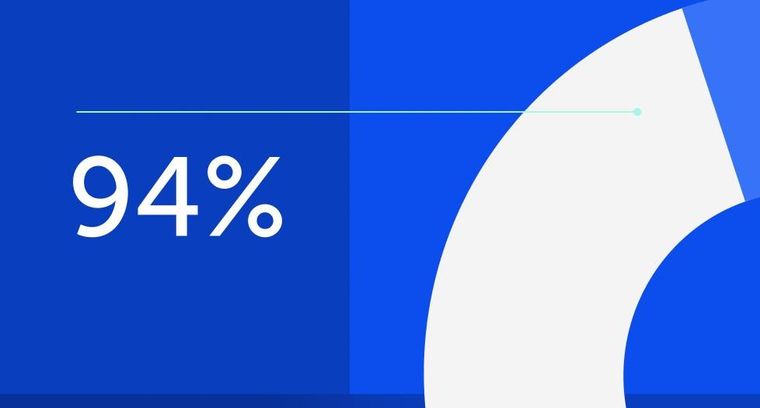
94% of researchers rate our articles as excellent or good
Learn more about the work of our research integrity team to safeguard the quality of each article we publish.
Find out more
REVIEW article
Front. Astron. Space Sci., 12 January 2022
Sec. Astrobiology
Volume 8 - 2021 | https://doi.org/10.3389/fspas.2021.810426
This article is part of the Research TopicMars analogs: Environment, Habitability and BiodiversityView all 11 articles
The Atacama Desert is by far the driest and oldest desert on Earth, showing a unique combination of environmental extremes (extreme dryness, the highest UV radiation levels on Earth, and highly saline and oxidizing soils), explaining why the Atacama has been largely investigated as a Mars analog model for almost 20 years. Based on the source and the amount of water available for life and its analogy with Mars, two ecosystems are of interest in the Atacama: its Coastal Range and the much drier hyperarid core, which we here review in detail. Members of the three domains of life have been found across these ecosystems living at the limit of habitability, suggesting the potential dry limits for each domain and also unveiling the highly patchy distribution of microbial life in its most extreme regions. The thorough study of the Atacama has allowed us to understand how life has adapted to its extreme conditions, the specific habitats that life occupies in each case (thus suggesting the most likely places in which to search for evidence for life on Mars), and the number of biosignatures detected across this desert. Also, the characterization of west-to-east transects across this desert has shown to be of significant value to understand the potential adaptations that Martian microorganisms may have followed in an ever-drying planet. All of this explains why the Atacama is actively used as the testing ground of the technologies (detection instruments, rovers, etc.) that were sent and will be sent to Mars. We also highlight the need to better inform the exact locations of the sites studied to understand general trends, the need to identify the true native microbial species of the Atacama, and the impact of climate change on the most arid and most Martian desert of Earth.
The Atacama Desert is located in northern Chile, framed by the Andes Mountains on the east and by the Pacific Ocean on the west (Figures 1A,B). The Atacama is well known as the driest place on Earth (Houston and Hartley, 2003; Hartley et al., 2005; Rech et al., 2006; Sun et al., 2018). The extreme aridity of the Atacama is explained by three main factors (Figure 2); its location within the southern hemisphere dry subtropical climate belt, the pronounced rain shadow effect of the Andean Mountains that prevent the incoming of humid masses of air coming from the Amazon Basin, and the presence of the upwelling, north-flowing, cold Humboldt Current, which significantly reduces sea water evaporation and cloud formation (which when they form, are stopped by the Coastal Range) (Somoza and Tomlinson, 2002; Houston and Hartley, 2003; Hartley et al., 2005; Azua-Bustos et al., 2015). In addition, since the South American plate has maintained its latitudinal position relatively in the same place for the past 150 million years, the Atacama is also the oldest desert on Earth, due to its zonal position relative to the Hadley circulation (Hartley et al., 2005). As a consequence, rains in some regions of the Atacama are as infrequent as once every 20 years (Lettau and Lettau 1978; McKay et al., 2003), with places with mean annual precipitations of only 0.15 mm (Houston 2006).
FIGURE 1. Location of the Atacama Desert. (A) Location of the Atacama in South America. (B) Zoom of the white frame shown in A. Note the masses of clouds at the eastern side of the Andes over Argentina and Bolivia. (C) General location of the Coastal Range (light blue), the hyperarid core (Yellow), and the Andes Mountains and its foothills (green).
Since the times of Darwin the Atacama was considered a “sterile wasteland” (Darwin, 1845; James, 1927); however, this view dramatically changed in 2003, when Yungay, a site located in the hyperarid core of the Atacama, was suggested to be a good analog model of Mars (Navarro-González et al., 2013) (details on this report commented later in the Hyperarid Core section). In addition, the Atacama is known for having the highest UV radiation on Earth, with annual UVB doses ranging from 3.5 to 5 kWh/m2 on the Coastal Range of the Atacama and UVA doses ranging from 130 to 160 kWh/m2 on the Andes Mountains (Cordero et al., 2018).
This assemblage of extreme environmental conditions explains why since 2003, many reports have described the meteorological, geophysical, and microbiological characteristics of the Atacama in order to study it as a Mars analog model (Azua-Bustos et al., 2012). To aid the organization of the findings covered in this review, we summarize them into two main ecosystems from the point of view of the analogy with Mars (Figure 1C); the Coastal Range and the hyperarid core, a selection also based on the source and critically, the amount of water available for life in each region.
The Coastal Range is a 3,000-km-long mountain that runs from north to south along the Pacific coast at the west margin of Chile. With a maximum altitude of 3,100 m in front of the Atacama, it is much lower than the Andes Mountains (Morata et al., 2008). With a mean width of approximately 50 km, it still causes a very effective rain shadow effect, impeding the entrance of the few clouds that form due to the low temperatures of the Pacific Ocean. Even so, the main source of water for life in the Coastal Range is these low clouds that regularly cover and moist this area typically during the night and the early hours of the morning (Figure 3) (Cereceda et al., 2008; Azua-Bustos et al., 2010; Azua-Bustos et al., 2011).
FIGURE 3. Low-altitude clouds that originate in the Pacific Ocean (locally known as camanchaca) as they encounter the Coastal Range of the Atacama.
It is at the eastern face of the Coastal Range that the studies with a focus of the Atacama as a Mars analog model were first undertaken, when in advance of the Viking missions, a team from the Jet Propulsion Laboratory inspected soil samples from deserts around the world, reporting in this case a number of microorganisms (Streptomyces, Bacillus, and Micrococcus) in soils samples of Uribe, a now abandoned train station (Cameron and Gensel, 1966; Bollen, 1967).
More than forty years later, Zöe (an autonomous rover) was also tested in the Coastal Range (Weinstein et al., 2008), using a daylight fluorescence imager to detect surface biosignatures near the Salar Grande (Figure 4), a small salt pan flanking the eastern side of the Coastal Range. Using this imager, autofluorescent biomolecules such as chlorophyll were readily detected, along other molecules such as DNA, proteins, carbohydrates, and lipids, when previously sprayed with specific fluorescent probes.
FIGURE 4. Salar Grande, located at the eastern margin of the Coastal Range near the coastal city of Iquique.
This salt pan was further investigated as a Mars analog model by drilling, in which subsurface samples were analyzed with the LDChip300 (Parro et al., 2011), a chip containing an array of antibodies part of the SOLID Instrument (Signs of Life Detector), planned as one of the payloads of the proposed IceBreaker Life mission to Mars (Davé et al., 2013). Using this instrument (Figure 5), these authors detected the biosignatures of bacteria and archaea associated with halite, nitrate, and perchlorate salts at a depth of 2 m. Phylogenetic analyses of 16 S rRNA gene sequences suggested the presence of species such as Vibrio and Pseudomonas. This same group of authors later analyzed samples from a pit 100 m of depth in this salt pan as an analog of Martian halite deposits (Sánchez-García et al., 2018). Using a number of techniques, including the LDChip, biosignatures from Haloarchaea and Cyanobacteria were found in near surface halite-rich samples, while deeper samples, composed by albite and quartz, contained biosignatures from methanogenic archaea and sulfur-oxidizing bacteria. Altogether, the analyses undertaken at Salar Grande suggested that similar subsurface environments could shelter Martian microorganisms, based on the deliquescence of hygroscopic salts such as halite, perchlorates, nitrates, and sulfates, with some of these which could also be used as potential electron acceptors by Martian microorganisms.
FIGURE 5. SOLID, the Signs of Life Detector, developed a the Centro de Astrobiology (Center of Astrobiology) in Spain. Photo credit: Victor Robles.
Other interesting biosignatures have also been found in the Coastal Range; a miniaturized Raman system was tested with samples of the Coastal Range, along other samples collected further inland (Vitek et al., 2014). Gypsum crusts from a site south of the Salar Grande and from the Salar de Llamara saline ponds (both located at the eastern margin of the Coastal Range) were analyzed, reporting the evaporites (sulfates, carbonates, and halites) characteristic of this region. These authors found that only carotenoids were detected as biosignatures in these samples, while carotenoids and chlorophyll were detected in the hyperarid core samples.
Caves of the Coastal Range have also been studied as analogs of Martian caves, of which several have been detected up to date (Cushing et al., 2007; Cushing, 2017; Titus et al., 2021). In order to test whether phototrophic microorganisms (or its remnants) could be found inside caves on Mars, well protected from the outside radiation environment, but still close enough to nearby entrances in order to perform photosynthesis, one of such caves (Figure 6A) was inspected north of the city of Antofagasta (Azua-Bustos et al., 2009). In support of this hypothesis, a biofilm composed by the red microalgae Cyanidium was found at the bottom of this cave (Figure 6B), surviving with only 0.06% (1 μE) of the outside photosynthetic active radiation. Transmission electron microscopy micrographs showed that cells of these microalgae had a single chloroplast using more than 50% of the cell interior, unveiling a unique adaptation to the extremely low-light conditions of the cave. Another cave, about 250 km north (Figure 6C), was also inspected by this team of researchers, where the only known subaerial member of the Dunaliella genus of microalgae was reported growing on top of the spider webs that cover the cave wall entrance (Figure 6D) (Azua-Bustos et al., 2010). Such adaptation suggested that this microalgae is able to use the water condensing on the hydrophilic spider webs for photosynthesis.
FIGURE 6. Caves of astrobiological interest in the Coastal Range of the Atacama. (A) La Portada cave near the coastal city of Antofagasta. (B) Cyanidium sp. Atacama epilithic biofilms covering the rocks at the bottom of La Portada Cave. (C) Huanillos cave entrance (center of image shown). (D) A spider web colonized with Dunaliella atacamensis cells at Huanillos cave entrance walls.
The unique adaptations to alternative sources of water and low-light conditions inside these caves suggest that microbial life on Mars may have used similar strategies and similar hygroscopic materials in order to photosynthesize in a protected cave environment (Figure 7), where different types of chlorophyll-like molecules may be used for this end (Behrendt et al., 2020), in one of the last refuge potentially used by life in an ever-drying planet.
FIGURE 7. Hypothetic location of phototrophic microorganisms in Martian caves. Modified from image of Melissa Ausburn.
Hypolithic habitats have also been discovered and studied in the Coastal Range. Azua-Bustos et al. (2011) reported a community containing members of the three domains of life inhabiting the underside of translucent quartz stones (Figure 8) southeast of the city of Antofagasta. These authors reported that these hypolithic communities depended on fog as a source of water, and that the interaction of fog and the higher thermal conductivity of the quartz rocks (which keep them cooler than the surrounding soil environment during the day time hours), allowed for water condensation and colonization under them. Later, this team of researchers better described the diversity of microbial species under these quartzes (Azua-Bustos et al., 2014) reporting the finding of at least 70 new species from the three domains of life, among others, cyanobacteria (Cyanothece, Gloeocapsopsis, and Nostoc) microalgae (Oocystis and Chlorella), archaea (Haloferax and Halococcus), and a number of heterotrophic bacteria (Bacillus, Deinococcus, Geobacillus, Halomonas, and Streptococcus). From this hypolithic community, these authors isolated the cyanobacterium Gloeocapsopsis dulcis (Figure 9) (Azua-Bustos et al., 2014; Jung et al., 2021), a species phylogenetically close to Chroococcidiopsis which was used to study the molecular mechanisms explaining the tolerance of such species to extremely low water activity. These authors found that G. dulcis chlorophyll a conserved its structural integrity and autofluorescence at a water activity (aw) as low as 0.4 for several months and that cells desiccated at aw 0.4 synthesized increasing amounts of sucrose and trehalose, both sugars known for their role as compatible solutes involved in extreme desiccation tolerance (Azua-Bustos et al., 2014).
FIGURE 8. Hypolithic biofilms under quartz stones of the Coastal Range south of the city of Antofagasta.
FIGURE 9. Gloeocapsopsis dulcis, a cyanobacterium isolated from quartz hypolithic biofilms. Photo credit: Patrick Jung.
G. dulcis cells were also used in a study aimed to detect biosignatures in distant exoplanets using polarimetry (Sterzik et al., 2010). Considering that homochirality, common in amino acids and sugars used by life on Earth, is known to induce circular polarization in the diffuse reflectance spectra, these authors used the EFOSC2 (the European Southern Observatory Faint Object Spectrograph and Camera) to analyze samples of G. dulcis cells, in which chiral signatures were indeed detected coming from chlorophyll a. The studies on the hypolithic communities unveiled the role of such ecosystems as islands of biodiversity in an extremely dry environment (as originally proposed by Warren-Rhodes et al., 2006) of critical importance when searching for evidences of life on Mars.
Even plants of the Coastal Range of the Atacama have been of use for how to detect evidences of life on Mars (Azua-Bustos and Vega-Martínez 2013). These authors argued that any lifeform in the Universe should decrease their internal entropy at the expense of free energy obtained from its surroundings. Thus, among other study models used, by using satellite images of plant growth patterns on the Coastal Range (Figure 10) and software based on fractal mathematics, these authors found that plants could be readily differentiated from morphologically similar surrounding abiotic patterns, which along with the analysis of sedimentary patterns on both Earth and Mars, showed that similar entropy differences could be used to detect environments altered by life on Mars.
FIGURE 10. Plant (Tillandsia sp.) banding growth patterns on top of Coastal Range hills. The inset shows how these plants look from space.
The Coastal Range has also been searched for UV-tolerant microorganisms, with a number of Bacillus and Pseudomonas species isolated from its soils (Paulino-Lima et al., 2013). One of these Bacillus isolates showed a tolerance somewhat lower than that of Deinococcus radiodurans, suggesting that high UV tolerance was common in the Atacama. UV radiation reaches the surface of Mars unimpeded, and the finding of species extremely tolerant to UV radiation on deserts such as the Atacama suggests that microbial life in Mars may have developed similar adaptations in time.
With the analysis of samples of this region, the Atacama was first proposed as a pertinent model for the extremely dry conditions of Mars (Dose et al., 2001), which explains why this region of the Atacama was by far the most studied since then. These authors reported the environmental parameters (relative humidity and temperature) of two sites, Chacabuco and Yungay (Figure 11), in order to assess the survivability of a number of desiccation-tolerant microorganisms under the extremely dry conditions of the hyperarid core. By using the cells and spores of two bacteria and three fungi (Bacillus subtilis spores, Deinococcus radiodurans, Aspergillus niger, A. ochraceus, and A. versicolor), these authors found that the cells of D. radiodurans barely tolerated the direct daily solar radiation and extreme dryness of Yungay, although B. subtilis spores and A. niger conidia survived for more than a year in shaded areas, when exposed to direct sunlight, they survived only for a few hours.
FIGURE 11. Yungay, located in the hyperarid core of the Atacama. This site, reported by NASA in 2003, although not the driest, is one of the best studied on the Atacama as analog of Mars.
A similar experiment was performed a few years later by Cockell et al. (2008), which tested whether UV radiation could prevent epilithic colonization in Yungay. With maximal UV radiation fluxes of 0.58 W/m2 taking place at 13:00 h, two “biological dosimeters” were tested: Chroococcidiopsis cells from the Negev Desert and Bacillus subtilis spores. These authors found that the viability of Chroococcidiopsis cells was relatively stable until noon, with a sharp reduction in the afternoon. In the case of B. subitilis, its spores were completely killed within 1 day of exposure, with maximum inactivation rates taking place between 14:00 and 15:00 h. When the cells of these species were protected by 1 mm of either gypsum or quartz in order to simulate the hypolithic and cryptoendolithic environment, there was no measurable loss of viability, suggesting that the hyperarid core represented a critical threshold where extreme desiccation and UV radiation completely inhibited epilithic colonization. These results suggested that although the UV radiation fluxes are much higher on Mars, micro-habitats within minerals may similarly attenuate UV fluxes and allow for photosynthesis to take place. However, when Yungay soil Bacteria were tested in a Mars simulation chamber (Thomas et al., 2007), including heterotrophic isolates such as Klebsiella oxytoca, and Bacillus licheniformis, these authors reported that these bacteria survived for 2 weeks in the Mars simulation chamber, with survival rates between 35% (Bacillus licheniformis) and 100% (Bacillus sp.).
The most important study that fully established the Atacama as an analog model of Mars was reported 2 years later, also using samples of Yungay (Navarro-González et al., 2013). By using surface samples collected along a north to south precipitation gradient and pyrolysis–gas chromatography–mass spectrometry (pyr-GC-MS), these authors reported that Yungay samples were almost depleted of organics, with almost undetectable amounts of formic acid and benzene, which ratio suggested that Yungay organics were highly oxidized. When these analyses were repeated at the highest temperatures (500°C) used by the Viking landers, the amount of formic acid was greatly diminished, while benzene was undetectable. This suggested that metastable organics such as the salts of aromatic and aliphatic mono and polycarboxylic acids may have been indeed present in the Martian regolith, but would not have been detected by the Viking instruments. These authors also performed a modified version of the Viking labeled release experiment (Levin and Straat, 1979) by incubating Yungay samples in an 13C-labeled sodium formate aqueous solution, observing that a fraction of the added formate was decomposed even when no cultivable microorganisms were detected, suggesting the presence of strong oxidant soil species. Yungay samples had extremely low levels of heterotrophic bacteria (1 × 103), and in many cases, no bacterial colonies were observed at any dilutions of the growing media used. In addition, no DNA was recoverable from the samples analyzed. Altogether, the collection of results allowed us to propose Yungay to be a good analog model of Martian soils, thus providing an invaluable testing ground for instruments and experiments designed for future Mars missions.
This team of researchers later continued their analysis of samples of the hyperarid core to better understand the results obtained by the Viking mission (Navarro-González et al., 2010). As the Phoenix Lander found perchlorates in the Martian regolith, these researchers reported that when Yungay samples containing small amounts (32 ppm) of organic carbon were mixed with 1% of magnesium perchlorate and then heated, most of the organics present were decomposed to water and carbon dioxide, with a small amount getting chlorinated. This result suggested that the Viking landing sites may have contained small amounts of perchlorate (0.1%) and as much as 6.5 ppm of organic carbon.
The potential effects that perchlorates would have in the detection of organics on Mars were examined using soil samples from the hyperarid core (Yungay and Lomas Bayas, among others) (Montgomery et al., 2019). These authors reported that perchlorates were highly soluble and easily leached with water. Thus, when organics were searched for (by pyrolysis–GC-MS) in unleached samples, no organics were detected, but when dried samples after leaching were analyzed, organics were easily detected. This approach unveiled that leaching prevented the complete destruction of organic compounds mediated by highly oxidant species (such as perchlorates) in detection methods where pyrolysis is used, of particular importance for the case of Mars, where perchlorates are present almost three orders of magnitude higher than those of hyperarid core soil samples. These authors suggested that on Mars, ancient surface or near-surface water flows may have concentrated organic compounds downslope, leaching away highly oxidant species at the same time, thus making the detection of organics easier in such regions.
In order to better understand the pH changes that took place with Viking Martian surface samples were wetted during the Labeled release experiment, Yungay samples among other samples from the hyperarid core were studied on the magnitude and kinetics of pH shifts upon wetting (Quinn R. et al., 2005). After wetting, both the Martian and Yungay surface samples pH underwent a rapid shift from acidic to slightly basic, shift that was not observed in the samples collected from the wetter regions of the Atacama or in the Yungay subsurface samples. The response of Yungay surface samples was consistent with a dry deposition and accumulation of atmospheric acid aerosols and acid precursors on the soil surface. These findings suggested that the sites of the hyperarid core such as Yungay are so dry that acids accumulate on its surface, and that the addition of water (mostly coming from fog) causes its dissolution and neutralization. To test such a hypothesis, this group of authors then characterized the chemical reactivity of surface and near-surface atmosphere samples by using the Mars oxidation instrument (MOI, an array of sensors that measures the reaction rates of chemical films sensitive to oxidants or that mimics chemical characteristics of prebiotic and biotic materials) which allowed the in situ characterization of oxidation mechanisms and kinetics (Quinn R. C. et al., 2005).
On the one hand, MOI confirmed that RH at Yungay typically reached below 10% in the afternoon hours and that each day shortly after sunset, the fog that typically covers this area caused a rapid increase up to 90% of RH in some days. In parallel, MOI recorded a chemical response immediately following the rapid increase in RH, suggesting that during the day, dry acids were created photochemically in the atmosphere from SO2 and NOx precursors, which then adsorbed onto dust and regolith surfaces, with oxidative acid reactions taking place at night during periods of high RH. Altogether, these responses suggested that these types of acids play an important role in the oxidizing nature and the modification of organics of Yungay surface soils, unveiling the hyperarid core photochemical environment as a pertinent model to understand the photochemical origin of Martian oxidants.
An additional approach to understand the results of the Viking labeled release experiment was focused on the analysis of the decomposition of organic compounds in aqueous solutions of Yungay soil samples by this same group of authors (Quinn et al., 2007). In the LR experiment, the addition of a 14C-labeled aqueous organic substrate to a sealed test cell that contained a Martian regolith sample resulted in the evolution of 14CO2. Thus, these authors added 13C-labeled formate to Yungay surface soil samples, using similar volumetric ratios used by Vikings, along a peroxide-modified titanium dioxide to simulate a nonbiological LR response. The initial pattern of CO2 released caused by decomposition of formate was found to match those of Martian surface samples. When the D and L enantiomers of glucose and alanine were used, both decomposed at approximately equal linear rates during the first days, suggesting a nonbiological decomposition. However, an increase in 13CO2 production in the D-glucose and L-Alanine samples compared to the L-glucose and D-Alanine samples was observed after 5 days. This lagged response followed by an increase in CO2 production was deemed to be an indicator of biological activity. Heating the soil samples significantly attenuated the release of CO2, suggesting the death of the microorganisms present in these samples. Although a number of alternative explanations that do not involve biology have been suggested for the Viking LR results, Quinn et al.’s (2007) results were consistent with the decomposition of formate by OH radicals as the primary mechanism for the decomposition of organics. Thus, considering the results first reported by this group of authors on the magnitude and kinetics of pH shifts of these samples upon wetting (Quinn R. et al., 2005), it seems that the three criteria established for a positive detection of life in an LR experiment were indeed met: the decomposition of organics until the added organic substrate is completely used, additional CO2 release after additional nutrient is added, and an attenuated response of the heated control, again showing the value of analog studies with samples of the hyperarid core.
As commented before, Yungay soils have extremely low organic carbon contents, but oddly, PLFA (phospholipid fatty acids) surface concentrations were found to be five times higher than that of subsurface samples by Lester et al. (2007) (although Wilhelm et al. (2017) reported an increased presence in depth of a number of fatty acids with a high degree of structural conservancy in Yungay soils). In both cases PLFA composition was dominated by monoenoic PLFA, suggesting the presence of Gram-negative Proteobacteria. In turn, monoenoic PLFA were lower in subsurface samples, suggesting a Firmicutes community, while Mid-branched saturated PLFAs represented a small percentage of the total content, suggesting the presence of Actinobacteria. R2A and TSA growth media plate counts revealed up to 4.6 × 103 CFU per gram of soil. In order to reveal endospore-forming bacteria, a heat shock was applied to these samples, resulting in up to 300 CFU per gram of soil (for comparison, other desert soils range significantly higher, starting at 104 CFU/g, Kieft, 2002). 16S rRNA gene sequencing analysis of the heat-treated samples unveiled bacteria such as Bradyrhizobium, Rhodopseudomonas palustris, and Bacillus pumilus. Thus, given the high variations in the cultivable biomass at Yungay, these authors first unveiled the patchy distribution of microbial life in soils of the hyperarid core, suggesting that a similar, and significant spatial and possibly temporal heterogeneities in microbial distribution may also be the case on Mars.
A number of biosignatures have also been found in the soils of the hyperarid core (Wilhelm et al., 2018). In a continuation of their report of 2017, these authors reported on the diversity and abundance of lipids and other biomolecules in surface soil samples, using among other techniques, the LDChip. By inspecting a number of sites, including Yungay and Maria Elena South (details on the discovery of this site later), these authors reported that the abundance and diversity of free and membrane-bound fatty acids decreased with increasing aridity. Similarly, the LDChip also unveiled a decrease in total fluorescence by a factor of 24 in the driest sites compared to wetter sites further south, again suggesting that the surface soils of the hyperarid core were a good analog model for developing new instruments to search for evidences of life in the Martian surface.
Yungay samples were also studied using a method able to estimate bacterial cell counts based on the sublimation of adenine from E. coli (Glavin et al., 2004), with only traces of nucleobases identified in subsurface soils samples and no adenine detected in surface samples. DAPI staining unveiled bacterial counts of 0.7 × 106 cells/g in surface samples and 9.6 × 106 cells/g in subsurface samples, values coherent with sublimation cell count estimates. DAPI counts were higher than total counts of viable cultivable heterotrophic bacteria measured by Navarro-González et al. (2013), suggesting that the Yungay soil samples contained mostly noncultivable bacteria or again, a patchy distribution of microorganisms due to abiotic causes such as perchlorates. These authors also suggested that the Yungay soil samples were a valuable analog for life-detecting instruments on Mars, as bacterial cells should be detectable in the Martian regolith with the reported sublimation technique. These results later helped this group of authors to develop the first steps conducive to SAM, the Sample Analysis at Mars instrument now onboard the Curiosity rover (Glavin et al., 2006). By coupling their previously reported sublimation/chemical derivatization technique (Glavin et al., 2004) to GCMS (gas chromatography mass spectrometry), hyperarid core samples were analyzed in order to detect nucleobases and other volatile organic compounds derived from soil bacteria. Yungay soil surface samples showed adenine concentrations of 0.04 nmol/g, value at least two orders of magnitude higher than total viable counts previously reported in Yungay samples by Navarro-González et al. (2013), again suggesting that these samples contained mostly noncultivable bacteria.
Similarly, MOA, the Mars Organic Analyzer, a microfabricated capillary electrophoresis instrument for the detection of amino acids as biosignatures originally developed for the ExoMars mission, was also tested with Yungay samples (Skelley et al., 2005). These authors found low levels (up to 70 ppb) of alanine, aspartic acid, glycine, glutamic acid, and serine on northern and mid-latitude samples of the Atacama, while southern samples showed several amino acids, including alanine, aspartic acid, glycine, glutamic acid, serine, and valine, with values up to 500 ppb. Continuing their studies, this same group of authors then tested two instruments using Yungay samples (Skelley et al., 2007). The first was Urey, an in situ organics analyzer containing a subcritical water extractor. The second was MOA, already tested in 2005. Samples were collected from the top soil surface centimeter, shielded from the Sun either by rocks or from the Sun-exposed surface. These authors found that shielded samples contained low monoamine and neutral amino acid signals (i.e., valine, alanine/serine, glycine), while the exposed samples showed no signals. To improve the sensitivity limits, homogenized samples were extracted using a portable subcritical water extractor (Amashukeli et al., 2007), which also showed that shielded samples contained higher levels of amino acids than exposed samples. These results showed that the exposure context significantly influenced the amount of biosignatures, an important finding to consider for the search for life on Mars. In addition, a chiral analysis used to examine the potential biotic/abiotic source of the amino acids showed similar D/L ratios of alanine/serine in shielded samples compared to gypsum-rich surface samples, unveiling the presence of viable microorganisms. The findings in gypsum-rich samples also unveiled that surface moisture is an important factor for biosignature synthesis and preservation in soils of the hyperarid core. The site containing the gypsum-rich samples is located in an ancient and now dry water flow channel, similar to those observed on Mars (Malin and Edgett, 2003). As gypsum is an evaporite of watery origin, it is interesting that samples containing it showed the presence of amines and amino acids, as it validated the capability of Urey to find potential biosignatures on similar samples on Mars.
Among other interesting discoveries in Yungay, microbial communities were then found living inside surface halites (Wierzchos et al., 2006), a finding that then gave rise to one of the best studied models on how life is still able to persist under the extreme conditions of the hyperarid core. Halite rocks in this site are composed of almost pure sodium chloride, with minor amounts of gypsum and traces of potassium chloride and quartz. Following the initial observation that many of these halites had a thin gray/greenish layer a few mm below the rock surface (Figure 12), samples were collected and further inspected. Light microscopy examination of this layer revealed cells similar to the cyanobacterium Chroococcidiopsis, with low temperature scanning electron microscopy (LTSEM) unveiling abundant cells in the spaces between salt crystals along the condensation of water in the pores among halite crystals where these cells were located. In a follow-up, these authors reported the microclimate inside and around colonized halites, to test whether the hygroscopicity of halite was involved in the survivability of its endolithic microorganisms (Davila et al., 2008). These authors found that depending on the season, air temperatures close to the soil were similar to those inside the halites, reaching up to around 50°C during the day, and as low −3°C during the night. However, during the day, RH inside the halites remained relatively high and constant for one to 3 days, while the outside RH showed the normal daily variations. These events took place when the RH inside the halites reached higher than 75% (value that matched the deliquescence RH of this mineral) and interpreted as water condensing within its internal porous spaces. This was caused by humid masses of air coming from the Pacific Ocean which with some periodicity are able to reach Yungay resulting in dew and fog, thus providing a source of water for the endolithic communities living inside halites. These findings are relevant for the case of Mars, as large regions such as Meridiani Planum or Gale Crater once hosted evaporitic environments that resulted in the precipitation of salt-rich deposits (Nachon et al., 1991; Vaniman et al., 2018). Thus, if life ever arose on Mars, biosignatures left behind by similar microorganisms may still be found inside halites. Later it was confirmed that halites were also able to condense and retain liquid water inside them due to the presence of a nano-porous phase with a smooth surface that covered large crystals and filled the larger pore spaces inside these halites (Wierzchos et al., 2012). This nano-porous phase contained pores smaller than 100 nm, allowing for capillary water condensation at RH lower than the deliquescence RH of halites, which in turn allowed for this water to be used by the endolithic microorganisms.
FIGURE 12. Colonized halites in Yungay. Note the gray/greenish layer a few mm below the artificially fractured halite surface.
Continuing the characterization of hyperarid core halites, de los Ríos et al. (2010) compared the endolithic communities inside halites from three different sites (Yungay, Salar Grande, and Salar de Llamara), finding by Denaturing Gradient Gel Electrophoresis analysis (DGGE), that all these communities were composed by a single cyanobacterial species (Chroococcidiopsis), along a few diverging heterotrophic bacteria and archaea. An additional study of Yungay halites using Raman spectroscopy (along halites of Salar Grande and an additional unmanned site) reported the presence of chlorophyll and UV-protective biomolecules such as scytonemin, b-carotene, zeaxanthine, and lutein, all indicators of the photosynthetic activity being undertaken by these cyanobacteria (Vitek et al., 2010), deemed to be potential biosignatures to be searched for on Mars. This study was later complemented by the use of two miniaturized Raman spectrometers that searched for such biosignatures inside Yungay halites (Vítek et al., 2012). By directly analyzing halites or homogenized powdered samples (in order to simulate the sampling of Mars rovers), these authors found that carotenoids were readily detected in both samples. This suggested that carotenoids are an interesting biosignature to be searched for, as it was detectable down to 100 ppb.
These findings made possible to model what may happen with the water activity of the deliquescent solutions of chloride salts as a function of temperature in the regions of Mars containing chloride-bearing evaporites (Davila et al., 2010). According to the model proposed, RH in these regions often reached the deliquescence points of different chloride salts, and temperatures reached levels above their eutectic points in the course of a Martian year. Considering the limits of water activity which define the so-called Mars special regions, these authors found that the deliquescence of Martian calcium chloride deposits would allow the colonization of terrestrial microorganisms at temperatures between −8 and −20°C, and metabolic activity (but not growth) at temperatures between −20 and −40°C. Altogether, the findings on halites added to the concept that hygroscopic salts may have been one of the last refuges for life on Mars (Fairén et al., 2010).
The key processes involved in the formation of the soils of the hyperarid core have also been studied (Ewing et al., 2006). These authors showed that soil formation may be explained by a shift in the balance of pedogenic processes as a function of extreme aridity. By taking soil samples across the hyperarid core, including Yungay, these authors found that along decreasing rainfall and biotic activity, soil formation crossed a threshold from net long-term mass loss (due to biogeochemical alteration of the geologic substrate), to increasing soil volume with retention of atmospheric inputs. These authors suggested that the hyperarid core pedogenic processes could be of use to understand the accumulation of atmospheric dust and solutes in the ancient Martian landscapes, as the Martian regolith contains sulfates and chlorides comparable to those of hyperarid core.
These authors also studied the still enigmatic origin of the extensive nitrate deposits only present in the hyperarid core of the Atacama have also been investigated (Ewing et al., 2007). By analyzing soil samples up to 2 m deep in a decreasing rainfall gradient from Yungay to Copiapó, these authors reported that while organic carbon decreased to 0.3 kg Cm−2 with decreasing rainfall, biological activity became insignificant, and nitrates and organic nitrogen increased to 4 kg Nm−2 and 1.4 kg Nm−2. As rainfall decreased, isotopic analyses showed that atmospheric NO3 increased from 39 to 80% of total soil NO3. The type of soil nitrogen also changed, from 88% of organic origin at the less arid sites to 28% at the driest sites of the hyperarid core. These results suggested that the origin of the atmospheric nitrates in hyperarid core could be caused by the photochemical mobilization of upwelling marine NO2/NO3, as a marine origin was also consistent with observations of soil sulfates in sites of the coasts in front of the studied sites (Rech et al., 2003). This observation added an additional factor of analogy with Mars, as this report unveiled that extreme aridity stalls the nitrogen cycle by limiting the biotic component, resulting in the accumulation of oxidized N. Thus, nitrates (Stern et al., 2015) and the relatively low N2 content of the Martian atmosphere may be reflecting the storage of nitrates in its regolith similar to the Atacama case.
The response of the direct addition of water to Yungay soils has also been studied (Davis et al., 2010). These authors performed a series of simulated rain experiments in the early evening hours south of Yungay, observing that the addition of the equivalent of less than 1 mm of rain did not saturate the surface soil. Davis and colleagues also found that the crust that covered the soil surface acted as a strong barrier to diffusion of subsurface moisture and subsequent evaporation. In turn, simulated rain events of two or more mm generated free water in the pore space of the soil surface, which could be used by microbial life, with larger amounts of water resulting in moisture retention at depth that during subsequent nights, which then diffused upward as water vapor, mobilizing salts and rewetting the dry surface. These results were deemed of value for modeling how water vapor in the upper portion of the surface of Mars could get moisture from the environment.
As oxalates in the Martian surface might account for the CO2 release from Martian regolith observed by Viking missions (Benner et al., 2000; Applin et al., 2015), it was proposed that its detection in soils of the hyperarid core may be taken as a mineral marker that could help to understand the sources of organic carbon on Mars (Cheng et al., 2016). Thus, by reporting the presence of two oxalates at the Salar Grande, weddellite and whewellite, these authors found that weddellite was associated with surface biota, and that its presence in subsurface detrital rocks also suggested a potential biological origin, supporting the idea that life is uniquely capable of concentrating oxalates at detectable levels.
A related report focused on the hyperarid core as a model to understand the evolution of the Martian atmosphere, as the anomalous 17O enrichment found in the nitrate ore fields only known in this region was proposed to be evidence of the atmospheric deposition of particles accumulated in a period of 200,000–2.0 Myears (Michalski et al., 2004). These authors suggested that 17O values were a preserved isotopic signature of atmospheric processes that took place over long periods of time, and that 17O measurements could be used to identify products of nitrification by potential biologic processes in Martian samples.
Similar to the case of the Coastal Range, hypolithic cyanobacteria have also been reported in Yungay (Warren-Rhodes et al., 2006). These authors reported a decrease in cyanobacterial colonization between much wetter sites such as Copiapó to nearly zero at Yungay, with a decreasing loss of diversity along the aridity gradient. Among the cyanobacteria found in Copiapó were species of Chroococcidiopsis, Nostoc, and Phormidium (along heterotrophic bacteria such as Acidobacteria, Bradyrhizobium, and Caulobacter), while below quartz stones at Yungay, only two species were found; Chroococcidiopsis and an unidentified γ-proteobacterium. Radiocarbon analysis of these cyanobacterial communities unveiled that microbial activity declined abruptly along the aridity gradient, with steady-state residence time values of about a year at the wettest end of the transect, and 3,000 years at Yungay. These authors also found that total organics were five times less in the surface soils than in the soils under the quartz stones where the hypolithic communities were located, first suggesting that quartz stones acted as islands of fertility and biodiversity in the extreme environment of the Atacama. Radiocarbon values showed an important difference between carbon cycling rates of the hypolithic soils and the surrounding surface soils; at wetter sites mean turnover times of hypolithic soils were the same as those of surface soils. In contrast, Yungay surface soils showed a turnover time of 13,000 years, four times greater than that of the hypolithic soils (3,200 years), suggesting that as colonization rates exponentially decline in the hyperarid core, the photosynthetic communities drove a carbon cycle in an extremely confined scale. These results suggested that at Yungay, the biological threshold for photosynthetic life was mainly defined by water availability, contributing to the question of where to specifically search for photosynthetic life on Mars.
The metabolic state of the microbial species found in the hyperarid core has also been inspected (Schulze-Makuch et al., 2018). By revisiting a number of sites, including those reported by Azua-Bustos et al. (2015) (Maria Elena South and Lomas Bayas), as well as Yungay, these authors reported that surface soils were dominated by species already reported such as Geodermatophilus and Rubrobacter, while subsurface soils contained species such as Betaproteobacteria, Firmicutes (Bacillaceae, Alicyclobacillaceae), and halophilic archaea (Halobacterium). These authors confirmed what was previously known that although microbial biomass and microbial diversity in the Atacama Desert decrease with increasing aridity, extremely low inputs of water in this regions can sustain microbial activity, again, of interest for the case of Mars.
Although Yungay was considered the paradigm of the extreme dryness of the Atacama, the original exploration and characterization of this site was not the result of a systematic search for the driest sites of the hyperarid core, but chosen for logistical reasons (Christopher McKay, per. comm). Thus, by setting atmospheric temperature/relative humidity loggers during a 4-year interval, three sites were later reported as being much drier than Yungay; Moctezuma, Cerritos Bayos, and María Elena South (MES) with MES (Figure 13) being the driest of all (Azua-Bustos et al., 2015). These authors reported that while mean atmospheric RH at MES was 17.3%, at the same time period, RH at Yungay was 28.8%, with previous works reporting even higher mean atmospheric RH (36.9%) at Yungay (de los Ríos et al., 2010). Although minimum atmospheric RH was extremely low at both MES and Yungay (0 and 1%, respectively), maximum atmospheric RH was much lower at MES (54.7%) that that at Yungay (86.8%). These authors then set RH/temperature loggers in the soil profile up to a depth of 1 m at MES, finding that at 80 cm down RH was only 14.2% (equivalent to a water activity of 0.142), with little variations in time (between 12.7 and 17%). In this way, these authors reported the driest site of Atacama and of Earth, as in comparison, other hyperarid sites such as the Dry Valleys of Antarctica showed mean atmospheric RH values in the range of 55–74% (Doran et al., 2002). Extraordinarily, soil RH in depth at MES matched the lowest RH values recorded by the Remote Environmental Monitoring Station (REMS) recorded at the same time by NASA’s Curiosity rover (Haberle et al. 2014), making MES one of the best analog models of Mars in terms of the extreme aridity. MES soil samples had extremely low levels of organics (up to 1.1%) and although these authors were aiming to find the dry limit of life on Earth, even soils of this site contained a number of different microorganisms, such as Aciditerrimonas, Geodermatophilus, Caulobacter, and Sphingomonas, as detected by DGGE. Culturing also allowed the isolation of a number of bacteria (Bacillus, Streptomyces, and Geodermatophilus). Interestingly, no archaea or any type of phototrophs were found with the culture dependent or independent methods used, suggesting that although the dry limit for life on Earth has not yet been found, at least MES was too dry for archaea and photosynthetic microorganisms such as cyanobacteria and microalgae.
FIGURE 13. María Elena South. Located in the middle of the hyperarid core and the driest site of the Atacama.
Zöe also investigated the spatial distribution of microbial life in subsurface soils of the hyperarid core (including Yungay) (Warren-Rhodes et al., 2019). By analyzing a number of samples up to a depth of 80 cm, these authors found that surface sediments and subsurface horizons showed the known patchy distribution of life at the hyperarid core, with extremely low yields of DNA (0.067–6.5 ng/g), unveiling the extremely low habitability of soils of the hyperarid core, with some sites where again no DNA could be recovered at any depth, findings again in line with the first reports on the hyperarid core (Navarro-Gonzalez et al., 2013). Bacterial diversity was found to decrease in depth, with mid-depth soils showing the highest bacterial diversity dominated by Chloroflexi, Actinobacteria, and Alphaproteobacteria, a finding adding to previous reports where the Firmicutes was shown as one of the most prevalent phyla on hyperarid core samples, most likely explained by the type of sites inspected (desert pavement and desert playa only) and the amount of subsurface salts present in the sites inspected that decreased water activity.
UV-tolerant microorganisms have also been isolated from soils of the hyperarid core (Paulino Lima et al., 2016). These authors reported a number of species encompassing 28 genera of bacteria (Bacillus, Hymenobacter, Arthrobacter, and Hydrogenophaga, among others) from UVC-irradiated samples, with one of these isolates, Hymenobacter sp. AT01-02, found to be more tolerant to UVC than Radiococcus radiodurans. Intracellular Mn/Fe ratios in these isolates were found to be correlated with the level of tolerance; however, these authors found no correlation between the presence of the isolates and the contents of manganese in the soil in which they were found, suggesting that these species were able to uptake and accumulate manganese even in soils with low manganese concentrations.
The small lagoons of the Salar de Llamara (Figure 14) have also been investigated (Rasuk et al., 2014). This salt pan is unique as it contains a number of highly saline shallow lagoons with partially submerged fungus-shaped structures composed of calcium sulfate (Azua-Bustos et al., 2009), highly reminiscent of stromatolites. Rasuk et al. (2014) analyzed the microbial communities associated with these structures by 16S rDNA gene sequencing, finding a number of bacteria (Bacteroidetes, Proteobacteria, and Planctomycetes) as the main phylogenetic groups, a diversity that increased in winter time. Although the presence of bacteria was found in these stromatolite-like structures, it is still unclear whether these are made by these microorganisms or are simply used as support, nevertheless still important as a model for similar structures that may be found on Mars (Rhawn et al., 2020).
The highly unexpected effects on the massive rain event that affected the hyperarid core in 2017 were also reported (Azua-Bustos et al., 2018). It must be noted that rains are common at the western edge of the Atacama (at the foothills of the Andes Mountains), caused by the masses of moist air coming from the Amazon basin that sometimes are able to go over the Andes during the southern hemisphere summer, thus the cause of a completely different ecosystem in this region. However, in 2015 (and also 2017 and 2019), extensive mass of rain clouds entered the hyperarid core from the Pacific Ocean, meteorological events never recorded in this region. These resulted in a number of lagoons in sites such as Yungay in 2017, which again were never recorded before in the middle of the hyperarid core of the Atacama (Figure 15). Strikingly, these authors found only four types of bacteria inhabiting these lagoons (Halomonas, Marinimicrobia, Marinobacter, and Acinetobacter), which compared with the microorganisms reported in the soils of Yungay where these lagoons formed, unveiled that the rains caused a reduction of up to 87% in the soil microbial diversity. These authors suggested that the microbial species that inhabit the hyperarid core are extremely adapted to survive with almost no water, thus when confronted with the sudden input of abundant water, they quickly perished from osmotic burst. Thus, as the Martian transition to hyperaridity is suggested to have been punctuated by episodes of vast aqueous discharges (Baker, 2004), these authors suggested that similar decimated local microbial ecosystems may have not adapted to a fast changing environment, resulting in a patchy distribution of life across the Martian surface.
The potential sources of origin of the species that have been found in the hyperarid core have been inspected too (Azua-Bustos et al., 2019). By setting plates with a number of growth media in west-to-east transects across the Coastal Range and the hyperarid core, these authors showed that wind-driven particles were a highly efficient mode of transport for microbial life across the Atacama, a process that took place in a matter of hours. As winds in this region first come over the Pacific Ocean from the south and then go east inland into the Atacama, these authors found the potential origin of the species that colonize the driest regions of the Atacama on the beaches and hills of the Coastal Range. These authors also found that, as the speed of these winds are higher in the late afternoon and nighttime hours, the transport of viable microorganisms was favored by the relatively higher RH and the lower or complete absence of UV radiation during the night. Altogether, these findings suggested that due to global wind storms on Mars, potential Martian microorganisms (both past or extant) may similarly use wind to colonize the entire planet, an observation of particular importance for planetary protection and the advent of future human colonies.
Finally, other caves have also been investigated at the eastern edge of the hyperarid core (Wynne et al., 2008), a region much wetter due to its proximity to the foothills of the Andes. Here, infrared thermal imaging was confirmed as a tool to detect caves entrances on Mars (Cushing et al., 2007). As temperatures inside a cave differ from the environment where they are located, these researchers, by studying two caves in the Cordillera de la Sal (a highly saline mountainous range at the foothills of the Andes), found that indeed cave entrances could be detected due to the thermal differences with their surroundings, particularly during midday hours.
Due to its extreme aridity, high UV radiation, and highly saline soils, the Atacama Desert is well known in the scientific community as a good analog model of Mars (Table 1). Its environmental and geological characteristics have been well studied, which along the description and diversity of microorganisms and the habitats colonized by life have helped to better suggest where to look for evidences of life on Mars. Epilithic, hypolithic, endolithic, chasmoendolithic, and subsurface habitats have been reported all across the Atacama, suggesting that extreme aridity, nor high UV radiation or highly saline/oxidizing soil species, would completely hinder the presence of life on Mars. Water is available for life even in the most extreme habitats of the Atacama through different mechanisms that are still being uncovered, such as thermal differences, capillarity, hygroscopicity, or deliquescence, which explain the existence of microhabitats as true “islands of biodiversity” in an extremely harsh environment, and thus, the most interesting analog targets for the search of evidences of life on Mars. Microbial members of the three domains of life have been reported across the Atacama, with only heterotrophic bacteria reported in its driest regions, thus suggesting a potential “dry limit” for photosynthetic bacteria, eukaryotes, and archaea in such sites. For these reasons, the Atacama has turned into a leading location to test the technologies (detection instruments, rovers, etc.) to be sent to Mars (Table 2), and from the point of view of extreme desiccation and extremely high UV radiation, the site “Maria Elena South,” located in the middle of the hyperarid core of the Atacama, is, up to now, one of the closest analogs of Mars on Earth. Also, contradictory reports on the presence and diversity of microbial life in Atacama soils have unveiled the uneven, extremely “patchy” distribution in regions in which life is at the limit of detectability, as well as the advantages and drawbacks of a number of techniques used for detecting microorganisms that are at extremely low population numbers.
In general terms, west-to-east transects from the relatively wetter Coastal Range inland into the hyperarid core of the Atacama may in fact be considered one of the best analogs to retrace and study the potential adaptations to different habitats for life on Mars from a wetter to a dryer planet. Thus, Martian microorganisms, if ever existed, may have evolved from being able to thrive on its surface (as they do in some sites of the Coastal Range), then step-wise colonized more protected habitats as Mars got progressively drier and colder in order to survive, with epilithic habitats in the Noachian, hypolithic in the Noachian/Hesperian transition, endolithic during the Hesperian, and then into the subsurface during the Amazonian, as is the case of the hyperarid core of the Atacama.
There are a number of key research areas and subjects to be pursued in the coming years. Currently, there are but a few reports focused on the molecular mechanisms that explain how such microorganisms are able to tolerate the extreme conditions of the Atacama, their levels of metabolic activity in different extreme environmental conditions (including Mars simulation chambers), and the potential origin and age of these species. Although wind-transported dust particles have been reported as an efficient transport mechanism to disperse microbial life across the Atacama, we still do not understand how these species are able to adapt to its new environment, and thus understand which of these can be considered distinctly “native.” Also, there is a need to search for more evidences of xerophilic species unique to the Atacama, analogous to polar or saline environments elsewhere. The tools provided by the “omics” will undoubtedly help to solve these interesting questions. Similarly, few reports have studied the transition between the eastern edge of the hyperarid core of the Atacama and the much wetter foothills of the Andes Mountains. It is also important to understand the general impact of the unusual precipitations that have recently affected the Atacama, which, thought to be caused by global warming, have randomly affected different regions of this desert with yet unpredictable long-term consequences on the geology and diversity of the xerotolerant microbial species of the Atacama.
Finally, we recommend that future reports should all inform the precise location of their study sites as most give incomplete coordinates and/or the exact depths from where samples were taken, critical for understanding general trends. Care must also be taken to properly inform the general geographical frame of the study sites as there are a number of reports that comment on the well-known characteristics of the hyperarid core but with the actual study sites located in the Andes Mountains or its foothills, quite different ecosystems, and not precisely analogs of Mars. These considerations are particularly important given the highly patchy distribution of life in the Atacama, which, in addition, takes place at the limit of habitability in the most Martian place on Earth.
AA-B wrote the manuscript. All authors contributed to the manuscript revision, read, and approved the submitted version.
AAB was supported by the project UVEnergy RGY0066/2018 funded by the Human Frontiers Science Program. AAB and AGF were supported by the Project “MarsFirstWater”, funded by the European Research Council, ERC Consolidator Grant No. 818602 to AGF.
The authors declare that the research was conducted in the absence of any commercial or financial relationships that could be construed as a potential conflict of interest.
All claims expressed in this article are solely those of the authors and do not necessarily represent those of their affiliated organizations, or those of the publisher, the editors and the reviewers. Any product that may be evaluated in this article, or claim that may be made by its manufacturer, is not guaranteed or endorsed by the publisher.
Amashukeli, X., Pelletier, C. C., Kirby, J. P., and Grunthaner, F. J. (2007). Subcritical Water Extraction of Amino Acids from Atacama Desert Soils. J. Geophys. Res. Biogeosci 11, 1–10. doi:10.1029/2006jg000308
Applin, D. M., Izawa, M. R. M., Cloutis, E. A., Goltz, D., and Johnson, J. R. (2015). Oxalate Minerals on Mars? Earth Planet. Sci. Lett. 420, 127–139. doi:10.1016/j.epsl.2015.03.034
Azua-Bustos, A., González-Silva, C., Fernández-MartínezArenas-Fajardo, M. Á. C., Arenas-Fajardo, C., Fonseca, R., Martín-Torres, F. J., et al. (2019). Aeolian Transport of Viable Microbial Life across the Atacama Desert, Chile: Implications for Mars. Sci. Rep. 9, 11024. doi:10.1038/s41598-019-47394-z
Azua-Bustos, A., Caro-Lara, L., and Caro-Lara, L. (2015). Discovery and Microbial Content of the Driest Site of the Hyperarid Atacama Desert, Chile. Environ. Microbiol. Rep. 7, 388–394. doi:10.1111/1758-2229.12261
Azua-Bustos, A., Fairén, A. G., González-Silva, C., Ascaso, C., Carrizo, D., Fernández-Martínez, M. Á., et al. (2018). Unprecedented rains Decimate Surface Microbial Communities in the Hyperarid Core of the Atacama Desert. Sci. Rep. 8, 16706. doi:10.1038/s41598-018-35051-w
Azúa-Bustos, A., González-Silva, C., Mancilla, R. A., Salas, L., Gómez-Silva, B., McKay, C. P., et al. (2011). Hypolithic Cyanobacteria Supported Mainly by Fog in the Coastal Range of the Atacama Desert. Microb. Ecol. 61, 568–581. doi:10.1007/s00248-010-9784-5
Azúa-Bustos, A., González-Silva, C., Mancilla, R. A., Salas, L., Palma, R. E., Wynne, J. J., et al. (2009). Ancient Photosynthetic Eukaryote Biofilms in an Atacama Desert Coastal Cave. Microb. Ecol. 58, 485–496. doi:10.1007/s00248-009-9500-5
Azúa-Bustos, A., González-Silva, C., Salas, L., Palma, R. E., and Vicuña, R. (2010). A Novel Subaerial Dunaliella Species Growing on Cave Spiderwebs in the Atacama Desert. Extremophiles 14, 443–452. doi:10.1007/s00792-010-0322-7
Azua-Bustos, A. (2009). Living Stromatolites in the Midst of the Atacama Desert: A Paradigm for the Origin of Life. Astrobiology 9, 518–519.
Azua-Bustos, A., Urrejola, C., and Vicuña, R. (2012). Life at the Dry Edge: Microorganisms of the Atacama Desert. FEBS Lett. 586, 2939–2945. doi:10.1016/j.febslet.2012.07.025
Azua-Bustos, A., and Vega-Martínez, C. (2013). The Potential for Detecting 'life as We Don't Know it' by Fractal Complexity Analysis. Int. J. Astrobiology 12, 314–320. doi:10.1017/s1473550413000177
Azua-Bustos, A., Zúñiga, J., Arenas-Fajardo, C., Orellana, M., Salas, L., and Rafael, V. (2014). Gloeocapsopsis AAB1, an Extremely Desiccation-Tolerant Cyanobacterium Isolated from the Atacama Desert. Extremophiles 18, 61–74. doi:10.1007/s00792-013-0592-y
Baker, V. R. (2004). “A Brief Geological History of Water on Mars,” in Origins. Cellular Origin, Life in Extreme Habitats and Astrobiology. Editor J. Seckbach (Dordrecht: Springer), Vol. 6.
Behrendt, L., Trampe, E. L., Nord, N. B., Nguyen, J., Kühl, M., Lonco, D., et al. (2020). Life in the Dark: Far‐red Absorbing Cyanobacteria Extend Photic Zones Deep into Terrestrial Caves. Environ. Microbiol. 22, 952–963. doi:10.1111/1462-2920.14774
Benner, S. A., Devine, K. G., Matveeva, L. N., and Powell, D. H. (2000). The Missing Organic Molecules on MarsMolecules on Mars. Proc. Natl. Acad. Sci. 97, 2425–2430. doi:10.1073/pnas.040539497
Bollen, W. B. (1967). “Identification of Chile Atacama Desert Soil Isolants,” in Progress Report Microorganisms Study. JPL Contract No 9500783.
Cameron, R. E., and Gensel, D. R. (1966). Soil Studies—Desert Microflora. XII. Abundance of Microflora in Soil Samples from the Chile Atacama Desert. JPL Space Programs Summ. No IV, 37–38.
Cereceda, P., Larrain, H., Osses, P., Farías, M., and Egaña, I. (2008). The Spatial and Temporal Variability of Fog and its Relation to Fog Oases in the Atacama Desert, Chile. Atmos. Res. 87, 3–4. doi:10.1016/j.atmosres.2007.11.012
Cheng, Z. Y., Fernández-Remolar, D. C., Izawa, M. R. M., Applin, D. M., Chong Díaz, M., Fernandez-Sampedro, M. T., et al. (2016). Oxalate Formation under the Hyperarid Conditions of the Atacama Desert as a mineral Marker to Provide Clues to the Source of Organic Carbon on Mars. J. Geophys. Res. Biogeosci. 121, 1593–1604. doi:10.1002/2016jg003439
Cockell, C. S., McKay, C. P., Warren-Rhodes, K., and Horneck, G. (2008). Ultraviolet Radiation-Induced Limitation to Epilithic Microbial Growth in Arid Deserts - Dosimetric Experiments in the Hyperarid Core of the Atacama Desert. J. Photochem. Photobiol. B: Biol. 90, 79–87. doi:10.1016/j.jphotobiol.2007.11.009
Cordero, R. R., Damiani, A., Jorquera, J., Sepúlveda, E., Caballero, M., Fernandez, S., et al. (2018). Ultraviolet Radiation in the Atacama Desert. Antonie Van Leeuwenhoek 111, 1301–1313. doi:10.1007/s10482-018-1075-z
Cushing, G. E. (2017). Mars Global Cave Candidate Catalog Archive Bundle. Reston, Va: U.S. Geological Survey. Available at: astrogeology.usgs.gov/search/map/Mars/MarsCaveCatalog/mars_cave_catalog.zip.
Cushing, G. E., Titus, T. N., Wynne, J. J., and Christensen, P. R. (2007). THEMIS Observes Possible Cave Skylights on Mars. Geophys. Res. Lett. 34, L17201. doi:10.1029/2007gl030709
Darwin, C. (1845). The Voyage of the Beagle. Excerpts from Charles Darwin, Journal of Researches into the Natural History and Geology of the Countries Visited during the Voyage of HMS Beagle Round the World: Under the Command of Capt. Patagonia: Fitz Roy.
Davé, A., Thompson, S. J., McKay, C. P., Stoker, C. R., Zacny, K., Paulsen, G., et al. (2013). The Sample Handling System for the Mars Icebreaker Life Mission: From Dirt to Data. Astrobiology 13, 354–369. doi:10.1089/ast.2012.0911
Davila, A. F., Duport, L. G., Melchiorri, R., Jänchen, J., Valea, S., de los Rios, A., et al. (2010). Hygroscopic Salts and the Potential for Life on Mars. Astrobiology 10, 617–628. doi:10.1089/ast.2009.0421
Davila, A. F., Gómez-Silva, B., de los Rios, A., Ascaso, C., Olivares, H., McKay, C. P., et al. (2008). Facilitation of Endolithic Microbial Survival in the Hyperarid Core of the Atacama Desert by mineral Deliquescence. J. Geophys. Res. 113, 01028. doi:10.1029/2007jg000561
Davis, W. L., de Pater, I., and McKay, C. P. (2010). Rain Infiltration and Crust Formation in the Extreme Arid Zone of the Atacama Desert, Chile. Planet. Space Sci. 58, 616–622. doi:10.1016/j.pss.2009.08.011
de Los Ríos, A., Valea, S., Ascaso, C., Davila, A., Kastovsky, J., McKay, C. P., et al. (2010). Comparative Analysis of the Microbial Communities Inhabiting Halite Evaporites of the Atacama Desert. Int. Microbiol. 13, 79–89. doi:10.2436/20.1501.01.113
Doran, P. T., McKay, C. P., Clow, G. D., Dana, G. L., Fountain, A. G., Nylen, T., et al. (2002). Valley Floor Climate Observations from the McMurdo Dry Valleys, Antarctica, 1986–2000. J. Geophys. Res. 107, 1–12. doi:10.1029/2001jd002045
Dose, K., Bieger-Dose, A., Ernst, B., Feister, U., Gómez-Silva, B., Klein, A., et al. (2001). Survival of Microorganisms under the Extreme Conditions of the Atacama Desert. Orig. Life Evol. Biosph. 31, 287–303. doi:10.1023/a:1010788829265
Ewing, S. A., Michalski, G., Thiemens, M., Quinn, R. C., Macalady, J. L., Kohl, S., et al. (2007). Rainfall Limit of the N Cycle on Earth. Glob. Biogeochem. Cycles 21, GB3009. doi:10.1029/2006gb002838
Ewing, S. A., Sutter, B., Owen, J., Nishiizumi, K., Sharp, W., Cliff, S. S., et al. (2006). A Threshold in Soil Formation at Earth's Arid-Hyperarid Transition. Geochimica et Cosmochimica Acta 70, 5293–5322. doi:10.1016/j.gca.2006.08.020
Fairén, A. G., Davila, A. F., Lim, D., Bramall, N., Bonaccorsi, R., Zavaleta, J., et al. (2010). Astrobiology through the Ages of Mars: the Study of Terrestrial Analogues to Understand the Habitability of Mars. Astrobiology 10, 821–843. doi:10.1089/ast.2009.0440
Glavin, D. P., Cleaves, H. J., Buch, A., Schubert, M., Aubrey, A., Bada, J. L., et al. (2006). Sublimation Extraction Coupled with Gas Chromatography-Mass Spectrometry: A New Technique for Future In Situ Analyses of Purines and Pyrimidines on Mars. Planet. Space Sci. 54, 1584–1591. doi:10.1016/j.pss.2005.12.023
Glavin, D. P., Cleaves, H. J., Schubert, M., Aubrey, A., and Bada, J. L. (2004). New Method for Estimating Bacterial Cell Abundances in Natural Samples by Use of Sublimation. Appl. Environ. Microbiol. 70, 5923–5928. doi:10.1128/aem.70.10.5923-5928.2004
Haberle, R. M., Gómez-Elvira, J., de la Torre Juárez, M., Harri, A.-M., Hollingsworth, J. L., Kahanpää, H., et al. (2014). Preliminary Interpretation of the REMS Pressure Data from the First 100 Sols of the MSL mission. J. Geophys. Res. Planets 119, 440–453. doi:10.1002/2013je004488
Hartley, A. J., Chong, G., Houston, J., and Mather, A. E. (2005). 150 Million Years of Climatic Stability: Evidence from the Atacama Desert, Northern Chile. J. Geol. Soc. 162, 421–424. doi:10.1144/0016-764904-071
Houston, J. (2006). Evaporation in the Atacama Desert: An Empirical Study of Spatio-Temporal Variations and Their Causes. J. Hydrol. 330, 402–412. doi:10.1016/j.jhydrol.2006.03.036
Houston, J., and Hartley, A. J. (2003). The central Andean West-Slope Rainshadow and its Potential Contribution to the Origin of Hyper-Aridity in the Atacama Desert. Int. J. Climatol. 23, 1453–1464. doi:10.1002/joc.938
James, P. E. (1927). Iquique and the Atacama Desert. Scottish Geographical Mag. 43, 203–215. doi:10.1080/00369222708734550
Jung, P., Azua-Bustos, A., Gonzalez-Silva, C., Mikhailyuk, T., Zabicki, D., Holzinger, A., et al. (2021). Emendation of the Coccoid Cyanobacterial Genus Gloeocapsopsis and Description of the New Species Gloeocapsopsis Diffluens Sp. Nov. And Gloeocapsopsis Dulcis Sp. Nov. Isolated from the Coastal Range of the Atacama Desert (Chile). Front. Microbiol. 12, 671742. doi:10.3389/fmicb.2021.671742
Kieft, T. L. (2002). “Hot Desert Soil Communities,” in Encyclopedia of Environmental Microbiology. Editor G. S. Bitton (New York: John Wiley), 1576–1586.
Lester, E. D., Satomi, M., and Ponce, A. (2007). Microflora of Extreme Arid Atacama Desert Soils. Soil Biol. Biochem. 39, 704–708. doi:10.1016/j.soilbio.2006.09.020
Lettau, H., and Lettau, K. (1978). Exploring the World’s Driest Climate. Madison, Wisconsin: Center for Climatic Research, Institute for Environmental Studies, University of Wisconsin-Madison.
Levin, G. V., and Straat, P. A. (1979). Completion of the Viking Labeled Release experiment on Mars. J. Mol. Evol. 14, 167–183. doi:10.1007/bf01732376
Malin, M. C., and Edgett, K. S. (2003). Evidence for Persistent Flow and Aqueous Sedimentation on Early Mars. Science 302, 1931–1934. doi:10.1126/science.1090544
McKay, C. P., Friedmann, E. I., Gómez-Silva, B., Cáceres-Villanueva, L., Andersen, D. T., and Landheim, R. (2003). Temperature and Moisture Conditions for Life in the Extreme Arid Region of the Atacama Desert: Four Years of Observations Including the El Niño of 1997-1998. Astrobiology 3, 393–406. doi:10.1089/153110703769016460
Michalski, G., Böhlke, J. K., and Thiemens, M. (2004). Long Term Atmospheric Deposition as the Source of Nitrate and Other Salts in the Atacama Desert, Chile: New Evidence from Mass-independent Oxygen Isotopic Compositions. Geochimica et Cosmochimica Acta 68, 4023–4038. doi:10.1016/j.gca.2004.04.009
Montgomery, W., Jaramillo, E. A., Royle, S. H., Kounaves, S. P., Schulze-Makuch, D., and Sephton, M. A. (2019). Effects of Oxygen-Containing Salts on the Detection of Organic Biomarkers on Mars and in Terrestrial Analog Soils. Astrobiology 19, 711–721. doi:10.1089/ast.2018.1888
Morata, D., Féraud, G., Aguirre, L., Arancibia, G., Belmar, M., Morales, S., et al. (2008). Geochronology of the Lower Cretaceous Volcanism from the Coastal Range at the 29°20-30°s, Chile. Revista Geológica De Chile 35, 123–145. doi:10.4067/s0716-02082008000100006
Nachon, M., Clegg, S. M., Mangold, N., Schröder, S., Kah, L. C., Dromart, G., et al. (1991). Calcium Sulfate Veins Characterized by ChemCam/Curiosity at Gale Crater, Mars. J. Geophys. Res. Planets 119, 1991–2016. doi:10.1002/2013JE004588
Navarro‐González, R., Vargas, E., de La Rosa, J., Raga, A. C., and McKay, C. P. (2010). Reanalysis of the Viking Results Suggests Perchlorate and Organics at Mid Latitudes on Mars. J. Geophys. Res. Planets 115, E12.
Navarro-González, R., Rainey, F. A., Molina, P., Bagaley, D. R., Hollen, B. J., de la Rosa, J., et al. (2013). Mars-like Soils in the Atacama Desert, Chile, and the Dry Limit of Microbial Life. Science 302, 1018–1021. doi:10.1126/science.1089143
Parro, V., de Diego-Castilla, G., Moreno-Paz, M., Blanco, Y., Cruz-Gil, P., Rodríguez-Manfredi, J. A., et al. (2011). A Microbial Oasis in the Hypersaline Atacama Subsurface Discovered by a Life Detector Chip: Implications for the Search for Life on Mars. Astrobiology 11, 969–996. doi:10.1089/ast.2011.0654
Paulino-Lima, I. G., Azua-Bustos, A., Vicuña, R., González-Silva, C., Salas, L., Teixeira, L., et al. (2013). Isolation of UVC-Tolerant Bacteria from the Hyperarid Atacama Desert, Chile. Microb. Ecol. 65, 325–335. doi:10.1007/s00248-012-0121-z
Paulino-Lima, I. G., Fujishima, K., Navarrete, J. U., Galante, D., Rodrigues, F., Azua-Bustos, A., et al. (2016). Extremely High UV-C Radiation Resistant Microorganisms from Desert Environments with Different Manganese Concentrations. J. Photochem. Photobiol. B: Biol. 163, 327–336. doi:10.1016/j.jphotobiol.2016.08.017
Quinn, R. C., Ehrenfreund, P., Grunthaner, F. J., Taylor, C. L., and Zent, A. P. (2007). Decomposition of Aqueous Organic Compounds in the Atacama Desert and in Martian Soils. J. Geophys. Res. 112, 04–18. doi:10.1029/2006jg000312
Quinn, R. C., Zent, A. P., Ehrenfreund, P., Taylor, C. L., McKay, C. P., Garry, J. R. C., et al. (2005a). Dry Acid Deposition and Accumulation on the Surface of Mars and in the Atacama Desert, Chile. 36th Annu. Lunar Planet. Sci. Conf. 36, 2282.
Quinn, R. C., Zent, A. P., Grunthaner, F. J., Ehrenfreund, P., Taylor, C. L., and Garry, J. R. C. (2005b). Detection and Characterization of Oxidizing Acids in the Atacama Desert Using the Mars Oxidation Instrument. Planet. Space Sci. 53, 1376–1388. doi:10.1016/j.pss.2005.07.004
Rasuk, M. C., Kurth, D., Flores, M. R., Contreras, M., Novoa, F., Poire, D., et al. (2014). Microbial Characterization of Microbial Ecosystems Associated to Evaporites Domes of Gypsum in Salar de Llamara in Atacama Desert. Microb. Ecol. 68, 483–494. doi:10.1007/s00248-014-0431-4
Rech, J. A., Currie, B. S., Michalski, G., and Cowan, A. M. (2006). Neogene Climate Change and Uplift in the Atacama Desert, Chile. Geol 34, 761–764. doi:10.1130/g22444.1
Rech, J. A., Quade, J., and Hart, W. S. (2003). Isotopic Evidence for the Source of Ca and S in Soil gypsum, Anhydrite and Calcite in the Atacama Desert, Chile. Geochimica et Cosmochimica Acta 67, 575–586. doi:10.1016/s0016-7037(02)01175-4
Rhawn, G. J., Planchon, O., Duxbury, N. S., Latif, K., Kidron, G. J., Consorti, L., et al. (2020). Oceans, Lakes, and Stromatolites on Mars. Adv. Astron. 2020, 6959532. doi:10.1155/2020/6959532
Sánchez-García, L., Aeppli, C., Parro, V., Fernandez-Remolar, D., Chong-Díaz, M. G., Blanco, Y., et al. (2018). Molecular Biomarkers in the Subsurface of the Salar Grande (Atacama, Chile) Evaporitic Deposits. Biogeochemistry 140, 31–52.
Schulze-Makuch, D., Wagner, D., Kounaves, S. P., Mangelsdorf, K., Devine, K. G., de Vera, J. P., et al. (2018). Transitory Microbial Habitat in the Hyperarid Atacama Desert. Proc. Natl. Acad. Sci. U S A. 115, 2670–2675. doi:10.1073/pnas.1714341115
Skelley, A. M., Aubrey, A. D., Willis, P. A., Amashukeli, X., Ehrenfreund, P., Bada, J. L., et al. (2007). Organic Amine Biomarker Detection in the Yungay Region of the Atacama Desert with the Urey Instrument. J. Geophys. Res. Biogeo. 112, 04–11. doi:10.1029/2006jg000329
Skelley, A. M., Scherer, J. R., Aubrey, A. D., Grover, W. H., Ivester, R. H. C., Ehrenfreund, P., et al. (2005). Development and Evaluation of a Microdevice for Amino Acid Biomarker Detection and Analysis on Mars. Proc. Natl. Acad. Sci. 102, 1041–1046. doi:10.1073/pnas.0406798102
Somoza, R., and Tomlinson, A. (2002). Paleomagnetism in the Precordillera of Northern Chile (22°30'S): Implications for the History of Tectonic Rotations in the Central Andes. Earth Planet. Sci. Lett. 194, 369–381. doi:10.1016/s0012-821x(01)00548-9
Stern, J. C., Sutter, B., Freissinet, C., Navarro-González, R., McKay, C. P., Archer, P. D., et al. (2015). Evidence for Indigenous Nitrogen in Sedimentary and Aeolian Deposits from the Curiosity Rover Investigations at Gale Crater, Mars. Proc. Natl. Acad. Sci. USA 112, 4245–4250. doi:10.1073/pnas.1420932112
Sterzik, M., Bagnulo, S., Azua, A., Salina, F., Alfaro, J., and Vicuna, R. (2010). Astronomy Meets Biology: EFOC2 and the Chirality of Life. ESO, The Messenger 142, 25–27.
Sun, T., Bao, H., Reich, M., and Hemming, S. R. (2018). More Than Ten Million Years of Hyper-Aridity Recorded in the Atacama Gravels. Geochimica et Cosmochimica Acta 227, 123–132. doi:10.1016/j.gca.2018.02.021
Thomas, D. J., Boling, J., Boston, P. J., Campbell, K. A., McSpadden, T., McWilliams, L., et al. (2007). Extremophiles for Ecopoiesis: Desirable Traits for and Survivability of pioneer Martian Organisms. Gravit. Space Biol. 19, 91–103.
Titus, T. N., Wynne, J. J., Malaska, M. J., Agha-Mohammadi, A-A., Buhler, P. B., Alexander, E. C., et al. (2021). A Roadmap for Planetary Caves Science and Exploration. Nat. Astron. 5, 524–525. doi:10.1038/s41550-021-01385-1
Vaniman, D. T., Martínez, G. M., Rampe, E. B., Bristow, T. F., Blake, D. F., Yen, A. S., et al. (2018). Gypsum, Bassanite, and Anhydrite at Gale Crater, Mars. Am. Mineral. J. Earth Planet. Mater. 103, 1011–1020. doi:10.2138/am-2018-6346
Vítek, P., Edwards, H. G. M., Jehlička, J., Ascaso, C., Ríos, A. D. L., Valea, S., et al. (2010). Microbial Colonization of Halite from the Hyper-Arid Atacama Desert Studied by Raman Spectroscopy. Phil. Trans. R. Soc. A. 368, 3205–3221.
Vítek, P., Jehlička, J., Edwards, H. G. M., Hutchinson, I., Ascaso, C., and Wierzchos, J. (2014). Miniaturized Raman Instrumentation Detects Carotenoids in Mars-analogue Rocks from the Mojave and Atacama Desert. Phil. Trans. R. Soc. A. 372, 20140196.
Vítek, P., Jehlička, J., Edwards, H. G. M., Hutchinson, I., Ascaso, C., and Wierzchos, J. (2012). The Miniaturized Raman System and Detection of Traces of Life in Halite from the Atacama Desert: Some Considerations for the Search for Life Signatures on Mars. Astrobiology 12, 1095–1099.
Warren-Rhodes, K. A., Archer, S. D. J., Cabrol, N., Ng-Boyle, L., Wettergreen, D., Zacny, K., et al. (2019). Subsurface Microbial Habitats in an Extreme Desert Mars-Analog Environment. Front. Microbiol. 10, 69. doi:10.3389/fmicb.2019.00069
Warren-Rhodes, K. A., Rhodes, K. L., Pointing, S. B., Ewing, S. A., Lacap, D. C., Gómez-Silva, B., et al. (2006). Hypolithic Cyanobacteria, Dry Limit of Photosynthesis, and Microbial Ecology in the Hyperarid Atacama Desert. Microb. Ecol. 52, 389–398. doi:10.1007/s00248-006-9055-7
Weinstein, S., Pane, D., Ernst, L. A., Warren-Rhodes, K., Dohm, J. M., Hock, A. N., et al. (2008). Application of Pulsed-Excitation Fluorescence Imager for Daylight Detection of Sparse Life in Tests in the Atacama Desert. J. Geophys. Res. Biogeosci. 113, 01–90. doi:10.1029/2006jg000319
Wierzchos, J., Ascaso, C., and McKay, C. P. (2006). Endolithic Cyanobacteria in Halite Rocks from the Hyperarid Core of the Atacama Desert. Astrobiology 6, 415–422. doi:10.1089/ast.2006.6.415
Wierzchos, J., Davila, A. D., Sánchez-Almazo, I. M., Hajnos, M., Swieboda, R., and Ascaso, C. (2012). Novel Water Source for Endolithic Life in the Hyperarid Core of the Atacama Desert. Biogeosciences 9, 3071–3098. doi:10.5194/bg-9-2275-2012
Wilhelm, M. B., Davila, A. F., Eigenbrode, J. L., Parenteau, M. N., Jahnke, L. L., Liu, X.-L., et al. (2017). Xeropreservation of Functionalized Lipid Biomarkers in Hyperarid Soils in the Atacama Desert. Org. Geochem. 103, 97–104. doi:10.1016/j.orggeochem.2016.10.015
Wilhelm, M. B., Davila, A. F., Parenteau, M. N., Jahnke, L. L., Abate, M., Cooper, G., et al. (2018). Constraints on the Metabolic Activity of Microorganisms in Atacama Surface Soils Inferred from Refractory Biomarkers: Implications for Martian Habitability and Biomarker Detection. Astrobiology 18, 955–966. doi:10.1089/ast.2017.1705
Keywords: Atacama, desert, astrobiology, hyperarid, Mars analog
Citation: Azua-Bustos A, González-Silva C and Fairén AG (2022) The Atacama Desert in Northern Chile as an Analog Model of Mars. Front. Astron. Space Sci. 8:810426. doi: 10.3389/fspas.2021.810426
Received: 06 November 2021; Accepted: 06 December 2021;
Published: 12 January 2022.
Edited by:
André Antunes, Macau University of Science and Technology, ChinaReviewed by:
Don A. Cowan, University of Pretoria, South AfricaCopyright © 2022 Azua-Bustos, González-Silva and Fairén. This is an open-access article distributed under the terms of the Creative Commons Attribution License (CC BY). The use, distribution or reproduction in other forums is permitted, provided the original author(s) and the copyright owner(s) are credited and that the original publication in this journal is cited, in accordance with accepted academic practice. No use, distribution or reproduction is permitted which does not comply with these terms.
*Correspondence: Armando Azua-Bustos, YWF6dWFAY2FiLmludGEtY3NpYy5lcw==
Disclaimer: All claims expressed in this article are solely those of the authors and do not necessarily represent those of their affiliated organizations, or those of the publisher, the editors and the reviewers. Any product that may be evaluated in this article or claim that may be made by its manufacturer is not guaranteed or endorsed by the publisher.
Research integrity at Frontiers
Learn more about the work of our research integrity team to safeguard the quality of each article we publish.