Atmos. Chem. Phys. 18, 371–384. doi:10.5194/acp-18-371-2018
Becker, E., Vadas, S. L., Bossert, K., Harvey, V. L., Zülicke, C., and Hoffmann, L. (2022). A high-resolution whole-atmosphere model with resolved gravity waves and specified large-scale dynamics in the troposphere and stratosphere. JGR. Atmos. 127. doi:10.1029/2021JD035018
Becker, E., and Vadas, S. L. (2018). Secondary gravity waves in the winter mesosphere: Results from a high-resolution global circulation model. J. Geophys. Res. Atmos. 123, 2605–2627. doi:10.1002/2017JD027460
Berger, U., and Lübken, F. J. (2011). Mesospheric temperature trends at mid-latitudes in summer. Geophys. Res. Lett. 38, L22804. doi:10.1029/2011GL049528
Bremer, J., and Berger, U. (2002). Mesospheric temperature trends derived from ground-based LF phase-height observations at mid-latitudes: Comparison with model simulations. J. Atmos. Sol. Terr. Phys. 64, 805–816. doi:10.1016/s1364-6826(02)00073-1
Chau, J. L., Urco, J. M., Vierinen, J. P., Volz, R. A., Clahsen, M., Pfeffer, N., et al. (2019). Novel specular meteor radar systems using coherent MIMO techniques to study the mesosphere and lower thermosphere. Atmos. Meas. Tech. 12, 2113–2127. doi:10.5194/amt-12-2113-2019
Feofilov, A. G., and Kutepov, A. A. (2012). Infrared radiation in the mesosphere and lower thermosphere: Energetic effects and remote sensing. Surv. Geophys. 33, 1231–1280. doi:10.1007/s10712-012-9204-0
Fiedler, J., Baumgarten, G., Berger, U., and Lübken, F. J. (2017). Long-term variations of noctilucent clouds at ALOMAR. J. Atmos. Sol. Terr. Phys. 162, 79–89. doi:10.1016/j.jastp.2016.08.006
Fricke, K. H., and von Zahn, U. (1985). Mesopause temperatures derived from probing the hyperfine structure of the D2 resonance line of sodium by lidar. J. Atmos. Terr. Phys. 47, 499–512. doi:10.1016/0021-9169(85)90116-3
Fritts, D. C., Baumgarten, G., Wan, K., Werne, J., and Lund, T. (2014). Quantifying kelvin-helmholtz instability dynamics observed in noctilucent clouds: 2. Modeling and interpretation of observations. J. Geophys. Res. Atmos. 119, 9359–9375. doi:10.1002/2014JD021833
Gray, L. J., Beer, J., Geller, M., Haigh, J. D., Lockwood, M., Matthes, K., et al. (2010). Solar influences on climate. Rev. Geophys. 48, RG4001. doi:10.1029/2009RG000282
Hocking, W. (1996). An assessment of the capabilities and limitations of radars in measurements of upper atmosphere turbulence. Adv. Space Res. 17 (11), 37–47. doi:10.1016/0273-1177(95)00728-w
Hoffmann, P., Rapp, M., Singer, W., and Keuer, D. (2011). Trends of mesospheric gravity waves at northern middle latitudes during summer. J. Geophys. Res. 116, D00P08. doi:10.1029/2011JD015717
Kero, J., Campbell-Brown, M. D., Stober, G., Chau, J. L., Mathews, J. D., and Pellinen-Wannberg, A. (2019). “Radar observations of meteors,” in Meteoroids: Sources of meteors on Earth and beyond. Editors G. O. Ryabova, D. J. Asher, and M. J. Campbell-Brown (Cambridge: Cambridge University Press), Vol. 336, 65–89.
Latteck, R., Renkwitz, T., and Chau, J. L. (2021). Two decades of long-term observations of polar mesospheric echoes at 69°N. J. Atmos. Sol. Terr. Phys. 216, 105576. doi:10.1016/j.jastp.2021.105576
Lehmacher, G. A., Croskey, C. L., Mitchell, J. D., Friedrich, M., Lübken, F. J., Rapp, M., et al. (2006). Intense turbulence observed above a mesospheric temperature inversion at equatorial latitude. Geophys. Res. Lett. 33, L08808. doi:10.1029/2005GL024345
Lübken, F. J., Baumgarten, G., and Berger, U. (2021). Long term trends of mesopheric ice layers: A model study. J. Atmos. Sol. Terr. Phys. 214, 105378. doi:10.1016/j.jastp.2020.105378
Lübken, F. J., Baumgarten, G., Hildebrand, J., and Schmidlin, F. (2016). Simultaneous and co-located wind measurements in the middle atmosphere by lidar and rocket-borne techniques. Atmos. Meas. Tech. 9, 3911–3919. doi:10.5194/amt-9-3911-2016
Lübken, F. J., Dingler, F., von Lucke, H., Anders, J., Riedel, W. J., and WolfMaserati, H. (1999). MASERATI: A rocketborne tunable diode laser absorption spectrometer. Appl. Opt. 38, 5338–5349. doi:10.1364/ao.38.005338
Lübken, F. J., Fricke, K. H., and Langer, M. (1996). Noctilucent clouds and the thermal structure near the Arctic mesopause in summer. J. Geophys. Res. 101, 9489–9508. doi:10.1029/96jd00444
Lübken, F. J., Hillert, W., Lehmacher, G., and von Zahn, U. (1993). Experiments revealing small impact of turbulence on the energy budget of the mesosphere and lower thermosphere. J. Geophys. Res. 98, 20, 369–20, 384. doi:10.1029/93jd02055
Lübken, F. J., and Höffner, J. (2021). VAHCOLI, a new concept for lidars: Technical setup, science applications, and first measurements. Atmos. Meas. Tech. 14, 3815–3836. doi:10.5194/amt-14-3815-2021
Lübken, F. J., Jarvis, M. J., and Jones, G. O. L. (1999). First in situ temperature measurements at the Antarctic summer mesopause. Geophys. Res. Lett. 26, 3581–3584. doi:10.1029/1999gl010719
Lübken, F. J., Latteck, R., Becker, E., Höffner, J., and Murphy, D. (2017). Using polar mesosphere summer echoes and stratospheric/mesospheric winds to explain summer mesopause jumps in Antarctica. J. Atmos. Sol. Terr. Phys. 162, 106–115. doi:10.1016/j.jastp.2016.06.008
Lübken, F. J. (1992). On the extraction of turbulent parameters from atmospheric density fluctuations. J. Geophys. Res. 97, 20, 385–20, 395. doi:10.1029/92jd01916
Lübken, F. J. (1997). Seasonal variation of turbulent energy dissipation rates at high latitudes as determined by insitu measurements of neutral density fluctuations. J. Geophys. Res. 102, 13, 441–13, 456. doi:10.1029/97jd00853
Lübken, F. J., Strelnikov, B., Rapp, M., Singer, W., Latteck, R., Brattli, A., et al. (2006). The thermal and dynamical state of the atmosphere during polar mesosphere winter echoes. Atmos. Chem. Phys. 6, 13–24. doi:10.5194/acp-6-13-2006
Lübken, F. J. (1999). Thermal structure of the Arctic summer mesosphere. J. Geophys. Res. 104, 9135–9149.
Lübken, F. J. (2014). Turbulent scattering for radars: A summary. J. Atmos. Sol. Terr. Phys. 107, 1–7. doi:10.1016/j.jastp.2013.10.015
Rapp, M., and Lübken, F. J. (2003). On the nature of PMSE: Electron diffusion in the vicinity of charged particles revisited. J. Geophys. Res. 108, 8437. doi:10.1029/2002JD002857
Roble, R. G. (1995). Major grenhouse cooling (yes, cooing): The upper atmosphere response to increased CO2. Rev. Geophys. 33, 539–546. doi:10.1029/95rg00118
Russell, J. M., Bailey, S. M., Gordley, L. L., Rusch, D. W., Horányi, M., Hervig, M. E., et al. (2009). The aeronomy of ice in the mesosphere(AIM)mission: Overview and early science results. J. Atmos. Sol. Terr. Phys. 71, 289–299. doi:10.1016/j.jastp.2008.08.011
Senf, F., and Achatz, U. (2011). On the impact of middle-atmosphere thermal tides on the propagation and dissipation of gravity waves. J. Geophys. Res. 116, D24110. doi:10.1029/2011JD015794
Seppälä, A., Randall, C. E., Clilverd, M. A., Rozanov, E., and Rodger, C. J. (2009). Geomagnetic activity and polar surface air temperature variability. J. Geophys. Res. 114. doi:10.1029/2008JA014029
Shepherd, T. G. (2014). Atmospheric circulation as a source of uncertainty in climate change projections. Nat. Geosci. 7, 703–708. doi:10.1038/ngeo2253
Smith, A. K., Garcia, R. R., Marsh, D. R., and Richter, J. H. (2011). WACCM simulations of the mean circulation and trace species transport in the winter mesosphere. J. Geophys. Res. 116, D20115. doi:10.1029/2011JD016083
Strelnikov, B., Rapp, M., Fritts, D. C., and Wang, L. (2022). Assessment of the precision of spectral model turbulence analysis techniques using direct numerical simulation data. J. Geophys. Res. 127, e2021JD035516. doi:10.1002/2021JD035516
Strelnikov, B., Rapp, M., and Lübken, F. J. (2013). “In-situ density measurements in the mesosphere/lower thermosphere region with the TOTAL and CONE instruments,” in An introduction to space instrumentation. Editor K. Oyama (Moscow, Russia: Terra Publishers), 1–11.
Strelnikova, I., Almowafy, M., Baumgarten, G., Baumgarten, K., Ern, M., Gerding, M., et al. (2021). Seasonal cycle of gravity wave potential energy densities from lidar and satellite observations at 54° and 69°N. J. Atmos. Sci. 78, 1359–1386. doi:10.1175/JAS-D-20-0247.1
Strelnikova, I., Baumgarten, G., and Lübken, F. J. (2020). Advanced hodograph-based analysis technique to derive gravity-wave parameters from lidar observations. Atmos. Meas. Tech. 13, 479–499. doi:10.5194/amt-13-479-2020
Theuerkauf, A., Gerding, M., and Lübken, F. J. (2011). LITOS – A new balloon-borne instrument for fine-scale turbulence soundings in the stratosphere. Atmos. Meas. Tech. 4, 55–66. doi:10.5194/amt-4-55-2011
Thomas, G. (1996). Is the polar mesosphere the miner’s canary of global change? Adv. Space Res. 18 (3), 149–158. doi:10.1016/0273-1177(95)00855-9
Thrane, E., Blix, T., Hoppe, U. P., Lübken, F. J., Hillert, W., Lehmacher, G., et al. (1994). A study of small-scale waves and turbulence in the mesosphere using simultaneous in situ observations of neutral and plasma fluctuations. J. Atmos. Terr. Phys. 56, 1797–1808. doi:10.1016/0021-9169(94)90011-6
Triplett, C. C., Li, J., Collins, R. L., Lehmacher, G. A., Barjatya, A., Fritts, D. C., et al. (2018). Observations of reduced turbulence and wave activity in the arctic middle atmosphere following the January 2015 sudden stratospheric warming. J. Geophys. Res. Atmos. 123, 13259–13276. doi:10.1029/2018JD028788
von Zahn, U., and Höffner, J. (1996). Mesopause temperature profiling by potassium lidar. Geophys. Res. Lett. 23, 141–144. doi:10.1029/95gl03688
von Zahn, U., Lübken, F. J., and Pütz, C. (1990). ’BUGATTI’ experiments: Mass spectrometric studies of lower thermosphere eddy mixing and turbulence. J. Geophys. Res. 95, 7443–7465. doi:10.1029/jd095id06p07443
Keywords: mesosphere, lower thermosphere, summer mesopause, noctilucent clouds, turbulence, gravity waves, mesopause jumps, climate change
Citation: Lübken F-J (2022) Physics in the mesosphere/lower thermosphere: A personal perspective. Front. Astron. Space Sci. 9:1000766. doi: 10.3389/fspas.2022.1000766
Received: 22 July 2022; Accepted: 24 August 2022;
Published: 30 September 2022.
Edited by:
Yoshizumi Miyoshi, Nagoya University, JapanReviewed by:
Nickolay Ivchenko, Royal Institute of Technology, SwedenHitoshi Fujiwara, Seikei University, Japan
Copyright © 2022 Lübken. This is an open-access article distributed under the terms of the Creative Commons Attribution License (CC BY). The use, distribution or reproduction in other forums is permitted, provided the original author(s) and the copyright owner(s) are credited and that the original publication in this journal is cited, in accordance with accepted academic practice. No use, distribution or reproduction is permitted which does not comply with these terms.
*Correspondence: Franz-Josef Lübken, bHVlYmtlbkBpYXAta2Jvcm4uZGU=